- Departamento de Microbiología Molecular, Instituto de Biotecnología, Universidad Nacional Autónoma de México, Cuernavaca, Mexico
The Gulf of Mexico Research Consortium (Consorcio de Investigación del Golfo de México (CIGoM), 2020) was founded in 2015 as a consortium of scientific research and consulting services, specializing in multidisciplinary projects related to the potential environmental impacts of natural and human-induced oil spills in marine ecosystems, to understand and act in the case of possible large-scale oil spills in the Gulf of Mexico. CIGoM comprises more than 300 specialized researchers trained at the most recognized Mexican institutions. Among the main interests of CIGoM are developing the first baseline of the bacterial community inhabiting the southern Gulf of Mexico, investigating the natural degradation of hydrocarbons by bacterial communities and microbial consortia and identifying and characterizing industrially relevant enzymes. In this review, using third-generation sequencing methodologies coupled to function screening methodologies, we report the bacterial profile found in samples of water and sediments in Mexican regions that include the Perdido Fold Belt (northwest of Mexico), Campeche Knolls (in the southeast) and Southwest region of the Gulf of Mexico. We also highlight some examples of novel lipases and dioxygenases with high biotechnological potential and some culturable hydrocarbon-degrading strains used in diverse bioremediation processes.
Introduction
The Gulf of Mexico (GoM), formed between the early and mid-Jurassic period, is a basin for hydrocarbon fossil and gas deposits. Unfortunately, because of its uncontrolled exploitation, the GoM has been one of the most affected ocean ecosystems regarding hydrocarbon spills. One example was the spill at the IXTOC-I rig in 1979 caused by an explosion of the well in the bay of Campeche, adjacent to the peninsula of Yucatan. There, approximately 30,000 oil barrels spilled daily for 10 months, critically polluting the shores of Campeche and Veracruz. The largest oil spill in history occurred in 2010, where an oil extraction well in the Deepwater Horizon rig (DWH) (located in the GoM, approximately 41 miles off the coast of Louisiana) exploded and spilled 4.9 million crude oil barrels from the Macondo well. This massive oil spill affected the shorelines of four Gulf States of the United States—Louisiana, Alabama, Mississippi, and Florida—and caused an immediate and extreme environmental disturbance in the area, killing thousands of birds, mammals, and sea turtles. Presently, the effects of the DWH disaster persist, a recent study found that fish in the GoM continue to show evidence of contamination by polycyclic aromatic hydrocarbons (PAHs) (Pulster et al., 2020a). These catastrophic events have highlighted the imminent need for scientific studies on the GoM, enabling risk assessment and appropriate mitigation actions in the case of future oil spills.
In 2015, a research initiative called the GoM Research Consortium (Consorcio de Investigación del Golfo de México (CIGoM), 2020) was created. This organization was formed by approximately 300 researchers from the most renowned national institutions specializing in different disciplines such as Oceanography, Biology, Physics, Chemistry and Engineering. The main goal of CIGoM is to generate baseline environmental information on the GoM accompanied by the development of biotechnology processes and numeric models that will allow our country the establishment of contingency plans in the case of large-scale hydrocarbon spills.
After five years of the CIGoM foundation, we have strengthened our human capacity and technological structure in the Mexican oceanography by training dozens of new researchers. Additionally, during this period of intensive research in deep waters, with dozens of oceanographic cruises conducted, we have consolidated an environmental baseline that encompasses a wide range of biodiversity from cetaceans to bacteria inhabiting the south of the GoM, which includes the north of the Tamaulipas state to the south of the peninsula of Yucatan.
At CIGoM, we consider that the best way to protect the GoM environment is to perform periodic assessments to determine its health index. One way to determine the GoM health to identify diverse microorganisms as reporters of hydrocarbons or plastic pollution, as well as the presence of certain pathogens. Comparisons of the GoM bacterial baseline with taxonomic profiles obtained from polluted water should also be incorporated to determine the ocean health index. Using the information obtained with metagenomics, mitigation strategies that extend beyond what is commonly done in common environmental impact assessments can be proposed, where only classical microbiology techniques are used to quantify autotrophic/heterotrophic bacteria.
However, the bacterial biodiversity obtained from metagenomic data and its associated metadata provide substantial information, creating the necessity to implement databases for its analysis and visualization on specialized websites. In this paper, we describe the design and construction of a relational database and implementation of the associated computational capacity to generate a unique system, where the data of bacteria and their biogeochemical parameters in the southern GoM (sGoM) were deposited, localized, analyzed and visualized.
Previous studies that analyzed the microbial diversity in the GoM covered a relatively small fraction of the sGoM. Given the great extension of the GoM, with sharp differences in geographical characteristics and environmental variables, well-differentiated spatiotemporal patterns in the structure of the microbial communities are expected. Studies on the variation of these communities will help better understand how marine bacteria respond to environmental changes, such as those caused by hydrocarbon pollution on marine micro and macro ecosystems.
Another objective of this review is to characterize the microorganisms that inhabit sediments contaminated with hydrocarbons for potential bioremediation applications. We also seek biotechnology applications, through the search and characterization of genes and enzymes, to obtain new beneficial products involved in the degradation of hydrocarbons; these products may help manage natural disasters or disasters caused by oil exploitation. Thus, we collected sediments near oil-contaminated areas to generate genomic libraries and isolate diverse culturable strains. In both cases, we conducted functional screenings to trace essential enzymatic activities to identify specific genes involved in hydrocarbon degradation.
We believe this study sets a solid precedent in the scientific knowledge of the sGoM and its future environmental monitoring and encourages investment in multidisciplinary projects by government agencies or oil companies.
Gulf of Mexico Natural Gas and Oil Seeps
An oil seep is a fracture on the seafloor through which crude oil and natural gas leak or “seep” out of the earth and into the water; the oil flows slowly up through networks of cracks, and lighter compounds rise buoyantly to the water’s surface and evaporate or become entrained in ocean currents. Seeps are often found in places where oil and gas extraction activities are also conducted. Oil from natural seeps has a deleterious impact; as much as one half of the oil that enters the coastal environment comes from natural seeps of oil and natural gas; global estimates suggest that naturally occurring oil seeps account for approximately 47% of the oil released into the ocean environment. On average, 160,000 tons of petroleum leaks into waters surrounding North America each year. As one of the most prolific oil and gas basins in the world, the GoM has abundant natural seeps that are broadly distributed (De Beukelaer et al., 2003; MacDonald et al., 2015); an analysis identified 914 distinct seep zones that are concentrated north-to-south from the Texas-Louisiana Slope. These seeps release considerable amounts of oil and gas to the environment each year, and they are estimated to account for approximately 95% of the oil annually discharged to the GoM waters.
The GoM seeps are highly variable in composition and volume and include gases, volatiles, liquids, pitch, asphalt, tars, water, brines, and fluidized sediments. Green Canyon 600 is considered one of the most prolific natural seeps in the region (Johansen et al., 2020). When oil is released from a natural seep, it rises to the sea surface and spreads out into a thin layer, remaining visible on the surface until it weathers and disperses. Some efforts have been made to study how wind and currents affect the length of the oil slick and amount of time it remains on the surface (Shen et al., 2019). The material that flows out of the seep is still often very toxic, but some organisms that live nearby are adapted to conditions in and around seeps, using the hydrocarbons and other chemicals released as a source of metabolic energy (Hazen et al., 2010).
In the GoM, some oil deposits and natural seeps remain unexplored, and some are of special interest because they are sites of easy extraction. Experts who study the seafloor can identify the areas where there are shingles, which have gas leaks. In areas such as “El Perdido,” 57 natural seeps have been discovered. This finding is important for the oil industry and climate change because scientists can determine how much of these hydrocarbons are naturally integrated into the water column, reach the surface and then enter the atmosphere. The proposal to investigate this area came from the parastatal company PEMEX because this area is one of two places in the country with the most challenges associated with oil extraction.
Chemical Characteristics of Oil in Gulf of Mexico
Crude oils are complex mixtures comprising hydrocarbons in varying proportions and small amounts of sulfur, nitrogen, and oxygen (heteroatoms). Four main fractions comprise oil: saturated hydrocarbons, aromatic hydrocarbons, resins, and asphaltenes (Overton et al., 2016; Varjani, 2017).
Saturated hydrocarbons or aliphatic are chains of simple bonds between carbons and hydrogen, forming straight and branched chains or rings called cycloalkanes. Aromatic hydrocarbons comprise ring structures conjugated with alkyl substituents; according to the number of rings, they are classified as monoaromatic (one ring) and PAHs (more than one ring). Resins are thick viscous liquids to dark brown semi solids that can contain several aromatic rings in their molecular structures (aromatic fraction) and paraffinic chains (saturated fraction) and a higher content of heteroatoms than the aromatic fraction; they are also soluble in light alkanes (n-pentane and n-heptane) and polar solvents (methanol and toluene). Asphaltenes are the most viscous and insoluble fraction of crude oil; therefore, they are the most resistant to biodegradation. Its molecular structure comprises aromatic and heterocyclic rings with heteroatoms and some metals, among which vanadium (V) and nickel (Ni) stand out. They are insoluble in n-pentane, n-hexane and n-heptane but soluble in toluene and benzene (Castro and Vazquez, 2009; Overton et al., 2016; Varjani, 2017).
The proportions of high-molecular-weight components present in crude oil classify it as light, medium or heavy oil (Varjani, 2017). API gravity compares the densities of the different types of crude oil; thus, on a larger scale (> 10), the oil is lighter. The classification values are as follows: < 28, heavy; 28–33, medium; > 33, light (American Petroleum Institute (API), 2021).
SARA analysis fractionates crude oil into its main components (saturated, aromatic, resins and asphaltenes) and characterizes and quantifies each one (Castro and Vazquez, 2009; Overton et al., 2016). Four samples of Mexican crude oil (extra heavy, heavy, medium and light) were characterized by SARA analysis. A higher percentage of saturated fractions was found in light crude (38%) than in heavy crude (10%). A similar content of the aromatics fraction was found between light and medium crude (14.5% and 14.7%, respectively), while the content of the aromatics fraction in heavy crude was 9% of its weight and that in extra-heavy crude was 19%. The values of aromatic hydrocarbons recorded in sediments of the continental platform off Campeche are in a range of 16 to 953 μg/kg (Gracia, 2010). In the southwestern of the GoM the values of PAHs in sediment samples from depths of 500 m were measured between 13 and 60 μg/kg, while in some samples with depths < 500 m the PAHs concentrations increased (> 100 μg/kg and < 500 μg/kg) (Godoy-Lozano et al., 2018). The highest percentage of resins was 64% in the medium crude; however, the content was high in all samples starting from 41% in light crude. All the samples presented sulfur values > 1.9%. The high sulfur content is related to the higher density of the crude (Overton et al., 2016). Finally, asphaltene content was between 5% and 19%, the lowest found in light crude oil and the highest found in extra-heavy crude oil after fractionation with n-heptane; the asphaltene content was 8–36% after fractionation with n-pentane. Vanadium and nickel were the most prominent metals in all the samples, followed by calcium, iron, magnesium and copper (Castro and Vazquez, 2009).
Oil Spill History in the Gulf of Mexico
The GoM covers more than 1.5 million km2 and has over 6,000 km of shoreline; it is extremely congested with more than 25,000 miles of active oil platforms and gas pipelines. The demand for energy has increased the marine exploration of crude oil and its production and transport, as well as the risk of oil spills (National Research Council (NRC), 2003; Dalsøren et al., 2007). The two largest marine oil spills occurred in the GoM, namely, the Ixtoc-I spill in 1979-1980 and Deepwater Horizon (DWH) spill in 2010.
The Ixtoc-I Oil Spill
On December 10, 1978, PEMEX (Petróleos Mexicanos) started to drill the Ixtoc-I exploratory well approximately 80 kilometers northwest (NW) of Ciudad del Carmen in the Bay of Campeche. On June 3, 1979, the well blew out and caught fire, and the platform was destroyed, causing the oil and gas to mix with water close to the seafloor. For the 290 days that the well remained uncapped, an estimated 140 million gallons of oil were spilled into the waters of the GoM (Head et al., 2006). According to PEMEX, the Ixtoc-I disaster is considered one of the largest marine oil spills in oil history. The oil reached the ocean at a pressure of 350 kg/cm2 and a depth of 51 m, and approximately 3.4 million barrels leaked into the GoM (Jernelöv and Lindén, 1981; Soto et al., 2014). 72% of the spilled oil evaporated or sank to the sea floor, 6% washed ashore, 3% drifted to the beaches in the United States, 12% biodegraded, and approximately 7% was burned or recovered from the site (Soto et al., 2014).
The oil spill killed many species of shrimp and polluted sandy beaches, mangroves, coastal lagoons, and rivers (Jernelöv and Lindén, 1981). However, the lack of knowledge of the prespill conditions made it difficult to quantify the damage (Soto et al., 2014).
Deepwater Horizon Oil Spill
On April 20, 2010, an explosion on the Deepwater Horizon drilling rig in the GoM from the Macondo well at a depth of 1522 m led to a catastrophic oil and gas blowout, where approximately 4.9 million barrels of oil were released into the ocean, contaminating 68,000 sq. miles on the coastal zone from Texas to Florida (Diaz, 2011; Griffiths, 2012; Michel et al., 2013). The explosion and fire resulted in the sinking of the platform, death of 11 workers, and injury of 17 workers. The blowout caused a massive offshore oil spill in the GoM, considered the largest accidental marine oil spill worldwide and the largest environmental disaster in United States history (Goldstein et al., 2011). After several attempts to stop the flow, the well was capped on July 15, 2010 and declared sealed on September 19, 2010. A massive cleanup, restoration, and research program followed and is ongoing, mostly funded by BP Exploration and Production, Inc. (BP).
Oil dispersant chemicals are used to break up oil into smaller droplets, leading to enhanced bacterial degradation and bioavailability of oil because small oil droplets are more prone to degradation by physical and microbial processes; however, the movement of oil during the spill is controlled by surface currents depending on ocean circulation. During the cleanup efforts, millions of gallons of dispersants were used in the DWH spill to break up the crude oil (Biello, 2010); thousands of workers and volunteers participated during the cleanup activities and were exposed to the toxicological properties of the oil components and chemicals used to break up the oil, resulting in health risks (Peres et al., 2016). The long-term illness effects among the participants were evaluated 7 years after exposure and included impaired hematological, hepatic, pulmonary and cardiac functions. Higher rates of reproductive failure, heart issues, lung disease in sea animals and impaired lung and heart function among cleanup workers and United States Coast Guard personnel who contacted the oil have been observed (D’Andrea and Reddy, 2013; D’Andrea and Reddy, 2018).
Ten years after this disaster, many species, such as deep-sea corals, dolphins, and turtles, are still struggling; however, some other populations have shown robust recovery. Pulster et al. conducted one of the most comprehensive baseline studies of PAH exposure in fishes for a large marine ecosystem. They implemented Gulf-wide fish surveys extending over seven years (2011–2018). In total, 2,503 fishes, comprising 91 species, sampled from 359 locations were evaluated for biliary concentrations of PAH. They reported liver PAH concentrations were the highest where the DWH oil spill occurred (Pulster et al., 2020a, b).
Gulf of Mexico Research Consortium (And Other Consortia)
The study of oceans, ecosystems, and biological and non-biological resources is addressed holistically by different scientific disciplines. Research consortia create multi and interdisciplinary efforts that allow understanding of this complex global system to develop mutual strategies for solving problems and the sustainable use of the resources of the planet (Dañobeitia et al., 2020). Additionally, consortia contribute to the generation of knowledge, promote research, participate in specialist training and the development and innovation of new technologies and elaborate scientifically sound strategies in decision making. At the same time, consortia provide support to coordinate and integrate solutions in synergy among academia, government, society and industry (OceanObs, 2019; Dañobeitia et al., 2020; Rotter et al., 2020).
Marine research consortia have been formed worldwide. However, because of the vast expanse of the oceans, the area of study is defined by each consortium, which determines its priorities and objectives. In Europe, the study of oceans is conducted via an international coordination approach with the participation of European Union member countries and is grouped into infrastructures, consortia, groups, or research networks to monitor the sea and predict the marine environment (Dañobeitia et al., 2020; Rotter et al., 2020). In the United States, national consortia monitor the Pacific Ocean, Atlantic Ocean and GoM, which are permanently exposed to oil exploration and exploitation activities. Because of the DWH event and lack of previous information on the general state of the GoM, specialized research consortia were created whose objectives are based on understanding the extent of damage caused by oil spills. The (Gulf of Mexico Research Initiative (GoMRI), 2020) was formed after DWH with funding from BP to create a broad and independent research program mainly in research institutions in the states of the GoM coast in the United States. In GoMRI, 12 consortia, including ECOGIG (Ecosystem impacts of Oil and Gas Inputs to the Gulf (ECOGIG), 2020) and CSOMIO (Consortium for Simulation of Oil-Microbial Interactions in the Ocean (CSOMIO), 2020), measure the movement, destination and dynamics of oil in the GoM, as well as study the microorganisms involved in the degradation of hydrocarbons and their response to the spill. C-IMAGE (Center for Integrated Modeling and Analysis of Gulf Ecosystems (C-IMAGE), 2020) focuses on establishing the ecological baseline of the GoM, predicting the long-term fate and degradation of oil using sediment cores from Campeche, Mexico, of IXTOC-I spill zone as a study model. For their part, CONCORDE (Consortium Coastal River-Dominated Ecosystems (CONCORDE), 2020) and ADDOMex (Aggregation and Degradation of Dispersants and Oil by Microbial Exopolymers (ADDOMex), 2020) perform studies on the effect of oil dispersants on the ecosystems of the GoM.
Because of the possibility of another oil catastrophe in the Mexican territory of the GoM, where the oil industry of the country is located, the Gulf of Mexico Research Consortium (Consorcio de Investigación del Golfo de México (CIGoM), 2020) was created in 2015. CIGoM is a multidisciplinary research group with more than 300 Mexican researchers from different scientific disciplines from national and international research institutes, government agencies and the private sector. CIGoM developed the project “Implementation of oceanographic observation networks (physical, geochemical, and ecological) for generating scenarios in case of possible contingencies related to the exploration and production of hydrocarbons in deep waters of the GoM” to study the movement and persistence of hydrocarbons in the different marine strata, ranging from the water column to sediments; to evaluate biological responses such as adaptation to hydrocarbon exposure and their natural degradation; and to determine the baseline of the GoM in case of another spill.
CIGoM has conducted oceanographic research cruises from Tamaulipas to Yucatan to obtain water samples from different depths and sediments. This is one of the largest scientific projects in Mexico and perhaps the most important for oceanography in the country. Observation and study of the ocean help create plans, models and forecasts to mitigate the risk of potential disasters (Visbeck, 2018; OceanObs, 2019). CIGoM projects are focused on generating knowledge of the impact of the oil industry, as well as on developing contingency plans and action strategies against possible oil spills and are organized into five tasks of action: (1). Oceanographic observation platforms; (2). Baseline and environmental monitoring; (3). Circulation models and biogeochemistry; (4). Natural hydrocarbon degradation; and (5). Spill scenarios.
In this review, we will address lines 2 and 4, which involve the study of bacteria. In the next section, we focus on the baseline of bacteria of the GoM.
Bacterial Diversity From the Southern GoM
The statement “Every gene is everywhere but the environment selects” (Fondi et al., 2016) reflects the idea that the distribution of microorganisms on the planet is dependent on particular environmental conditions. Oceans are not an exception, harboring species adapted to conditions such as low temperatures, high salinity, and atmospheric pressure. Additionally, marine environments have microenvironments that increase microbial diversity. Although it is difficult to define spatial boundaries delimiting the water column, physical, chemical, and geographical features outline the dynamic and composition of marine zones. The cruises conducted along the CIGoM project sampled zones that covered water from the epipelagic to the bathypelagic zones. These samples were arranged considering their physical, chemical, or depth parameters into zones of maximum fluorescence (MAX), minimum oxygen (MIN), 1,000-m depth at the Antarctic Intermediate Water (AAIW) and bottom water (DEEP) samples, which included water collected between 550 and 3,200 m in depth. In CIGoM’s project, we conducted 10 oceanographic cruises and collected samples from which bacterial DNA was extracted to perform metagenomic analysis based on 16S amplicon sequencing of the water column and sediments. The sampling covered several regions, denominated as follows: Deep Southwest Gulf, Deep Water Zone, Perdido (Northwest Gulf) and the Yucatan Platform covering the area from Tamaulipas to the Yucatan platform (86° W, 18° N to 97° W, 26° N; Figure 1).
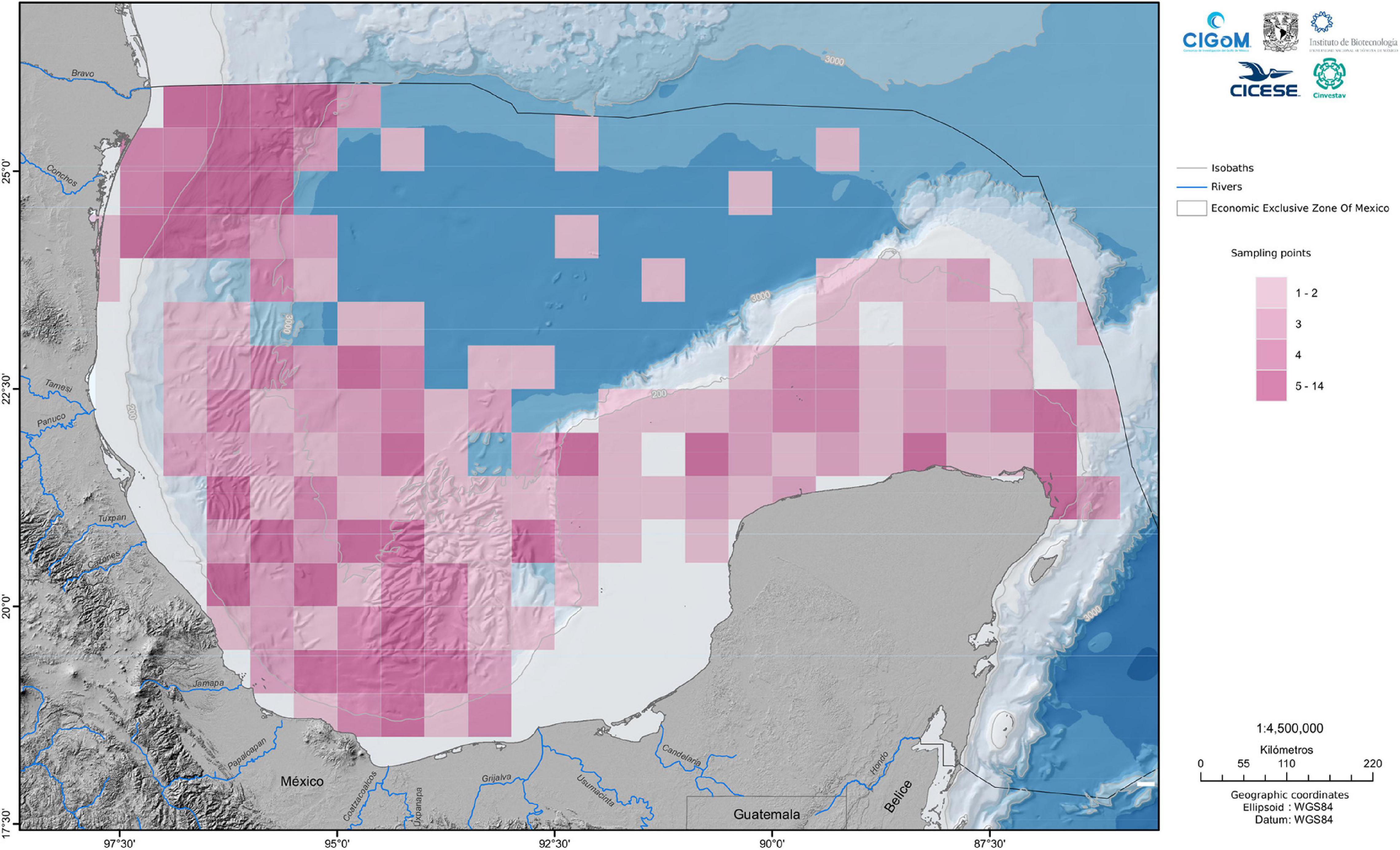
Figure 1. Sampled sites: We illustrated the sample sites of the campaigns performed from March 2015 to September 2017. The data were grouped in a grid, in cells of 0.5 degrees of latitude ×0.5 degrees of longitude.
In this review, we present the published data because some regions are still under analysis for independent publications, delving into the bacterial diversity of the areas explored in the entire project. To analyze variations in bacterial communities across the area under study, we estimated taxa abundance at the genus level, as described later in the text. For water samples, the percentage of sequences to which genera could be assigned was 84.4%; for sediment samples, this percentage was 79.4%. Given the great spatial and temporal extension of the sample collection, many sampling points were obtained. Data from the samples were grouped in a grid, with cells of 0.5 degrees of latitude × 0.5 degrees of longitude. Thus, the estimated genus abundances calculated as described in the following section, were averages over the sample points within a given cell.
The explored zones, similar to other areas of the Mexican exclusive economic zone along the GoM, termed the sGoM throughout, are poorly explored ecosystems in terms of their microbial diversity. Some previous studies have identified the bacterial abundance of a few areas (Lizárraga-Partida et al., 1986, 1991), but a larger study showing the bacterial diversity and abundance in the sGoM from Tamaulipas to Yucatan is lacking. Thus, with the support of the Hydrocarbon Fund (SENER/CONACyT), oceanographic cruises were conducted by three institutions: Ensenada Center for Scientific Research and Higher Education (CICESE), Centre of Investigation and Advanced Studies of the National Polytechnic Institute, Merida (CINVESTAV-IPN) and National Autonomous University of Mexico with the participation of Institute of Biotechnology (IBt-UNAM) and Institute of Marine Sciences and Limnology (ICMyL-UNAM). From the genetic material extracted from both the water column and sediments, we created the first baseline of endemic bacteria from the sGoM that is stored with other data as part of an information system, described in more detail below.
Metagenomics of the sGulf of Mexico
The methods to identify the oceanic environmental bacteria of the sGoM follows the strategies represented in Figure 2. These strategies were based on DNA extraction from samples collected from the water column and sediments based on the amplification of the V3-V4 16S rRNA gene variable regions, as described in Godoy-Lozano et al. (2018) and Raggi et al. (2020). The amplicon libraries for both water and sediments were constructed as described in the 16S Metagenomic Sequencing Library Preparation protocol from Illumina and sequenced on the Illumina MiSeq platform with a paired-end read configuration of 600 (Godoy-Lozano et al., 2018) or 300 cycles (Raggi et al., 2020). In both sample types, reads passing the QC filters (read quality = Q20) were used to rebuild the original amplicon region (450- to 490-bp length) by overlapping them using Flash v1.2.7 software (Magoč and Salzberg, 2011), and all non-overlapping sequences were discarded. For the sediment samples reported in Godoy-Lozano et al., 2018, the taxonomic classification was performed using Parallel-meta pipeline v2.4.1 (Su et al., 2014) against Metaxa2 database v2.1.1 (Bengtsson-Palme et al., 2015) as described in Escobar-Zepeda et al. (2018). This pipeline was used for all the collected samples, and abundance matrices at the genus taxonomic level were used to calculate the Good’s coverage and alpha diversity indexes—i.e., the Chao 1 and Shannon indexes—as described in Godoy-Lozano et al. (2018). The calculated abundance matrices were then used to populate the “Information system of bacterial diversity in the southern Gulf of Mexico,” described in the next section.
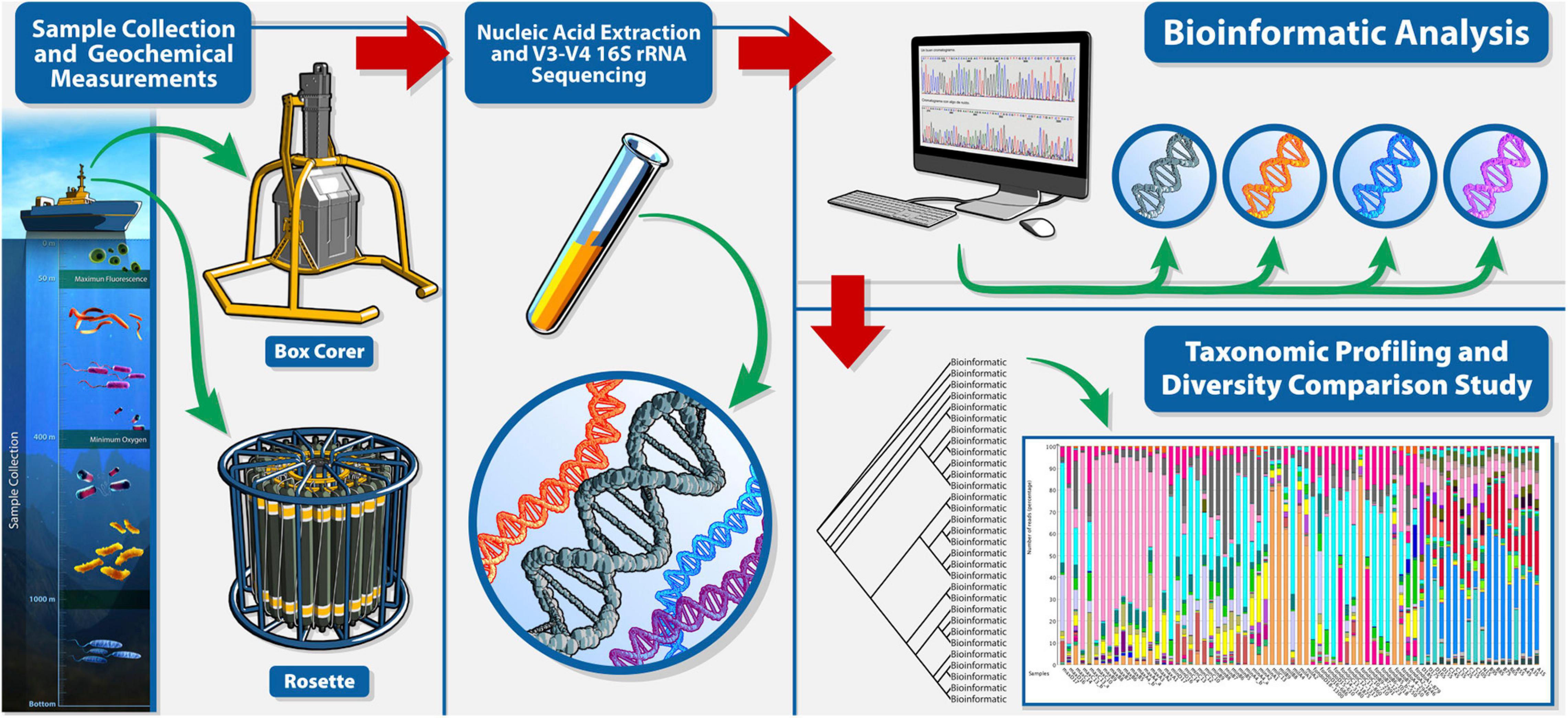
Figure 2. Data collection and workflow analysis. Representation and general workflow for sample collection, sequencing, and taxonomic assignment.
Information System of Bacterial Biodiversity in the Gulf of Mexico
In the marine science community, standards and protocols for sampling are available (Glover et al., 2015; Clark et al., 2016; Rabone et al., 2019); however, details regarding the handling of marine samples and genetic data are not often openly available and shared with the community. In CIGoM, we tried to apply best practices to achieve marine bacterial samples and data collection. All of our data are associated with metadata information—e.g., the date the sample was collected, its geographical localization, depths at which the water column and sediment were sampled, and the types of analyses performed for each sample. Bacterial taxonomy and the metabolic potential predicted for the sGoM were compiled in a MySQL database, organizing the predictions and metadata in reference tables. The database can be easily consulted using a developed web page, allowing exploration of the relative abundances and hierarchies found in metagenomic classifications using Krona tools (Ondov et al., 2011). The web tool also displays georeferenced maps, allowing exploration of the relative abundances along the different sample regions. BLAST searches can be performed using the sequences derived from the shotgun functional annotations. When published, the tool will be the first system capable of exploring metagenomic information related to the microbial diversity and metabolic potential of the sGoM. This system may complement other tools such as the GROS database, which gathers sequenced genomes with hydrocarbon-degrading capabilities (Karthikeyan et al., 2020).
The information system comes from different sources, as shown in Figure 3:
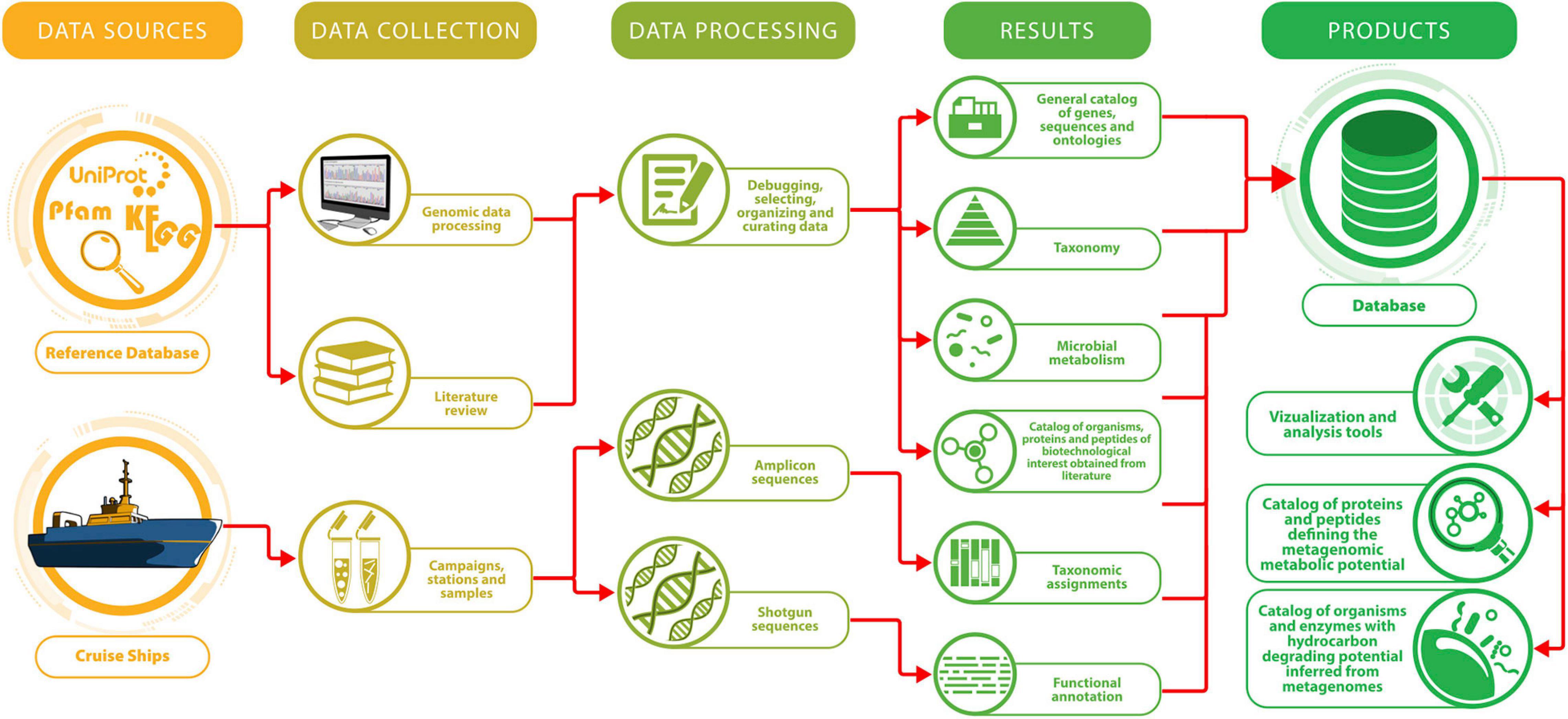
Figure 3. Metagenomic information System of the Gulf of Mexico. Workflow showing the followed steps for data and sample collection, processing and storage.
• Data collected in oceanographic campaigns.
• Information from references in genomic databases.
• Data collected from specialized literature.
The system is divided into five modules. The BLAST module identifies regions of similarity among biological sequences. The program compares a nucleotide or protein sequence of interest with sequences stored in a database constructed from metagenome shotgun sequences. The taxonomy module displays the taxonomic classifications inferred from amplicons. Currently, the taxonomic assignment results are available for 441 samples. Our database has a graphical submodule showing the bacterial relative abundances or assigned taxonomic diversity in georeferenced thematic maps. In the matrix module, the user can find the taxonomic distribution by depth and campaign, displayable in the Krona hierarchical tool (Ondov et al., 2011). In the list module, the user may consult all metagenomic products as Excel-type tables, containing detailed information concerning the processing, origin of the sample and characteristics such as geographic coordinates and depth. In the metabolism module in our database, we include information on enzymes, metabolic pathways and chemical reactions to relate them to the functional assignment derived from shotgun sequencing. The database also includes in this category a table of enzymes with hydrocarbon-degradation potential. This information has been curated through a meticulous process for which we developed quality parameters and is associated with bibliographic references linked to PubMed1 and KEGG2 databases.
The organization of the data collected helped identify more accurately the hydrocarbon-degrading bacteria present in the sampled areas. In the following sections, we present a description and comparison of the more abundant bacterial taxa found in the water sample and sediments, followed by our findings and characterization related to hydrocarbon-degrading bacteria.
Bacterial Taxonomy in the Sediments From the Southern GoM
The sediment collection presented in this review covered the Deep Southwest Gulf, Perdido Fold Belt (Northwest Gulf), and Southeast Bay of Campeche; however, the sediments of the Yucatan Platform were also explored (manuscript in preparation). The superficial sediments (≤ 10 centimeters in depth) were collected using a box corer (Figure 2). The observed taxonomy was diverse, as previously reported (Hoshino et al., 2020); however, similar to sediments from oil-polluted sites (Head et al., 2006), hydrocarbon degraders were also abundant in our samples. Sequencing reflects the presence of bacteria and archaea; however, because we were focused on collecting bacteria, the relative abundance was greater. The bacterial baseline of the sediments includes 3155 genera grouped in 917 families; however, some genera are highly represented. The sixteen predominant genera shown in Figure 4 are Thioprofundum, Rhodovibrio, Pseudomonas, Desulfovibrio, Colwellia, Desulfonatronum, Cycloclasticus, Phycisphaera, Dehalogenimonas, Geoalkalibacter, Nitrospira, Marinobacter, Alcanivorax, Desulfovirgula, Pelobacter and Spongiispira, some of which are also well distributed in other oceans, as reported by Godoy-Lozano et al. (2018). Some of these genera have oil-degradation capacities, such as Pseudomonas, Alcanivorax, Cycloclasticus, Marinobacter, and Pelobacter (Prince, 2010), Rhodovibrio found in the sea surface and sediment in the northern GoM after the Deepwater Horizon oil spill (Liu and Liu, 2013), and Colwellia, a PAHs degrader also found after the Deepwater Horizon oil spill (Gutierrez et al., 2013). These genera, as shown in Figure 4, are distributed throughout almost the entire region under study. However, differences were found among genus abundances for the elements of the grid (cells) situated south or north of 23.5°N. In particular, the absence of Colwellia in cells situated to the north of this latitude and a region comprising three cells situated roughly at 24.5° N, in which the genera Marinobacter, Alcanivorax and Cycloclasticus are predominant, is remarkable.
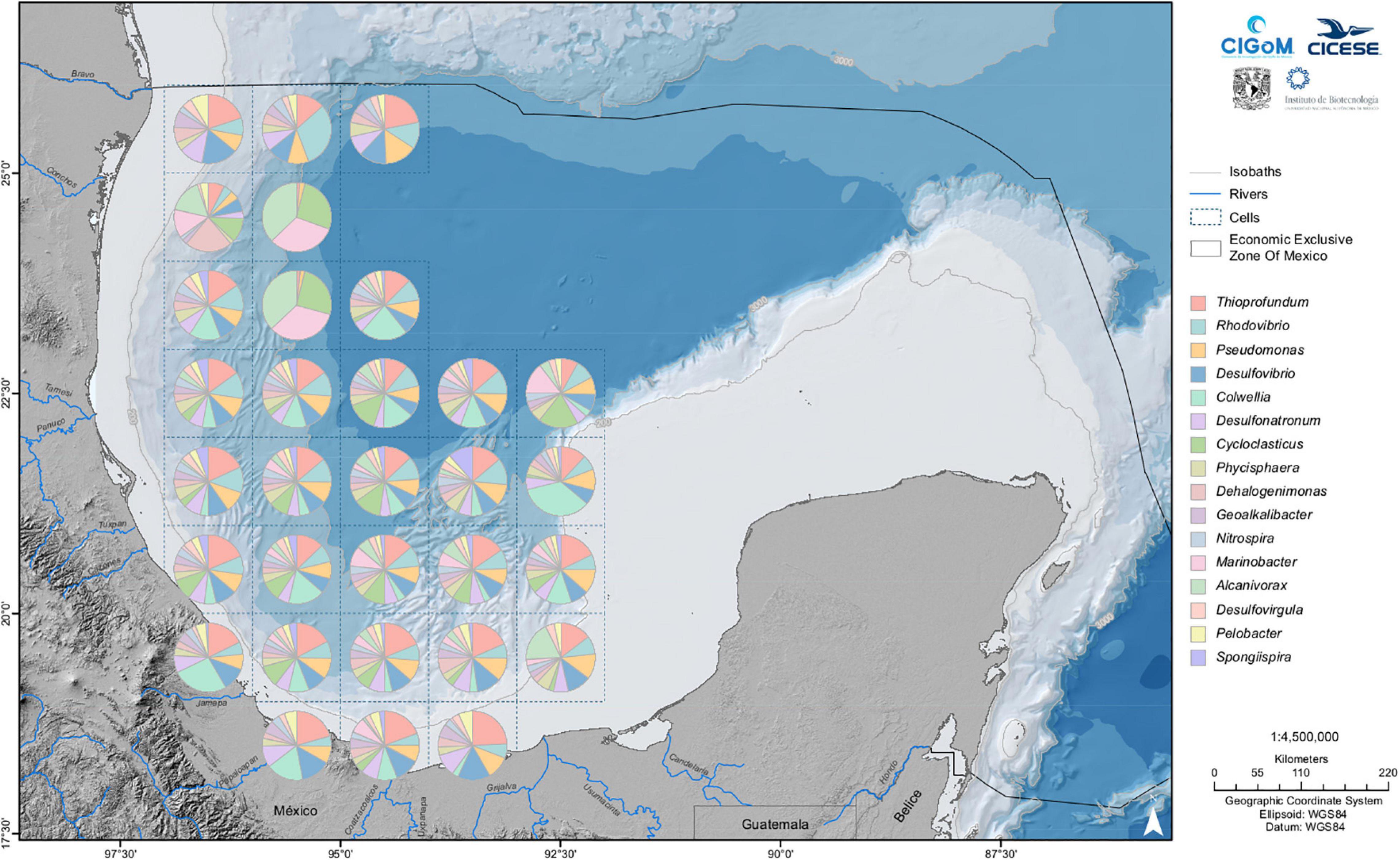
Figure 4. Sixteen most abundant genera in the superficial sediments of the Gulf of Mexico. The map covers a rectangular area with the bottom right corner at 18° N, 86° W to top left corner at 97° W, 26° N. The superficial sediments range from 12 to 3741 m.
The region of Perdido comprising sampled sites located between 26° N and 24° N, as shown in Figure 4, presents a higher abundance of bacteria of the genera Thioprofundum, Rhodovibrio, and Pseudomonas in the samples between 26° N and 25° N, contrasting with those between 25° N and 24°, where species of the genera Marinobacter and Cycloclasticus prevail. Remarkably, Thioprofundum, prevailing in all samples, is more abundant in this area, particularly in the deep zone. Also notable is the presence of members of the HDB Alcanivorax in this region.
The Deep Southwest Gulf holds samples from 1000 meters onward; those from 23.9° N to 19° show a high proportion of Thioprofundum, Rhodovibrio, and Pseudomonas, as observed in other sampled sites. However, representatives of the genus Colwellia and Spongiispira are also highly abundant, contrasting with the Perdido Fold Belt samples. The Southeast Bay of Campeche has a similar distribution to that in the Deep Southwest Gulf, with Thioprofundum Desulfovibrio and Colwellia prevailing in this region.
The bacterial diversity observed in the SW GoM sediments and that from sediments from distinct regions worldwide, showed a fingerprint that could be considered the baseline that describes the bacterial richness of unperturbed sediments with high hydrocarbon concentrations. This fingerprint is defined by a set of Gammaproteobacteria of the genera Oceaniserpentilla (absent in the DWH sediments), Gammaproteobacterium PS12-4, a psychrophilic bacterium, Blastococcus and Methylohalobius (Godoy-Lozano et al., 2018). This study also reveals that bacteria related to the genus Thioprofundum are also widely distributed in the Mexican exclusive economic zone and have potential applications in ecological surveillance.
Using non-metric multidimensional scaling analysis, Godoy-Lozano’s study compared the sequenced amplicons from 5 samples collected 4–5 months after the DWH in the northeastern GoM (NEGoM) with the sediments collected at the SWGoM. This comparison revealed differences in the sample composition and diversity. Our results showed one cluster holding the NEGoM and the second cluster grouping the SWGoM samples collected by our project. Despite these differences, it is remarkable that some hydrocarbon degraders, such as Haliea, Reinekea, Colwellia, Fodinicurvata, Rhodovulum, Thiohalomonas, Pseudomonas, Thiohalophilus, and Rhodovibrio, were abundant in all the analyzed samples. These observations provide evidence suggesting that the bacterial population present in the GoM are adapted to the ubiquitous presence of hydrocarbons.
Another study performed by our group and reported by Raggi et al. (2020), across the southeast (SE) and northwest (NW) of the GoM, showed that organic material discharged by the Laguna Madre, Bravo, Soto La Marina and Pánuco rivers contributes to the accumulation of a complex mixture of organic compounds in marine sediments, making it impossible to distinguish a specific profile between the NW and SE regions. However, significant differences for deep and shallow sediments were observed. In this region, taxonomic diversity was found for members of the classes Deltaproteobacteria (12–35%), Gammaproteobacteria (2–24%), Alphaproteobacteria (1–20%) and Dehalococcoidia (0.3–17%). However, some genera with sulfur metabolism and hydrocarbon degraders were also present in the Wb1-A12 (0.1–10%), Urania-1B-19 (1–6.8%), Nitrospira (0.3–3.5%), and Sva0081 (0–4.8%) sediment groups and in the newly reclassified NC10 class (0.1–10%) and Woeseia (0.7–6.8%) (Du et al., 2016; Bacosa et al., 2018a).
Bacterial Taxonomy of the Water Column in the Southern GoM
The water column covers regions from Perdido (Tampico) to Campeche Bay (see Figure 5). The genetic material was collected along 3 depths—that is, the epipelagic (0–100 m), mesopelagic (100–700 m) and bathypelagic (1,000 to 4,000 m) zones—for which the specific depths were measured using an electronic instrument that measures the conductivity, temperature, and depth (CTD), as well as the physical and chemical variables. The collected variables allow establishment of a zone of maximum fluorescence and minimum oxygen. Samples from near the seabed were also collected.
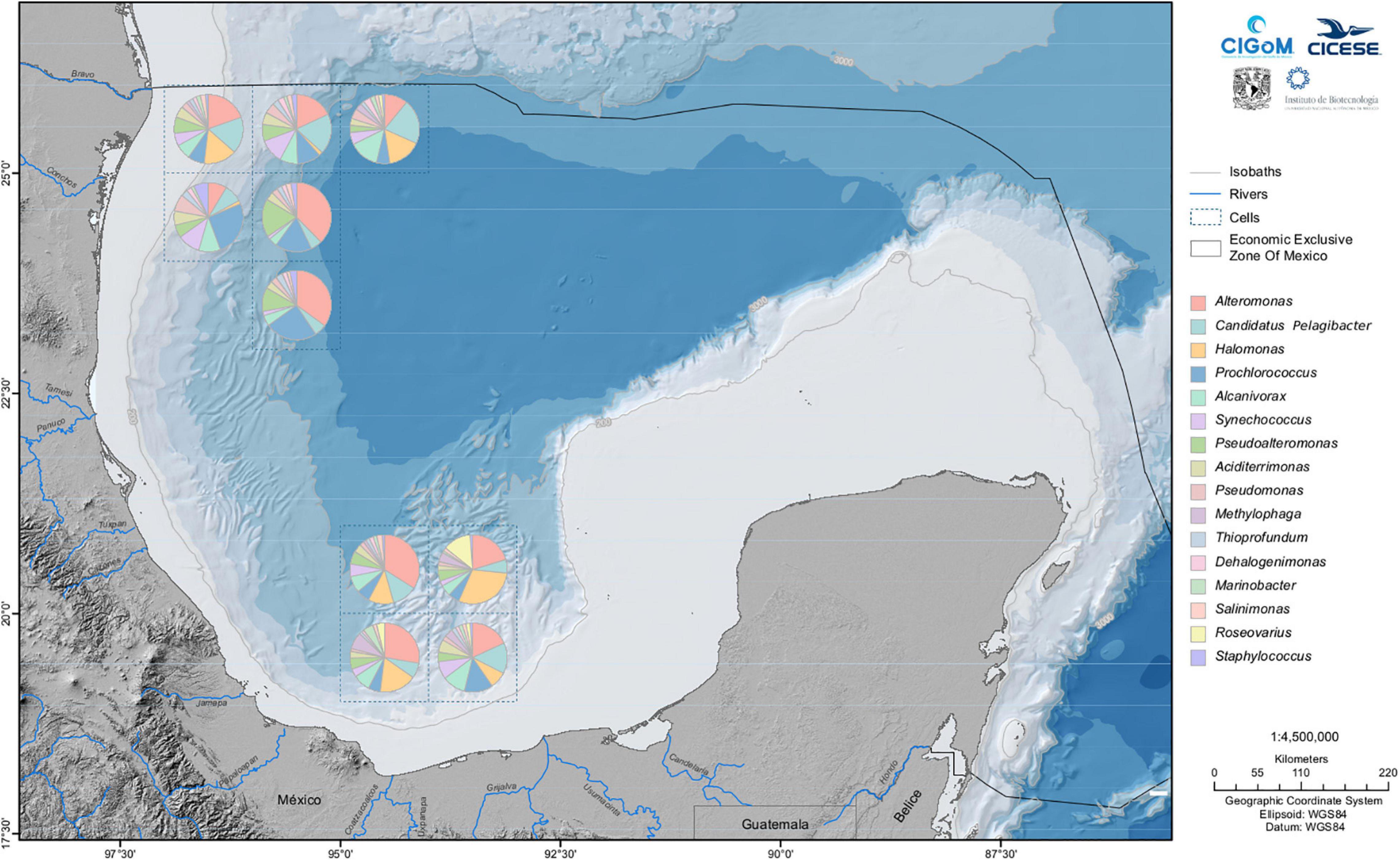
Figure 5. Sixteen most abundant genera on the water column of the Gulf of Mexico. The map covers a rectangular area with the bottom right corner at 18° N, 86° W to the top left corner at 97° W, 26° N. The water column depth ranges from superficial water to 3721 m from near the bottom.
The zone of maximum fluorescence (epipelagic zone) showed the presence of cyanobacteria, which are ubiquitous and abundant components of the marine microbiota. To date, the genera Prochlorococcus and Synechococcus dominate photoautotrophic picoplankton over vast tracts of the oceans worldwide. Both genera found to be abundant in the explored zone in the GoM occupy a key position in the ocean as the base of the marine food chain and contribute significantly to global primary productivity (Scanlan and West, 2002; Biller et al., 2015). Our metagenomic studies using shotgun sequencing also showed the presence of enzymes involved in photosynthesis (Raggi et al., 2020). Additionally, other studies performed by CIGoM researchers evaluated the relationship between the carbon distribution of Prochlorococcus (PRO) and loop current (LC) dynamics during the summer, finding that, on average, approximately half of the total depth-integrated carbon biomass of picoplankton was attributed to heterotrophic bacteria (HB; 54%) and three autotrophic populations (Prochlorococcus, Synechococcus, and pico-eukaryotes; 46%) (Linacre et al., 2019), a result that correlates well with our abundance observations.
Prochlorococcus and Synechococcus were not the predominant genera; instead, we found Alteromonas as the most abundant genus (Figure 5). Alteromonas was one of the first described marine genera. Since that initial report, all the subsequent species identified in this genus have been marine species. An important feature defining Alteromonas is that these species have been isolated from samples collected in seas contaminated with petroleum hydrocarbons, such as the SN2 strain capable of metabolizing aromatic hydrocarbons (Math et al., 2012). Another species, Alteromonas strain TK-46, that became enriched in sea surface oil slicks during the DWH spill, contributed to the formation of marine oil snow (MOS) and/or dispersion of the oil (Gutierrez et al., 2018). The presence of this genus as one of the most dominant in our samples in the water column as well as the presence of Pseudoalteromonas, both producers of extracellular polymeric substances (EPS) which are a major component of the total DOM pool in the ocean (Gregson et al., 2021), suggest that members of these genera may contribute to dispersion of the oil, if a spill occurs.
Another abundant genus was Candidatus Pelagibacter, belonging to the group of bacteria known as SAR11 (Pelagibacterales), which was one of the first groups to be described from environmental samples using 16S gene sequencing (Giovannoni, 2017). Regarding its ability to proliferate in polluted oil hydrocarbon environments, studies on samples derived from the spill associated with the Deepwater Horizon platform showed that the abundance of this bacterium decreases in the presence of dispersants, oil and light (Bacosa et al., 2015). By contrast, bacteria from the genera Marinobacter, Alcanivorax, Pseudomonas, Pseudoalteromonas (Prince et al., 2010), Halomonas (Cai et al., 2019), Methylophaga (Gutierrez and Aitken, 2014), Dehalogenimonas (Looper et al., 2013) and Roseovarius (Chronopoulou et al., 2015) are oil degraders. Among these genera, Halomonas was identified in surface water samples and in deep hydrocarbon plumes formed during the active phase of the DWH spill (Gutierrez et al., 2013). The analysis performed in our group by Raggi et al. (2020) reported different abundance percentages of Halomonas in the water column of the exclusive economic zone of the sGoM, confirming that these species inhabit different depths along the explored zone. Sample analysis showed amplicons that share homology with representatives of the genus Methylophaga characterized as halophilic, methylotrophic bacteria isolated from diverse marine environments (Garrity et al., 2004). For several years, analysis of the contribution of Methylophaga in hydrocarbon degradation was controversial; however, recent findings have also shown that methylotrophs, including Methylophaga, were in a heightened state of metabolic activity within oil plume waters during the active phase of the spill.
Other less abundant genera, as shown in Figure 5, are Aciditerrimonas, Salinimonas, Roseovarius, and Staphylococcus. The genus Aciditerrimonas belonging to the order Acidimicrobiales, as well as the other four genera in the group, are obligate acidophilic bacteria, all of which oxidize ferrous iron or reduce ferric iron and contain meso-diaminopimelic acid in their peptidoglycan (Rosenberg et al., 2014). The only member of Aciditerrimonas, Aciditerrimonas ferrireducens JCM 15389, was isolated from solfataric soil samples at Ohwaku-dani in Hakone, Japan. These solfataric soils are geothermally heated areas associated with fumaroles emitting sulfurous gases containing H2S and SO2 (Itoh et al., 2011). The shotgun metagenomic analysis performed by our group (Raggi et al., 2020) showed the presence of genes related to sulfur oxidation in Proteobacteria, suggesting the presence of sulfur-related compounds in this area. The marine species of the genus Salinimonas—Salinimonas chungwhensis and Salinimonas lutimari—were isolated from a solar saltern in the Chungwha area in the Yellow Sea in Korea and from a tidal flat sediment on the southern coast of Korea, respectively. The latter shows degradative activities against several polysaccharides (Rosenberg et al., 2014), but no hydrocarbon-degradation activity has been reported for the genus. Finally, Escobedo-Hinojosa and Pardo-López (2017) analyzed bacterial metagenomes from the southwestern GoM for pathogen detection, identifying Staphylococcus as one of the pathogens abundant in this zone. Interestingly, groups of predominant genera in the water column and sediments show little intersection. The genera appearing in both groups are Pseudomonas, Marinobacter, Alcanivorax and Thioprofundum.
Contrasting Bacterial Diversity From the Water Column and Sediments
As described above, the water column’s bacterial community composition is different from that of the sediment. Moreover, the calculated alpha diversity described in Raggi et al. (2020) showed that the total number of observed OTUs from both Perdido and Campeche sampling sites varies between 1,589 and 6,170 in the case of the water column samples and from 6,099 to 33,740 in sediment samples, indicating important differences among the bacterial abundances. In the same study, a Bray–Curtis distance matrix was generated, observing that the sediment samples segregate from the water samples into a single, separated cluster. In-depth inspection of the results showed that the sampled sediments from the deep and shallow are significantly different. Nevertheless, it was not possible to observe the differences between the NW and SE regions. Similar observations were also described by Sánchez-Soto Jiménez in Sánchez-Soto Jiménez et al. (2018), who performed sampling two years prior, in April 2014, in the Mexican region of the Perdido Fold Belt. These superficial sediments from 20 to 3,700 m showed an OTU richness and diversity higher in the shallow sediments (20–600 m) than in the samples from deep sediments (2,800–3,700 m), indicating important similarities between the studies. Remarkably, the differences between the shallow and deep sediments found by Sánchez-Soto Jiménez, correlated well with depth, redox potential, sulfur concentration, and grain size (lime and clay). Particularly, some genera such as Alcanivorax, Shewanella, Marinicella and ZD0117 showed oxidizing conditions in deep sediments. The map presented in Figure 4, shows enrichment of Alcanivorax in the deep sediments analyzed in the CIGOM project.
A hallmark shaping the structure of the bacterial community found in the water column in the nGoM is mainly the depth, being likely to result from differences in temperature, dissolved oxygen, and suspended particles (King et al., 2013). The data collected by Raggi et al. (2020) also showed differences in some water column parameters, such as oxygen, fluorescence, temperature, and salinity, surely responsible for the enrichment of genera that shape the bacterial community of the sGoM. For example, the map presented in Figure 5 shows that Prochlorococcus and Synechococcus were enriched in different proportions in the sampled sites. These results suggest gradients of light, temperature, and nutrients in the euphotic zone that affect the community members’ distribution. In other works, physicochemical parameters’ role was also tested, determining their influence in the selection of oil degraders through a series of incubation experiments (Bacosa et al., 2015; Liu et al., 2017).
The organization of the data collected helped identify more accurately the hydrocarbon-degrading bacteria present in the sampled areas. In the following section, we present a general description of the hydrocarbon-degrading bacteria in the GoM and emphasize the bacteria reported by CIGoM.
Hydrocarbon-Degrading Bacteria
Oceans contain many diverse microorganisms capable of metabolizing several compounds, including hydrocarbons from petroleum and transforming them into compounds that are less toxic to the environment. These microorganisms include hydrocarbon-degrading bacteria (HDB), which specialize in the biodegradation of hydrocarbons in contaminated marine waters, where the exploitation of oil fields has caused excessive release of hydrocarbons into the environment. Thus, in a spill, HDB represent the first line of defense against contamination because they spread rapidly and become dominant species in the microbial community (Head et al., 2006; Yakimov et al., 2007; Ron and Rosenberg, 2014; Cerqueda-García et al., 2020).
Crude oil varies from one site to another, generating differences in chemical and physical properties that affect its susceptibility to biodegradation. The preference for different types of oil is related to the increase or decrease in the genera of HDB and influences the structure of the microbial community. Environmental factors and the combination of these also influence and shape the bacterial community and the presence and abundance of HDB, such as temperature, nutrients, salinity, pressure, sunlight, pH, oxygen availability and depth (Lizárraga-Partida et al., 1982; Head et al., 2006; Yakimov et al., 2007; Das and Chandran, 2011; Kimes et al., 2014; Liu et al., 2017; Godoy-Lozano et al., 2018; Sánchez-Soto Jiménez et al., 2018; Bacosa et al., 2018b). Although environmental factors play a central role in the community of HDB, many microorganisms are able to degrade hydrocarbons under both aerobic, throughout the marine water column (Prince et al., 2013) and anaerobic conditions like in anoxic sediments, within hydrocarbon seeps (Head et al., 2014) and also at depths of 2000–5000 m.
In marine environments, temperature influences the fate of crude oil, affecting its physical properties and bioavailability. Temperature also affects the composition of the HDB community (Bacosa et al., 2018b). Liu et al. (2017) reported that bacterial genera such as Cycloclasticus, Pseudoalteromonas, Sulfitobacter and Reinekea had greater abundance at 4°C, while Oleibacter, Thalassobius, Phaeobacter, and Roseobacter increased when they were cultured at 24°C. Besides, the alkanes were degraded faster at 24°C while the concentration of PAHs decreased faster at 4°C. In the water column of the sGoM (50 m to 3,200 m depth), temperatures range from 21.7 to 4.3°C in the Perdido area and 24.86 to 4.38°C in the Campeche area. Alteromonas and Alcanivorax were detected at different depths in the water column (Raggi et al., 2020), perhaps because they developed well at both 4°C and 24°C (Liu et al., 2017).
Petroleum degradation in the sea is limited by the availability of nutrients such as nitrogen and phosphorus, Pomeroy et al. (1995) mentioned previously, that bacterial growth is primarily limited by phosphate availability in the central Gulf of Mexico; their results suggest that growth of heterotrophic bacteria, either in terms of abundance or biomass, was limited by the availability of nutrients. Liu et al. (2017) found that the difference in inorganic nutrients and trace elements explained 10% of the variation in bacterial community structure; in another report, Bacosa et al. (2018b) noted that Alteromonas, Pseudoalteromonas, Oleibacter, and Winowgradskyella developed better in the incubations using bottom water, while Reinekea and Thalassobius were favored in surface water, suggesting that high levels of nutrients may play a key role in the development of these bacteria.
Bacosa et al. (2015) demonstrated that natural solar radiation impacted the oil-degrading bacterial communities, sunlight-favored certain bacterial genera such as Alteromonas, Marinobacter, Labrenzia, Sandarakinotalea, Bartonella, and Halomonas while, on the other hand, the dark incubation increased abundances of Thalassobius, Winogradskyella, Alcanivorax, Formosa, Pseudomonas, Eubacterium, Erythrobacter, Natronocella, and Coxiella. In Godoy-Lozano et al. (2018) the variables that most influenced the structure of HDB was the presence of aromatic hydrocarbons and depth. The genera Microcoleus, Ahrensia and Thermococcus involved in the degradation of hydrocarbons, as well as Tropicimonas, Dethiosulfatibacter, Cellulosimicrobium, Roseobacter, Prolixibacter, Desulfuromusa, Oceanicola and Salinivibrio involved in the degradation of aromatics, were found in shallow sediments with a higher concentration of aromatics. The availability of oxygen leads to two types of hydrocarbon degradation, aerobic and anaerobic. Raggi et al. (2020) determined the oxygen concentration in the Perdido Fold Belt zone (7.07 and 3.6 mg/L) and in the Campeche Knolls area (6.9 to 3.5 mg/L), for water column and sediments, respectively. Likewise, in these areas, similar alkB genes sequences related to the aerobic degradation of alkanes (Muriel-Millán et al., 2019) were found mainly in the water column, while in a in Campeche Knolls area sediment sample where anaerobic conditions prevail, shows a high diversity of bssA-like sequences, involved in anaerobic degradation of hydrocarbon (Acosta-González et al., 2013).
Natural leaks of oil are widely distributed in the GoM (MacDonald et al., 2015), suggesting that it has a basal level of HDB that can grow to address large-scale oil spills. This hypothesis was validated during the oil spill of the DWH and in later studies, in which metagenomic analysis and isolated cultures showed significant enrichment of bacterial populations related to Oceanospirillum, Cycloclasticus, Colwellia, Pseudoalteromonas, Rhodobacterales, methylotrophs, Alcanivorax, Oleiphilus, Oleispira, Thalassolituus, Oceanospirillales, Marinobacter, Acinetobacter, Pseudomonas and Planomicrobium (Hazen et al., 2010; Kostka et al., 2011; Redmond and Valentine, 2012; Mason et al., 2012; Dubansky et al., 2013; Kimes et al., 2013; Mason et al., 2014; Kleindienst et al., 2015; Orcutt et al., 2017; García-Cruz et al., 2018; Muriel-Millán et al., 2019; Uribe-Flores et al., 2019; Cerqueda-García et al., 2020; Rodríguez-Salazar et al., 2020).
As already mentioned, we established the bacterial baseline in marine sediments from the southwestern GoM with a core of 450 genera, where genera such as Colwellia, Pseudomonas, Oleispira, Marinobacter, Alcanivorax, Shewanella, Pseudoalteromonas, Cycloclasticus and Phaeobacter related to HDB are present at basal levels (Rosano-Hernandez et al., 2009; Godoy-Lozano et al., 2018; Sánchez-Soto Jiménez et al., 2018; Raggi et al., 2020; Ramírez et al., 2020). We also evaluated the natural degradation of oil and oil derivatives using HDB isolated at different depths (water column to sediment) from the northwestern to the southwestern regions of the GoM (García-Cruz et al., 2018; Uribe-Flores et al., 2019; Muriel-Millán et al., 2019; Cerqueda-García et al., 2020; Rodríguez-Salazar et al., 2020), where the largest and most important base of the oil industry in Mexico is concentrated. Cerqueda-García et al. (2020) evaluated samples of water and sediment enriched with different types of oil (extra light to extra heavy), finding a higher abundance of Gammaproteobacteria in sediments, where aerobic HDB are mostly found, decreasing the presence of heavy oil. Some examples of bacteria found were Dietzia, Gordonia, Microvirga, Rhizobium, Paracoccus, Thalassobaculum, Sphingomonas, Moheibacter, Acinetobacter, Pseudohongiella, Porticoccus, Pseudoalteromonas, Pseudomonas, Shewanella, and Planctomyces.
In Figure 6, we plot the relative abundances of the 16 predominant HDB genera in the sediment. The hydrocarbon-degrading genera were taken from the HDB catalog developed during the project. However, the hydrocarbon-degrading capabilities of bacteria belonging to these genera are well known (see, for example, Prince, 2010). We observed that the Pseudomonas genus is present in all the samples collected, and its metabolic versatility has made it a ubiquitous genus in all ecosystems. We found a characteristic signature of the genera present between latitudes 23° N and 26° N; Alcanivorax and Marinobacter were abundant. Colwellia is a genus found mainly in the southwestern region of the GoM between latitudes 18° S and 26° N. Interestingly, Pelobacter, a genus that plays an important role in iron- and sulfur-reducing anaerobic processes (Schink, 1984), is an abundant genus throughout the sampled region, but we observed it mainly at latitude 26° N and latitude 18° S.
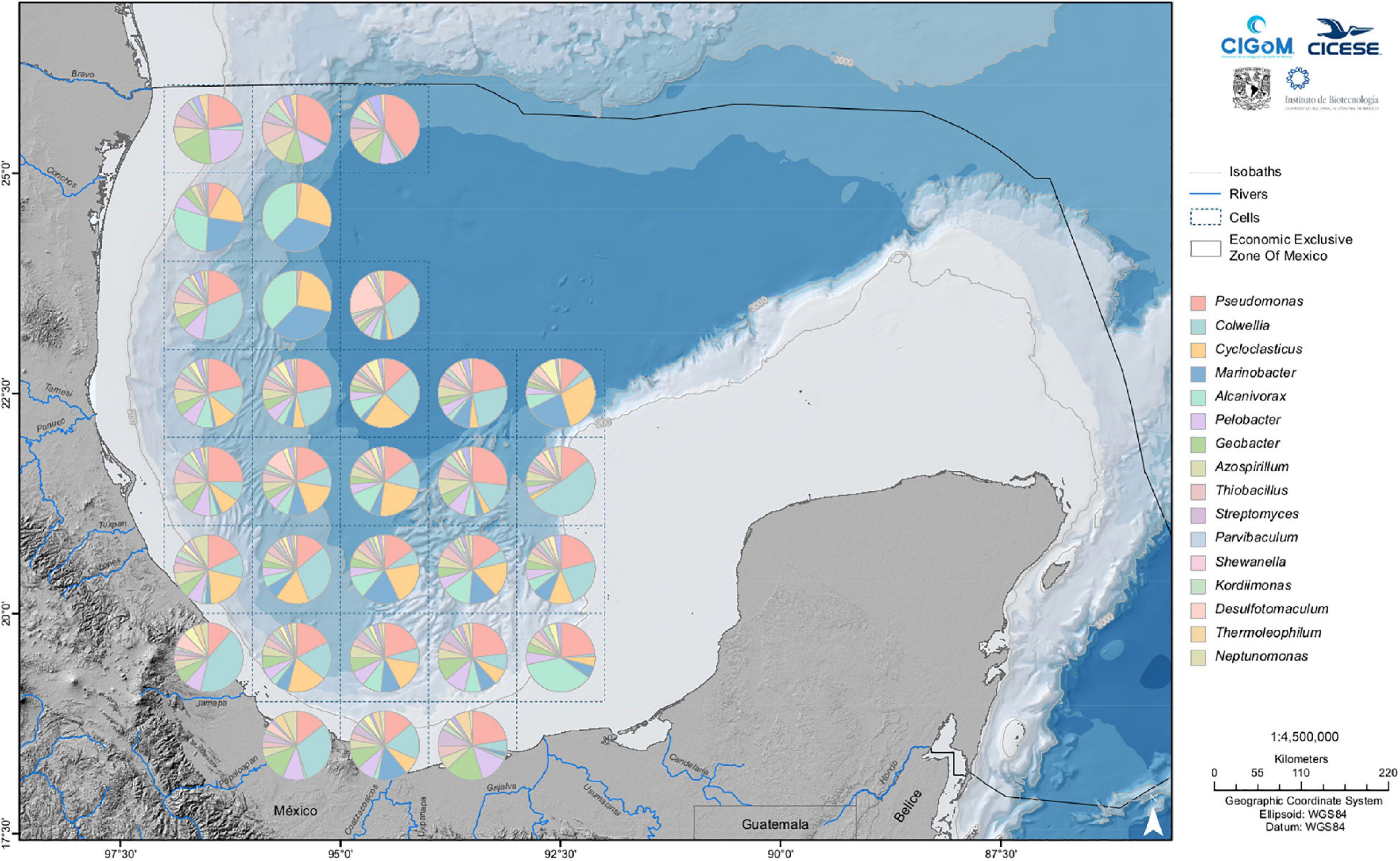
Figure 6. Most abundant genera of hydrocarbon-degrading bacteria in the sediments of the Gulf of Mexico. The genera appear in the legend according to their relative abundances.
Likewise, the microbial composition and distribution in the strata of different water depths in the northwestern and southeastern regions of the GoM were established. The abundance of PHDB (potential HDB) was evident; 39 genera reported as HDB were present, such as Pseudomonas, Acinetobacter, Alcanivorax, Alteromonas and Halomonas, which belong to the basal microorganisms and are found mainly in water columns (Raggi et al., 2020). Our data show differences between proportions in which HDB were detected in cells in the north and south of 23° N. In particular, although Halomonas was present in most cells with a significant proportion to the south of 23° N, it is almost absent in cells located at Perdido Fold Belt (23–24° N). However, the genera Alcanivorax and Alteromonas were present at all the different water depths sampled, and these genera exhibited high alkane and polycyclic aromatic hydrocarbon-degradation capacities, respectively (Figure 7; Jin et al., 2012; Liu and Liu, 2013).
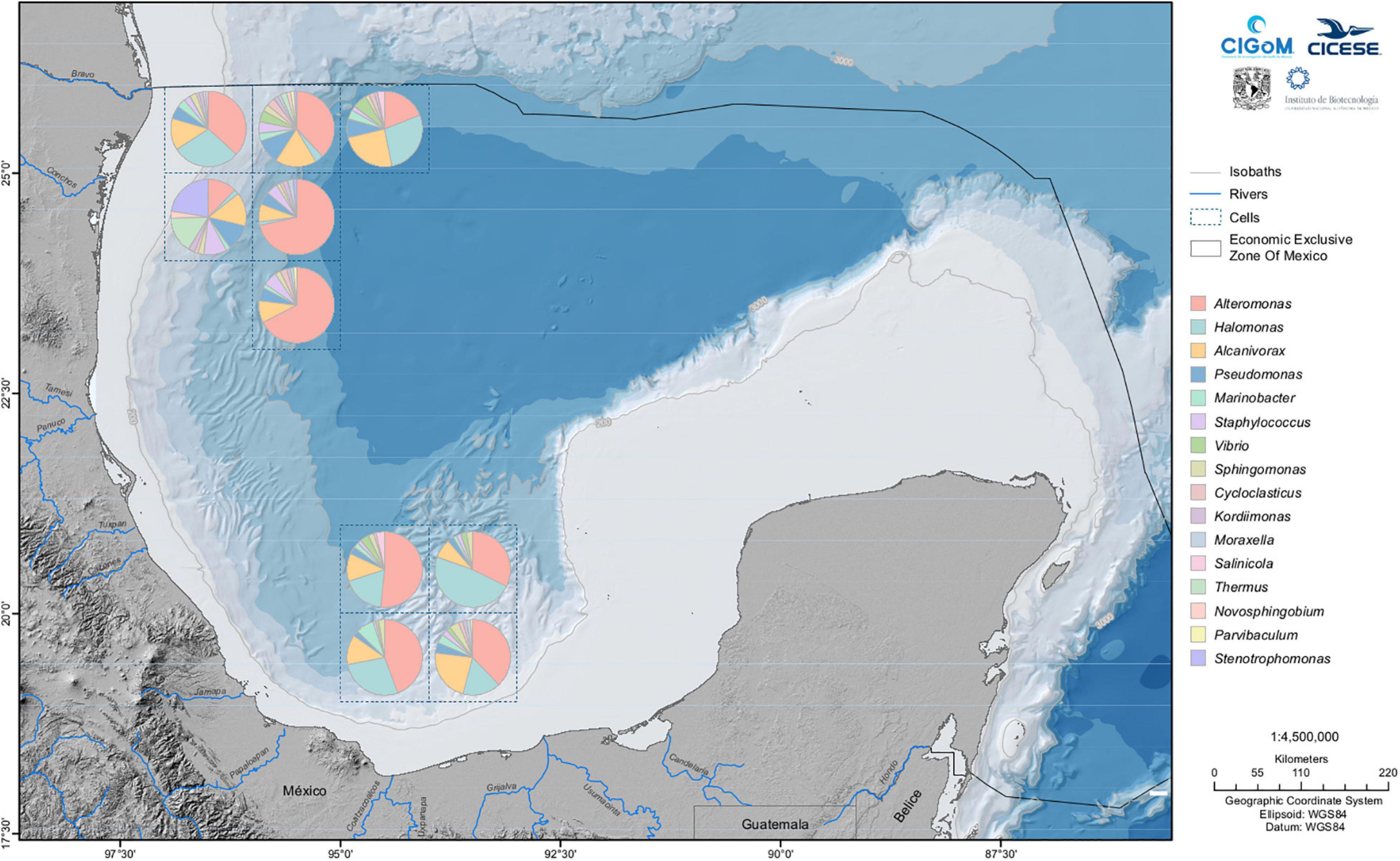
Figure 7. Most abundant genera of hydrocarbon-degrading bacteria in the water column of the Gulf of Mexico. The genera appear in the legend according to their relative abundances.
The presence and quantification of Alcanivorax and Cyclocasticus was determined by qPCR assays using primers to amplify a fragment of the 16S-SSU-rDNA gene in the water column and sediment samples. Thus, we determined that both genera are widely distributed from the surface to deep water and sediments in the sGoM where Alcanivorax spp. predominates between depths of 250 and 1000 m in the water column, while the abundance of Cycloclasticus spp. increases with the depth of the water column (1200 to 2500 m) (Lizárraga-Partida et al., 2019).
Functional Potential of the Gulf of Mexico
The identification of metabolic potential in environmental samples can be performed using high-throughput sequencing as the shotgun metagenomic technique. The shotgun fragments the DNA of the environmental sample into nucleotide sequences of sizes. In a subsequent phase, they are translated into a code that describes the proteins to be compared subsequently with reference protein databases, where many of them have been experimentally characterized. Whole-metagenome shotgun analysis was performed for 8 samples collected in the GoM, and the metabolic potential was inferred. The main idea underlying these studies was to determine the presence of enzymes involved in petroleum degradation; however, other capabilities were detected (Raggi et al., 2020). By analyzing the functional potential of the water column and sediments, we found evidence of the presence of enzymes involved in aerobic and anaerobic hydrocarbon-degradation metabolism in some samples, which is important for the ecological dynamics of hydrocarbons and potential use of water and sediment bioremediation processes (Raggi et al., 2020). The sediments also showed the presence of anaerobic metabolism involved in methanogenesis, sulfur reduction and inorganic carbon fixation. Thus, the sediments analyzed are mostly oxygen-depleted sediments harboring an anaerobic bacterial community (Raggi et al., 2020).
Marker genes involved in the aerobic and anaerobic degradation of hydrocarbons, such as AlkB (alkane hydroxylase) and CYP153 (cytochrome P53) for the degradation of alkanes and Rieske oxygenase and LigB (protocatechuate dioxygenase), gentisate (cupin superfamily), and EDO (extradiol dioxygenase) for the degradation of aromatic hydrocarbons, were found in the water and sediment samples. Several marker genes of anaerobic degradation were found, such as bssA (benzyl succinate), napcA (naphthalene carboxylase), abcA (putative benzene carboxylase), ppcA (phenylphosphate carboxylase), apcA (acetophenone carboxylase), ppsA (phenylphosphate synthase), ahyA (alkane C2-methylene hydroxylase), ebdA (ethylbenzene dehydrogenase), and cmdA (p-cymene dehydrogenase) (Kimes et al., 2013; Raggi et al., 2020).
The global metabolome of marine consortia from sediments of the GoM grown with a complex hydrocarbon mixture was reported. Hydrocarbon derivatives were detected as carboxylic acids or alcohols, and tetracycline-related chemicals and sphinganines were also detected as non-hydrocarbon derivatives, corroborating that marine microbes can synthesize novel molecules with therapeutic potential (Moreno-Ulloa et al., 2020).
Applications and Perspectives
Conducting experiments in marine systems presents various challenges. In the 1970s, mesocosm use became popular in marine research (Hodson et al., 1977). A mesocosm is a bounded and partially enclosed outdoor experimental setup that bridges the gap between the laboratory and real world in environmental science (Odum, 1984; Crossland and La Point, 1992; Bruckner et al., 1995). Mesocosm studies have been used as a reference to understand the complex process of the bioremediation of oil spills. This process scalability has provided extended value to understand and test what has been studied at the microscale level to analyze the complexity of biological processes. Microcosms or in vitro experiments test variables in a controlled, reduced and reproducible manner; however, these are conditions far from reality. Mesocosm experiments offer a good tradeoff between variable control and real condition emulation at a larger scale.
Santas et al. (1999) tested the augmentation effect of 2 fertilizers on the biodegradation of light oil by native bacteria in the Mediterranean ecosystem in 3 m3 tanks filled with unsterilized seawater. Cappello et al. (2007) studied the changes in the native bacterial community of seawater used to degrade light oil (0.1 g/L) in 10 m3 mesocosm systems, finding enrichment of Alcanivorax species in the consortium. Kadali et al. (2012) tested the degradation of crude oil (1%) with a synthetic consortium made of 6 bacterial isolates in seawater mesocosm systems. Hassanshahian et al. (2014) tested a synthetic consortium with 2 isolates for light crude oil degradation in 10 m3 mesocosms with seawater. Dellagnezze et al. (2016) similarly tested a synthetic consortium of 4 bacterial strains in tanks with unsterilized seawater and light oil (0.9 g/L). Likewise, biodegradation studies at a mesocosm scale (Venosa and Zhu, 2003; Delille and Coulon, 2008) have been conducted in situ in polluted soils. For bacterial consortia in marine mesocosm systems, variables related to the oil biodegradation process must be considered, such as temperature (Bagi et al., 2013; Al-Hawash et al., 2018), the concentration of dissolved oxygen (Vilcáez et al., 2013), hydrocarbon biodegradation, and availability of nutrients, such as nitrogen and phosphorus (Cappello et al., 2007; Bagi et al., 2013; Ron and Rosenberg, 2014; Valencia-Agami et al., 2019).
In the next section, some applications and perspectives will be discussed; the characterization of the bacterial taxa found in the GoM could be used for micro, meso and macrocosm experiments that, when combined with some of the techniques described below, could offer a possible application to in situ hydrocarbon biodegradation.
Bioprospecting of Enzymes
Bioprospecting is defined as a systematic and organized search for useful and potentially commercially valuable chemical compounds, genes, proteins, secondary metabolites, and microorganisms derived from bioresources, including plants, microorganisms, and animals, that can be developed to have desirable benefits for society (Pardo-López, 2019). Organisms inhabiting the ocean can synthesize compounds in response to environmental stimuli; these compounds are not always essential for growth or development, but they are important for adaptation and survival in the environment (Rotter et al., 2020). In recent years, there has been an increase in the exploration of new metabolites from the ocean with essential properties for industrial applications, promoting marine biotechnology (Rotter et al., 2020). A great demand exists for suitable enzymes with high process performances that are ‘greener’ alternatives to chemical synthesis (Adrio and Demain, 2003; Fernández-Arrojo et al., 2010). Marine enzymes have essential properties for industrial applications, such as thermostability and tolerance to a wide pH range and salinity conditions (Rotter et al., 2020); furthermore, the degradation of petroleum hydrocarbons may be mediated by a specific enzyme system (Das and Chandran, 2011). In marine environments, hydrolases participate in the degradation of organic compounds. Industrially, hydrolases are used to process food, medicine, paper, starch, and textiles and for the manufacture of detergents. The most commonly used hydrolases are amylases, cellulases, xylanases, proteases, lipases and esterases (Dalmaso et al., 2015). Lipases have been found to be involved in the degradation of alkanes (Hausmann and Jaeger, 2010).
However, the search for enzymes with degradation capacity may be limited because the percentage of cultivable marine bacteria in this environment is considerably lower than that in other habitats (Amann et al., 1995); approximately 99.9% of microorganisms cannot be cultivated by standard laboratory techniques (Amann et al., 1990). The discovery of new enzymes without having to culture the microorganisms has been improved by metagenomics analysis, allowing the genetic screening of communities of microorganisms present in different environments in the ocean without cultivation. There are two metagenomic screening approaches: sequence- and function-based techniques (Figure 8; Lee et al., 2010; Mora et al., 2011; Hess et al., 2011; Kube et al., 2013; Trindade et al., 2015).
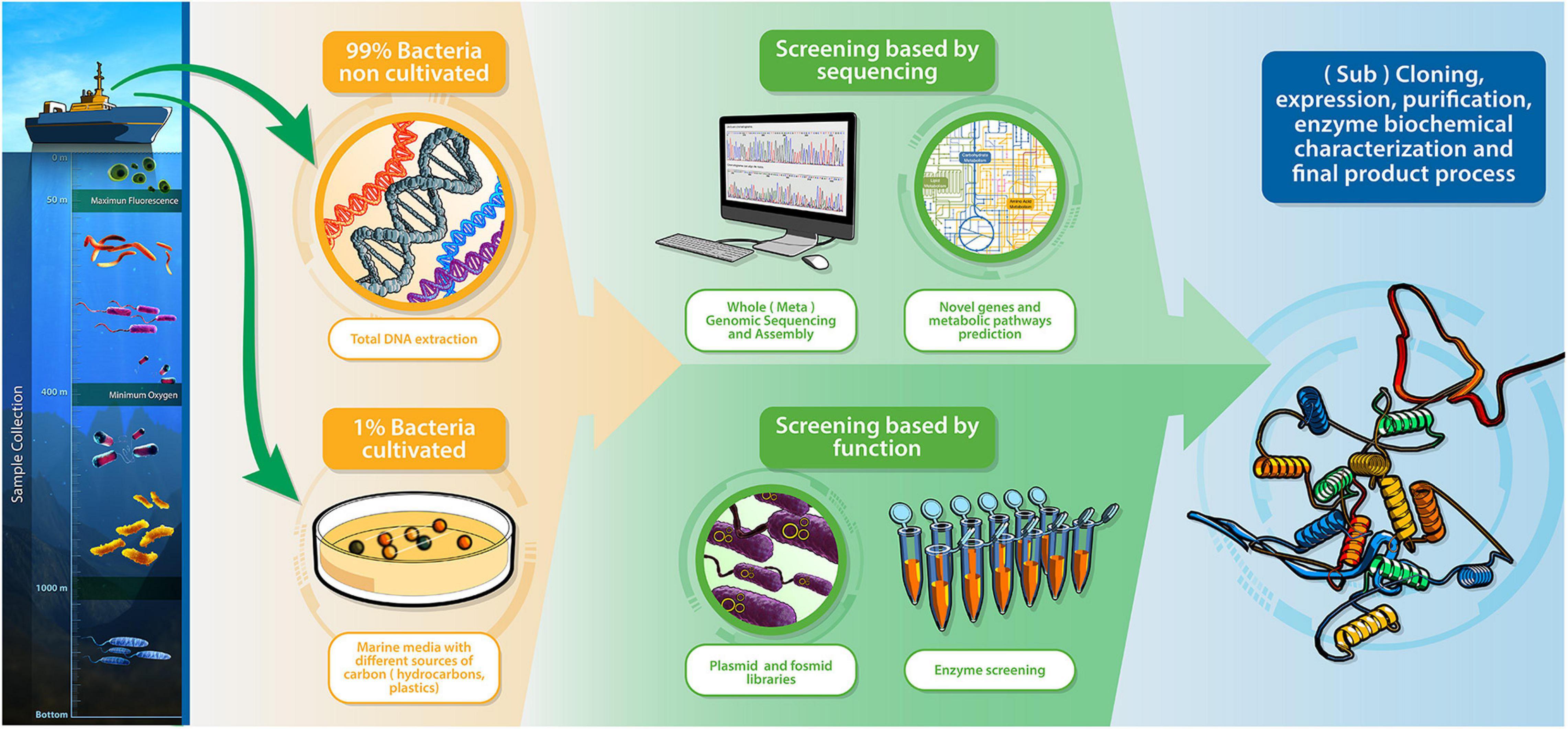
Figure 8. Metagenomics as a tool for enzyme discovery. Overview of screening by sequence and function.
In screening by sequence-based techniques, genome or sequence information is preferred or necessary. One option is to perform targeted or whole-genome sequencing to identify the desired enzyme gene sequence and amplify a specific target gene using degenerate primers (Berón et al., 2005). When metagenomic libraries are constructed, clones are screened using primers for the gene of interest to discard negative clones, leading to the possibility of finding enzymes with high activity. The resulting clones are sequenced and cloned in specific vectors for heterologous expression. This is a straightforward and promising approach to identify novel enzyme candidates with better enzymatic properties.
The identification process can be performed in two ways: searching databases and using bioinformatic tools to screen and analyze putative clones or candidates. The first involves utilizing search tools to search for homology, consensus sequences, conserved motifs, percentage identity, e-value and query coverage. The second involves analyzing distinct functional properties using bioinformatic tools such as ProtParam, ExPASy, and GRAVY to examine physicochemical properties and structural information. Programs such as MEGA (Molecular Evolutionary Genetics Analysis) and SWISS-MODEL are used for phylogenetic analysis. Thus, enrichment of databases is necessary when in silico bioprospecting of novel enzymes is performed.
Identification based on sequence information has been used at CIGoM in the search for enzymes that degrade aromatic compounds. The genome sequence of the marine bacterium Pseudomonas stutzeri GOM2, isolated from the southwestern GoM, revealed the presence of the benABC operon, which is involved in benzoate catabolism. This information allowed the characterization of a novel catechol 1,2 dioxygenase that is active in a trimeric state (Rodríguez-Salazar et al., 2020). Using this methodology, several enzymes have been successfully identified, expressed and tested, such as epoxide hydrolases (Jiménez et al., 2015), haloalkane dehalogenases (Barth et al., 2004) and carbohydrate esterases (Tasse et al., 2010).
Screening by function includes enzyme activity screens performed in culture, where most can be detected phenotypically by employing chromogens, dyes and substrates as target enzymes incorporated into the culture plate. Sequence information is not necessary, and novel genes and enzymes can be identified. However, some disadvantages were observed related to the vector used that can carry large inserts and allow expression in multiple hosts but can exhibit failure in gene expression, defective translation, and protein misfolding. Screening by function is based on the generation of metagenomic libraries in artificial expression vehicles, such as plasmids, BACs, YACs, cosmids and fosmids, to preserve and subsequently analyze the genomic DNA of the microbial community under study.
Genomic libraries in fosmids have been explored at CIGoM using metagenomic DNA from consortia or environmental samples in which the presence of hydrocarbonoclastic bacteria has been determined (Figure 8). The selection of consortia from the GoM with hydrocarbon-degradation capacity was performed using samples of water columns from depths of 50 m and 1000 m (Muriel-Millán et al., 2019). The samples were inoculated with marine medium and mineral medium with crude oil and kerosene (0.01–0.1%) as the only carbon sources. From the samples collected off the coast of Tamaulipas in the Perdido Escarpment area, northwest of the GoM, the B9 consortia showed the highest growth in the presence of hydrocarbons and were selected for the construction of metagenomic libraries. Moreover, the environmental metagenomic library came from sediment samples from the Southwest of the GoM on the coast of Campeche and is currently under evaluation.
One functional screening strategy is the search for a specific activity through the detection of a pigment or use of chromogenic and fluorogenic enzymatic substrates that allow the detection of specific catalytic functions (Trindade et al., 2015). This strategy is useful for functional screening in the search for extradiol dioxygenase (EDO) activity by the enzyme catechol 2,3 dioxygenase (C23D) or lipolytic activity by lipases and esterases, and the experiment is performed using agar plates with tributyrin or olive oil as the substrate (Glogauer et al., 2011) and rhodamine B (Carissimi et al., 2007).
Bioremediation
Remediation is the application of several processes to convert environmental contaminants to harmless substances based on the microbial metabolism of specific harmless microorganisms, plants, and their enzymatic sets. Two different strategies have been developed: engineering based on physical and chemical methods (Bollag and Bollag, 1995) and biological strategies that require the involvement of biological agents (Gianfreda and Rao, 2008). Regardless of the selected method, bioremediation may be performed in situ, involving cleaning soils and water directly on site in the contaminated environment and being usually less expensive and involving less physical treatment, or ex situ, relying on removal by excavation or transport of the sample to another site, followed by extraction of the contaminant before its degradation into harmless substances. These treatments have higher costs and cause increased environmental disturbance.
The main problems in the treatment process for the in situ bioremediation of contaminated sites include the following: the concentrations of pollutants; the solubility, adsorption and volatility of compounds; the chemistry and microbiology of groundwater and soil; and the biodegradability of contaminants. Depending on their properties, contaminants enter the environment as solids, liquids or gases; factors such as water, soil and biological materials will determine, in part, the bioavailability of any given pollutant (Harms and Bosma, 1997). At higher concentrations in the environment, they may be resistant to biodegradation or are biodegraded at low rates; therefore, fewer organisms will tolerate the toxic effects. Some impediments to biodegradation also result from physical phenomena, limiting substrate and cofactor bioavailability or the lack of appropriate biochemical machinery in microorganisms.
Several reports indicate that an important factor for hydrocarbon biodegradation rates is the bioavailability of oil components. De Jonge et al. (1997) indicates that the bioavailability of oil is controlled by two mechanisms. At higher alkane concentrations, bioavailability is controlled by solubilization from a non-aqueous-phase liquid into the aqueous soil water phase. However, at low concentrations, desorption and diffusion are rate-limiting factors, showing that the biodegradation rates of n-alkanes increase with decreasing carbon number and that n-alkane ratio monitoring can be used to improve the efficiency of bioremediation treatments.
In Huesemann et al. (2004) tested whether the bioavailability of petroleum hydrocarbons limits its biodegradability by measuring the biodegradation and abiotic desorption rates of PAHs and n-alkanes in aged soils. They concluded that PAH biodegradation was limited by microbial factors and not because of bioavailability limitations.
Petroleum degradation at sea is mainly performed by microorganisms, and the communities involved in this process comprise many members (Harayama et al., 1999). Cappello et al. (2007) evaluated a sample of a marine bacterial community after an oil spill accident in Messina, Italy, based on its population dynamics and light crude degradation capacity in microcosms with seawater for fifteen days. Tao et al. (2017) studied the effect of the addition of an isolated strain to a natural consortium and its effect on bacterial diversity and the increase in its degradation capacity. Other studies in which microbial communities have been tested for oil degradation include those conducted by Xu et al. (2013) and Marietou et al. (2018), where they validate the potential use of bacterial communities in bioremediation. However, one of the main limitations is the loss of enzyme activity in these microorganisms due to unfavorable conditions, which can be overcome by the immobilization of one of the components.
Immobilization of Strains and Enzymes
The implementation of wild-type or genetically engineered microorganisms has been developed for the bioremediation of contaminated water, land and soil because these microbes can easily adapt to contaminants; otherwise, the ability of enzymes to catalyze reactions has made them indispensable to science for decades (Ngo, 1980), offering several advantages over traditional technologies and over microbial remediation. Enzymes are catalysts with either narrow or broad specificity, can be easily reused multiple times for the same reaction, can be applied to a large range of different compounds and environments, are effective at low pollutant concentrations and are active in the presence of microbial predators. These features make them good candidates to overcome some disadvantages related to the use of microorganisms (Karam and Nicell, 1997; Nicell, 2001; Gianfreda and Bollag, 2002). However, some multimeric enzymes are not stable under certain environmental conditions, such as shifts in pH, temperature, ionic strength, cofactor requirements and the presence of inhibitors; these factors have a strong impact on the loss of enzyme activity and low stability as a result of protein denaturation and inactivation (Schnell and Hanson, 2007; Alemzadeh and Nejati, 2009).
To overcome this limitation, immobilization has been proposed as a successful method (Van de Velde et al., 2002). Most enzymes function in water, leading to the impossibility of recovery for reuse; in these situations, enzymes can be fixed physically or chemically to solid supports by weak interactions or covalent bonds to stabilize their structure and maintain their activity; thus, compared with free enzymes in solution, enzymes are more robust and resistant to environmental changes. Among the advantages of immobilized enzymes versus enzymes in solution are higher activity, selectivity and specificity, and this method allows us to obtain more stable and reusable enzymes than the free solution-based method (Katchalski et al., 1971; Garcia-Galan et al., 2011). Immobilization of enzymes involves physical or chemical attachment to an inert material that can be organic or inorganic, such as calcium alginate; this attachment can increase the resistance to some changes, such as changes in pH and temperature (Cherry and Fidantsef, 2003). The choice of a specific immobilized enzyme or mode of immobilization must be based on a specific compromise considering all the advantages and disadvantages of free and immobilized enzymes.
Different methods of enzyme immobilization have been developed for commercial use, and more than 5,000 publications and patents have been published on enzyme immobilization techniques. Immobilized enzymes can be found in industry, medicine, and research. Some examples are proteases, lipases, invertases and several enzymes involved in hydrocarbon degradation.
A manganese peroxidase produced by the rot fungus Anthracophyllum discolor, was immobilized on nanoclay. Compared with the free enzyme, immobilized peroxidase showed increased stability to high temperature, pH and time storage, as well as enhanced PAHs degradation efficiency in soil. The immobilized enzyme could degrade pyrene and anthracene, alone or in a mixture, fluoranthene and phenanthrene as valuable options for in situ bioremediation purposes (Acevedo et al., 2010). However, a recombinant oxidative enzyme that catalyzes ring cleavage of catechol and its analogs from Arthrobacter chlorophenolicus was immobilized on single-walled carbon nanotubes. The immobilized enzyme was more stable toward extreme pH, temperature, and ionic strength conditions than the free enzyme (Suma et al., 2015). Some efforts to improve the functional stability of enzymes by increasing its structural rigidity have been made. A catechol 1,2-dioxygenase from Stenotrophomonas maltophilia was immobilized in alginate hydrogel. Activity of the immobilized enzyme was still observed on the 28th day of incubation at 4°C, whereas the free enzyme lost its activity after 14 days. Immobilization of the enzyme promoted its stabilization against any distorting agents: aliphatic alcohols, phenols, and chelators (Guzik et al., 2014).
For aliphatic hydrocarbons, several reports have indicated that the immobilization of enzymes results in better performance. The crude alkane hydroxylase and lipase enzymes from the hydrocarbonoclastic bacterium Alcanivorax borkumensis were entrapped into chitosan nanoparticles. The immobilized alkane hydroxylase and lipase exhibited a more than two-fold increase in the in vitro half-life compared with the free enzymes, maintaining approximately 70% of the initial activity after 5 days (Kadri et al., 2018).
The immobilization of strains is another strategy that works in cooperation with the immobilization of enzymes; some studies report that immobilized cells increase the tolerance ability to unfavorable conditions compared with free-living bacteria, being more effective, with a longer shelf life, lower cost price and higher crude oil degrading activity. Analysis of hydrocarbon residues revealed that the biodegradation capacity of the microorganisms is not compromised by the immobilization. Rahman et al. (2006) studied the capacity of immobilized bacteria in alginate beads to degrade hydrocarbons. The results showed no decline in the biodegradation activity of the microbial consortium, concluding that immobilization of cells is a promising application in the bioremediation of hydrocarbon-contaminated sites. Different successful cases have been reported. For example, Pseudomonas aeruginosa was tested for its ability to degrade highly concentrated crude oil-contaminated water after immobilizing it on the surface of polyurethane foam. The results demonstrated that, after 12 h, the average oil removal rate in 2 g of crude oil/L of contaminated water was approximately 90% for 40 days (Nie et al., 2016).
In some cases, immobilization materials improve the survival and activity of the immobilized strain. The potential of an immobilized HDB strain for crude oil-polluted seawater bioremediation was tested in seawater microcosms. Concerning the removal percentage of crude oil after 15 days, the microcosms treated with the immobilized inoculants proved to be the most successful (Gentili et al., 2006). In a similar case, a bacterial consortium comprising four strains of marine bacteria for degrading pyrene, two strains for benzo(a)pyrene, and three strains for indeno(1,2,3-cd)pyrene was isolated from oil-contaminated seawater and immobilized in magnetic floating biochar gel beads to remove high-molecular-weight PAHs. The immobilized consortium performed better than single strains and had better tolerance to pH, temperature and salinity than free cells (Qiao et al., 2020).
Biosensors
Biosensors offer great advantages over conventional analytical techniques because they integrate biological systems with transducers tailored for a target analyte. Biosensors have potential applications in biotechnology because of their high specificity, sensitivity, and effectiveness. Most immobilized enzymes are used as biosensors (Wilson and Hu, 2000) among them, several dioxygenases and peroxidases are used to detect hydrogen peroxide, phenolic compounds, and metal ions (Bouyahia et al., 2011; Shamsipur et al., 2012).
A biosensor is a molecular device used to analyze a sample in the presence of a specific target and is constructed from a biological component comprising two elements immobilized onto a surface; a recognition element that is a specific enzyme in an enzyme-based biosensor and a detector component or transducer, which can interact with target molecules. This interaction would produce physicochemical changes that are converted to measurable signals to determine the amount of analyte present in the sample. The signal is generated directly by the interaction of the analyzed material with the transducer or integration of a signal generated by a colorimetric, fluorescence-based or luminescence-based method. The goal is to improve or increase the enzyme stability or sensitivity of detection.
A bioreporter is available to detect alkanes and alkenes with carbon chain lengths from C7 to C36 in water, seawater and soil, developed from a strain of Acinetobacter that can adhere to oil-water interfaces, search for crude oil droplets and sense oil spills in water and soils (Zhang et al., 2011). So far, it was able to detect alkane with carbon chain length greater than C18 and was applied to detect mineral oil, Brent, Chestnut and Sirri crude oils in water and seawater in the range 0.1–100 mg/L. A different method was developed for the construction of a microbial whole-cell biosensor to measure water-dissolved concentrations of middle-chain-length alkanes and some related compounds. The biosensor was used to detect the bioavailable concentration of alkanes in heating oil-contaminated groundwater samples and responding also to middle-chain-length alkanes but not to alicyclic or aromatic compounds (Sticher et al., 1997).
For aromatic hydrocarbons, a green fluorescent protein-based Pseudomonas fluorescens strain A506 biosensor was constructed and characterized for its potential to measure benzene, toluene, ethylbenzene, and related compounds in aqueous solutions. The biosensor is based on a plasmid carrying the toluene-benzene utilization pathway from Ralstonia pickettii PKO. The fluorescence response was specific for alkyl-substituted benzene derivatives and branched alkenes (di- and trichloroethylene, 2-methyl- 2-butene) and was unaffected by the presence of compounds that were not inducers, such as those present in gasoline (Stiner and Halverson, 2002).
Concluding Remarks
Oceanographic campaigns performed throughout the exclusive economic zone of the GoM, in conjunction with the performed metagenomic studies, allowed the exploration of the bacterial diversity and functional potential of the sGulf for the first time. The observed taxonomy and inferred metabolic potential show the impact on the bacterial diversity that natural oil emissions and anthropogenic spills have had in different areas of the Gulf, in which abundant taxa related to hydrocarbon degradation were observed. Metagenomic studies based on the 16S gene marker let the group propose the first bacterial baseline of the exclusive economic zone of the sGoM, work that without a doubt will be a reference for future metagenomic studies and the development of microbiological strategies and biotechnological tools to manage oil spill products from oil exploration, extraction and transport. In addition to this effort, we isolated several bacterial strains from the GoM (Escobedo-Hinojosa and Pardo-López, 2017; Muriel-Millán et al., 2019), as well as enzymes (Rodríguez-Salazar et al., 2020), selected based on their hydrocarbon-degradation capacity and potential.
The microbial diversity of the GoM has great potential to contribute to biotechnology-based research and development, principally in the agriculture, pharmaceutical, detergent and pollution remediation industries. Currently, bioremediation and related technologies have mainly been applied to solve contamination-related problems in soil and groundwater. Only a few alternative applications have been implemented for the marine environment because the characteristics of this environment continue to pose challenges. Compared with soil and groundwater, marine environmental contamination occurs in a non-static and unconfined matrix, where the polluted water is in constant flux because of marine streams. Additionally, changes in temperature, climate conditions and the native bacterial population could affect the biodegradation processes, increasing the challenge for oil spill remediation applications.
Bioprospecting of enzymes and bacteria from marine environments opens the possibility to explore a rich reservoir of unique life systems because oceans harbor unique habitats mostly unexplored. Complex approaches for the screening of molecules from marine microorganisms with biotechnological potential have been developed for the successful application of lipases/esterase, xylanases, and dioxygenases among others.
Several technologies based on novel methods have been developed, such as the current advances in biosensor technologies as analytical tools that use biological specificity in sensing a target molecule, identifying several types of reporter systems tracking different levels of pollutants in different habitats and toxic compounds. These technologies lead to the identification and development of different designs of microbial systems using immobilization supports for enzymes or bacteria to increase their potential applications in bioremediation. Thus, an excellent base is provided to increase the availability of enzymes to the substrate with greater turnover over a considerable period of time and offer a microsystem when strains are used. Presently, immobilized enzymes are preferred over their free counterparts because of their prolonged availability that curtails redundant downstream and purification processes.
Author Contributions
JR-S, AL, KO-O, RMG-R, and LP-L wrote sections of the manuscript. JR-S, KO-O, and LP-L contributed to the conceptualization of the manuscript. AL and RMG-R organized the database and performed the computational and bioinformatic analysis. LP-L coordinated the IBt-L4-CIGoM group. All the authors contributed to provide feedback to the manuscript and approved the submitted version.
Conflict of Interest
The authors declare that the research was conducted in the absence of any commercial or financial relationships that could be construed as a potential conflict of interest.
Funding
This research was funded by the National Council of Science and Technology of Mexico – Mexican Ministry of Energy- Hydrocarbon Trust, project 201441, and PAPIIT-DGAPA IN207019. This is a contribution of the Gulf of Mexico Research Consortium (Consorcio de Investigación del Golfo de México (CIGoM), 2020).
Acknowledgments
We acknowledge PEMEX, and specifically the Hydrocarbon Fund, for addressing the environmental effects of oil spills in the Gulf of Mexico. We are grateful to Daniel Mayer Martínez for the drawings and diagrams and Rigel Zaragoza Álvarez and Ana Patricia Arias Torres for the map design images.
Footnotes
References
Acevedo, F., Pizzul, L., Castillo, M. D. P., González, M. E., Cea, M., Gianfreda, L., et al. (2010). Degradation of polycyclic aromatic hydrocarbons by free and nanoclay-immobilized manganese peroxidase from Anthracophyllum discolor. Chemosphere 80, 271–278. doi: 10.1016/j.chemosphere.2010.04.022
Acosta-González, A., Rosselló-Móra, R., and Marqués, S. (2013). Diversity of benzylsuccinate synthase-like (bssA) genes in hydrocarbon-polluted marine sediments suggests substrate-dependent clustering. Appl. Environ. Microbiol. 79, 3667–3676. doi: 10.1128/aem.03934-12
Adrio, J. L., and Demain, A. L. (2003). Fungal biotechnology. Int. Microbiol. 6, 191–199. doi: 10.1007/s10123-003-0133-0
Aggregation and Degradation of Dispersants and Oil by Microbial Exopolymers (ADDOMex) (2020). Available online at: https://www.tamug.edu/addomex/ (accessed September 2020).
Alemzadeh, I., and Nejati, S. (2009). Phenols removal by immobilized horseradish peroxidase. J. Hazard. Mater. 166, 1082–1086. doi: 10.1016/j.jhazmat.2008.12.026
Al-Hawash, A. B., Dragh, M. A., Li, S., Alhujaily, A., Abbood, H. A., Zhang, X., et al. (2018). Principles of microbial degradation of petroleum hydrocarbons in the environment. Egypt. J. Aquat. Res. 44, 71–76. doi: 10.1016/j.ejar.2018.06.001
Amann, R. I., Ludwig, W., and Schleifer, K. H. (1995). Phylogenetic identification and in situ detection of individual microbial cells without cultivation. Microbiol. Rev. 59, 143–169.
Amann, R. J., Binder, B. L., Chisholm, S. W., Devereux, R., and Stahl, D. A. (1990). Combination of 16S rRNA targeted oligonucleotide probes with flow cytometry for analysing mixed microbial populations. Appl. Environ. Microbiol. 56, 1910–1925. doi: 10.1128/AEM.56.6.1919-1925.1990
American Petroleum Institute (API) (2021). Available online at: https://www.api.org/ (accessed January 18, 2021).
Bacosa, H. P., Erdner, D., Rosenheim, B., Shetty, P., Seitz, K., Baker, B., et al. (2018a). Hydrocarbon degradation and response of seafloor sediment bacterial community in the northern gulf of mexico to light louisiana sweet crude oil. ISME J. 12, 2532–2543.
Bacosa, H. P., Evans, M. M., Wang, Q., and Liu, Z. (2018b). “Assessing the role of environmental conditions on the degradation of oil following the deepwater horizon oil spill,” in Oil Spill Environmental Forensics Case Studies, eds S. A. Stout and Z. Wang (Oxford: Butterworth-Heinemann), 617–637. doi: 10.1016/B978-0-12-804434-6.00028-8
Bacosa, H. P., Liu, Z., and Erdner, D. L. (2015). Natural sunlight shapes crude oil-degrading bacterial communities in northern Gulf of Mexico surface waters. Front. Microbiol. 6:1325. doi: 10.3389/fmicb.2015.01325
Bagi, A., Pampanin, D. M., Brakstad, O. G., and Kommedal, R. (2013). Estimation of hydrocarbon biodegradation rates in marine environments: a critical review of the Q10 approach. Mar. Environ. Res. 89, 83–90. doi: 10.1016/j.marenvres.2013.05.005
Barth, S., Fischer, M., Schmid, R. D., and Pleiss, J. (2004). The database of epoxide hydrolases and haloalkane dehalogenases: one structure, many functions. Bioinformatics 20, 2845–2847. doi: 10.1093/bioinformatics/bth284
Bengtsson-Palme, J., Hartmann, M., Eriksson, K. M., Pal, C., Thorell, K., Larsson, D. G. J., et al. (2015). METAXA2: improved identification and taxonomic classification of small and large subunit rRNA in metagenomic data. Mol. Ecol. Resour. 15, 1403–1414. doi: 10.1111/1755-0998.12399
Berón, C. M., Curatti, L., and Salerno, G. L. (2005). New strategy for identification of novel cry-type genes from Bacillus thuringiensis strains. Appl. Environ. Microbiol. 71, 761–765. doi: 10.1128/aem.71.2.761-765.2005
Biello, D. (2010). Is Using Dispersants on the BP Gulf Oil Spill Fighting Pollution with Pollution? Sustainability. Scientific American. Available online at: https://www.scientificamerican.com/article/is-using-dispersants-fighting-pollution-with-pollution/ (accessed November 19, 2020).
Biller, S. J., Berube, P. M., Lindell, D., and Chisholm, S. W. (2015). Prochlorococcus: the structure and function of collective diversity. Nat. Rev. Microbiol. 13, 13–27. doi: 10.1038/nrmicro3378
Bollag, J.-M., and Bollag, W. B. (1995). “Soil contamination and the feasibility of biological remediation,” in Bioremediation: Science and Applications, Vol. 43, eds H. D. Skipper and R. F. Turco (Madison, WI: SSSA Special Publications), 1–12. doi: 10.2136/sssaspecpub43.c1
Bouyahia, N., Hamlaoui, M. L., Hnaien, M., Lagarde, F., and Jaffrezic-Renault, N. (2011). Impedance spectroscopy and conductometric biosensing for probing catalase reaction with cyanide as ligand and inhibitor. Bioelectrochemistry 80, 155–161. doi: 10.1016/j.bioelechem.2010.07.006
Bruckner, A., Wright, J., Kampichler, C., Bauer, R., and Kandeler, E. (1995). A method of preparing mesocosms for assessing complex biotic processes in soils. Biol. Fertil. Soils 19, 257–262. doi: 10.1007/BF00336169
Cai, Q., Zhu, Z., Chen, B., and Zhang, B. (2019). Oil-in-water emulsion breaking marine bacteria for demulsifying oily wastewater. Water Res. 149, 292–301. doi: 10.1016/j.watres.2018.11.023
Cappello, D., Caruso, G., Zampino, D., Monticelli, L. S., Maimone, G., Denaro, R., et al. (2007). Microbial community dynamics during assays of harbour oil spill bioremediation: a microscale simulation study. J. Appl. Microbiol. 102, 184–194. doi: 10.1111/j.1365-2672.2006.03071.x
Carissimi, M., de Souza, T. F., Corbellini, V. A., and Scroferneker, M. L. (2007). Comparison of lipolytic activity of Sporothrix schenckii strains utilizing olive oil-rhodamine B and Tween 80. Tecno Lógica 11, 33–36.
Castro, L. V., and Vazquez, F. (2009). Fractionation and characterization of Mexican crude oils. Energy Fuels 23, 1603–1609. doi: 10.1021/ef8008508
Center for Integrated Modeling and Analysis of Gulf Ecosystems (C-IMAGE) (2020). Available online at: https://www.marine.usf.edu/c-image/ (Accessed July 21, 2020).
Cerqueda-García, D., García-Maldonado, J. Q., Aguirre-Macedo, L., and García-Cruz, U. (2020). A succession of marine bacterial communities in batch reactor experiments during the degradation of five different petroleum types. Mar. Poll. Bull. 150:110775. doi: 10.1016/j.marpolbul.2019.110775
Cherry, J. R., and Fidantsef, A. L. (2003). Directed evolution of industrial enzymes: an update. Curr. Opin. Biotechnol. 14, 438–443. doi: 10.1016/s0958-1669(03)00099-5
Chronopoulou, P. M., Sanni, G. O., Silas-Olu, D. I., van der Meer, J. R., Timmis, K. N., Brussaard, C. P. D., et al. (2015). Generalist hydrocarbon-degrading bacterial communities in the oil-polluted water column of the North Sea. Microb. Biotechnol. 8, 434–447. doi: 10.1111/1751-7915.12176
Clark, M. R., Consalvey, M., and Rowden, A. A. (2016). Biological Sampling in the Deep Sea. Chichester: John Wiley & Sons.
Consorcio de Investigación del Golfo de México (CIGoM) (2020). Available online at: https://cigom.org/ (accessed July 15, 2020).
Consortium Coastal River-Dominated Ecosystems (CONCORDE) (2020). Available online at: https://www.con-corde.org/ (accessed September 9, 2020).
Consortium for Simulation of Oil-Microbial Interactions in the Ocean (CSOMIO) (2020). Available online at: https://csomio.org/ (accessed September 9, 2020).
Crossland, N. O., and La Point, T. W. (1992). The design of mesocosm experiments. Environ. Toxicol. Chem. 11, 1–4. doi: 10.1002/etc.5620110101
D’Andrea, M. A., and Reddy, G. K. (2013). Health consequences among subjects involved in Gulf Oil spill clean-up activities. Am. J. Med. 126, 966–974. doi: 10.1016/j.amjmed.2013.05.014
D’Andrea, M. A., and Reddy, G. K. (2018). The development of long-term adverse health effects in oil spill cleanup workers of the deepwater horizon offshore drilling rig disaster. Front. Public Health 6:117. doi: 10.3389/fpubh.2018.00117
Dalmaso, G. Z. L., Ferreira, D., and Vermelho, A. B. (2015). Marine extremophiles a source of hydrolases for biotechnological applications. Mar. Drugs 13, 1925–1965. doi: 10.3390/md13041925
Dalsøren, S. B., Endresen, Ø, Isaksen, I. S. A., Gravir, G., and Sørgard, E. (2007). Environmental impacts of the expected increase in sea transportation, with a particular focus on oil and gas scenarios for Norway and northwest Russia. J. Geophys. Res. 112:D02310. doi: 10.1029/2005JD006927
Dañobeitia, J. J., Pouliquen, S., Johannessen, T., Basset, A., Cannat, M., Pfeil, B. G., et al. (2020). Toward a comprehensive and integrated strategy of the European marine research infrastructures for ocean observations. Front. Mar. Sci. 7:180. doi: 10.3389/fmars.2020.00180
Das, N., and Chandran, P. (2011). Microbial degradation of petroleum hydrocarbon contaminants: an overview. Biotechnol. Res. Int. 2011:941810. doi: 10.4061/2011/941810
De Beukelaer, S. M., MacDonald, I. R., Guinnasso, N. L., and Murray, J. A. (2003). Distinct side-scan sonar, RADARSAT SAR, and acoustic profiler signatures of gas and oil seeps on the Gulf of Mexico slope. Geo. Mar. Lett. 23, 177–186. doi: 10.1007/s00367-003-0139-9
De Jonge, H., Freijer, J. I., Verstraten, J. M., Westerveld, J., and Van der Wielen, F. M. (1997). Relation between bioavailability and fuel oil hydrocarbon composition in contaminated soils. Environ. Sci. Technol. 31, 771–775. doi: 10.1021/es960456+
Delille, D., and Coulon, F. (2008). Comparative mesocosm study of biostimulation efficiency in two different oil-amended sub-antarctic soils. Microb. Ecol. 56, 243–252. doi: 10.1007/s00248-007-9341-z
Dellagnezze, B. M., Vasconcellos, S. P., Angelim, A. L., Melo, V. M. M., Santisi, S., Cappello, S., et al. (2016). Bioaugmentation strategy employing a microbial consortium immobilized in chitosan beads for oil degradation in mesocosm scale. Mar. Pollut. Bull. 107, 107–117. doi: 10.1016/j.marpolbul.2016.04.011
Diaz, J. H. (2011). The legacy of the Gulf oil spill: analyzing acute public health effects and predicting chronic ones in Louisiana. Am. J. Disaster. Med. 6, 5–22. doi: 10.5055/ajdm.2011.0040
Du, Z.-J., Wang, Z.-J., Zhao, J.-X., and Chen, G.-J. (2016). Woeseia oceani gen. nov., sp. nov., a chemoheterotrophic member of the order chromatiales, and proposal of woeseiaceae fam. nov. Int. J. Syst. Evol. Microbiol. 66, 107–112. doi: 10.1099/ijsem.0.000683
Dubansky, B., Whitehead, A., Miller, J. T., Rice, C. D., and Galvez, F. (2013). Multitissue molecular, genomic, and developmental effects of the deepwater horizon oil spill on resident Gulf Killifish (Fundulus grandis). Environ. Sci. Technol. 47, 5074–5082. doi: 10.1021/es400458p
Ecosystem impacts of Oil and Gas Inputs to the Gulf (ECOGIG) (2020). Available online at: https://www.ecogig.org/ (accessed July 10, 2020)
Escobar-Zepeda, A., Godoy-Lozano, E. E., Raggi, L., Segovia, L., Merino, E., Gutiérrez-Rios, R. M., et al. (2018). Analysis of sequencing strategies and tools for taxonomic annotation: defining standards for progressive metagenomics. Sci. Rep. 8:12034. doi: 10.1038/s41598-018-30515-5
Escobedo-Hinojosa, W., and Pardo-López, L. (2017). Analysis of bacterial metagenomes from the Southwestern Gulf of Mexico for pathogens detection. Pathog. Dis. 75, 1–9. doi: 10.1093/femspd/ftx058
Fernández-Arrojo, L., Guazzaroni, M. E., López-Cortés, N., Beloqui, A., and Ferrer, M. (2010). Metagenomic era for biocatalyst identification. Curr. Opin. Biotechnol. 21, 725–733. doi: 10.1016/j.copbio.2010.09.006
Fondi, M., Karkman, A., Tamminen, M. V., Bosi, E., Virta, M., Fani, R., et al. (2016). “Every gene is everywhere but the environment selects”: global geolocalization of gene sharing in environmental samples through network analysis. Genome Biol. Evol. 8, 1388–1400. doi: 10.1093/gbe/evw077
García-Cruz, N. U., Sánchez-Avila, J. I., Valdés-Lozano, D., Gold-Bouchot, G., and Aguirre-Macedo, L. (2018). Biodegradation of hexadecane using sediments from rivers and lagoons of the Southern Gulf of Mexico. Mar. Pollut. Bull. 128, 202–207. doi: 10.1016/j.marpolbul.2018.01.026
Garcia-Galan, C., Berenguer-Murcia, A., Fernandez-Lafuente, R., and Rodrigues, R. C. (2011). Potential of different enzyme immobilization strategies to improve enzyme performance. Adv. Synth. Catal. 353, 2885–2904. doi: 10.1002/adsc.201100534
Garrity, G. M., Bell, J. A., and Lilburn, T. G. (2004). Taxonomic Outline of the Prokaryotes. Bergey’s Manual of Systematic Bacteriology, 2nd Edn. New York: Springer-Verlag.
Gentili, A. R., Cubitto, M. A., Ferrero, M., and Rodriguéz, M. S. (2006). Bioremediation of crude oil polluted seawater by a hydrocarbon-degrading bacterial strain immobilized on chitin and chitosan flakes. Int. Biodeterior. Biodegradation 57, 222–228. doi: 10.1016/j.ibiod.2006.02.009
Gianfreda, L., and Bollag, J.-M. (2002). “Isolated enzymes for the transformation and detoxification of organic pollutants,” in Enzymes in the Environment: Activity, Ecology and Applications, eds R. G. Burns and R. Dick (New York, NY: Marcel Dekker), 491–538.
Gianfreda, L., and Rao, M. A. (2008). “Bonifica di suoli contaminati e depurazione dell’acqua,” in Agrofarmaci. Conoscenze per un Uso Sostenibile, eds M. Gennari and M. Trevisan (Bologna: Oasi Alberto Perdisa), 521–564.
Giovannoni, S. J. (2017). SAR11 bacteria: the most abundant plankton in the oceans. Ann. Rev. Mar. Sci. 9, 231–255. doi: 10.1146/annurev-marine-010814-015934
Glogauer, A., Martini, V. P., Faoro, H., Couto, G. H., Müller-Santos, M., Monteiro, R. A., et al. (2011). Identification and characterization of a new true lipase isolated through metagenomic approach. Microb. Cell Fact. 10:54. doi: 10.1186/1475-2859-10-54
Glover, A. G., Dahlgren, T. G., Wiklund, H., Mohrbeck, I., and Smith, C. R. (2015). An end-to-end DNA taxonomy methodology for benthic biodiversity survey in the Clarion-Clipperton Zone, central Pacific abyss. J. Mar. Sci. Eng. 4:2. doi: 10.3390/jmse4010002
Godoy-Lozano, E. E., Escobar-Zepeda, A., Raggi, L., Merino, E., Gutierrez-Rios, R. M., Juarez, K., et al. (2018). Bacterial diversity and the geochemical landscape in the Southwestern Gulf of Mexico. Front. Microbiol. 9:2528. doi: 10.3389/fmicb.2018.02528
Goldstein, B. D., Osofsky, H. J., and Lichtveld, M. Y. (2011). The Gulf oil spill. N. Engl. J. Med. 364, 1334–1348. doi: 10.1056/NEJMra1007197
Gracia, A. (2010). Campaña Oceanográfica (SGM-2010). Informe Final. Gerencia de Seguridad Industrial, Protección Ambiental y Calidad Región Marina Noreste, PEMEX – EXPLORACIÓN – PRODUCCIÓN. México: Instituto de Ciencias del Mar y Limnología, UNAM.
Gregson, B. H., McKew, B. A., Holland, R. D., Nedwed, T. J., Prince, R. C., and McGenity, T. J. (2021). Marine oil snow, a microbial perspective. Front. Mar. Sci. 8:619484. doi: 10.3389/fmars.2021.619484
Griffiths, S. K. (2012). Oil release from Macondo well MC252 following the deepwater horizon accident. Environ. Sci. Technol. 46, 5616–5622. doi: 10.1021/es204569t
Gulf of Mexico Research Initiative (GoMRI) (2020). Available online at: https://gulfresearchinitiative.org/ (accessed September 7, 2020).
Gutierrez, T., and Aitken, M. D. (2014). Role of methylotrophs in the degradation of hydrocarbons during the deepwater horizon oil spill. ISME J. 8, 2543–2545. doi: 10.1038/ismej.2014.88
Gutierrez, T., Morris, G., Ellis, D., Bowler, B., Jones, M., Salek, K., et al. (2018). Hydrocarbon-degradation and MOS-formation capabilities of the dominant bacteria enriched in sea surface oil slicks during the Deepwater Horizon oil spill. Mar. Pollut. Bull. 135, 205–215. doi: 10.1016/j.marpolbul.2018.07.027
Gutierrez, T., Singleton, D. R., Berry, D., Yang, T., Aitken, M. D., and Teske, A. (2013). Hydrocarbon-degrading bacteria enriched by the deepwater horizon oil spill identified by cultivation and DNA-SIP. ISME J. 7, 2091–2104. doi: 10.1038/ismej.2013.98
Guzik, U., Hupert-Kocurek, K., Krysiak, M., and Wojcieszyńska, D. (2014). Degradation potential of protocatechuate 3,4-dioxygenase from crude extract of Stenotrophomonas maltophilia strain KB2 immobilized in calcium alginate hydrogels and on glyoxyl agarose. BioMed Res. Int. 2014:138768.
Harayama, S., Kishira, H., Kasai, Y., and Shutsubo, L. (1999). Petroleum biodegradation in marine environments. J. Mol. Microbiol. Biotechnol. 1, 63–70.
Harms, H., and Bosma, T. N. P. (1997). Mass transfer limitation of microbial growth and pollutant degradation. J. Ind. Microbiol. Biotechnol. 18, 97–105. doi: 10.1038/sj.jim.2900259
Hassanshahian, M., Emtiazi, G., Caruso, G., and Capello, S. (2014). Bioremediation (bioaugmentation/biostimulation) trials of oil polluted sea. water: a mesocosm simulation study. Mar. Environ. Res. 95, 28–38. doi: 10.1016/j.marenvres.2013.12.010
Hausmann, S., and Jaeger, K.-E. (2010). “Lipolytic enzymes from bacteria,” in Handbook of Hydrocarbon and Lipid Microbiology, ed. K. N. Timmis (Berlin: Springer-Verlag), 1099–1126. doi: 10.1007/978-3-540-77587-4_77
Hazen, T. C., Dubinsky, E. A., DeSantis, T. Z., Andersen, G. L., Piceno, Y. M., Singh, N., et al. (2010). Deep-sea oil plume enriches indigenous oil-degrading bacteria. Science 330, 204–208. doi: 10.1126/science.1195979
Head, I. M., Gray, N. D., and Larter, S. R. (2014). Life in the slow lane; biogeochemistry of biodegraded petroleum containing reservoirs and implications for energy recovery and carbon management. Front. Microbiol. 5:556. doi: 10.3389/fmicb.2014.00566
Head, I. M., Jones, D. M., and Roling, W. F. M. (2006). Marine microorganisms make a meal of oil. Nat. Rev. Microbiol. 4, 173–182. doi: 10.1038/nrmicro1348
Hess, M., Sczyrba, A., Egan, R., Kim, T. W., Chokhawala, H., Schroth, G., et al. (2011). Metagenomic discovery of biomass-degrading genes and genomes from cow rumen. Science 331, 463–467. doi: 10.1126/science.1200387
Hodson, R. E., Azam, F., and Lee, R. F. (1977). Effects of four oils on marine bacterial populations: controlled ecosystem pollution experiment. Bull. Mar. Sci. 27, 119–126.
Hoshino, T., Doi, H., Uramoto, G. I., Wörmer, L., Adhikari, R. R., Xiao, N., et al. (2020). Global diversity of microbial communities in marine sediment. Proc. Natl. Acad. Sci. U.S.A. 117, 27587–27597. doi: 10.1073/pnas.1919139117
Huesemann, M. H., Hausmann, T. S., and Fortman, T. J. (2004). Does bioavailability limit biodegradation? A comparison of hydrocarbon biodegradation and desorption rates in aged soils. Biodegradation 15, 261–274. doi: 10.1023/b:biod.0000042996.03551.f4
Itoh, T., Yamanoi, K., Kudo, T., Ohkuma, M., and Takashina, T. (2011). Aciditerrimonas ferrireducens gen. nov., sp. nov., an iron-reducing thermoacidophilic actinobacterium isolated from a solfataric field. Int. J. Syst. Evol. Microbiol. 61, 1281–1285. doi: 10.1099/ijs.0.023044-0
Jernelöv, A., and Lindén, O. (1981). Ixtoc I: a case study of the world’s largest oil spill. Ambio 10, 299–306.
Jiménez, D. J., Dini-Andreote, F., Ottoni, J. R., de Oliveira, V. M., van Elsas, J. D., and Andreote, F. D. (2015). Compositional profile of α / β-hydrolase fold proteins in mangrove soil metagenomes: prevalence of epoxide hydrolases and haloalkane dehalogenases in oil-contaminated sites. Microb. Biotechnol. 8, 604–613. doi: 10.1111/1751-7915.12157
Jin, H. M., Kim, J. M., Lee, H. J., Madsen, E. L., and Jeon, C. O. (2012). Alteromonas as a key agent of polycyclic aromatic hydrocarbon biodegradation in crude oil-contaminated coastal sediment. Environ. Sci. Technol. 46, 7731–7740. doi: 10.1021/es3018545
Johansen, C., Macelloni, L., Natter, M., Silva, M., Woosley, M., Woolsey, A., et al. (2020). Hydrocarbon migration pathway and methane budget for a Gulf of Mexico natural seep site: Green Canyon 600. Earth Planet. Sci. Lett. 545:116411. doi: 10.1016/j.epsl.2020.116411
Kadali, K. K., Simons, K. L., Sheppard, P. J., and Ball, A. (2012). Mineralization of weathered crude oil by a hydrocarbonoclastic consortia in marine mesocosms. Water Air Soil Pollut. 223, 4283–4295. doi: 10.1007/s11270-012-1191-8
Kadri, T., Cuprys, A., Rouissi, T., Brar, S. K., Daghrir, R., and Lauzon, J.-M. (2018). Nanoencapsulation and release study of enzymes from Alkanivorax borkumensis in chitosan-tripolyphosphate formulation. Biochem. Eng. J. 137, 1–10. doi: 10.1016/j.bej.2018.05.013
Karam, J., and Nicell, J. A. (1997). Potential application of enzymes in waste treatment. J. Chem. Technol. Biotechnol. 69, 141–153. doi: 10.1002/(SICI)1097-4660(199706)69:2<141::AID-JCTB694>3.0.CO;2-U
Karthikeyan, S., Rodriguez-R, L. M., Heritier-Robbins, P., Hatt, J. K., Huettel, M., Kostka, J. E., et al. (2020). Genome repository of oil systems: an interactive and searchable database that expands the catalogued diversity of crude oil-associated microbes. Environ. Microbiol. 22, 2094–2106. doi: 10.1111/1462-2920.14966
Katchalski, E., Silman, I., and Goldman, R. (1971). Effect of the microenvironment on the mode of action of immobilized enzymes. Adv. Enzymol. Relat. Areas Mol. Biol. 34, 445–536.
Kimes, N. E., Callaghan, A. V., Aktas, D. F., Smith, W. L., Sunner, J., Golding, B. T., et al. (2013). Metagenomic analysis and metabolite profiling of deep-sea sediments from the Gulf of Mexico following the deepwater horizon oil spill. Front. Microbiol. 4:50. doi: 10.3389/fmicb.2013.00050
Kimes, N. E., Callaghan, A. V., Suflita, J. M., and Morris, P. J. (2014). Microbial transformation of the deepwater horizon oil spill-past, present, and future perspectives. Front. Microbiol. 5:603. doi: 10.3389/fmicb.2014.00603
King, G. M., Smith, C. B., Tolar, B., and Hollibaugh, J. T. (2013). Analysis of composition and structure of coastal to mesopelagic bacterioplankton communities in the northern Gulf of Mexico. Front. Microbiol. 3:438. doi: 10.3389/fmicb.2012.00438
Kleindienst, S., Grim, S., Sogin, M., Bracco, A., Crespo-Medina, M., and Joye, S. B. (2015). Diverse, rare microbial taxa responded to the Deepwater Horizon deep-sea hydrocarbon plume. ISME J. 10, 400–415. doi: 10.1038/ismej.2015.121
Kostka, J. E., Prakash, O., Overholt, W. A., Green, S. J., Freyer, G., Canion, A., et al. (2011). Hydrocarbon-degrading bacteria and the bacterial community response in Gulf of Mexico beach sands impacted by the deepwater horizon oil spill. Appl. Environ. Microbiol. 77, 7962–7974. doi: 10.1128/AEM.05402-11
Kube, M., Chernikova, T. N., Al-Ramahi, Y., Beloqui, A., Lopez-Cortez, N., Guazzaroni, M. E., et al. (2013). Genome sequence and functional genomic analysis of the oil-degrading bacterium Oleispira antarctica. Nat. Commun. 4:2156. doi: 10.1038/ncomms3156
Lee, H. S., Kwon, K. K., Kang, S. G., Cha, S. S., Kim, S. J., and Lee, J. H. (2010). Approaches for novel enzyme discovery from marine environments. Curr. Opin. Biotechnol. 21, 353–357. doi: 10.1016/j.copbio.2010.01.015
Linacre, L., Durazo, R., Camacho-Ibar, V. F., Selph, K. E., Lara-Lara, J. R., Mirabal-Gómez, U., et al. (2019). Picoplankton carbon biomass assessments and distribution of Prochlorococcus ecotypes linked to loop current eddies during summer in the southern Gulf of Mexico. J. Geophys. Res. Ocean. 124, 8342–8359. doi: 10.1029/2019JC015103
Liu, J., Bacosa, H. P., and Liu, Z. (2017). Potential environmental factors affecting oil-degrading bacterial populations in deep and surface waters of the Northern Gulf of Mexico. Front. Microbiol. 7:2131. doi: 10.3389/fmicb.2016.02131
Liu, Z., and Liu, J. (2013). Evaluating bacterial community structures in oil collected from the sea surface and sediment in the northern Gulf of Mexico after the deepwater horizon oil spill. Microbiologyopen 2, 492–504. doi: 10.1002/mbo3.89
Lizárraga-Partida, M. L., Hernández López, E. L., Gasperin Bulbarela, J., Bernáldez Sarabia, J., Licea Navarro, A. F., and Guerrero, A. (2019). Detection of Alcanivorax spp., Cycloclasticus spp., and Methanomicrobiales in water column and sediment samples in the Gulf of Mexico by qPCR. Environ. Sci. Pollut. Res. 26, 35131–35139. doi: 10.1007/s1156-019-06551-7
Lizárraga-Partida, M. L., Izquierdo-Vicuña, F., and Wong-Chang, I. (1991). Marine bacteria on the Campeche Bank Oil field. Mar. Pollut. Bull. 22, 401–405. doi: 10.1016/0025-326X(91)90344-R
Lizárraga-Partida, M. L., Porras-Aguirre, J., Izquierdo-Vicuña, F. B., and Rosano-Hernandez, M. C. (1986). Bacteriology of the southern Gulf of Mexico and Yucatan channel area. Ciencias Mar. 12, 21–34. doi: 10.7773/cm.v12i2.511
Lizárraga-Partida, M. L., Rodriguez-Santiago, H., and Romero-Jarer, J. M. (1982). Effects of the Ixtoc I Blowout on heterotrophic bacteria. Mar. Pollut. Bull. 13, 67–70. doi: 10.1016/0025-326X(82)90445-3
Looper, J. K., Cotto, A., Kim, B. Y., Lee, M. K., Liles, M. R., Ní Chadhain, S. M., et al. (2013). Microbial community analysis of Deepwater Horizon oil-spill impacted sites along the Gulf coast using functional and phylogenetic markers. Environ. Sci. Process. Impacts 15, 2068–2079. doi: 10.1039/c3em00200d
MacDonald, I. R., Garcia-Pineda, O., Beet, A., Daneshgar, A. S., Feng, L., Graettinger, G., et al. (2015). Natural and unnatural oil slicks in the Gulf of Mexico. J. Geophys. Res. Oceans. 120, 8364–8380. doi: 10.1002/2015JC011062
Magoč, T., and Salzberg, S. L. (2011). FLASH: fast length adjustment of short reads to improve genome assemblies. Bioinformatics 27, 2957–2963. doi: 10.1093/bioinformatics/btr507
Marietou, A., Chastain, R., Beulig, F., Scoma, A., Hazen, T. C., and Bartlett, D. H. (2018). The effect of hydrostatic pressure on enrichments of hydrocarbon degrading microbes from the Gulf of Mexico following the deepwater horizon oil spill. Front. Microbiol. 9:808. doi: 10.3389/fmicb.2018.00808
Mason, O. U., Han, J., Woyke, T., and Jansson, J. K. (2014). Single-cell genomics reveals features of a Colwellia species that was dominant during the deepwater horizon oil spill. Front. Microbiol. 5:332. doi: 10.3389/fmicb.2014.00332
Mason, O. U., Hazen, T. C., Borglin, S., Chain, P. S., Dubinsky, E. A., Fortney, J. L., et al. (2012). Metagenome, metatranscriptome and single-cell sequencing reveal microbial response to deepwater horizon oil spill. ISME J. 6, 1715–1727. doi: 10.1038/ismej.2012.59
Math, R. K., Jin, H. M., Kim, J. M., Hahn, Y., Park, W., Madsen, E. L., et al. (2012). Comparative genomics reveals adaptation by Alteromonas sp. SN2 to marine tidal-flat conditions: cold tolerance and aromatic hydrocarbon metabolism. PLoS One 7:e35784. doi: 10.1371/journal.pone.0035784
Michel, J., Owens, E. H., Zengel, S., Graham, A., Nixon, Z., Allard, T., et al. (2013). Extent and Degree of shoreline oiling: deepwater horizon oil spill, Gulf of Mexico, USA. PLoS One 8:e65087. doi: 10.1371/journal.pone.0065087
Mora, C., Tittensor, D. P., Adl, S., Simpson, A. G., and Worm, B. (2011). How many species are there on Earth and in the ocean? PLoS Biol. 9:e1001127. doi: 10.1371/journal.pbio.1001127
Moreno-Ulloa, A., Sicairos-Diaz, V., Tejeda-Mora, J. A., Macias-Contreras, M. I., Díaz-Castillo, F., Guerrero, A., et al. (2020). Metabolic and metagenomic profiling of hydrocarbon-degrading microorganisms obtained from the deep biosphere of the Gulf of México. mSystems 5, 1–20. doi: 10.1101/606806
Muriel-Millán, L. F., Rodríguez-Mejía, J. L., Godoy-Lozano, E. E., Rivera-Gómez, N., Gutierrez-Rios, R.-M., Morales-Guzmán, D., et al. (2019). Functional and genomic characterization of a Pseudomonas aeruginosa strain isolated from the southwestern Gulf of Mexico reveals an enhanced adaptation for long-chain alkane degradation. Front. Mar. Sci. 6:572. doi: 10.3389/fmars.2019.00572
National Research Council (NRC) (2003). Oil in the Sea III: Inputs, Fates and Effects. Washington, DC: National Academy Press, 280.
Ngo, T. T. (1980). Bioanalytical applications of immobilized enzymes. Int. J. Biochem. 1, 459–465. doi: 10.1016/0020-711X(80)90253-0
Nicell, J. A. (2001). Environmental applications of enzymes. Interdisc. Environ. Rev. 3, 14–41. doi: 10.1504/IER.2001.053866
Nie, M., Nie, H., He, M., Lin, Y., Wang, L., Jin, P., et al. (2016). Immobilization of biofilms of Pseudomonas aeruginosa NY3 and their application in the removal of hydrocarbons from highly concentrated oil-containing wastewater on the laboratory scale. J. Environ. Manage. 173, 34–40. doi: 10.1016/j.jenvman.2016.02.045
OceanObs (2019). Conference Statement. Available online at: http://www.oceanobs19.net/statement/ (accessed June 10, 2020)
Ondov, B. D., Bergman, N. H., and Phillippy, A. M. (2011). Interactive metagenomic visualization in a Web browser. BMC Bioinformatics 12:385. doi: 10.1186/1471-2105-12-385
Orcutt, B. N., Lapham, L. L., Delaney, J., Sarode, N., Marshall, K. S., Whaley-Martin, K. J., et al. (2017). Microbial response to oil enrichment in Gulf of Mexico sediment measured using a novel long-term benthic lander system. Elem. Sci. Anth. 5:18. doi: 10.1525/elementa.129
Overton, E. B., Wade, T. L., Radović, J. R., Meyer, B. M., Miles, M. S., and Larter, S. R. (2016). Chemical composition of Macondo and other crude oils and compositional alterations during oil spills. Oceanography 29, 50–63. doi: 10.5670/oceanog.2016.62
Pardo-López, L. (2019). “Marine bioprospecting,” in Marine and Fisheries Policies in Latin America: A Comparison of Selected Countries, eds M. R. Muller, R. Oyanedel, and B. Monteferri (Abingdon-on-Thames: Routledge), 192. doi: 10.4324/9780429426520
Peres, L. C., Trapido, E., Rung, A. L., Harrington, D. J., Oral, E., Fang, Z., et al. (2016). The deepwater horizon oil spill and physical health among adult women in southern Louisiana: the women and their children’s health (WaTCH) study. Environ. Health Perspect. 124, 1208–1213. doi: 10.1289/ehp.1510348
Pomeroy, L. R., Sheldon, J. E., Sheldon, W. M., and Peters, F. (1995). Limits to growth and respiration of bacterioplankton in the Gulf of Mexico. Mar. Ecol. Prog. Ser. 117, 259–268.
Prince, R. C. (2010). “Bioremediation of marine oil spills,” in Handbook of Hydrocarbon and Lipid Microbiology, ed. K. N. Timmis (Berlin: Springer), 2597–2608. doi: 10.1007/978-3-540-77587-4_194
Prince, R. C., Gramain, A., and McGenity, T. J. (2010). “Prokaryotic hydrocarbon degraders,” in Handbook of Hydrocarbon and Lipid Microbiology, ed. K. N. Timmis (Berlin: Springer), 1669–1692. doi: 10.1007/978-3-540-77587-4_118
Prince, R. C., McFarlin, K. M., Butler, J. D., Febbo, E. J., Wang, F. C. Y., and Nedwed, T. J. (2013). The primary biodegradation of dispersed crude oil in the sea. Chemosphere 90, 521-526.
Pulster, E. L., Gracia, A., Armenteros, M., Carr, B. E., Mrowicki, J., and Murawski, S. A. (2020a). Chronic PAH exposures and associated declines in fish health indices observed for ten grouper species in the Gulf of Mexico. Sci. Total Environ. 703:135551. doi: 10.1016/j.scitotenv.2019.135551
Pulster, E. L., Gracia, A., Armenteros, M., Toro-Farmer, G., Snyder, S. M., Carr, B. E., et al. (2020b). A first comprehensive baseline of hydrocarbon pollution in Gulf of Mexico fishes. Sci. Rep. 10:6437. doi: 10.1038/s41598-020-62944-6
Qiao, K., Tian, W., Bai, J., Wang, L., Zhao, J., Song, T., et al. (2020). Removal of high-molecular-weight polycyclic aromatic hydrocarbons by a microbial consortium immobilized in magnetic floating biochar gel beads. Mar. Pollut. Bull. 159:111489. doi: 10.1016/j.marpolbul.2020.111489
Rabone, M., Harden-Davies, H., Collins, J. E., Zajderman, S., Appeltans, W., Droege, G., et al. (2019). Access to marine genetic resources (MGR): raising awareness of best-practice through a new agreement for biodiversity beyond national jurisdiction (BBNJ). Front. Mar. Sci. 6:520. doi: 10.3389/fmars.2019.00520
Raggi, L., García-Guevara, F., Godoy-Lozano, E. E., Martínez-Santana, A., Escobar-Zepeda, A., Gutierrez-Rios, R. M., et al. (2020). Metagenomic profiling and microbial metabolic potential of perdido fold belt (NW) and campeche knolls (SE) in the Gulf of Mexico. Front. Microbiol. 11:1825. doi: 10.3389/fmicb.2020.01825
Rahman, R. N., Ghaza, F. M., Salleh, A. B., and Basri, M. (2006). Biodegradation of hydrocarbon contamination by immobilized bacterial cells. J. Microbiol. 44, 354–359.
Ramírez, D., Vega-Alvarado, L., Taboada, B., Estradas-Romero, A., Soto, L., and Juárez, K. (2020). Bacterial diversity in surface sediments from the continental shelf and slope of the North West gulf of Mexico and the presence of hydrocarbon degrading bacteria. Mar. Pollut Bull. 150:110590. doi: 10.1016/j.marpolbul2019.110590
Redmond, M. C., and Valentine, D. L. (2012). Natural gas and temperature structured a microbial community response to the deepwater horizon oil spill. Proc. Natl. Acad. Sci. U.S.A. 109, 20292–20297. doi: 10.1073/pnas.1108756108
Rodríguez-Salazar, J., Almeida-Juarez, A. G., Ornelas-Ocampo, K., Millán-López, S., Raga-Carbajal, E., Rodríguez-Mejía, J. L., et al. (2020). Characterization of a novel functional trimeric catechol 1,2-dioxygenase from a Pseudomonas stutzeri isolated from the Gulf of Mexico. Front. Microbiol. 11:1100. doi: 10.3389/fmicb.2020.01100
Ron, E. Z., and Rosenberg, E. (2014). Enhanced bioremediation of oil spills in the sea. Curr. Opin. Biotech. 27, 191–194. doi: 10.1016/j.copbio.2014.02.004
Rosano-Hernandez, M. C., Fernandez-Linares, L. C., and Xoconostle-Cazares, B. (2009). Bacterial diversity of marine seeps in the southeastern Gulf of Mexico. Pak. J. Biol. Sci. 12, 683–689. doi: 10.3923/pjbs.2009.683.689
Rosenberg, E., DeLong, E. F., Lory, S., Stackebrandt, E., and Thompson, F. (2014). The Prokaryotes. Berlin: Springer-Verlag.
Rotter, A., Bacu, A., Barbier, M., Bertoni, F., Bones, A. M., Cancela, M. L., et al. (2020). A new network for the advancement of marine biotechnology in Europe and beyond. Front. Mar. Sci. 7:278. doi: 10.3389/fmars.2020.00278
Sánchez-Soto Jiménez, M. F., Cerqueda-García, D., Montero-Muñoz, J. L., Aguirre-Macedo, M. L., and García-Maldonado, J. Q. (2018). Assessment of the bacterial community structure in shallow and deep sediments of the Perdido Fold Belt region in the Gulf of Mexico. Peer J. 6:e5583. doi: 10.7717/peerj.5583
Santas, R., Korda, A., Tenente, A., Buchhloz, K., and Santas, P. (1999). Mesocosm assays of oil spill bioremediation with oleophilic fertilizers: inipol, F1 or both? Mar. Pollut. Bull. 38, 44–48. doi: 10.1016/S0025-326X(99)80011-3
Scanlan, D. J., and West, N. J. (2002). Molecular ecology of the marine cyanobacterial genera Prochlorococcus and Synechococcus. FEMS Microbiol. Ecol. 40, 1–12. doi: 10.1111/j.1574-6941.2002.tb00930.x
Schink, B. (1984). Fermentation of 2,3-butanediol by Pelobacter carbinolicus sp.nov. and Pelobacter propionicus sp. nov., and evidence for propionate formation from C2 compounds. Arch. Microbiol. 137, 33–41. doi: 10.1007/BF00425804
Schnell, S., and Hanson, S. M. (2007). A test for measuring the effects of enzyme inactivation. Biophys. Chem. 125, 269–274. doi: 10.1016/j.bpc.2006.08.010
Shamsipur, M., Asgari, M., Maragheh, M. G., and Moosavi-Movahedi, A. A. (2012). A novel impedimetric nanobiosensor for low level determination of hydrogen peroxide based on biocatalysis of catalase. Bioelectrochemistry 83, 31–37. doi: 10.1016/j.bioelechem.2011.08.003
Shen, H., Perrie, W., and Wu, Y. (2019). Wind drag in oil spilled ocean surface and its impact on wind-driven circulation. Can. Sci. Publ. 2, 244–260. doi: 10.1139/anc-2018-0019
Soto, L. A., Botello, A. V., Licea-Durán, S., Lizárraga-Partida, M. L., and Yáñez-Arancibia, A. (2014). The environmental legacy of the Ixtoc-I oil spill in Campeche sound, Southwestern Gulf of Mexico. Front. Mar. Sci. 1:57. doi: 10.3389/fmars.2014.00057
Sticher, P., Jaspers, M. C., Stemmler, K., Harms, H., Zehnder, A. J., and Van der Meer, J. R. (1997). Development and characterization of a whole-cell bioluminescent sensor for bioavailable middle-chain alkanes in contaminated groundwater samples. Appl. Environ. Microbiol. 63, 4053–4060.
Stiner, L., and Halverson, L. J. (2002). Development and characterization of a green fluorescent protein-based bacterial biosensor for bioavailable toluene and related compounds. Appl. Environ. Microbiol. 68, 1962–1971. doi: 10.1128/aem.68.4.1962-1971.2002
Su, X., Pan, W., Song, B., Xu, J., and Ning, K. (2014). Parallel-META 2.0: enhanced metagenomic data analysis with functional annotation, high performance computing and advanced visualization. PLoS One 9:e89323. doi: 10.1371/journal.pone.0089323
Suma, Y., Kang, C. S., and Kim, H. S. (2015). Noncovalent and covalent immobilization of oxygenase on single-walled carbon nanotube for enzymatic decomposition of aromatic hydrocarbon intermediates. Environ. Sci. Pollut. Res. 23, 1015–1024. doi: 10.1007/s11356-015-4168-5
Tao, K., Liu, X., Chen, X., Hu, X., Cao, L., and Yuan, X. (2017). Biodegradation of crude oil by a defined co-culture of indigenous bacterial consortium and exogenous Bacillus Subtilis. Bioresour. Technol. 224, 327–332. doi: 10.1016/j.biortech.2016.10.073
Tasse, L., Bercovici, J., Pizzut-Serin, S., Robe, P., Tap, J., Klopp, C., et al. (2010). Functional metagenomics to mine the human gut microbiome for dietary fiber catabolic enzymes. Genome Res. 20, 1605–1612. doi: 10.1101/gr.108332.110
Trindade, M., van Zyl, L. J., Navarro-Fernández, J., and Elrazak, A. A. (2015). Targeted metagenomics as a tool to tap into marine natural product diversity for the discovery and production of drug candidates. Front. Microbiol. 6:890. doi: 10.3389/fmicb.2015.00890
Uribe-Flores, M. M., Cerqueda-García, D., Hernández-Nuñez, E., Cadena, S., García-Cruz, N. U., Trejo-Hernández, M. R., et al. (2019). Bacterial succession and co-occurrence patterns of an enriched marine microbial community during light crude oil degradation in a batch reactor. J. Appl. Microbiol. 127, 495–507. doi: 10.1111/jam.14307
Valencia-Agami, S. S., Cerqueda-García, D., Putzeys, S., Uribe-Flores, M. M., García-Cruz, N. U., and Pech, D. (2019). Changes in the bacterioplankton community structure from the Southern Gulf of Mexico during a simulated crude oil spill at mesocosm Scale. Microorganisms 7:441. doi: 10.3390/microorganisms7100441
Van de Velde, F., Lourenço, N. D., Pinheiro, H. M., and Bakker, M. (2002). Carrageenan: a food-grade and biocompatible support for immobilisation techniques. Adv. Synth. Catal. 344, 815–835. doi: 10.1002/1615-4169(200209)344:8<815::aid-adsc815>3.0.co;2-h
Varjani, S. J. (2017). Microbial degradation of petroleum hydrocarbons. Bioresour. Technol. 223, 277–286. doi: 10.1016/j.biortech.2016.10.037
Venosa, A. D., and Zhu, X. (2003). Biodegradation of crude oil contaminating marine shorelines and freshwater wetlands. Spill Sci. Technol. Bull. 8, 163–178. doi: 10.1016/s1353-2561(03)00019-7
Vilcáez, J., Li, L., and Hubbard, S. S. (2013). A new model for the biodegradation kinetics of oil droplets: application to the deepwater horizon oil spill in the Gulf of Mexico. Geochem. Trans. 14:4. doi: 10.1186/1467-4866-14-4
Visbeck, M. (2018). Ocean science research is key for a sustainable future. Nat. Commun. 9:690. doi: 10.1038/s41467-018-03158-3
Wilson, G. S., and Hu, Y. (2000). Enzyme-based biosensors for in vivo measurements. Chem. Rev. 100, 2693–2704. doi: 10.1021/cr990003y
Xu, N., Bao, M., Sun, P., and Li, Y. (2013). Study on bioadsorption and biodegradation of petroleum hydrocarbons by a microbial consortium. Bioresour. Technol. 149, 22–30. doi: 10.1016/j.biortech.2013.09.024
Yakimov, M. M., Timmis, K. N., and Golyshin, P. N. (2007). Obligate oil-degrading marine bacteria. Curr. Opin. Biotech. 18, 257–266. doi: 10.1016/j.copbio.2007.04.006
Keywords: Gulf of Mexico, biodiversity, hydrocarbon-degrading bacteria, bioprospection, enzymatical biotechnology
Citation: Rodríguez-Salazar J, Loza A, Ornelas-Ocampo K, Gutierrez-Rios RM and Pardo-López L (2021) Bacteria From the Southern Gulf of Mexico: Baseline, Diversity, Hydrocarbon-Degrading Potential and Future Applications. Front. Mar. Sci. 8:625477. doi: 10.3389/fmars.2021.625477
Received: 03 November 2020; Accepted: 22 February 2021;
Published: 17 March 2021.
Edited by:
Tony Gutierrez, Heriot-Watt University, United KingdomReviewed by:
Smruthi Karthikeyan, University of California, San Diego, United StatesHernando Bacosa, Mindanao State University-Iligan Institute of Technology (MSU-IIT), Philippines
Copyright © 2021 Rodríguez-Salazar, Loza, Ornelas-Ocampo, Gutierrez-Rios and Pardo-López. This is an open-access article distributed under the terms of the Creative Commons Attribution License (CC BY). The use, distribution or reproduction in other forums is permitted, provided the original author(s) and the copyright owner(s) are credited and that the original publication in this journal is cited, in accordance with accepted academic practice. No use, distribution or reproduction is permitted which does not comply with these terms.
*Correspondence: Liliana Pardo-López, bGlsaWFuYS5wYXJkb0BpYnQudW5hbS5teA==
†These authors have contributed equally to this work