Acclimation History of Elevated Temperature Reduces the Tolerance of Coralline Algae to Additional Acute Thermal Stress
- School of Environment and Science & Australian Rivers Institute, Griffith University, Nathan, QLD, Australia
Increasing atmospheric CO2 is driving major environmental changes in the ocean, such as an increase in average ocean temperature, a decrease in average ocean pH (ocean acidification or OA), and an increase in the number and severity of extreme climatic events (e.g., anomalous temperature events and heatwaves). Uncertainty exists in the capacity for species to withstand these stressors occurring concomitantly. Here, we tested whether an acclimation history of ocean warming (OW) and OA affects the physiological responses of an abundant, reef-building species of crustose coralline algae (CCA), Porolithon cf. onkodes, to chronic and acute thermal stress. To address this, we exposed algae to varying temperature and pH levels for 6 weeks and this chronic treatment experiment was followed by an acute exposure to an anomalous temperature event (+4–6°C from acclimation temperature). Net photosynthetic rate was negatively affected across all treatments by increasing temperature during the acute temperature event, however, algae acclimated to the control temperature were able to maintain photosynthetic rates for +4°C above their acclimation temperature, whereas algae acclimated to elevated temperature were not. Average relative change in O2 produced resulted in a 100–175% decrease, with the largest decrease found in algae acclimated to the combined treatment of elevated temperature and reduced pH. We conclude that acclimation to chronic global change stressors (i.e., OW and OA) will reduce the tolerance of P. cf. onkodes to anomalous increases in temperature, and this may have implications for reef building processes.
Introduction
Global change and increasing atmospheric CO2 are driving multiple marine environmental changes such as ocean warming (OW) and ocean acidification (OA), which are both predicted to be major threats to marine organisms and ecosystems globally (Doney et al., 2012; Poloczanska et al., 2013). OW and OA can negatively impact phenological events, physiological processes of organisms, and their overall distributions (Doney et al., 2012; Poloczanska et al., 2013). Alongside OW and OA, the number and severity of extreme climatic events (e.g., heatwaves and anomalous temperature events) are projected to increase, which have been found to have significant negative ecological impacts on species and ecosystems more broadly (Wernberg et al., 2016; Smale et al., 2019).
Tropical species inhabiting coral reef ecosystems are particularly susceptible to thermal stress as many reef organisms already exist at or near their upper thermal tolerance limit (Baker et al., 2008; Lough et al., 2018) and this could be exacerbated by OW in recent decades (Hoegh-Guldberg et al., 2007; Kroeker et al., 2020). Studies investigating the effects of warming on coral reef macroalgae are limited however (Pakker et al., 1995; Bennett et al., 2018; Cornwall et al., 2019), with even fewer experiments examining the effects of warming on reef-building crustose coralline algae (CCA) (Cornwall et al., 2019). CCA are a group of calcifying, red macroalgae that are the cement of reefs, aiding in building and maintaining the carbonate framework of coral reefs worldwide (Adey, 1998). To date, many OW studies on CCA have been done over short durations (Vásquez-Elizondo and Enríquez, 2016) and/or have not coupled warming with additional stressors such as OA (Anton et al., 2020) and subsequent anomalous temperature events, like heatwaves (Cornwall et al., 2019). From the current literature, we know that tropical coralline algae are likely less robust than fleshy macroalgae living in the same environment (Anton et al., 2020) and potentially equally or sometimes more robust than corals living in the same environment (Kornder et al., 2018; Cornwall et al., 2019; Anton et al., 2020). Furthermore, we know that increasing temperatures, even without the addition of global change drivers, can cause decreases in physiological processes, such as calcification and photosynthesis (Vásquez-Elizondo and Enríquez, 2016; Schubert et al., 2019). Despite the current knowledge on the responses of CCA to global change drivers both in isolation and in combination, it is still not known whether an acclimation history of OW and OA plays a role in the physiological responses of coralline algae to chronic and acute thermal stress.
The aim of this study was to investigate how acclimation history impacts the ability of a reef building species of CCA, Porolithon cf. onkodes, hereinafter, P. onkodes, to tolerate sudden, acute increases in temperature. Global change drivers, such as OW and OA, have been found to variably impact processes of coralline algae (Johnson and Carpenter, 2012; Kim et al., 2020; Page and Diaz-Pulido, 2020), however, how the acclimation history of these stressors will impact plasticity to additional acute stressors is less understood. In this study, P. onkodes was held under constant temperature and/or pH conditions that reflect IPCC global predictions, specifically the representative concentration pathway (RCP) 8.5 (IPCC, 2013), or present day control conditions for 6 weeks. After the 6-week acclimation period P. onkodes fragments were subjected to an acute temperature stress (+1°C h–1 until 33°C) whilst percent oxygen (O2) was monitored, and calcification/dissolution was estimated as proxies for physiological stress. CCA as a group have been found to be variably, but largely negatively impacted under combined stressors of OW and OA (Martin and Gattuso, 2009; Brodie et al., 2014; Hofmann and Bischof, 2014; Scherner et al., 2016), therefore, it was hypothesized that a history of exposure to these two stressors will cause additive pressure during an acute warming event, and therefore result in decreased O2 production and increased skeletal dissolution.
Materials and Methods
Crustose coralline algae fragments, ∼ 3 cm × 3 cm in size, of the orange color morph of P. onkodes, were collected in October 2018 from the reef flat area of Yonge Reef (14°37′32.1″S, 145°38′06.2″E), Great Barrier Reef (GBR), Australia between 1 and 3 m depth. After collection, CCA fragments were brought back to Lizard Island Research Station (LIRS) and kept in an outdoor, flow-through, common garden aquarium at constant temperature (∼27.2°C), pH (8.0), and salinity (35 ppt), similar to seawater parameters measured at collection site. Seawater at LIRS is pumped from the reef lagoon into header tanks and then gravity fed into the aquarium system. Algae were thoroughly cleaned of epiphytes within 24 h of their collection. All fragments were examined under a dissecting microscope to ensure all were the same species based on morphological identification. Exposed calcium carbonate skeleton was sealed using Coral Glue® (Ecotech Marine). Light at collection site was between 520 and 650 μmol quanta m–2 s–1 at ∼3 m depth (∼14:00). Within the experimental tanks, unshaded natural light ranged from 100 to 200 μmol quanta m–2 s–1 dependent on time of day.
After CCA were acclimated in the common garden, ambient conditions, fragments were allocated to treatment tanks. Four experimental treatments were targeted and used during this experiment: ambient control (27.2°C and pH 8.0; 450 μatm pCO2), reduced pH/elevated pCO2 (27.2°C and pH 7.7; 1,000 μatm pCO2), elevated temperature (29.5°C and pH 8.0; 450 μatm pCO2), and the combined treatment of elevated temperature and reduced pH/elevated pCO2 (29.5°C and pH 7.7; 1,000 μatm pCO2). The experimental setup included an outdoor, flow-through, four-header sump (275 L each) system and twenty 32 L individual treatment tanks, allocated randomly to the four experimental treatments. Each header tank was supplied constantly with water from the reef lagoon and water from treatment sumps was continuously pumped into respective treatment tanks using Pondmaster 3600 Fountain Pumps (Pond One®). Light was provided naturally and measured daily, around 12:00, using an underwater quantum sensor LI-192 connected to a light meter LI-250 (LI-COR, United States), averaging about 140 μmol quanta m–2 s–1. In treatments where temperature and/or pH were manipulated, levels were increased over 7 days, ultimately reaching a 0.3 unit decrease in pH (∼1,000 μatm pCO2) and a 2.3°C increase in temperature, relating to levels predicted by the IPCC under RCP8.5 scenario for end of century levels (IPCC, 2019). P. onkodes fragments were held at constant experimental treatments for 6 weeks. pH was regulated by the use of pH-controllers (AquaController, Neptune Systems, United States) that injected either pure CO2 or ambient air into the header sumps until desired value was reached. To obtain equivalent, desired pH value the offset for each of the Neptune Systems pH probes (one probe per treatment and offset accordingly after each measurement) was calculated from values obtained from a pH meter (Mettler Toledo, SevenGo Duo SG98) paired with a pH electrode with integrated temperature probe (Mettler Toledo, InLab Routine Pro) calibrated on total scale (pHT) using Tris–HCl buffers (Dickson et al., 2007). pHT and temperature were measured twice daily (08:00 and 15:00) in each experimental tank and sump. Salinity was measured daily using a conductivity meter (Mettler Toledo, SevenGo Pro). Temperature was maintained and manipulated by use of titanium heaters (EcoPlus, Aqua Heat, 300 W) within each sump and small 50 W glass heaters (Aqua One®) within respective experimental tanks that were set to desired temperature. Temperature was adjusted daily to mimic ambient temperature based on season (publicly available data from Australian Institute of Marine Science)1 and +2.3°C was added in addition to the adjusted seasonal temperature for elevated temperature treatments. Flow to each experimental tank was maintained at a rate of 20 L h–1, allowing for complete turnover of the experimental tanks twice per hour. A submersible water pump (Resun® SP-1100 8 W) provided additional water movement within each experimental tank. Total alkalinity (AT) was measured every 3 days, water was collected around 09:00, for the first week of the experiment, and then every 6 days following the initial week. AT was determined through potentiometric titration on an automatic titrator (Mettler Toledo, T50) following standard operating procedures (SOP) 3b (Dickson et al., 2007). AT, pHT, temperature, and salinity were used to calculate the remaining carbonate chemistry parameters using the seacarb package version 3.2.12 (Gattuso et al., 2019) in R (Supplementary Table 1). High Mg-calcite saturation state was calculated for a 16.4% MgCO3 following Diaz-Pulido et al. (2012). CCA mortality or changes in health (identified as a paling or bleaching of the pigmented layer) were monitored throughout the 6-week experiment.
After 6 weeks of acclimation, or preconditioning, to temperature and pH treatments, we tested the role of acclimation history on the response of P. onkodes to acute thermal stress by conducting short-term temperature experiments. Five fragments of P. onkodes from each of the four treatments were thoroughly cleaned under a microscope 48 h prior to incubations and then were gently brushed 2 h before incubations to remove epiphytes from surface of algae. Fragments were placed into sealed, acrylic, incubation chambers (∼150 mL) filled with respective OW and OA treatment water (n = 5). Chambers were placed within a water bath on top of a magnetic stir plate. Light was maintained at 140 μmol quanta m–2 s–1 (MarsAqua 300 W LED, Full Spectrum), which was the average daily light intensity during the 6-week experiment. Temperature of the water bath was controlled via an immersion circulating heater (CORIO CD, Julabo). Each chamber was equipped with a magnetic stir bar to ensure water movement throughout incubation, a temperature sensor (Pt100), and a PreSens dipping O2 optode (DP-PSt3). Optodes were connected to a 10-channel trace O2 meter (OXY-10 SMA trace, G2, PreSens, Germany). O2 sensors were calibrated prior to every incubation to 0% O2, obtained through addition of sodium dithionite (Na2S2O4), and 100% O2 seawater, obtained through bubbling water with air. Before beginning the acute warming experiment, algae were held at acclimation temperature for 30 min and dissolved O2 was measured to establish O2 production after 6-weeks in acclimation treatment. Once these values were obtained, temperature was lowered to closest whole number (i.e., 27°C or 29°C dependent on acclimation temperature treatment) and algae were allowed to acclimate again for 30 min before beginning the acute warming experiment. Temperature was then increased by 1°C h–1 (a rate of about 0.017°C min–1). Dissolved O2 and temperature were continuously measured and recorded every minute throughout the incubation. Incubations lasted up to 6 h to allow for an ending temperature of 33°C within the incubation chambers from respective acclimation temperature. Temperature data on reef crest sites and surrounding reef environments have recorded temperatures of over 33°C or an increase of over +4°C within a daily cycle (Lough, 1998). Therefore, the maximum temperature of heating during this experiment allowed for an environmentally realistic temperature maximum for a day on a reef flat or crest in the Northern GBR. A seawater blank with no CCA was run with each incubation and used for normalization in data analysis. All incubations were started at the same time every day to ensure no diurnally caused differences in photosynthetic output and calcification. Net photosynthesis (NP) was calculated as follows:
where sO2 is the slope of the linear regression line for change in O2 versus time (μmol L–1 h–1) at each measurement temperature, V is the volume of the incubation chamber (L), and SA is the surface area (cm2) determined through aluminum foil technique (Marsh, 1970).
Crustose coralline algae net calcification (gn, μmol CaCO3 cm–2 h–1) was estimated using the TA anomaly technique (Smith and Key, 1975; Schneider and Erez, 2006; Anthony et al., 2013; Cohen et al., 2017). Prior to the start of the incubations, seawater was sampled from the experimental tanks (initial AT) and directly from incubation chambers at the end of each acute temperature experiment (final AT). Totaling two AT samples (initial AT and final AT) for each individual and blank seawater chamber. Seawater samples were filtered through 20 μm filters into titration cups (47–55 mL of sample) and immediately processed through potentiometric titration. Net calcification was estimated based on the difference in AT (ΔAT), which was normalized to the density of seawater at sampling temperatures (i.e. 27.2, 29.5, or 33°C) after the incubations, assuming AT was solely affected by calcification and dissolution processes, and for every 1 mol of CaCO3 precipitated, AT is reduced by 2 molar equivalents (Chisholm and Gattuso, 1991),
where V is the volume of the incubation chamber (L), Δt is the time between initial AT and final AT (h), and S is the surface area of the CCA (cm2). Changes in AT ranged from 18.33 to 572.20 μeq–L.
All data were analyzed in the statistical software R v3.6.1. The absolute value of O2, or net photosynthesis, at acclimation temperatures (27.2 and 29.5°C), and net calcification data were analyzed by two-way ANOVA (with pH and temperature treatments as main factors and fragments as replicates, n = 4–5). Although there was no mortality in any of the treatments, one sample is missing from the elevated temperature and reduced pH treatment due to a faulty O2 probe. Net photosynthesis and calcification data were tested for normality using Shapiro Wilk’s and Levene tests for homoscedasticity. Linear mixed effect model residuals were tested for normality through graphical analyses of residuals, using QQ normality plots. All data met tests of normality.
Relative change in O2 was calculated by taking the starting, absolute O2 value at respective acclimation temperature and subtracting that value from each following measurement during the acute warming experiment. To find the variation of the relative change in O2 production at each ramp temperature due to acclimation treatment a linear mixed effects model was used with a random intercept. Acclimation temperature and pH were set as fixed factors/effects and sample as the random effect, which allowed for variation of sample effects across ramping temperatures. The linear mixed effect model was done using the lme function from the nlme package (v3.1.141) (Pinheiro et al., 2013) in R and if significant differences were detected, Tukey’s pairwise tests were conducted using the glht function from the Multcomp package (v1.4.10) (Hothorn et al., 2016).
Results
After 6 weeks in acclimation treatments, net photosynthesis, or O2 production (μmol O2 cm–2 h–1), was measured on adult fragments of P. onkodes. At the end of the 6-week acclimation period, no significant effect of acclimation temperature, pH, or the interaction of the two (ANOVA, p = 0.427, 0.102, and 0.105, respectively, Supplementary Table 2 and Figure 1) was found. Absolute values of mean O2 production ranged from 1.17 to 4.84 μmol O2 cm–2 h–1 in samples acclimated to the control temperature of 27.2°C and ranged from 1.80 to 6.61 μmol O2 cm–2 h–1 in samples acclimated to elevated temperature of 29.5°C (Supplementary Table 2 and Figure 1).
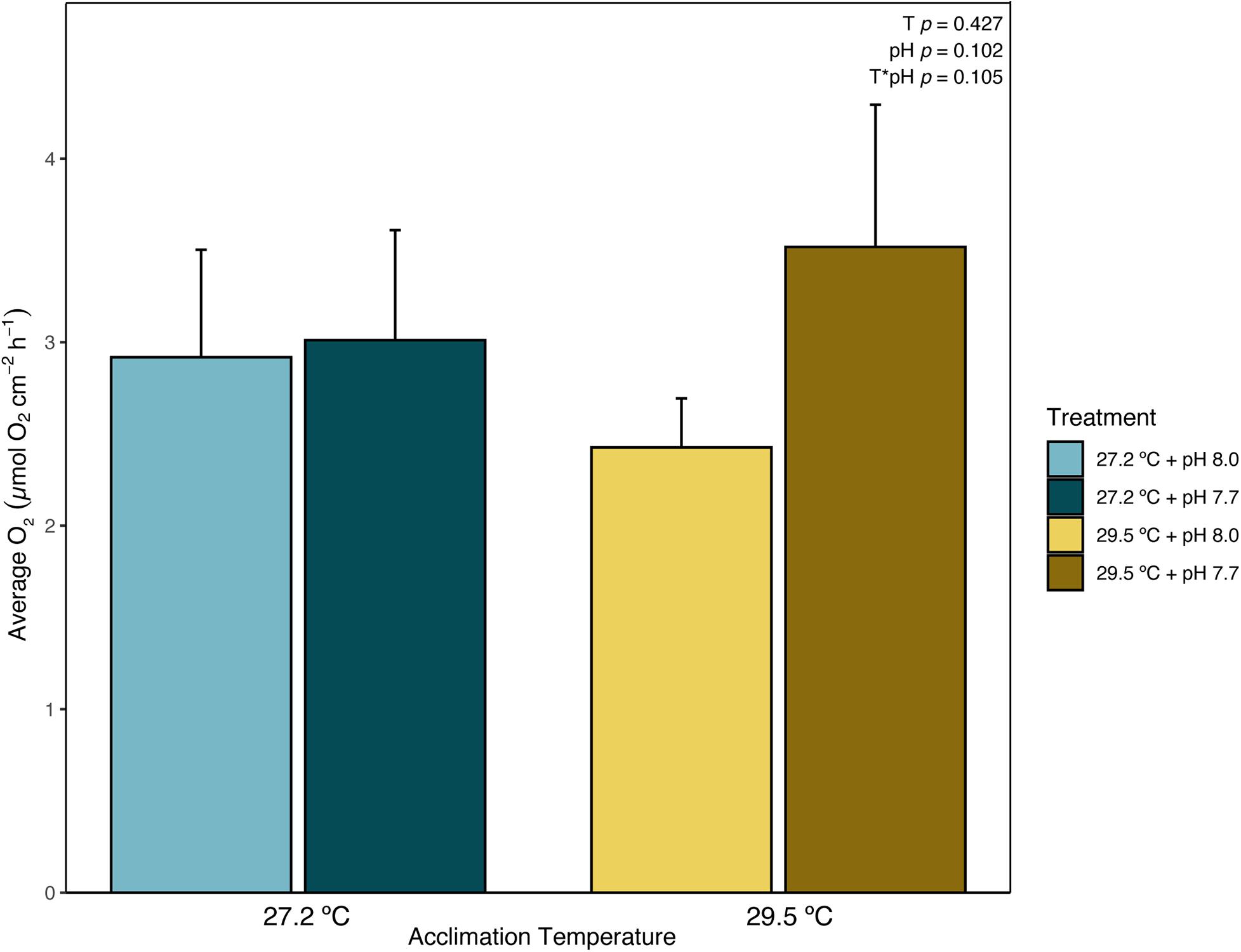
Figure 1. Average net photosynthesis (μmol O2 cm–2 h–1) of Porolithon cf. onkodes after a 6-week acclimation period to constant, experimental levels of temperature and/or pH (27.2 or 29.5°C and pH 8.0 or 7.7). Each bar is mean O2 production per treatment ± standard error (SE). n = 4–5. Blue bars equate to control temperature, 27.2°C, and yellow hued bars to elevated temperature, 29.5°C, with pH treatment shown as different shades within temperature treatment. p values for the effect of temperature (T), pH, and the interaction between temperature and pH (T × pH) are listed from Supplementary Table 2 within the figure.
O2 production of P. onkodes across all treatments was adversely affected by the acute warming experiment (Table 1 and Figure 2). Ramp temperature significantly affected the relative change in O2, with O2 primarily decreasing across all treatments during the acute temperature experiment (Figure 2). The decline in O2, on average a 0.530 μmol O2 cm–2 h–1 decrease with each degree of temperature increase, from starting acclimation temperature to the ending ramp temperature of 33°C can be attributed to ramp temperature (Table 1). There was a significant effect of acclimation temperature on O2 production at particular ramp temperatures, seen in a steeper slope of O2 production in the elevated acclimation temperature treatments than control temperature treatments, irrespective of pH treatment (Figure 2 and Supplementary Figure 1). The slope for the elevated temperature treatment was 42% higher/steeper than that of the control treatment (Supplementary Figure 1). Acclimation pH independently and in combination with elevated acclimation temperature and/or ramp temperature did not have a significant effect on the relative change of O2 during the acute temperature experiment (Table 1).
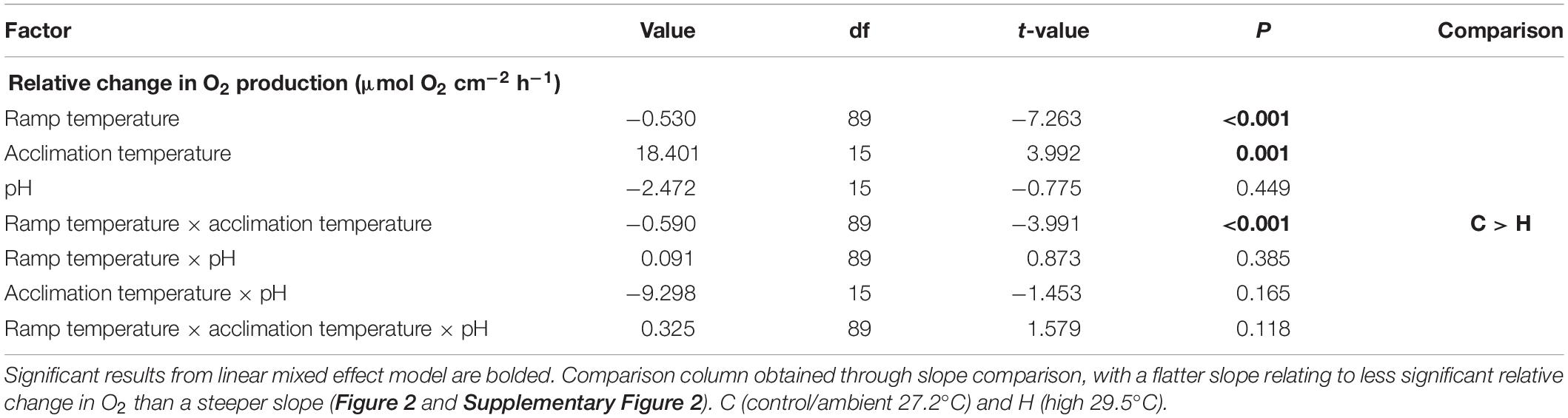
Table 1. Summary of statistical information from a linear mixed effects model with a random intercept on the relative change of O2 production during the acute warming experiment.
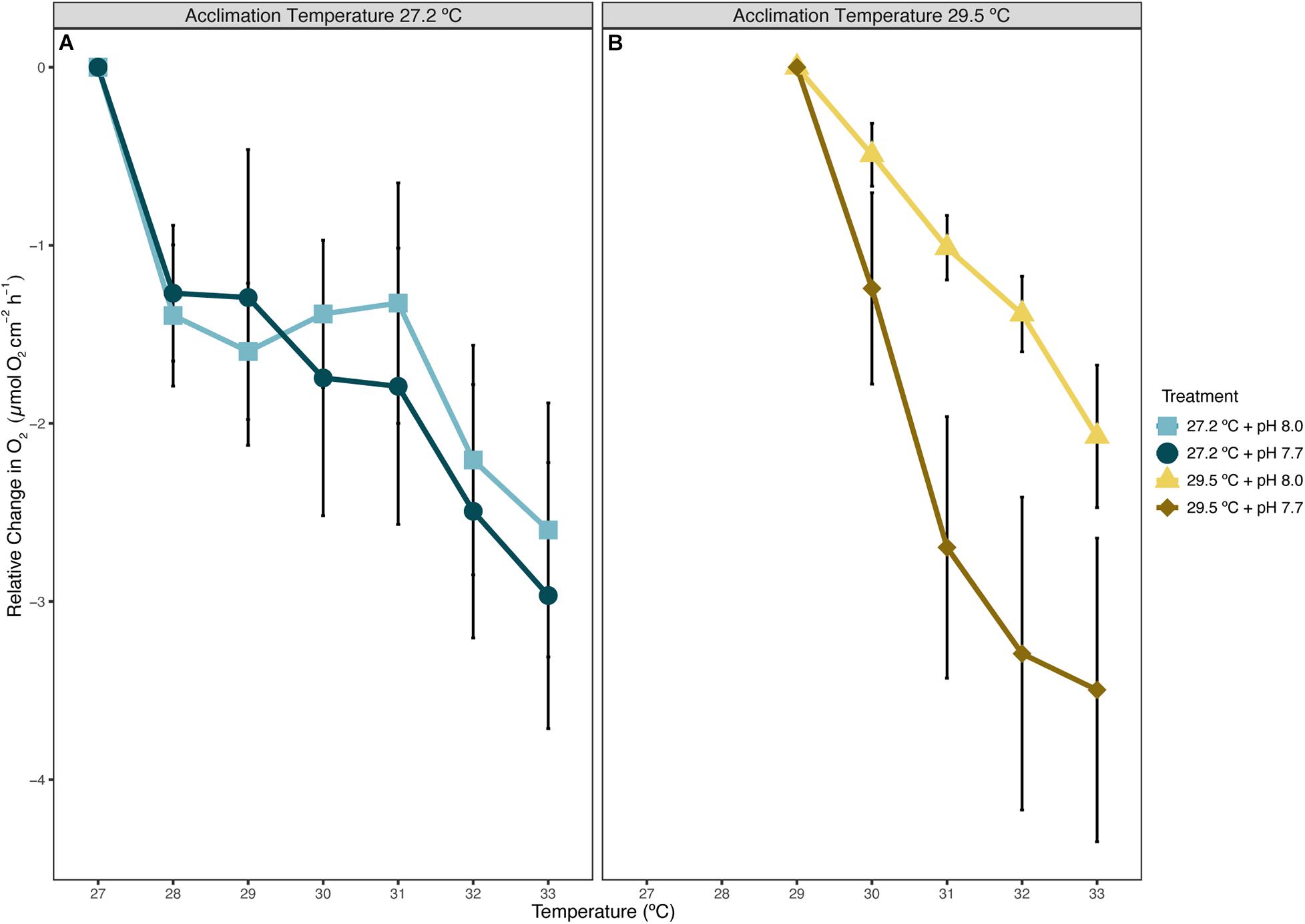
Figure 2. Relative change in average O2 produced by fragments of Porolithon cf. onkodes from mean, respective acclimation treatment temperature, panel (A) acclimated at 27.2°C for 6 weeks, (B) acclimated at 29.5°C for 6 weeks. O2 begins at “0” or the starting average O2 per acclimation temperature. Each point is the mean, relative change of O2 ± standard error (SE) for each treatment at each ramp temperature. n = 4–5. Blue points equate to control temperature, 27.2°C, and yellow hued points to elevated temperature, 29.5°C, with pH treatment shown as different shades and shapes within temperature treatment.
Average CCA net calcification ranged from 0.90 to 1.35 μmol CaCO3 cm–2 h–1 (Table 2). There was no significant effect of the temperature or pH treatment on net calcification (ANOVA, p = 0.079 and 0.402, respectively, Supplementary Table 3 and Supplementary Figure 2).
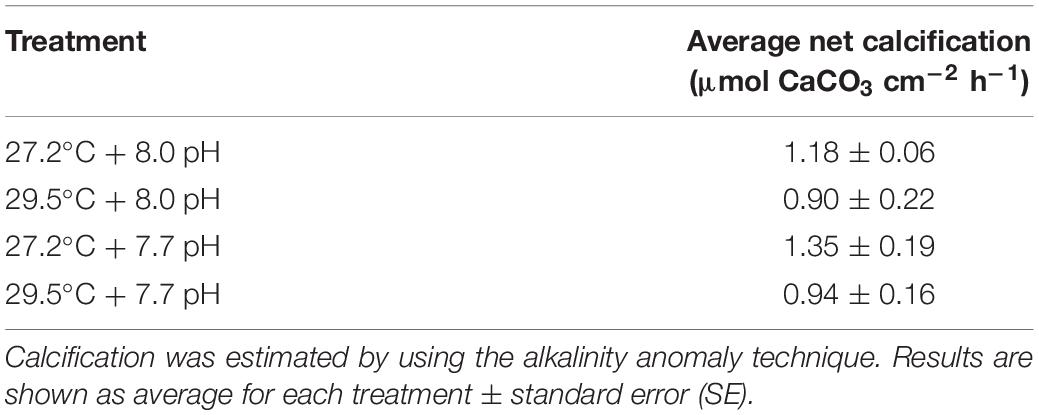
Table 2. Summary of average net calcification (μmol CaCO3 cm–2 h–1) results from Porolithon cf. onkodes samples.
Discussion
An acclimation history of elevated temperature may exacerbate the adverse effects of acute increases in temperature on CCA productivity. Our study provides insight into the role of OW and OA acclimation history in the thermal tolerance of an abundant and important species of reef-building coralline algae. Extreme climatic events such as rapid temperature increases (e.g., heat waves) are predicted to occur more frequently in future oceans with increasing atmospheric CO2 (Oliver et al., 2018) and are expected to have global impacts on marine ecosystems (Smale et al., 2019). Our findings show steady increases in temperature, only a few degrees above their summer average temperature, result primarily in decreases in amount of O2 produced by P. onkodes. The degree of effect was largely dependent on the temperature P. onkodes was acclimated to, with those kept under elevated temperature (∼2.3°C above ambient control temperature) experiencing a more rapid loss of performance in terms of O2 production. This study provides information on how this species of calcifying algae will fair in future oceans and sheds light on how increasing average ocean temperatures will play a role in the response of reef-forming species to acute, anomalous increases in temperature.
Exposure to chronic elevated temperature and/or reduced pH had a minimal effect on average O2 production when measured at a static acclimation temperature. These results are in line with some studies on tropical coralline algae, where reduced pH (Comeau et al., 2016) or the combination of elevated temperature and reduced pH (Page and Diaz-Pulido, 2020) had little to no effect on O2 production. On the other hand, there are also studies that have documented decreased photosynthetic rates in P. onkodes under high temperature and low pH in the southern GBR (Anthony et al., 2008), although this may have been due to the increased mortality in their experimental individuals (Diaz-Pulido et al., 2012). The final average O2 production data from the chronic acclimation temperature experiment suggest this algae population is largely robust to static, chronic changes in their environment, even possibly benefitting from a combination of elevated temperature and reduced pH, where O2 production was higher (5.07–44.86%) when compared to all other treatments, however not significantly. These results did not persist after P. onkodes was exposed to the acute warming experiment at the end of the 6-week acclimation.
A history of elevated temperature appeared to exacerbate the effects of acute thermal stress on P. onkodes. This response was predicted, as elevated, experimental acclimation temperature was expected to be an additive stressor when followed by an acute warming event. High temperature stress is understood to inhibit the photosynthetic apparatus and therefore photosynthetic processes (Mathur et al., 2014). There was a ∼125% total relative decrease in O2 production from starting acclimation temperature (27°C) to 33°C for P. onkodes acclimated to control temperature. In the individuals acclimated to elevated temperature, this relative decrease was ∼100% for the elevated temperature at control pH and ∼175% for the elevated temperature at reduced pH. The treatment of reduced acclimation pH combined with elevated temperature seemingly intensified the alga’s negative response to the acute warming experiment. Interestingly, P. onkodes acclimated to elevated temperature at control pH had the smallest relative change compared to other treatments, however, the slope of this change was 42% higher than the control temperature treatment, with an R2 of 0.99 compared to an R2 of 0.74, respectively. The steeper slope and higher R2 value of the elevated temperature treatment is most likely linked to their acclimation history of elevated temperature, in which further increases in temperature resulted in a steady, unbroken reduction in O2. This result of a long-term increase in temperature followed by a short-term heatwave-like experiment inducing a reduction in physiological rates has been seen previously in species of temperate articulated coralline algae (Rendina et al., 2019), but has not been documented for tropical reef-building corallines.
pH played a marginal role in magnifying the level of reduction in O2 production resulting from increased acclimation temperature. This was seen most prominently when comparing the control treatment to the reduced pH treatment, where a reduction in pH resulted in a 12.4% larger decrease in O2 production compared to the control pH treatments. This finding supports a meta-analysis that found CO2 enrichment reduces photosynthesis in calcifying algae by 28% on average (Kroeker et al., 2013), however, this result only took into account three species of CCA out of the eight studies used to calculate this result. Some CCA specific studies have found elevated pCO2/reduced pH can increase photosynthesis (Semesi et al., 2009; Noisette et al., 2013; Ordoñez-Alvarez et al., 2018), therefore it was expected in the current study that an acclimation history of elevated pCO2/reduced pH might partially mitigate the physiological stress incurred by an acute warming event (Koch et al., 2013). Our experiment, however, demonstrated that reduced pH slightly exacerbated the negative effect of acute thermal stress on coralline algae.
P. onkodes never consumed O2 faster than it was produced across any of the treatments. This result suggests some level of tolerance to acute, thermally stressful events both in current and future oceans. Additionally, unlike previous studies conducted with other calcifiers (Diaz-Pulido et al., 2012; Prada et al., 2017; Anderson et al., 2019), survivorship was not impacted amongst our algae. Our findings of O2 consumption never overtaking O2 production and no mortality are supported by previous work done on a tropical, Atlantic species of CCA, Neogoniolithon, that was exposed to elevated temperatures of 34°C, where positive net photosynthesis was maintained and no mortality occurred at elevated temperature, suggesting this species has a high thermal tolerance (Anderson, 2006). Our findings suggest that P. onkodes is able to largely withstand chronic, elevated levels of temperature up to +2.3°C and an increase in CO2 of about 600 μatm (from current levels), although further, rapid and acute temperature increases may cause considerable physiological stress to the algae.
O2 production results from the acute warming experiment suggest that acclimation history, particularly thermal, plays a role in the level of reduction seen in this specific physiological process, however, is this also seen in other crucial physiological processes of CCA? Data presented here did not show a significant effect of treatment condition on average net calcification. Temperature appeared to have more of an effect than pH on calcification in this study, with a marginal decrease in average net calcification under elevated temperature treatments. Although this result was not significant, it does support O2 production findings from this study that temperature, not pH, has a larger impact on physiological processes of P. onkodes. These data suggest that, at least after a period of 6 weeks and following an acute increase in temperature, this population of P. onkodes is largely able to maintain net calcification rates. Calcification and dissolution values found in this study were comparable with previous studies that manipulated environmental parameters using CCA species from the GBR (Diaz-Pulido et al., 2012) and elsewhere (Martin and Gattuso, 2009). Results from studies measuring calcification after exposure to increased temperature and/or increased pCO2/decreased pH vary (Martin and Gattuso, 2009; Diaz-Pulido et al., 2012; Vásquez-Elizondo and Enríquez, 2016; McNicholl et al., 2020). In coralline algae taxa, calcification can decrease significantly when temperature thresholds are crossed (Vásquez-Elizondo and Enríquez, 2016; Cornwall et al., 2019) and with elevated temperature and pCO2 (Martin and Gattuso, 2009). In a Southern GBR population of P. cf. onkodes, calcification decreased under reduced pH levels, with this result being enhanced when combined with increased temperature (Diaz-Pulido et al., 2012). The population of P. onkodes examined in the current study responded differently from this Southern GBR population. It is possible that the difference in response of these two populations is due to the acute temperature stress the algae were exposed to at the end of the acclimation period and/or slightly different experimental conditions or varying techniques to measure net calcification (e.g., buoyant weight vs. TA anomaly).
In conclusion, our findings suggest thermally stressful events such as acute, anomalous increases in temperature or heatwaves can induce different ecophysiological responses compared to those occurring from chronic (sustained), constant environmental changes, and understanding these responses could be more informative than those responses resulting from constant conditions (McCoy and Widdicombe, 2019). The wide thermal gradient that P. onkodes occupies may give them existing plasticity or tolerance to thermal stress, at least to constant levels of elevated temperature, however, when P. onkodes is further exposed to an acute, increase in temperature, this plasticity or tolerance does not persist. Future work into existing thermal tolerance, thermal limits, and standing genetic variation of populations of P. onkodes could generate illuminating data on phenotypes with different thermal tolerances and optima (Somero, 2010). This information could give us further insight into the fate of critical reef-building species in the face of rapid global change.
Data Availability Statement
The original contributions presented in the study are included in the article/Supplementary Materials, further inquiries can be directed to the corresponding authors.
Author Contributions
TP and GD-P designed the experiment. TP conducted the experiment and analyzed the data, with guidance from GD-P and wrote the manuscript, with crucial guidance and input from GD-P. EB aided in collecting the algae, conducting and maintaining the experiment, and reviewed and edited the manuscript. All authors contributed to the article and approved the submitted version.
Funding
This study was funded by the Australian Research Council grant DP160103071 awarded to GD-P.
Conflict of Interest
The authors declare that the research was conducted in the absence of any commercial or financial relationships that could be construed as a potential conflict of interest.
Acknowledgments
The authors would like to thank Patrick Gartrell for aiding during collections and setting up of the experiment and James McBroom for his valuable statistical help. The authors would also like to thank Alexander Carlson and Alea Laidlaw for assisting during the experiment and for day-to-day maintenance. The authors also thank the directors and maintenance staff of Lizard Island Research Station for their assistance and continued support throughout the duration of this study.
Supplementary Material
The Supplementary Material for this article can be found online at: https://www.frontiersin.org/articles/10.3389/fmars.2021.660196/full#supplementary-material
Footnotes
References
Adey, W. H. (1998). Coral reefs: algal structured and mediated ecosystems in shallow, turbulent, alkaline waters. J. Phycol. 34, 393–406. doi: 10.1046/j.1529-8817.1998.340393.x
Anderson, B. C. (2006). Response of Tropical Marine Macroalgae to Thermal Stress. MSc. thesis. Boca Raton, FL: Florida Atlantic University.
Anderson, K. D., Cantin, N. E., Casey, J. M., and Pratchett, M. S. (2019). Independent effects of ocean warming versus acidification on the growth, survivorship and physiology of two Acropora corals. Coral Reefs 38, 1225–1240. doi: 10.1007/s00338-019-01864-y
Anthony, K. R. N., Diaz-Pulido, G., Verlinden, N., Tilbrook, B., and Andersson, A. J. (2013). Benthic buffers and boosters of ocean acidification on coral reefs. Biogeosciences 10, 4897–4909. doi: 10.5194/bg-10-4897-2013
Anthony, K. R. N., Kline, D. I., Diaz-Pulido, G., Dove, S., and Hoegh-Guldberg, O. (2008). Ocean acidification causes bleaching and productivity loss in coral reef builders. Proc. Natl. Acad. Sci. U.S.A. 105:17442. doi: 10.1073/pnas.0804478105
Anton, A., Randle, J. L., Garcia, F. C., Rossbach, S., Ellis, J. I., Weinzierl, M., et al. (2020). Differential thermal tolerance between algae and corals may trigger the proliferation of algae in coral reefs. Glob. Chang. Biol. 26, 4316–4327. doi: 10.1111/gcb.15141
Baker, A. C., Glynn, P. W., and Riegl, B. (2008). Climate change and coral reef bleaching: an ecological assessment of long-term impacts, recovery trends and future outlook. Estuar. Coast. Shelf Sci. 80, 435–471. doi: 10.1016/j.ecss.2008.09.003
Bennett, J. M., Calosi, P., Clusella-Trullas, S., Martínez, B., Sunday, J., Algar, A. C., et al. (2018). GlobTherm, a global database on thermal tolerances for aquatic and terrestrial organisms. Sci. Data 5:180022. doi: 10.1038/sdata.2018.22
Brodie, J., Williamson, C. J., Smale, D. A., Kamenos, N. A., Mieszkowska, N., Santos, R., et al. (2014). The future of the northeast Atlantic benthic flora in a high CO2 world. Ecol. Evol. 4, 2787–2798. doi: 10.1002/ece3.1105
Chisholm, J. R. M., and Gattuso, J.-P. (1991). Validation of the alkalinity anomaly technique for investigating calcification of photosynthesis in coral reef communities. Limnol. Oceanogr. 36, 1232–1239. doi: 10.4319/lo.1991.36.6.1232
Cohen, S., Krueger, T., and Fine, M. (2017). Measuring coral calcification under ocean acidification: methodological considerations for the 45Ca-uptake and total alkalinity anomaly technique. PeerJ 5:e3749.
Comeau, S., Carpenter, R. C., and Edmunds, P. J. (2016). Effects of pCO2 on photosynthesis and respiration of tropical scleractinian corals and calcified algae. ICES J. Mar. Sci. 74, 1092–1102. doi: 10.1093/icesjms/fsv267
Cornwall, C. E., Diaz-Pulido, G., and Comeau, S. (2019). Impacts of ocean warming on coralline algal calcification: meta-analysis, knowledge gaps, and key recommendations for future research. Front. Mar. Sci. 6:186. doi: 10.3389/fmars.2019.00186
Diaz-Pulido, G., Anthony, K. R. N., Kline, D. I., Dove, S., and Hoegh-Guldberg, O. (2012). Interactions between ocean acidification and warming on the mortality and dissolution of coralline algae. J. Phycol. 1, 32–39. doi: 10.1111/j.1529-8817.2011.01084.x
Dickson, A. G., Sabine, C. L., and Christian, J. R. (2007). Guide to Best Practices for Ocean CO2 Measurements. Sidney, BC: North Pacific Marine Science Organization.
Doney, S. C., Ruckelshaus, M., Emmett Duffy, J., Barry, J. P., Chan, F., English, C. A., et al. (2012). Climate change impacts on marine ecosystems. Ann. Rev. Mar. Sci. 4, 11–37. doi: 10.1146/annurev-marine-041911-111611
Gattuso, J.-P., Epitalon, J.-M., Lavigne, H., Orr, J. C., Gentili, B., Hagens, M., et al. (2019). Seacarb: Seawater Carbonate Chemistry with R Package Version 3.2.12.
Hoegh-Guldberg, O., Mumby, P. J., Hooten, A. J., Steneck, R. S., Greenfield, P., Gomez, E., et al. (2007). Coral reefs under rapid climate change and ocean acidification. Science 318, 1737–1742. doi: 10.1126/science.1152509
Hofmann, L. C., and Bischof, K. (2014). Ocean acidification effects on calcifying macroalgae. Aquat. Biol. 22, 261–279. doi: 10.3354/ab00581
Hothorn, T., Bretz, F., Westfall, P., Heiberger, R. M., Schuetzenmeister, A., Scheibe, S., et al. (2016). Package ‘multcomp’. Simultaneous Inference in General Parametric Models. Vienna: Project for Statistical Computing.
IPCC (2013). Climate Change 2013: The Physical Science Basis. Contribution of Working Group I to the Fifth Assessment Report of the Intergovernmental Panel on Climate Change, eds T. F. Stocker, D. Qin, G.-K. Plattner, M. Tignor, S. K. Allen, J. Boschung, et al. (New York, NY: Cambridge University Press).
IPCC (2019). “Summary for policymakers,” in IPCC Special Report on the Ocean and Cryosphere in a Changing Climate, eds H.-O. Pörtner, D. C. Roberts, V. Masson-Delmotte, P. Zhai, M. Tignor, E. Poloczanska, et al. (in press).
Johnson, M. D., and Carpenter, R. C. (2012). Ocean acidification and warming decrease calcification in the crustose coralline alga Hydrolithon onkodes and increase susceptibility to grazing. J. Exp. Mar. Biol. Ecol. 434, 94–101. doi: 10.1016/j.jembe.2012.08.005
Kim, J.-H., Kim, N., Moon, H., Lee, S., Jeong, S. Y., Diaz-Pulido, G., et al. (2020). Global warming offsets the ecophysiological stress of ocean acidification on temperate crustose coralline algae. Mar. Pollut. Bull. 157:111324. doi: 10.1016/j.marpolbul.2020.111324
Koch, M., Bowes, G., Ross, C., and Zhang, X.-H. (2013). Climate change and ocean acidification effects on seagrasses and marine macroalgae. Glob. Chang. Biol. 19, 103–132. doi: 10.1111/j.1365-2486.2012.02791.x
Kornder, N. A., Riegl, B. M., and Figueiredo, J. (2018). Thresholds and drivers of coral calcification responses to climate change. Glob. Chang. Biol. 24, 5084–5095. doi: 10.1111/gcb.14431
Kroeker, K. J., Bell, L. E., Donham, E. M., Hoshijima, U., Lummis, S., Toy, J. A., et al. (2020). Ecological change in dynamic environments: accounting for temporal environmental variability in studies of ocean change biology. Glob. Chang. Biol. 26, 54–67. doi: 10.1111/gcb.14868
Kroeker, K. J., Kordas, R. L., Crim, R., Hendriks, I. E., Ramajo, L., Singh, G. S., et al. (2013). Impacts of ocean acidification on marine organisms: quantifying sensitivities and interaction with warming. Glob. Chang. Biol. 19, 1884–1896. doi: 10.1111/gcb.12179
Lough, J. (1998). Sea Surface Temperatures on the Great Barrier Reef: A Contribution to the Study of Coral Bleaching. Townsville QLD: Great Barrier Reef Marine Park Authority.
Lough, J. M., Anderson, K. D., and Hughes, T. P. (2018). Increasing thermal stress for tropical coral reefs: 1871–2017. Sci. Rep. 8:6079. doi: 10.1038/s41598-018-24530-9
Marsh, J. A. Jr. (1970). Primary productivity of reef-building calcareous red algae. Ecology 51, 255–263. doi: 10.2307/1933661
Martin, S., and Gattuso, J.-P. (2009). Response of Mediterranean coralline algae to ocean acidification and elevated temperature. Glob. Chang. Biol. 15, 2089–2100. doi: 10.1111/j.1365-2486.2009.01874.x
Mathur, S., Agrawal, D., and Jajoo, A. (2014). Photosynthesis: response to high temperature stress. J. Photochem. Photobiol. B 137, 116–126. doi: 10.1016/j.jphotobiol.2014.01.010
McCoy, S. J., and Widdicombe, S. (2019). Thermal plasticity is independent of environmental history in an intertidal seaweed. Ecol. Evol. 9, 13402–13412. doi: 10.1002/ece3.5796
McNicholl, C., Koch, M. S., Swarzenski, P. W., Oberhaensli, F. R., Taylor, A., Batista, M. G., et al. (2020). Ocean acidification effects on calcification and dissolution in tropical reef macroalgae. Coral Reefs 39, 1635–1647. doi: 10.1007/s00338-020-01991-x
Noisette, F., Egilsdottir, H., Davoult, D., and Martin, S. (2013). Physiological responses of three temperate coralline algae from contrasting habitats to near-future ocean acidification. J. Exp. Mar. Biol. Ecol. 448, 179–187. doi: 10.1016/j.jembe.2013.07.006
Oliver, E. C. J., Donat, M. G., Burrows, M. T., Moore, P. J., Smale, D. A., Alexander, L. V., et al. (2018). Longer and more frequent marine heatwaves over the past century. Nat. Commun. 9:1324. doi: 10.1038/s41467-018-03732-9
Ordoñez-Alvarez, A., Wangpraseurt, D., Lyndby, N. H., Kühl, M., and Diaz-Pulido, G. (2018). Elevated CO2 leads to enhanced photosynthesis but decreased growth in early life stages of reef building coralline algae. Front. Mar. Sci. 5:495. doi: 10.3389/fmars.2018.00495
Page, T. M., and Diaz-Pulido, G. (2020). Plasticity of adult coralline algae to prolonged increased temperature and pCO2 exposure but reduced survival in their first generation. PLoS One 15:e0235125. doi: 10.1371/journal.pone.0235125
Pakker, H., Breeman, A. M., Prud’homme van Reine, W. F., and van den Hock, C. (1995). A comparative study of temperature responses of Caribbean seaweeds from different biogeographic groups. J. Phycol. 31, 499–507. doi: 10.1111/j.1529-8817.1995.tb02543.x
Pinheiro, J., Bates, D., DebRoy, S., Sarkar, D., and Team, R. C. (2013). nlme: Linear and Nonlinear Mixed Effects Models. R Package Version 3 111.
Poloczanska, E. S., Brown, C. J., Sydeman, W. J., Kiessling, W., Schoeman, D. S., Moore, P. J., et al. (2013). Global imprint of climate change on marine life. Nat. Clim. Chang. 3, 919–925. doi: 10.1038/nclimate1958
Prada, F., Caroselli, E., Mengoli, S., Brizi, L., Fantazzini, P., Capaccioni, B., et al. (2017). Ocean warming and acidification synergistically increase coral mortality. Sci. Rep. 7:40842. doi: 10.1038/srep40842
Rendina, F., Bouchet, P. J., Appolloni, L., Russo, G. F., Sandulli, R., Kolzenburg, R., et al. (2019). Physiological response of the coralline alga Corallina officinalis L. to both predicted long-term increases in temperature and short-term heatwave events. Mar. Environ. Res. 150:104764. doi: 10.1016/j.marenvres.2019.104764
Scherner, F., Pereira, C. M., Duarte, G., Horta, P. A., E Castro, C. B., Barufi, J. B., et al. (2016). Effects of ocean acidification and temperature increases on the photosynthesis of tropical reef calcified macroalgae. PLoS One 11:e0154844. doi: 10.1371/journal.pone.0154844
Schneider, K., and Erez, J. (2006). The effect of carbonate chemistry on calcification and photosynthesis in the hermatypic coral Acropora eurystoma. Limnol. Oceanogr. 51, 1284–1293. doi: 10.4319/lo.2006.51.3.1284
Schubert, N., Salazar, V. W., Rich, W. A., Vivanco Bercovich, M., Almeida Saá, A. C., Fadigas, S. D., et al. (2019). Rhodolith primary and carbonate production in a changing ocean: the interplay of warming and nutrients. Sci. Total Environ. 676, 455–468. doi: 10.1016/j.scitotenv.2019.04.280
Semesi, I. S., Kangwe, J., and Björk, M. (2009). Alterations in seawater pH and CO2 affect calcification and photosynthesis in the tropical coralline alga, Hydrolithon sp. (Rhodophyta). Estuar. Coast. Shelf Sci. 84, 337–341. doi: 10.1016/j.ecss.2009.03.038
Smale, D. A., Wernberg, T., Oliver, E. C. J., Thomsen, M., Harvey, B. P., Straub, S. C., et al. (2019). Marine heatwaves threaten global biodiversity and the provision of ecosystem services. Nat. Clim. Chang. 9, 306–312. doi: 10.1038/s41558-019-0412-1
Smith, S. V., and Key, G. S. (1975). Carbon dioxide and metabolism in marine environments. Limnol. Oceanogr. 20, 493–495. doi: 10.4319/lo.1975.20.3.0493
Somero, G. N. (2010). The physiology of climate change: how potentials for acclimatization and genetic adaptation will determine ‘winners’ and ‘losers’. J. Exp. Biol. 213, 912–920. doi: 10.1242/jeb.037473
Vásquez-Elizondo, R. M., and Enríquez, S. (2016). Coralline algal physiology is more adversely affected by elevated temperature than reduced pH. Sci. Rep. 6:19030. doi: 10.1038/srep19030
Keywords: coralline algae, global change, acclimation history, thermal stress, net photosynthesis, ocean acidification, ocean warming
Citation: Page TM, Bergstrom E and Diaz-Pulido G (2021) Acclimation History of Elevated Temperature Reduces the Tolerance of Coralline Algae to Additional Acute Thermal Stress. Front. Mar. Sci. 8:660196. doi: 10.3389/fmars.2021.660196
Received: 29 January 2021; Accepted: 14 April 2021;
Published: 07 May 2021.
Edited by:
Peng Jin, University of Guangzhou, ChinaReviewed by:
Federica Ragazzola, University of Portsmouth, United KingdomDan Smale, Marine Biological Association of the United Kingdom, United Kingdom
Copyright © 2021 Page, Bergstrom and Diaz-Pulido. This is an open-access article distributed under the terms of the Creative Commons Attribution License (CC BY). The use, distribution or reproduction in other forums is permitted, provided the original author(s) and the copyright owner(s) are credited and that the original publication in this journal is cited, in accordance with accepted academic practice. No use, distribution or reproduction is permitted which does not comply with these terms.
*Correspondence: Tessa M. Page, tessa.page@griffithuni.edu.au; Guillermo Diaz-Pulido, g.diaz-pulido@griffith.edu.au