- 1Deltares, Delft, Netherlands
- 2United States Geological Survey, Santa Cruz, CA, United States
- 3IHE Delft Institute for Water Education, Delft, Netherlands
- 4Faculty of Civil Engineering and Geosciences, Delft University of Technology, Delft, Netherlands
Coral reefs are effective natural coastal flood barriers that protect adjacent communities. Coral degradation compromises the coastal protection value of reefs while also reducing their other ecosystem services, making them a target for restoration. Here we provide a physics-based evaluation of how coral restoration can reduce coastal flooding for various types of reefs. Wave-driven flooding reduction is greatest for broader, shallower restorations on the upper fore reef and between the middle of the reef flat and the shoreline than for deeper locations on the fore reef or at the reef crest. These results indicate that to increase the coastal hazard risk reduction potential of reef restoration, more physically robust species of coral need to be outplanted to shallower, more energetic locations than more fragile, faster-growing species primarily being grown in coral nurseries. The optimization and quantification of coral reef restoration efforts to reduce coastal flooding may open hazard risk reduction funding for conservation purposes.
Introduction
Coral reefs not only help sustain the economy of 500 million people in tropical coastal communities (Hoegh-Guldberg et al., 2019), but also protect them from wave-driven flooding and coastal erosion (Elliff and Silva, 2017; Reguero et al., 2018), especially in the face of climate change (Storlazzi et al., 2018). Coral reefs can substantially reduce coastal flooding by efficiently attenuating ocean wave energy (Ferrario et al., 2014). Over coral reefs, wave height rapidly decays by wave breaking, resulting in a mean surface elevation increase (setup) (Vetter et al., 2010). Waves are also damped by bottom friction caused by corals. At the shoreline, residual wave energy drives wave runup, which together with setup, results in coastal flooding that is greatest onshore of steep, narrow, and hydraulically smooth reefs (Quataert et al., 2015). The hydrodynamic behavior of coral reefs is generally well characterized by coastal engineering models (Van Dongeren et al., 2013; Taebi and Pattiaratchi, 2014; Quataert et al., 2015). Utilizing such models, the role of coral reefs in coastal hazard risk reduction has recently been assessed (Reguero et al., 2019; Storlazzi et al., 2019) and quantified to be in the order of $billions annually in the United States alone.
Coral degradation due to climate-change and anthropogenic activities has caused the loss of reef elevation (Yates et al., 2017) and roughness, thereby increasing coastal flooding hazards (Quataert et al., 2015). Coral reefs are under threat from both local stressors such as land-based pollution and overfishing (Carson et al., 2019) and global stressors such as global warming and ocean acidification (Pandolfi et al., 2011). The increasing frequency and magnitude of these impacts have retarded coral reefs’ natural ability to recover, contributing to an annual decline in coral cover of 1–2% (Bruno and Selig, 2007). To reverse this trend, coral restoration is increasingly being undertaken (Bostrom-Einarsson et al., 2020), and research efforts to support such measures have primarily focused on growth and outplanting techniques (Chan et al., 2018), monitoring (Montoya-Maya et al., 2016), upscaling of restoration works (Levy et al., 2018), long-term ecological resilience (Van Oppen et al., 2015), and reef management (Rinkevich, 2014; Lirman and Schopmeyer, 2016).
Coral reef restoration is suggested to reduce the flood risk to, and increase the resiliency of, tropical coastal communities (e.g., Ferrario et al., 2014; Beck and Lange, 2016). Ferrario et al. (2014) evaluated the wave attenuation capacity of entire coral reef features (including reef crest, reef flat), but noted both ecological and engineering (e.g., restoration location, height, and roughness) knowledge gaps in designing reef restoration projects for hazard mitigation. The current study tackles these knowledge gaps by providing practical guidelines to maximize restoration efforts for coastal risk reduction, as the large spatial scales of coral reefs and operational constraints of recovery efforts necessitate an efficient approach in designing and restoring coral reefs. We utilized a physics-based, hydrodynamic model to evaluate how the height, width, and relative location of a restoration on various reef morphologies found in nature influences the wave-driven runup reduction potential of the restoration to provide information on their hydrodynamic performance and to guide restoration design for coastal hazard risk reduction. Such information will significantly increase the efficiency of coral restoration efforts, assisting a range of stakeholders in their efforts on not only coral reef conservation and management, but also coastal hazard risk reduction, and possibly open new financing options for reef restoration via pre-disaster hazard mitigation funds or post-disaster restoration funds.
Materials and Methods
The extent to which coral restoration can enhance the protection of tropical coastal communities was investigated with a calibrated and validated physics-based hydrodynamic model that propagates offshore wave conditions over a one-dimensional reef profile with and without coral restorations to predict the wave-induced runup that leads to coastal flooding. First, coral reef profiles observed in nature were classified with a statistical tool into four dominant reef profile types. For each representative reef profile shape, coral reef restorations were designed based on operational and environmental constrictions. The effect of the different restorations on wave driven flooding was assessed with the XBeach model (Roelvink et al., 2009), using a wide variety of potential coral restoration configurations (different widths, heights, and locations), reef morphologies and hydrodynamic forcing conditions (water depths, wave heights, and wave periods).
Classification of Coral Reef Profiles
A global dataset of more than 30,000 coral reef profiles, encompassing a wide array of coral reef shapes and topographic features from the Pacific and Atlantic Oceans, was classified to obtain representative coral reef profile shapes. The dataset, compiled by Storlazzi et al. (2019), consists of measurements of the depth at cross-shore intervals of 2 m, starting 20 m above mean water level and extending to 30 m below mean water level. Several geographic regions are captured in the dataset: Hawaii, Florida, Guam, Puerto Rico, United States Virgin Islands, American Samoa, and the Northern Mariana Islands. Fringing reefs, barrier reefs, and atoll reefs are accounted for in the dataset.
Following Costa et al. (2016), geometrically distinct representative reef profiles were created by clustering with the k-means algorithm based on the similarity between all cross-shore depth locations. First, profiles were divided into length bins to prevent the focusing of cluster centers (here the mean reef profile of all profiles that belong to a cluster or group) on longer profiles. Length bin borders were derived from visual inspection of initial clustering on the full dataset: [0–600; 600–1,500; 1,500–2,600; 2,600–4,000; 4,000–17,000 m]. For each length bin, 10–15 cluster centers were obtained to find a good balance between bathymetric variability and compactness of the results. The number of clusters is chosen using the Davies–Bouldin evaluation index (Davies and Bouldin, 1979), which indicates how well the reef profiles fit to the cluster and not to other clusters. In total, 61 cluster centers, hence 61 clustered profile groups, were obtained with the k-means algorithm. However, as cluster centers are the mean of all profiles in a clustered profile group and hence not necessarily actual reef profiles, representative, observed reef profiles from the dataset were extracted for each clustered profile group. For each profile group, five representative reef profiles were extracted from the dataset to capture the bathymetric profile variability within each clustered group as well. All representative profiles, 305 in total, were then visually categorized based on their reef shape, from which 10 shapes were discerned (see Supplementary Figure 1), with an approximated frequency of occurrence as noted in Supplementary Table 1.
Four profiles with distinct hydrodynamic behavior and relatively high frequency of occurrence were extracted for subsequent modeling steps (Figure 1), representing approximately 70% of the analyzed data. The four types are categorized as a “fringing” reef (18% occurrence), a “convex” reef (in which the transition between the fore reef and reef flat is gradual, 11% occurrence), a “linear” reef (31% occurrence), and a “three-slope” reef (a steep nearshore slope, followed by a shelf, and an offshore fore reef slope, 10% occurrence). The barrier reef (13% occurrence) was omitted from the analysis for two reasons. First, wind-wave growth dominates the wave field inshore of barrier reefs and thus nearshore waves that cause flooding are relatively independent of barrier reef height (Gallop et al., 2014; Drost et al., 2019) and thus coral reef restorations far from the shore (more than a kilometer) on the barrier reef will have a minimal impact on coastal hazard risk reduction. Furthermore, the 1D modeling approach used here is not valid for wide (e.g., barrier) reef systems where wave refraction and horizontal circulation patterns are important for waves and wave-driven water levels (Lowe et al., 2010), with outflow through the channels in the barrier reef generally offsetting wave-driven set-up at the reef crest.
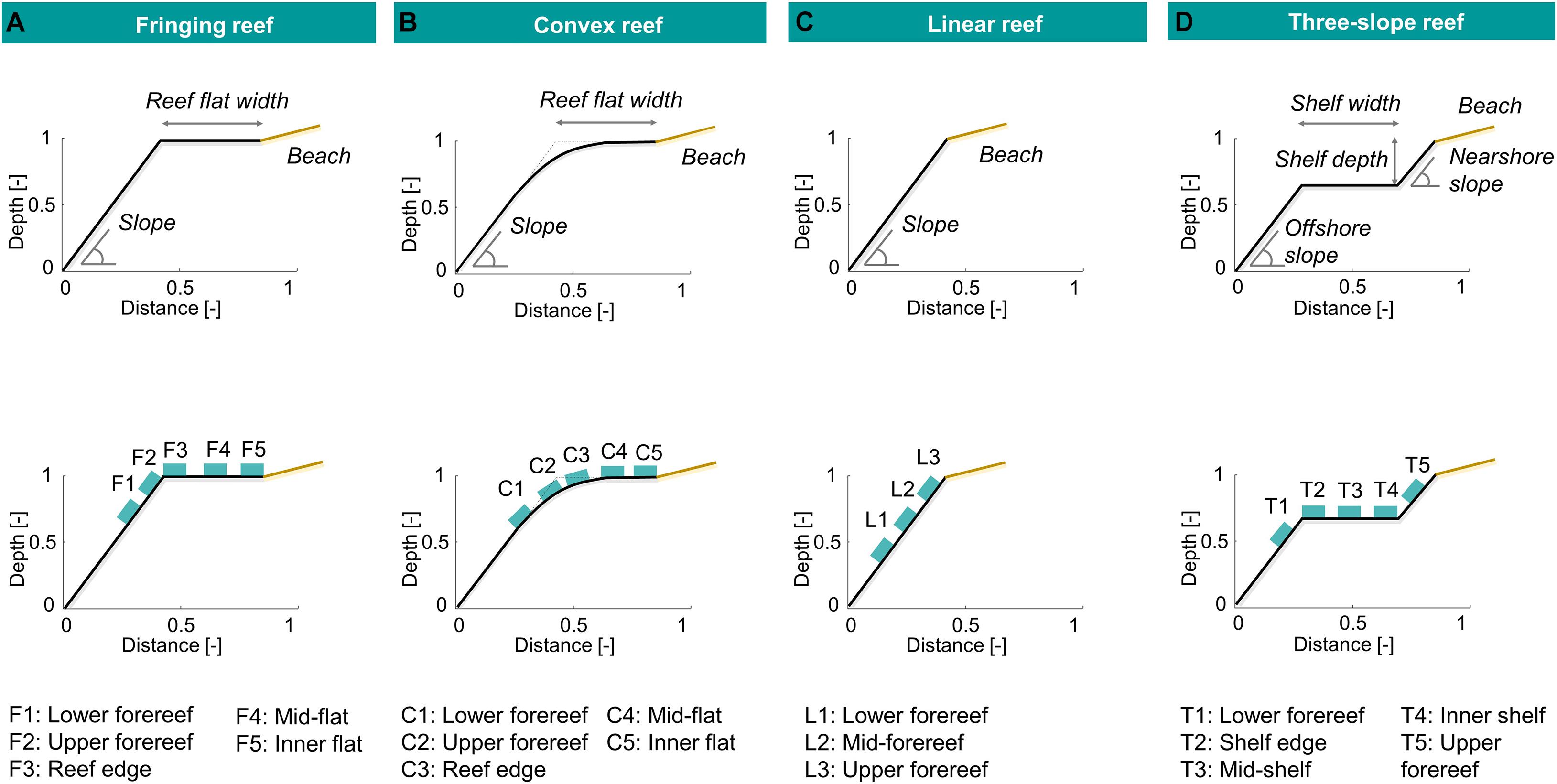
Figure 1. Overview of the four characteristic reef profile types and restoration locations. (A) Fringing, (B) convex, (C) linear, and (D) three-slope reef profile types.
For each profile shape, different profile parameter values (reef flat/shelf width and slope) were used in the modeling study to capture some of the profile variability encountered in nature: fringing reef: reef flat width = (100 and 250 m), fore reef slope = (0.1 and 0.5), convex reef: reef flat width = (100 and 250 m), fore reef slope = 0.1, linear reef: fore reef slope = (0.025, 0.1, and 0.5), three-slope reef: shelf width = (100 and 250 m), and offshore and nearshore slope = (0.1 and 0.5).
Restoration Schematization
Restorations were schematized according to Storlazzi et al.(2021, in press), who worked with stakeholders, scientists, and decision-makers to develop generalized restoration scenarios that considered (i) likelihood of delivering flood reduction benefits, (ii) existing coral restoration practices, and (iii) permitting factors such as depth for potential navigational hazards. Restorations can be either purely “green,” entailing solely outplanting corals, or “gray-green hybrid,” entailing emplacement of structures (such as ReefBalls) and then outplanting corals on top of them (Shaver and Silliman, 2017; Bostrom-Einarsson et al., 2020).
Here, restorations with dimensions of 5–25 m width and 0.25–1.25 m height were placed at 18 locations (F1–5, C1–5, L1–3, and T1–5 for the fringing reef, convex reef, linear reef, and three-slope reefs, respectively) across the four reef profile shapes (Figure 1). For each reef profile type, the different restoration configurations were designed based on restrictions of coral restoration works (restoration depth, width, and roughness). Restoration depth boundaries of 2 m (lower limit) and 7 m (upper limit) were set by operational constraints: deep enough to not interfere with small vessel traffic, and shallow enough to make outplanting by divers feasible. The effects of reef restorations were investigated for three different restoration widths: 5, 10, and 25 m, based on proposed reef restoration efforts by federal agencies and non-governmental organizations; these widths were constrained by projected costs of restoration measures per unit length of shoreline. Restorations were modeled as an increase in bed level: 0.25 m for “green” restoration representing outplanting 0.25-m high corals from a nursery and 1.25 m for “gray-green” hybrid restoration representing outplanting corals from a nursery on top of a 1-m high artificial structure (such as a ReefBall) across the width of the restoration, with enhanced hydrodynamic roughness due to the presence of the outplanted corals. Friction values were based on a meta-analysis of reef wave breaking studies by Storlazzi et al. (2019), who proposed a friction value (cf) of 0.15 for 90–100% coral cover and 0.01 for no coral cover. These values were adopted for the restored section and the unrestored reef bathymetry, respectively.
Wave-Driven Flood Modeling
XBeach (XB) is a physics-based nearshore model that solves the horizontal equations for flow, wave propagation, sediment transport, and changes in bathymetry (Roelvink et al., 2009). The non-hydrostatic version of XBeach (XB-NH, De Ridder et al., 2021), which solves wave evolution of both short and infragravity waves allows for an accurate estimation of all coastal runup components (Lashley et al., 2018). In particular, the XB-NH+ version was used, a reduced two-layer model which improves the dispersive behavior of the model compared to XB-NH. The XB-NH model was extensively validated for sandy coastlines (Roelvink et al., 2018), vegetated coasts (Van Rooijen et al., 2015), and most importantly, coral reef environments (Quataert et al., 2015, 2020; Lashley et al., 2018; Storlazzi et al., 2018).
Here, one-dimensional XB-NH+ models were set up based on the schematized reef bathymetric profiles; the grid resolution varies with depth, with the grid being coarser at greater depths and farther offshore than in the nearshore. A semi-infinite beach extended up to +30 m above mean sea level so that runup could be directly evaluated across the entire range of hydrodynamic forcing. The offshore boundary location was set by depth restrictions of n < 0.75 (the ratio of wave group speed over phase speed, which influences the generation of LF waves at the boundary), kh (relative depth, with k the wavenumber, and h depth), and for the wave height to depth ratio to prevent the breaking of waves at the boundary. XB-NH+ parameter values were obtained from a calibration study using field observations on coral-reef lined coasts (Quataert et al., 2020). Although Quataert et al. (2020) calibrated the XBeach model by varying the reef roughness, here the reef roughness is parametrized following Storlazzi et al. (2019), using friction values (cf) of 0.15 for the reef restoration, 0.01 for the (degraded) unrestored reef bathymetry, and 0.001 for the sandy beach.
The XB-NH+ models were forced with a range of water levels and wave conditions commonly observed in nature (Kolijn, 2014; Quataert et al., 2015; Shope et al., 2016). Kolijn (2014) provided a variation in significant wave heights of measured storm events from 1.0 to 4.5 m, whereas Quataert et al. (2015) and Cheriton et al. (2016) measured maximum wave heights of 6 m. Hence, wave heights of 2, 4, and 6 m were investigated in this study. These wave heights were combined with wave steepness values of 0.01 (typical for a swell event) and 0.05 (typical for a storm event). Kolijn (2014) also found that reef water levels of 68 investigated reef sites mostly range between 0 and +2.5 m relative to the reef flat, with tidal excursions of 0.5–1.0 m. Hence, water levels of 0.5, 1.0, 2.0, 3.0, and 4.0 m were imposed, where those of 0.5 and 1.0 m were excluded for simulations of reef flat restoration across the fringing and convex reef to prevent drying of the restoration. The corresponding JONSWAP spectra were imposed at the offshore boundary. Following Lashley et al. (2018), at cross-shore locations indicated in Figures 2–5, wave height components were discerned based on spectral analysis of local water level time series, using a split frequency of 0.5∗fp (peak frequency of incident SS waves) to distinguish the SS waves (frequency > 0.5∗fp) from the LF waves (frequency < 0.5∗fp).
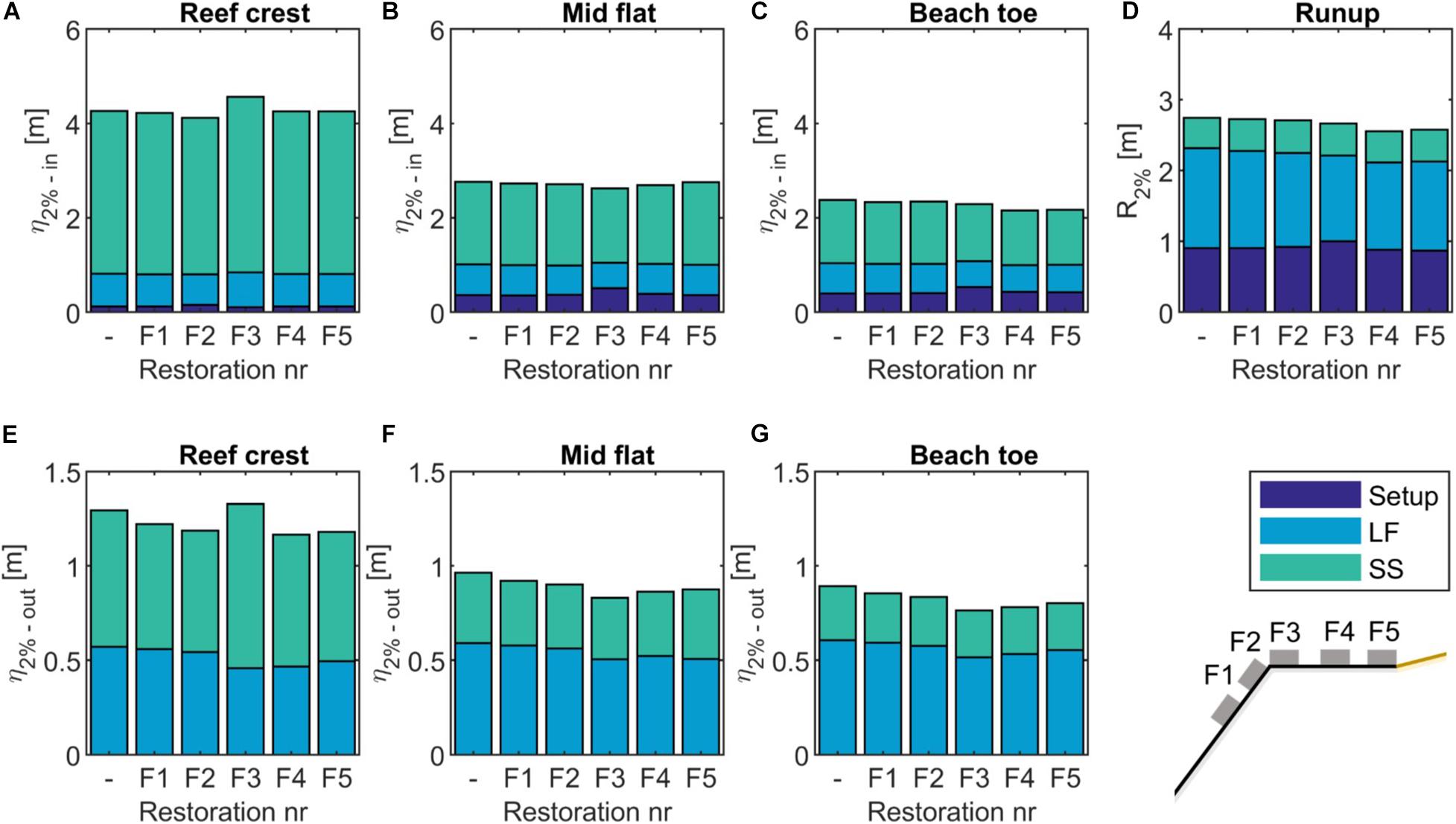
Figure 2. The influence of different restoration configurations on water levels across a fringing reef profile. The mean short (SS) wave, long (LF) wave, and setup water level extremes at three locations across the reef: (A) Reef crest, (B) mid reef flat, and (C) beach toe for profiles with no restoration [Restoration nr (-)] and restored profiles (Restoration nr = F1 to F5, corresponding to Figure 1), averaged across model variations. (D) Mean runup heights. Water levels are separated into incoming (A–C) and reflected (E–G) water levels.
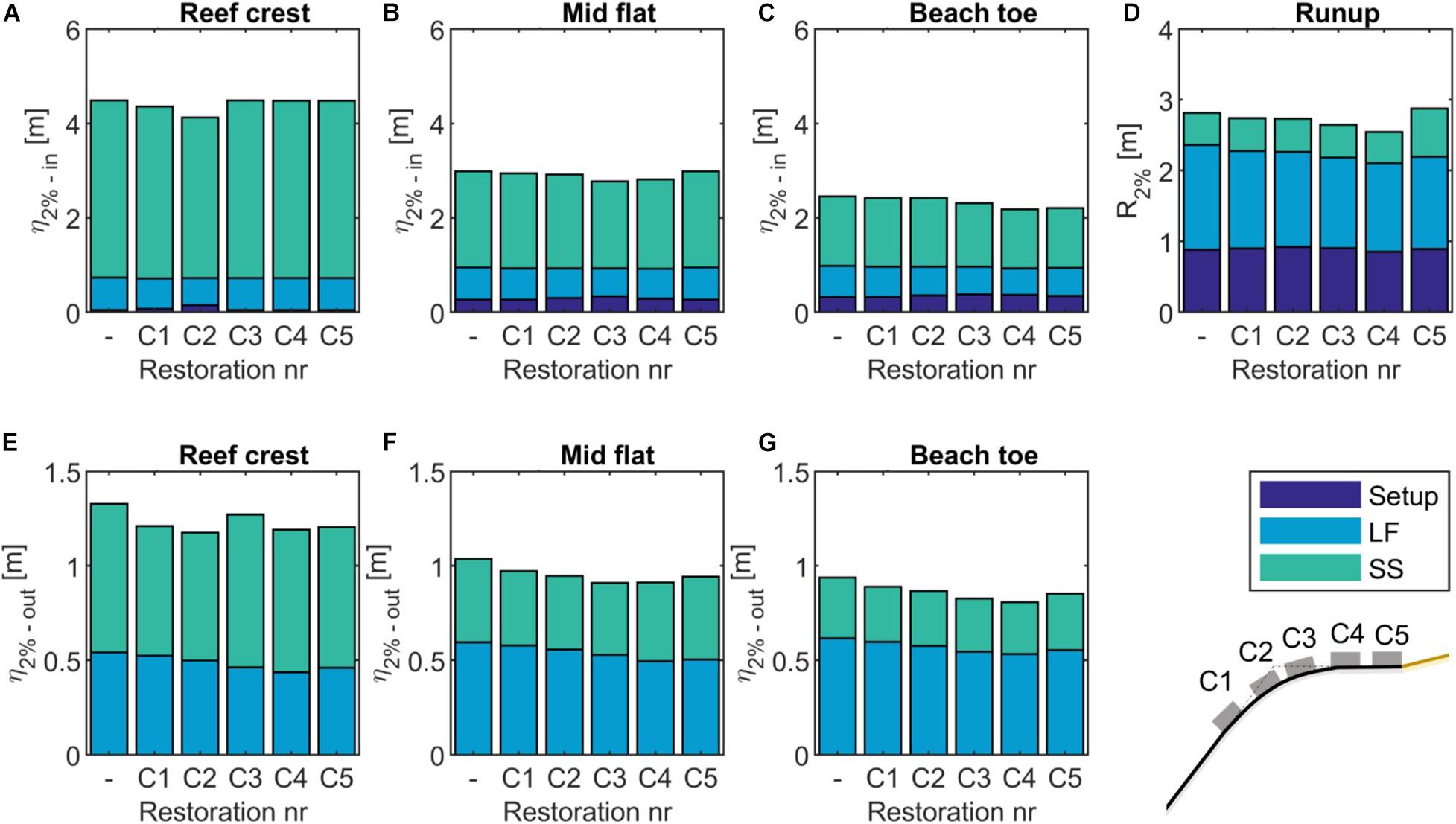
Figure 3. The influence of different restoration configurations on water levels across a convex reef profile. The mean short (SS) wave, long (LF) wave, and setup water level extremes at three locations across the reef: (A) Reef crest, (B) mid reef flat, and (C) beach toe for profiles with no restoration [Restoration nr (-)] and restored profiles (Restoration nr = C1 to C5, corresponding to Figure 1), averaged across model variations. (D) Mean runup heights. Water levels are separated into incoming (A–C) and reflected (E–G) water levels.
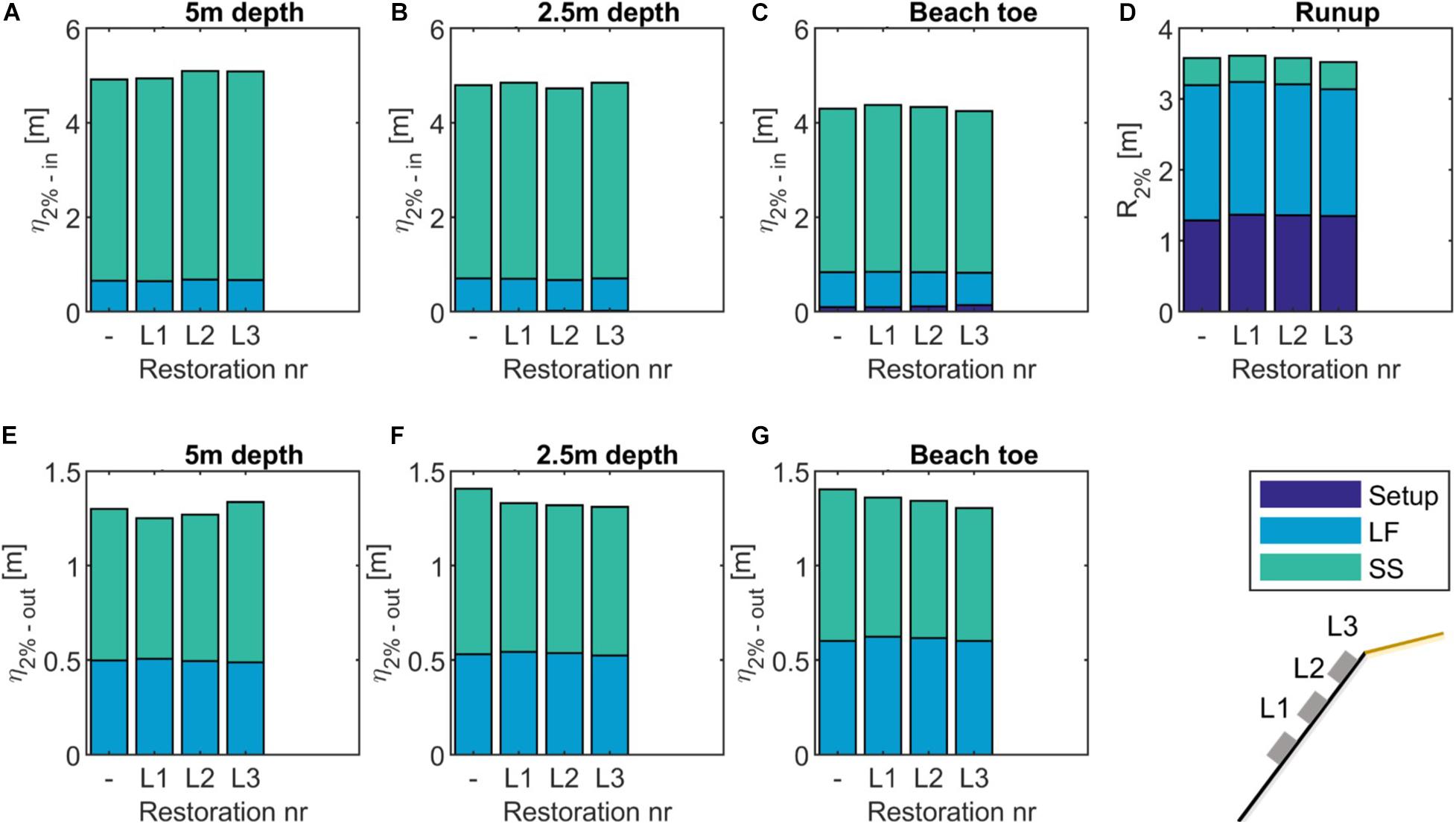
Figure 4. The influence of different restoration configurations on water levels across a linear reef profile. The mean short (SS) wave, long (LF) wave, and setup water level extremes at three locations across the reef: (A) Reef crest, (B) mid reef flat, and (C) beach toe for profiles with no restoration [Restoration nr (-)] and restored profiles (Restoration nr = L1 to L3, corresponding to Figure 1) averaged across model variations. (D) Mean runup heights. Water levels are separated into incoming (A–C) and reflected (E–G) water levels.
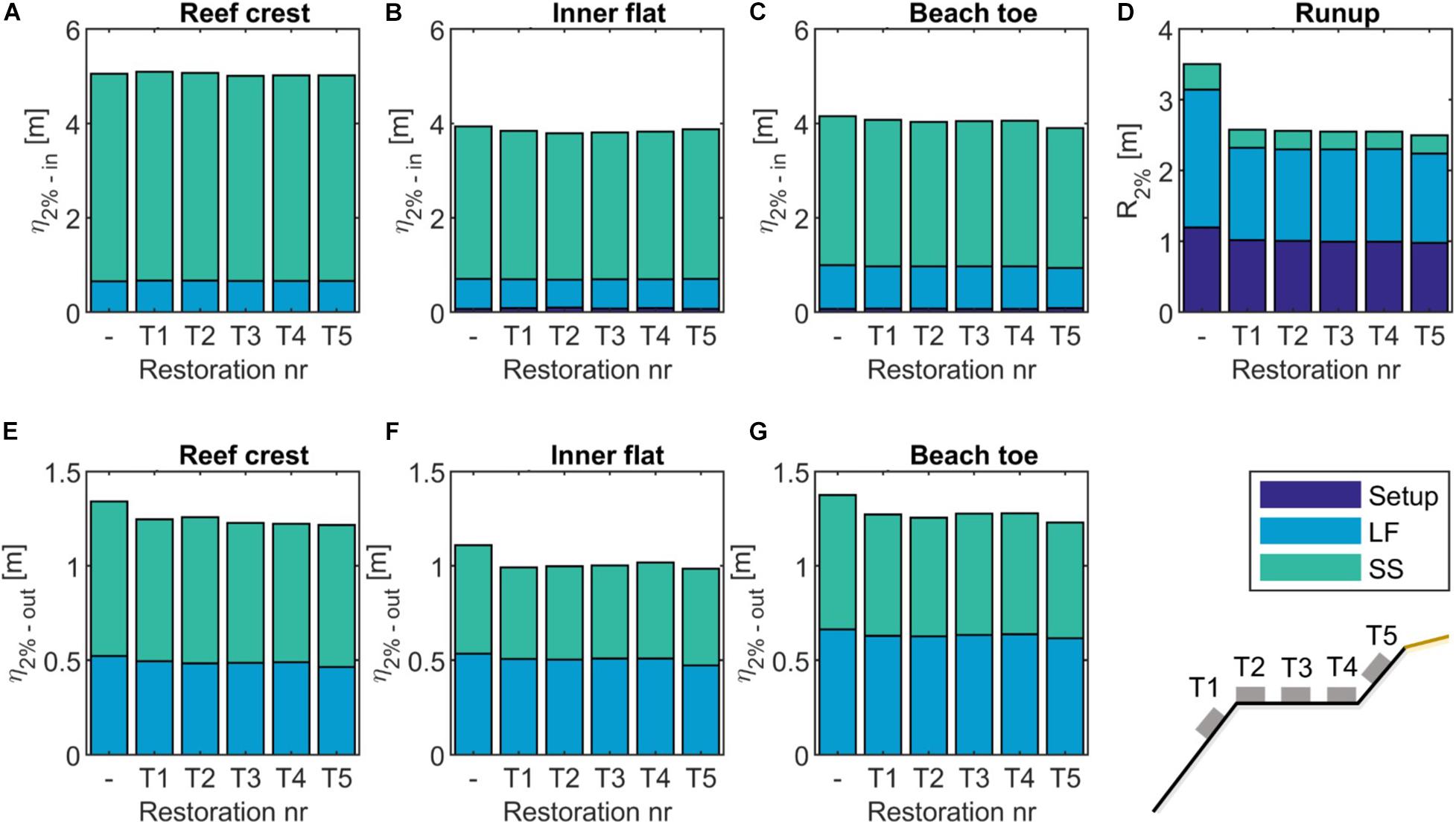
Figure 5. The influence of different restoration configurations on water levels across a three-slope reef profile. The mean short (SS) wave, long (LF) wave, and setup water level extremes at three locations across the reef: (A) Reef crest, (B) mid reef flat, and (C) beach toe for profiles with no restoration [Restoration nr (-)] and restored profiles (Restoration nr = T1 to T5, corresponding to Figure 1), averaged across model variations. (D) Mean runup heights. Water levels are separated into incoming (A–C) and reflected (E–G) water levels.
Water level time series were separated into incoming and outgoing components via the method of Guza et al. (1984) from local water level elevations and current velocities. Runup, defined as the elevation water reaches up the beach slope with a 2% exceedance value, was extracted from the runup water level time series, by solving for the SS wave, LF wave, and setup components of the runup. The steady setup component was obtained by extracting the mean water level relative to the still water level. The SS wave and LF wave runup components were obtained from the detrended water level time series by spectral composition. The total water level results were sorted in ascending order to select the 2% exceedance value.
Results
To identify promising coral reef restoration strategies for protecting the adjacent shoreline, runup reduction by coral reef restorations was investigated. Wave transformation across unrestored and restored representative reef profiles was investigated to identify processes governing the runup at the shoreline.
Wave Transformation Across Unrestored Coral Reef Profiles
To isolate the hydrodynamic effects of a restoration, we first evaluated wave transformation across unrestored coral reef profiles with the one-dimensional XBeach models. Results (not shown here) confirm general patterns found in previous studies (e.g., Cheriton et al., 2016; Pearson et al., 2017; Buckley et al., 2018). A narrow surf zone (steep slope) promotes the quick dissipation of sea-swell (“SS,” 5–25 s periods) waves and the generation of wave-induced setup and breakpoint-forced low-frequency (“LF,” 25–1,000 s periods) motions, resulting in increased wave runup and thus coastal flooding potential on steeper-sloped coasts. Increased water depth, narrower reef width and/or lower roughness reduce frictional dissipation across the reef (Quataert et al., 2015). Large reflection values at the beach, combined with greater water depths and lower roughness, promotes the amplification of LF wave heights by resonance and thus increases coastal flood risk (Cheriton et al., 2016).
Wave Transformation Across Restored Coral Reef Profiles
Coral reef restorations are expected to affect the wave transformation process and the subsequent wave-driven runup and potential coastal flooding by modifying the bathymetry and seabed roughness. Various restored reef profiles were tested for a range of hydrodynamic forcing conditions in order to account for the many non-linear interactions between reef morphology and hydrodynamics (Quataert et al., 2015).
Wave transformation is characterized by the water levels across the reef η2% and the runup R2%, defined as the upper 2% of the water level time series at a specified location (e.g., Merrifield et al., 2014; Pearson et al., 2017; Figures 2–5). Wave reflection and dissipation across a coral restoration are the main processes affecting wave transformation relative to unrestored profiles, as observed across all four profile types. The seaward reflection of both SS and LF waves reduces wave energy reaching the shore. SS wave reflection is clearly identifiable at restoration location F3 of the fringing reef, where just offshore of the reef crest restoration (Figure 2E), η2% –SS–out is significantly larger than at the unrestored profile (compare with first bar). The reduction in η2% at the beach toe (Figures 2–5C,G), relative to the unrestored profiles, indicates higher dissipation of both SS and LF waves by wave breaking and bottom friction across the restoration due to the decreased water depths above the restoration and enhanced roughness. However, the reduction in SS wave heights also leads to an increase in setup across the restoration. Especially for locations near the current (pre-restoration) breakpoint (i.e., location F3 of the fringing reef, location C2 of the convex reef), radiation stress gradients due to wave dissipation significantly increase, leading to an increase in setup (Figures 2–5B–D). Interestingly, although setup and LF wave height variations clearly translate into a change in their runup components (Figures 2–5C,D), the SS wave runup is hardly affected by the SS-wave damping. It is hypothesized that the depth-limitation on the SS-wave height causes its runup to be relatively constant, whereas the reduced breakpoint forcing and diminished energy transfer to LF waves by short wave damping induces a significant reduction in the setup and LF wave component of the runup. The SS wave runup is only reduced for cases with a strong reduction in setup (and hence a decrease of the nearshore water depth; Figure 2–5D).
Runup Reduction by Coral Reef Restorations
From the R2% reduction values across different restoration locations for the four reef types, the following two main observations can be made regarding the efficiency of restorations (Figure 6). First, runup and thus flooding reduction varies significantly between reef profile types and is dependent on the location and dimension of the restoration on the profile. The coastlines fronted by three-slope profiles are relatively unprotected from wave action in unrestored conditions, as the observed runup is much higher than at fringing and convex reef profiles. This renders these types of reef profiles vulnerable to coastal flooding but also responsive to restoration measures. For the three-slope profiles, mean reductions in runup of 23 to 30% are achieved, which increase as restorations are placed closer to shore. Across linear reef profiles, runup reduction increases significantly for shallower restoration depths, where runup reductions of up to 10% can be achieved, with absolute values significantly higher than across fringing and convex reefs. For restorations across the three-slope and linear reef profiles, restoration efficiency (reduced flooding potential) increases as dimensions (restoration height and width) increase, due to the enhanced dissipation and reflection across the restoration, which leads to a reduction in setup and LF component of the runup. Mean runup reductions were similar between fringing and convex reef profiles and in the range of −1 to 13%, with the restoration efficiency being strongly controlled by the location and dimension of the restoration. Restorations located near the current (pre-restoration) breakpoint (e.g., locations F3 of the fringing reef profile and location C2 of the convex reef profile) enhance radiation stress gradients and can therefore increase the setup, making them relatively ineffective as coastal protection measures. The amplification of the setup by restoring the reef is aggravated for larger restoration heights. Restorations on the lower fore reef (F1 and F2 of the fringing profile and C1 of the convex reef) can reduce flood risk, although runup reduction is low and can also be negative (relative to the unrestored state), as they are located in relatively deep water with little effect on the wave dynamics. Runup reduction efficiency is highest at the mid-flat, showing mean values of seven and 10% for the fringing and convex profile, respectively. Ideally, the reef flat restoration should be located in between the mid-flat restoration and the inner flat restoration, where wave heights have already been naturally dissipated across the reef, thereby minimizing the additional setup across the restoration. However, the restoration should not be located too close to the inner surf zone where radiation stress gradients are again increased by the restoration and reflection can cause unwanted effects.
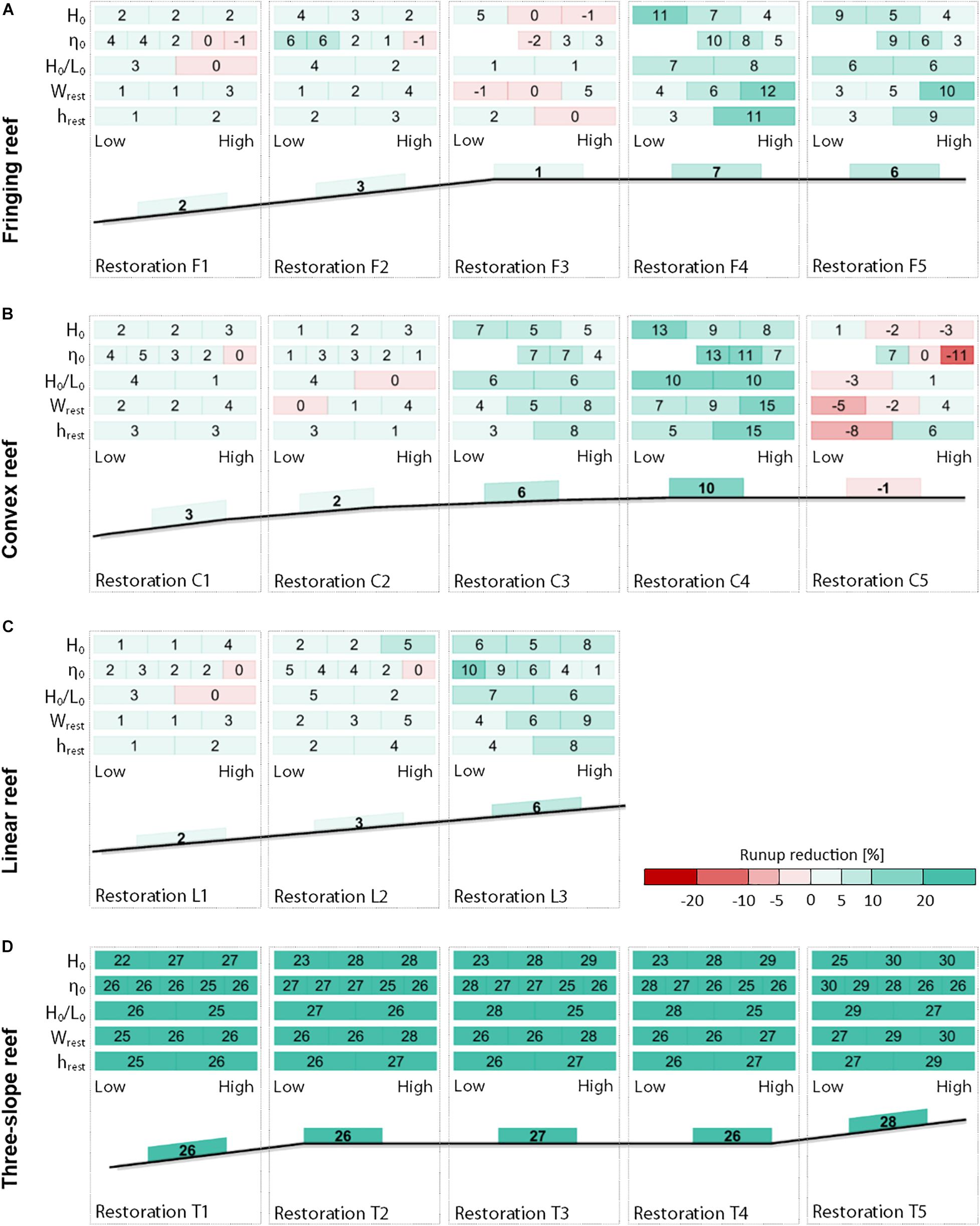
Figure 6. Runup reduction potential for restorations on the four characteristic reef profile types. Mean runup (R2%) reduction values [%] across different restoration locations (1–5) for the (A) fringing reef, (B) convex reef, (C) linear reef, and (D) three-slope reef. For each reef type, the mean runup reduction is calculated for filtered input parameters (varying offshore wave heights H0, water levels η0, wave steepness values H0/L0, restoration widths Wrest and restoration heights hrest), in which runup is averaged across all other model variations. “Low” and “High” refer to the minimum and maximum input values for the parameter. The total mean runup reduction is depicted for each restoration location as the value denoted in the illustrated reef restoration blocks.
Secondly, the runup reduction efficiency highly depends on oceanographic forcing conditions (i.e., the offshore water level, wave height, and wave period). The analysis of runup reduction indicates a strong correlation between the LF and setup component of the runup and these extrinsic parameters, whereas the SS wave runup component is relatively invariable due to the depth-limitation on the SS-wave height. Both the LF wave runup reduction and setup increase across the restoration, as they are proportional to the incident wave height and wave period and are inversely proportional to the depth on the reef. Although enhanced radiation stress gradients (large wave heights, low water depths) across the restoration locally enhance setup, enhanced wave dissipation actually leads to diminished setup near the shore. Across linear and three-slope profiles, this effect translates into a runup reduction that increases for shallower water depths, larger wave heights, and longer wave periods, as a result of nearshore setup and LF runup reduction. For the fringing and convex reef, trends in LF runup reduction are clear, but the total runup reduction is difficult to predict due to the large influence of the setup across these profiles, which is highly sensitive to varying hydrodynamic conditions.
Discussion
Coral restorations are often performed with the purpose of enhancing reef ecology, often with marginal consideration for, and research investments in, their coastal protection value. We demonstrate the positive impact of coral restorations on adjacent coastal flood reduction potential, showing a promising example of a nature-based solution for vulnerable coral reef-lined coasts. Characteristic reef profiles, deduced via a statistical analysis, were classified as the fringing reef, the convex reef, the linear reef and the three-slope reef, and were subjected to restorations at different across-shore locations along the profile. Model results indicate that the wave reflection and dissipation across coral restorations can decrease potential coastal flooding up to 30%, the exact reduction efficiency being highly dependent on (1) the reef profile shape, (2) the location of the restoration on the profile, (3) dimensions of the restoration, and (4) hydrodynamic forcing conditions. Fringing and convex reefs feature a reef flat that already acts as a natural wave attenuator, limiting the additional flood risk reduction effect that could be provided by coral restoration. Still, runup reductions of up to 10% can be achieved for shallow restorations located on the reef flat at some distance from the beach toe. For both reef types, the reduction of wave-driven flooding is greater for shallower restorations on the upper fore reef or middle reef flat than for deeper locations on the fore reef. The reef crest restoration of the fringing reef (F3), as well as the inner reef restoration of the convex reef (C1) can actually increase wave-driven flooding as a result of increased mean water levels (C3 or F3) and shoreward reflection of waves at the restoration (C1 or F1). The linear and three-slope profiles leave the coastline relatively unprotected from wave action, rendering them naturally more vulnerable to coastal flooding, but also more receptive to coral reef restoration measures. Average runup reductions of 26–30% are observed for the three-slope profile, and up to 10% for the linear profile, with greatest reduction for shallow restorations near the shore.
This study presents a first attempt at describing and quantifying the beneficial added value of coral reef restorations for coastal hazard risk reduction. However, this approach is not without limitations. First, coral reefs were modeled as highly schematized profiles, where impermeable locally raised bed levels with enhanced roughness imitate reef restorations. In nature, coral transplantations and artificial reef restorations are not impermeable, allowing canopy flow that likely reduces the wave-setup over and reflection at the restoration compared to the impermeable bed case modeled here. Whether simulated hydrodynamic patterns are consistent with observations in nature should be verified with field or laboratory experiments, which are currently not available. Second, one-dimensional reef models neglect two-dimensional effects such as horizontal circulation cells and longshore currents that may balance wave-induced set-up with offshore flow out of channels in the barrier reef (e.g., Lowe et al., 2010). In addition, structure-induced circulation patterns as commonly observed for submerged structures (Villani et al., 2012) are neglected in the 1D approach. Third, the assumption of normal wave incidence is likely to cause an overestimation of the LF-wave height and LF runup component due to the enhanced interactions between individual waves in a 1D approach (e.g., Herbers et al., 1994). However, this limitation is minor as focus is foremost on the relative comparison of flood risk rather than an absolute representation of flood risk. Fourth, estimates of runup on reef-fronted coastlines have scarcely been validated due to the global lack of runup recordings (e.g., Winter et al., 2020). Despite these limitations, model simulations of previously validated XB models (Quataert et al., 2015, 2020) suggest models presented in the present paper give a reasonable estimate of the relative impact of coral reef restoration measures on coastal flood risk.
It is apparent from the results presented here that most of the optimal locations on reefs for restorations to reduce coastal flooding are in shallow, energetic areas such as the upper fore reef and middle reef flat that are typically characterized by physically-robust coral species (e.g., Montaggioni and Braithwaite, 2009). Hence, to increase the coastal hazard risk reduction potential of coral reef restoration, physically robust species of coral need to be grown in nurseries and outplanted to shallow, energetic locations. This, however, contrasts the current practice of more fragile, faster-growing species primarily being grown in nurseries (Levy et al., 2010; Lohr et al., 2015) and outplanted in the field (Bostrom-Einarsson et al., 2020, and references therein). Further, ocean warming and acidification (Pandolfi et al., 2011) as a result of climate change will likely adversely impact coral growth on the reef flat, necessitating outplanting strategies with climate-resilient corals.
An uncertain future under climate change, with a possible increase in storm intensity or frequency, and rising sea levels, mandates the need for improved coastal resilience to hazards through effective adaptation measures. The results of this study suggest that, under storm conditions, the flood reduction potential provided by coral restorations across linear and three-slope profile reefs would likely increase due to the enhanced dissipation across restored reefs. In contrast, the flood reduction potential may decrease across fringing and convex reefs under similar conditions, reducing the overall efficiency of the restoration, although a small reduction in runup may still be achieved. Additionally, rising sea levels could make coral development more viable on the reef flat (Scopélitis et al., 2011), while deeper restorations on the fore reef may become less effective. At present, relatively few corals are typically found on shallow reef flats (relative to fore reef slopes) due to wave energy, thermal tolerances, and occasional exposure to open air during spring low tides. Rising sea levels may actually reduce the impact of these factors on coral growth patterns, which may be especially positive for fringing and convex reef restorations, as they would improve energy dissipation by increasing the hydrodynamic roughness of the reef flat.
Hazard mitigation strategies can help finance reef restoration in different ways. First, post-disaster recovery funding could support restoring coral reefs for coastal defense infrastructure (Beck and Lange, 2016). Second, because coral reefs protect coastal communities, reef restoration could be funded through such mechanisms as pre-disaster hazard mitigation funds (Beck and Lange, 2016). Third, the insurance industry can support incentives for habitat restoration by insuring their coastal protection service (Reguero et al., 2019) or through new resilience insurance mechanisms for coral reef restoration projects (Reguero et al., 2020). By allocating ever-limited funds to well-designed coral restorations, vulnerable coastal areas may receive the much-needed support to restore their adjacent reefs to support tourism, fisheries, and recreation via such mechanisms as pre-disaster mitigation, post-disaster restoration funding, and/or insurance. Through the improved understanding of their optimal runup reduction efficiency, coral reef restorations may become a physically and economically viable option for mitigating hazards under a changing climate with benefits for both coral ecosystems and human coastal populations.
Data Availability Statement
The XB-NH+ model input files are available as a NetCDF (*.nc) file at the following location: 10.5066/P991RSFO.
Author Contributions
FR, CS, and AD designed research and methodological approach. FR performed the modeling. All authors analyzed the results, performed the visualizations, and co-wrote the manuscript.
Funding
This work was supported by the U.S. Department of Interior, U.S. Geological Survey through the Coastal and Marine Hazards and Resources Program’s Coral Reef Project under Grant/Cooperative Agreement No. G20AC00009, and the Deltares Quantifying Flood Hazards and Impacts (11205283) and Natural Hazards (11206875) Strategic Research Programs. Any use of trade, firm, or product names is for descriptive purposes only and does not imply endorsement by the U.S. Government.
Conflict of Interest
The authors declare that the research was conducted in the absence of any commercial or financial relationships that could be construed as a potential conflict of interest.
Acknowledgments
We would like to thank Ben Norris (USGS) for his important insight and useful comments during the preparation of this article. We would also like to thank the two reviewers for their constructive comments, which improved this manuscript.
Supplementary Material
The Supplementary Material for this article can be found online at: https://www.frontiersin.org/articles/10.3389/fmars.2021.653945/full#supplementary-material
References
Beck, M. W., and Lange, G.-M. (eds) (2016). Wealth Accounting and the Valuation of Ecosystem Services Partnership (WAVES). Managing coasts with natural solutions: guidelines for measuring and valuing the coastal protection services of mangroves and coral reefs (English). Waves technical paper. Washington, D.C.: World Bank Group.
Bostrom-Einarsson, L., Babcock, R. C., Bayraktarov, E., Ceccarelli, D., Cook, N., Ferse, S. C. A., et al. (2020). PLoS One 15:e0226631. doi: 10.1371/journal.pone.0226631
Bruno, J. F., and Selig, E. R. (2007). Regional decline of coral cover in the IndoPacific: Timing, extent, and subregional comparisons. PLoS One 2:19326203. doi: 10.1371/journal.pone.0000711
Buckley, M. L., Lowe, R. J., Hansen, J. E., van Dongeren, A. R., and Storlazzi, C. D. (2018). Mechanisms of wave−driven water level variability on reef−fringed coastlines. J. Geophys. Res. 123, 3811–3831. doi: 10.1029/2018JC013933
Carson, R. R., Foo, S. A., and Asner, G. P. (2019). Land-use impacts on coral reef health: A ridge-to-reef perspective. Front. Mar. Sci. 2019:00562. doi: 10.3389/fmars.2019.00562
Chan, W. Y., Peplow, L. M., Menendez, P., Hoffmann, A. A., and Van Oppen, M. J. H. (2018). Interspecific hybridization may provide novel opportunities for coral reef restoration. Front. Mar. Sci. 5, 1–15. doi: 10.3389/fmars2018.00160
Cheriton, O. M., Storlazzi, C. D., and Rosenberger, K. J. (2016). Observations of wave transformation over a fringing coral reef and the importance of low−frequency waves and offshore water levels to runup, overwash, and coastal flooding. J. Geophys. Res. 121, 3121–3140. doi: 10.1002/2015JC011231
Costa, M. B., Araújo, M., Araújo, T. C., and Siegle, E. (2016). Influence of reef geometry on wave attenuation on a Brazilian coral reef. Geomorphology 253, 318–327. doi: 10.1016/j.geomorph.2015.11.001
Davies, D. L., and Bouldin, D. W. (1979). A cluster separation measure. IEEE Transac. Pattern Anal. Mach. Intellig. 2, 224–227. doi: 10.1109/TPAMI.1979.4766909
De Ridder, M. P., Smit, P. B., van Dongeren, A. R., McCall, R. T., Nederhoff, K., and Reniers, A. J. (2021). Efficient two-layer non-hydrostatic wave model with accurate dispersive behaviour. Coastal Eng. 164:103808. doi: 10.1016/j.coastaleng.2020.103808
Drost, E. J., Cuttler, M. V., Lowe, R. J., and Hansen, J. E. (2019). Predicting the hydrodynamic response of a coastal reef-lagoon system to a tropical cyclone using phase-averaged and surfbeat-resolving wave models. Coastal Eng. 152:103525. doi: 10.1016/j.coastaleng.2019.103525
Elliff, C. I., and Silva, I. R. (2017). Coral reefs as the first line of defense: Shoreline protection in face of climate change. Mar. Env. Res. 127, 148–154. doi: 10.1016/j.marenvres.2017.03.007
Ferrario, F., Beck, M. W., Storlazzi, C. D., Micheli, F., Shepard, C. C., and Airoldi, L. (2014). The effectiveness of coral reefs for coastal hazard risk reduction and adaptation. Nat. Commun. 5, 1–9. doi: 10.1038/ncomms4794
Gallop, S. L., Young, I. R., and Ranasinghe, R. (2014). The large-scale influence of the Great Barrier Reef matrix on wave attenuation. Coral Reefs 33, 1167–1178. doi: 10.1007/s00338-014-1205-7
Guza, R. T., Thornton, E. B., and Holman, R. A. (1984). Swash on steep and shallow beaches. Proc. Coastal Eng. Conference 1, 708–723. doi: 10.1061/9780872624382.049
Herbers, T. H. C., Elgar, S., and Guza, R. T. (1994). Infragravity-frequency (0.005–0.05 Hz) motions on the shelf. Part I: forced waves. J. Phys. Oceanogr. 24, 917–927.
Hoegh-Guldberg, O., Pendleton, L., and Kaup, A. (2019). People and the changing nature of coral reefs. Reg. Stud. Mar. 30:100699. doi: 10.1016/j.rsma.2019.100699
Kolijn, D. J. (2014). Effectiveness of a Multipurpose Artificial Underwater Structure as a Coral Reef Canopy: Hydrodynamic and Ecological Connectivity. M.Sc. thesis. Delft: Delft University of Technology, Faculty of Civil Engineering and Geosciences.
Lashley, L. H., Roelvink, J. A., Van Dongeren, A. R., Buckley, M. L., and Lowe, R. J. (2018). Nonhydrostatic and surfbeat model predictions of extreme wave run-up in fringing reef environments. Coastal Eng. 137, 11–27. doi: 10.1016/j.coastaleng.2018.03.007
Levy, G., Shaish, L., Haim, A., and Rinkevich, B. (2010). Mid-water rope nursery—Testing design and performance of a novel reef restoration instrument. Ecol. Eng. 36, 560–569. doi: 10.1016/j.ecoleng.2009.12.003
Levy, J. S., Ripple, K. J., and Winters, R. S. (2018). Lessons learned for increased scalability for in situ coral restoration efforts. Coral Restorat. Found. White Papers 2018, 1–17.
Lirman, D., and Schopmeyer, S. (2016). Ecological solutions to reef degradation: optimizing coral reef restoration in the Caribbean and Western Atlantic. PeerJ. 4, 1–19. doi: 10.7717/peerj.2597
Lohr, K. E., Bejarano, S., Lirman, D., Schopmeyer, S., and Manfrino, C. (2015). Optimizing the productivity of a coral nursery focused on staghorn coral Acropora cervicornis. Endang. Species Res. 27, 243–250. doi: 10.3354/esr00667
Lowe, R. J., Hart, C., and Pattiaratchi, C. B. (2010). Morphological constraints to wave−driven circulation in coastal reef−lagoon systems: A numerical study. J. Geophys. Res. 115:5753. doi: 10.1029/2009JC005753
Merrifield, M. A., Becker, J. M., Ford, M., and Yao, Y. (2014). Observations and estimates of wave−driven water level extremes at the Marshall Islands. Geophys. Res. Lett. 41, 7245–7253. doi: 10.1002/2014GL061005
Montoya-Maya, P. H., Smit, K. P., April, J. B., and Frias-Torres, S. (2016). Large-scale coral reef restoration could assist natural recovery in Seychelles, Indian Ocean. Nat. Conserv. 16, 442–446. doi: 10.3897/natureconservation.16.8604
Montaggioni, L. F., and Braithwaite, C. J. R. (2009). Quaternary coral reef systems: history, development processes and controlling factors. Dev. Mar. Geol. 5,, 67–122.
Pandolfi, J. M., Connolly, S. R., Marshall, D. J., and Cohen, A. L. (2011). Projecting coral reef futures under global warming and ocean acidification. Science 333, 418–422. doi: 10.1126/science.1204794
Pearson, S. G., Storlazzi, C. D., Van Dongeren, A. R., Tissier, M. F. S., and Reniers, A. J. H. M. (2017). A Bayesian-Based System to Assess Wave-Driven Flooding Hazards on Coral Reef-Lined Coasts. J. Geophys. Res. 122, 10099–10117. doi: 10.1002/2017JC013204
Quataert, E., Storlazzi, C., Van Rooijen, A., Cheriton, O., and Van Dongeren, A. R. (2015). The influence of coral reefs and climate change on wave driven fooding of tropical coastlines. Geophys. Res. Lett. 42, 6407–6415. doi: 10.1002/2015GL064861
Quataert, E., Storlazzi, C., van Dongeren, A., and McCall, R. (2020). The importance of explicitly modelling sea-swell waves for runup on reef-lined coasts. Coastal Eng. 160:103704. doi: 10.1016/j.coastaleng.2020.103704
Reguero, B. G., Beck, M. W., Agostini, V. N., Kramer, P., and Hancock, B. (2018). Coral reefs for coastal protection: A new methodological approach and engineering case study in Grenada. J. Env. Manag. 210, 146–161. doi: 10.1016/j.jenvman.2018.01.024
Reguero, B. G., Secaira, F., Toimil, A., Escudero, M., Díaz-Simal, P., Beck, M. W., et al. (2019). The risk reduction benefits of the mesoamerican reef in Mexico. Front.Earth Sci. 7:125. doi: 10.3389/feart.2019.00125
Reguero, B. G., Beck, M. W., Schmid, D., Stadtmüller, D., Raepple, J., Schüssele, S., et al. (2020). Financing coastal resilience by combining nature-based risk reduction with insurance. Ecological Economics 169, 106487. doi: 10.1016/j.ecolecon.2019.106487
Rinkevich, B. (2014). Rebuilding coral reefs: Does active reef restoration lead to sustainable reefs? Curr. Opin. Env. Sustain. 7, 28–36. doi: 10.1016/j.cosust.2013.11.018
Roelvink, J. A., Reniers, A. J. H. M., Van Dongeren, A. R., Van Thiel de Vries, J., McCall, R. T., and Lescinski, J. (2009). Modelling storm impacts on beaches, dunes and barrier islands. Coastal Eng. 56, 1133–1152. doi: 10.1016/j.coastaleng.2009.08.006
Roelvink, D., McCall, R., Mehvar, S., Nederhoff, K., and Dastgheib, A. (2018). Improving predictions of swash dynamics in XBeach: The role of groupiness and incident-band runup. Coastal Engineering 134, 103–123. doi: 10.1016/j.coastaleng.2017.07.004
Scopélitis, J., Andréfouët, S., Phinn, S., Done, T., and Chabanet, P. (2011). Coral colonisation of a shallow reef flat in response to rising sea level: quantification from 35 years of remote sensing data at Heron Island, Australia. Coral Reefs 30:951. doi: 10.1007/s00338-011-0774-y
Shaver, E. C., and Silliman, B. R. (2017). Time to cash in on positive interactions for coral restoration. PeerJ 5:e3499. doi: 10.7717/peerj.3499
Shope, J. B., Storlazzi, C. D., Erikson, L. H., and Hegermiller, C. A. (2016). Changes to extreme wave climates of islands within the Western Tropical Pacific throughout the 21st century under RCP 4.5 and RCP 8.5, with implications for island vulnerability and sustainability. Glob. Planetar. Change 141, 25–38. doi: 10.1016/j.gloplacha.2016.03.009
Storlazzi, C. D., Gingerich, S. B., van Dongeren, A. R., Cheriton, O. M., Swarzenski, P. W., Quataert, E., et al. (2018). Most atolls will be uninhabitable by the mid-21st century because of sea-level rise exacerbating wave-driven fooding. Sci. Adv. 4, 1–10. doi: 10.1126/sciadv.aap9741
Storlazzi, C. D., Reguero, B. G., Cole, A. D., Lowe, E., Shope, J. B., Gibbs, A. E., et al. (2019). Rigorously valuing the role of U.S. coral reefs in coastal hazard risk reduction, U.S. Geolog. Survey Open-File Rep. 1027:42. doi: 10.3133/ofr20191027
Storlazzi, C. D., Reguero, B. G., Cumming, K. A., Cole, A. D., Shope, J. A., Gaido, C. L., et al. (2021). Rigorously valuing the coastal hazard risks reduction provided by potential coral reef restoration.
Taebi, S., and Pattiaratchi, C. (2014). Hydrodynamic response of a fringing coral reef to a rise in mean sea level. Ocean Dynam. 64, 975–987.
Van Dongeren, A. P., Lowe, R., Pomeroy, A., Trang, D. M., Roelvink, D., Symonds, G., et al. (2013). Numerical modeling of low-frequency wave dynamics over a fringing coral reef. Coastal Eng. 73, 178–190. doi: 10.1016/j.coastaleng.2012.11.004
Van Oppen, M. J. H., Oliver, J. K., Putnam, H. M., and Gates, R. D. (2015). Building coral reef resilience through assisted evolution. Proc. Natl. Acad. Sci. 112, 2307–2313. doi: 10.1073/pnas.1422301112
Van Rooijen, A. A., Van Thiel de Vries, J. S. M., McCall, R. T., Van Dongeren, A. R., Roelvink, J. A., et al. (2015). “Modeling of wave attenuation by vegetation with XBeach,” in E-proceedings of the 36th IAHR World Congress, (Netherlands: IAHR).
Vetter, O., Becker, J. M., Merrifield, M. A., Pequignet, A. C., Aucan, J., Boc, S. J., et al. (2010). Wave setup over a Pacific Island fringing reef. J. Geophys. Res. 115, 1–13. doi: 10.1029/2010JC006455
Villani, M., Bosboom, J., Zijlema, M., and Stive, M. J. (2012). “Circulation patterns and shoreline response induced by submerged breakwaters,” in Proceedings of the 33rd International Conference on Coastal Engineering, (Spain: Coastal Engineering Research Council), 2012. doi: 10.9753/icce.v33.structures.25
Winter, G., Storlazzi, C., Vitousek, S., van Dongeren, A., McCall, R., Hoeke, R., et al. (2020). Steps to Develop Early Warning Systems and Future Scenarios of Storm Wave-Driven Flooding Along Coral Reef-Lined Coasts. Front. Mar. Sci. 7:199. doi: 10.3389/fmars.2020.00199
Keywords: coral reefs, wave runup, ecosystem services, coastal protection, reef degradation, reef restoration, climate change, coastal risk
Citation: Roelvink FE, Storlazzi CD, van Dongeren AR and Pearson SG (2021) Coral Reef Restorations Can Be Optimized to Reduce Coastal Flooding Hazards. Front. Mar. Sci. 8:653945. doi: 10.3389/fmars.2021.653945
Received: 15 January 2021; Accepted: 01 April 2021;
Published: 10 May 2021.
Edited by:
Fabrice Renaud, University of Glasgow, United KingdomReviewed by:
Siddharth Narayan, East Carolina University, United StatesBarbara Zanuttigh, University of Bologna, Italy
Copyright © 2021 Roelvink, Storlazzi, van Dongeren and Pearson. This is an open-access article distributed under the terms of the Creative Commons Attribution License (CC BY). The use, distribution or reproduction in other forums is permitted, provided the original author(s) and the copyright owner(s) are credited and that the original publication in this journal is cited, in accordance with accepted academic practice. No use, distribution or reproduction is permitted which does not comply with these terms.
*Correspondence: Floortje E. Roelvink, Zmxvb3J0amUucm9lbHZpbmtAZGVsdGFyZXMubmw=