- 1Department of Environmental Biology and Fisheries Science, National Taiwan Ocean University, Keelung, Taiwan
- 2College of Earth and Ocean Sciences, Xiamen University, Xiamen, China
- 3Fisheries Resources Monitoring Center of Fujian Province, Fuzhou, China
- 4Department of Marine Biology, Xiamen Ocean Vocational College, Xiamen, China
Microplastics (MPs) and heavy metals are two major types of pollutants that interact with each other, but they are poorly understood. Polystyrene (PS) is one type of MPs that is often detected in aquatic environments. In this study, we examined the adsorption capacity and release rate of heavy metals with respect to different particle sizes of PS, heavy metals, initial heavy metal concentrations, and salinities. Virgin (new) PS with diameters of 20, 50, 130, and 250 μm was used in this study, and four heavy metals (lead, cadmium, copper, and zinc) were used. The results showed that larger PS particle sizes adsorbed more heavy metals even though it took longer to achieve equilibrium adsorption. An increase in heavy metal concentration caused the adsorption capacity (μg g–1) of PS particles to also increase, but the adsorption rate (%) decreased. Increased salinity of the heavy metal solution resulted in a slower adsorption time and a lower adsorption capacity and release rate from the surface of PS particles. Different heavy metals also had different adsorption capacities. Pb was consistently more highly adsorbed by MPs, followed by Cu, Zn, and Cd. Larger PS sizes released heavy metals faster than smaller PS sizes, and the amounts of heavy metals released were higher. The heavy metal with the highest release rate was Cd, followed by Pb, Cu, and Zn. Finally, our findings highlight the interactions between PS and heavy metals and strongly support that PS particles can act as vectors for heavy metals in aquatic systems.
Introduction
The term microplastic (MP), defined as microscopic plastic particles with a diameter of approximately 20 μm, was first used in 2004 (Thompson et al., 2004). MPs are derived from plastics that break down into small particles. MP sources are divided into two types, namely, primary MPs, derived from cosmetic products such as body scrubs, and secondary MPs, derived from plastics that have fragmented and been reduced in size (GESAMP Joint Group of Experts on the Scientific Aspects of Marine Environmental Protection, 2015). MPs are found in many coastal and marine areas. In surface waters, the maximum number of MPs reached 9,200 particles m–3 (Desforges et al., 2014), and in sediment, the maximum number reached 100,000 items m–3 (Wright et al., 2013). Hidalgo-Ruz et al. (2012) stated that in sediments and water, MPs are found in the form of fragments, fibers, and films. The entry of MPs into the bodies of organisms can damage the function of organs such as the digestive tract, reduce the growth rate, inhibit enzyme production, reduce the levels of steroid hormones, affect reproduction and cause exposure to plastic additives with even greater toxicity (Wright et al., 2013; Xu et al., 2018).
Heavy metals can be divided into two categories: toxic and essential. Toxic metals such as Ni, Cd, Pb, and Hg are harmful to organisms even at low concentrations, but essential metals such as Zn, Cu, Mn, Se, and Fe can also be toxic if they accumulate at high concentrations in organisms (Zhang et al., 2014; Gu et al., 2015). Heavy metal pollution comes from the disposal of industrial waste, such as wastes from the paint industry, agriculture, and fuel combustion (Brennecke et al., 2016; Yousefzadeh et al., 2018). Various studies have been conducted on heavy metals in the environment due to pollution, which continues to increase due to anthropogenic activity (Edmunds et al., 2003; Marengo et al., 2016). Heavy metals can also be adsorbed by objects such as MPs (Holmes et al., 2014; Gao et al., 2019; Li et al., 2020).
Although they are very small, MPs are very difficult to degrade because of their physical and chemical properties, so they persist and accumulate in the ocean (Lu et al., 2016; Pedà et al., 2016). In addition, MPs have the potential to carry other pollutants, such as heavy metals, to ecosystems in both seawater (Ashton et al., 2010; Holmes et al., 2012; Brennecke et al., 2016) and freshwater (Holmes et al., 2012). Holmes et al. (2014) reported that the rate of adsorption of heavy metals by MPs in river water was higher than that in seawater. The heavy metals lead (Pb), copper (Cu), zinc (Zn), aluminum (Al), iron (Fe), manganese (Mn), and silver (Ag) were found in virgin (new) MP pellets, including polystyrene (PS) pellets, in similar and higher concentrations than in local sediments in SW England harbor waters (Ashton et al., 2010). MPs and heavy metals were very difficult to degrade in the environment and persist in organisms, causing bioaccumulation in the food chain and potentially reaching higher trophic levels (Rios et al., 2007; Galloway, 2015).
Interactions between MPs and heavy metals may occur via two pathways. First, heavy metals can be adsorbed by MPs, leading to adverse effects when the particles are eaten by organisms. Second, the adsorbed metals may be released into the water, contributing to exposure from direct contact between marine organisms and metal-contaminated water.
Polystyrene is known for its insulating characteristics and low weight, as it is made from the petroleum-based plastic monomer styrene (vinyl benzene). PS is synthesized for a variety of applications, such as packaging. In 2011, approximately 32.7 million tons of PS plastic was produced globally (Lithner et al., 2011). Overproduction, use, and disposal of PS can cause various harms to the environment. PS contains a carcinogenic substance, styrene monomer, which can pose a serious threat to aquatic organisms. PS is one type of MPs that is often detected in aquatic environments (Hidalgo-Ruz et al., 2012; Sjollema et al., 2016; Andrady, 2017; Gambardella et al., 2017). PS particles measuring 20–50 μm have caused severe damage to a wide variety of aquatic species, including crustaceans, fish, microalgae, sea urchins, and mussels (Mattsson et al., 2015; Yooeun et al., 2018). PS is mobile, spreads across ocean boundaries via currents, and is an invasive species because of its light weight (Brandts et al., 2018; Jin et al., 2018). PS particles generated from land activities are distributed and accumulate in aquatic ecosystems through various processes (Kik et al., 2020). Aquatic ecosystems include not only marine waters but also fresh and brackish waters. Each of these water types has a different salinity value. Therefore, we speculate that differences in salinity are thought to influence the interactions between PS particles and heavy metals in these waters.
Although there have been many studies on heavy metals and MPs, it is very rare to study the interaction of the two, especially for PS. Some studies only focus on the absorption of heavy metals in MPs but did not analyze heavy metals that were adsorbed in MPs which can be released and polluted the aquatic environment. The purpose of this study was to examine the adsorption capacity and release rate of heavy metals (Pb, Zn, Cd, and Cu) for different PS particle sizes, initial heavy metal concentrations, and salinities. This study would provide an overview of PS particles as carriers for heavy metal pollutants in aquatic environments, both fresh, brackish, and marine waters with various factors that influence them.
Materials and Methods
Materials
Virgin (new) PS with diameters of 20, 50, 130, and 250 μm was purchased from Tersulan Chemical Co., Ltd. (Guangdong, China). To ensure the correct size of the PS particles, we made observations under a microscope. The sizes after checking for PS 20, 50, 130, and 250 μm particles were 21.224, 50.328, 130.876, and 250.773 μm, respectively. We chose PS because this type of plastic is often found in the environment, especially in aquatic environments (Hidalgo-Ruz et al., 2012; Sjollema et al., 2016; Andrady, 2017; Gambardella et al., 2017).
The four types of heavy metals used in this study were lead (Pb), copper (Cu), cadmium (Cd), and zinc (Zn), which were purchased from Merck KGaA (Darmstadt, Germany). The heavy metal test solution was prepared by dissolving heavy metals in 1 L of distilled water to make a 1,000 mg L–1 stock solution, which was then diluted to 1, 2, 5, and 10 mg L–1. To prepare a stock solution of 1,000 mg L–1 heavy metal, we first calculated how much heavy metal powder was needed and dissolved it in distilled water. These four concentrations were used to test the effect of differences in heavy metal concentrations on heavy metal adsorption. Tests were also carried out under different salinity conditions. Heavy metal test solutions were also prepared by dissolving heavy metals in artificial seawater with salinities of 0, 15, and 30‰. The use of an artificial seawater to ensure that the heavy metals dissolved in the water remain evenly mixed so as not to interfere with the adsorption process of heavy metals in PS particles.
Adsorption Capacity Experiment
This experiment investigated how the adsorption capacity of heavy metals on PS particle was influenced by different sizes of particle, different heavy metal concentrations, and different salinities.
A PS sample weighing 0.05 g was placed in a beaker, 10 mL of heavy metals was added, and the mixture was stirred on a magnetic stirrer with a fixed stirring speed of 180 rpm. Different exposure times (5, 15, 30, 60, 75, and 90 min) between the PS and heavy metal were used, and the test continued until it reached the equilibrium adsorption point. In the equilibrium adsorption point, the entire surface of the PS particles has reached its maximum capacity to adsorb heavy metals so that there is no significant change in adsorption value after that time. PS particles were filtered using filter paper with a size of 0.45 μm (Advantec, Toyo Roshi Kaisha, Ltd., Japan). Filtered water was put in 50 mL glass tubes. Each test was repeated three times. The test was performed under different initial heavy metal concentrations (1, 2, 5, and 10 mg L–1) and salinities (0, 15, and 30‰). The concentrations of metals in the filtrate were determined using a flame atomic adsorption spectrophotometer (SpeactAA 240-FS, VARIAN, Palo Alto, CA, United States). The adsorption percentage was calculated based on the following Eq. 1 (Oz et al., 2019):
where %ads is the adsorption rate; [A]ta is the concentration of the heavy metal remaining in solution after time t; and [A]0 is the concentration of the heavy metal at time 0.
Release Efficiency Experiment
This experiment was conducted to analyze the release efficiency (μg g–1) of heavy metals from PS particles with respect to different sizes of particle and different salinities.
The measured heavy metal adsorption values for the PS particles were used as the standard initial values for heavy metal release. In this test, we used 0.1 μg g–1 as the heavy metal amount adsorbed by PS particles. PS particles that had adsorbed 0.1 μg g–1 heavy metals were put into a glass beaker and washed using 10 mL distilled water (salinity 0‰) to analyze the heavy metal release efficiency (μg g–1) from the surface of the particles. The test was also carried out under different salinity conditions (15 and 30‰) using artificial seawater. The heavy metal content in the filtered water was analyzed after different exposure times (5, 15, 30, 60, 120, 150, 180, and 240 min). Three replicates were carried out for each test. The filtrate was put in 50 mL glass tubes. The heavy metal concentrations in the filtered water were analyzed by a flame atomic adsorption spectrophotometer (SpeactAA 240-FS, VARIAN, Palo Alto, CA, United States). The release rate was calculated based on Eq. 2:
where %Rls is the release rate; [R]tr is the concentration of the heavy metal remaining in the solution after time t; and [R]0 is the concentration of the heavy metal at time 0.
Data Analysis
All adsorption and release data were assessed by two-way analysis of variance (ANOVA). If the results showed significant differences (p < 0.05), then Duncan’s multiple range test was used to analyze significant differences between all treatments. In this study, we tested for significant differences between the adsorption capacity (μg g–1) and adsorption rate (%) of heavy metals with respect to different sizes of PS, different salinities, and different types of heavy metals. Significant differences in the release efficiency (μg g–1) and release rate (%) of heavy metals with respect to different sizes of PS and different salinities were also assessed. The linear relationships among the adsorption and release rates of each heavy metal in this study under all conditions were tested using a general linear model and Statistica computer software. For all tests, statistical significance was considered at the p < 0.05 level.
Results
Heavy Metal Adsorption Capacity
The adsorption capacity of heavy metals on the PS surface increased with increasing PS size. PS with a size of 250 μm adsorbed most of heavy metals, followed by PS with sizes of 130, 50, and 20 μm. Increase in heavy metal concentration caused the adsorption capacity (μg g–1) of PS particles to also increase but the adsorption rate (%) to decrease. A higher salinity of the solution containing heavy metals resulted in slower adsorption and a lower adsorption rate.
Statistical analysis indicated that the initial heavy metal concentration, particle size, and salinity had a significant effect on the equilibrium adsorption of all heavy metals in this study. The relationships between the adsorption rate (Ads. Rate) for each heavy metal in this study and the initial heavy metal concentration (C), particle size (Sz) and salinity (S) are as follows:
Ads. Rate Zn = 13.791 − 8.481 C + 11.607 Sz − 4.641 S (R2 = 0.836)
Ads. Rate Pb = 12.390 − 8.909 C + 9.962 Sz − 2.652 S (R2 = 0.808)
Ads. Rate Cd = 14.140 − 10.332 C + 14.572 Sz − 3.615 S (R2 = 0.882)
Ads. Rate Cu = 11.422 − 8.672 C + 9.183 Sz − 2.844 S (R2 = 0.792)
The size of the PS particles affected the time to adsorption equilibrium. At a salinity of 0‰, PS with sizes of 20 and 50 μm adsorbed increasing amounts of heavy metals until reaching the equilibrium adsorption point after 45 min, and then the adsorption curve flattened. For the larger particles (130 and 250 μm), the rate of adsorption of heavy metals increased until 60 min and then flattened out. A similar adsorption duration was found for all types of heavy metals except Zn. For all PS sizes, Zn reached an equilibrium absorption point at 45 min of contact duration (Figure 1).
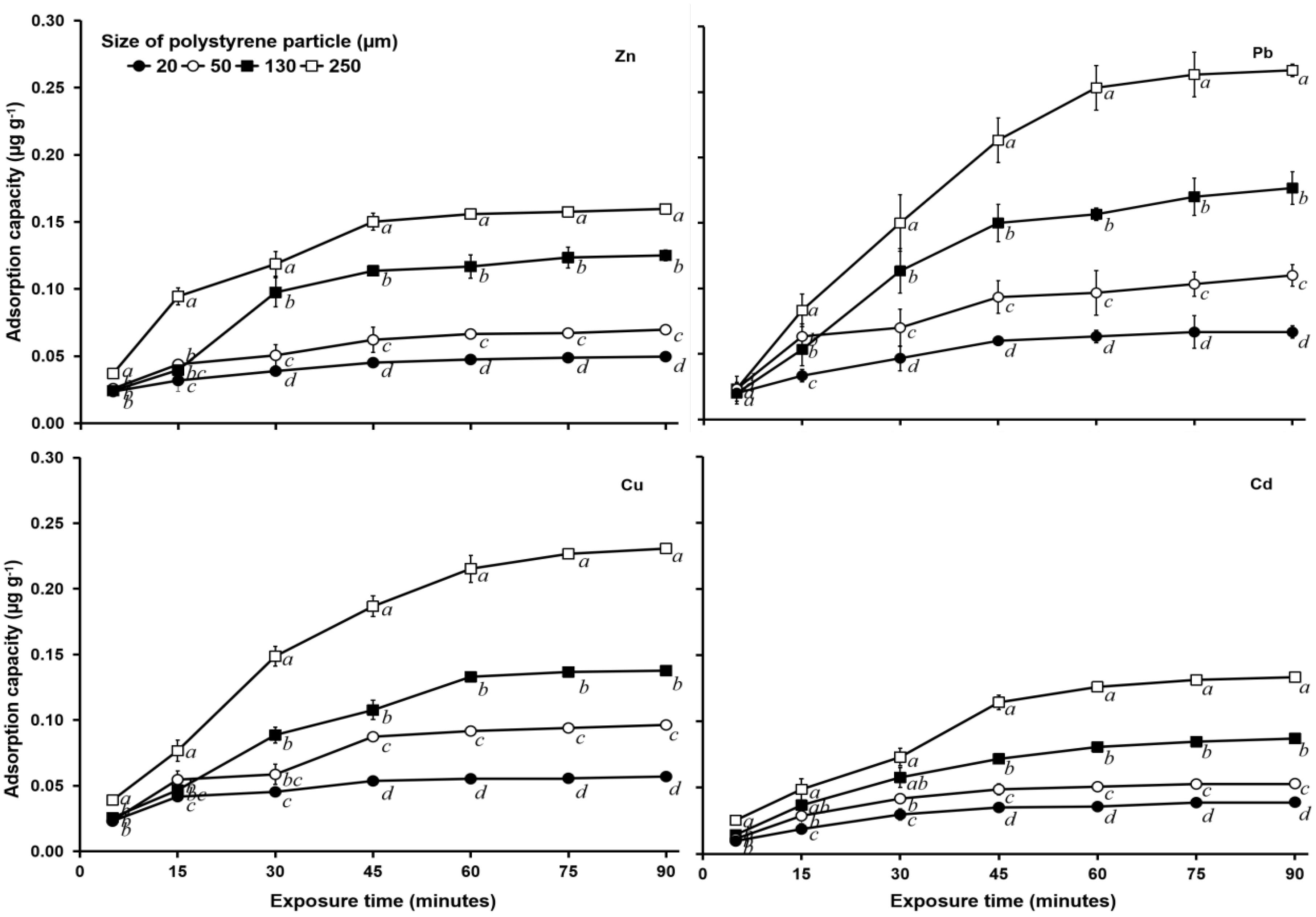
Figure 1. Heavy metal (Zn, Pb, Cu, and Cd) adsorption capacity (μg g–1) of PS of different sizes (20, 50, 130, and 250 μm) with a 1 mg L–1 heavy metal concentration and 0‰ salinity. Data for the adsorption capacity (μg g–1) of the same heavy metal with different letters (a, b, c, and d) are significantly different (p < 0.05) between each PS size.
Figure 1 shows that the adsorption of the heavy metals. Zn and Cd in PS particles with a size of 250 μm was significantly higher (p < 0.05) than that of the other three sizes at a 5-min exposure time. At 60 min, the adsorption capacities of all types of heavy metals at all PS sizes were significantly different (p < 0.05). This difference persisted up to 90 min. At equilibrium point, the Zn adsorption of 20 μm PS was 0.048 ± 0.005 μg g–1, that of 50 μm was 0.067 ± 0.003 μg g–1, that of 130 μm was 0.125 ± 0.019 μg g–1, and that of 250 μm was 0.170 ± 0.021 μg g–1.
The optimum adsorption rate (%) was found at a heavy metal concentration of 1 mg L–1. The adsorption rates of Zn and Cd at an initial concentration of 1 mg L–1 by all PS particle sizes differed significantly (p < 0.05) from those at 2 mg L–1, whereas the adsorption rates of Pb and Cu at an initial concentration of 1 mg L–1 by 20 μm particles were not significantly different (p > 0.05) from those at 2 mg L–1. The adsorption rates of Pb and Cd at 2 mg L–1 by 250 and 130 μm particles were not significantly different (p > 0.05) from those at 5 mg L–1, whereas the adsorption rates by 50 and 30 μm PS particles were significantly different (p < 0.05). For Zn, the adsorption rate by all sizes of PS particles at a concentration of 2 mg L–1 was not significantly different (p > 0.05) from that at a concentration of 5 mg L–1. For Cu, the adsorption rate by all particle sizes of PS at an initial concentration of 2 mg L–1 differed significantly (p < 0.05) from that at 5 mg L–1 except for 130 μm particles. The adsorption rate of all heavy metals at an initial concentration of 5 mg L–1 by all particle sizes differed significantly (p < 0.05) from that at 10 mg L–1. These results indicated that the initial concentration was also an important factor affecting the adsorption of heavy metals by MP particles.
In the test with an initial concentration of 1 mg L–1 and salinity of 15‰, adsorption equilibrium at all particle PS sizes was achieved at 90 min of heavy metal exposure (Figure 2), whereas at a salinity of 30‰, adsorption equilibrium was achieved at 120 min (Figure 3). The equilibrium adsorption amount of Zn by 250 μm PS at a salinity of 0‰ was 0.156 μg g–1, while that at a salinity of 15‰ was 0.131 μg g–1, and that at a salinity of 30‰ was 0.116 μg g–1.
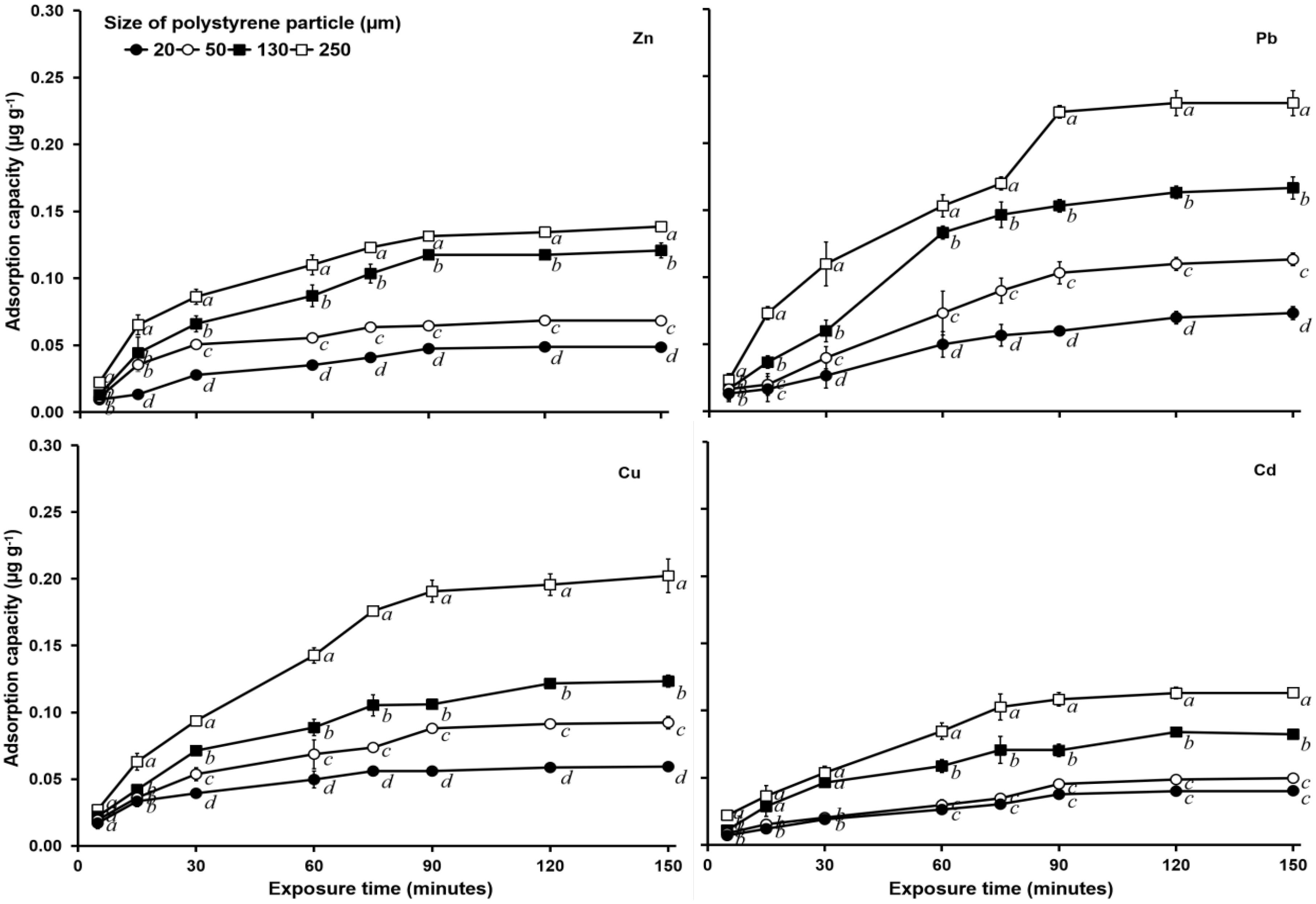
Figure 2. Heavy metal (Zn, Pb, Cu, and Cd) adsorption capacity (μg g–1) of PS of different sizes (20, 50, 130, and 250 μm) with a 1 mg L–1 heavy metal concentration and salinity of 15‰. See Figure 1 for statistical information.
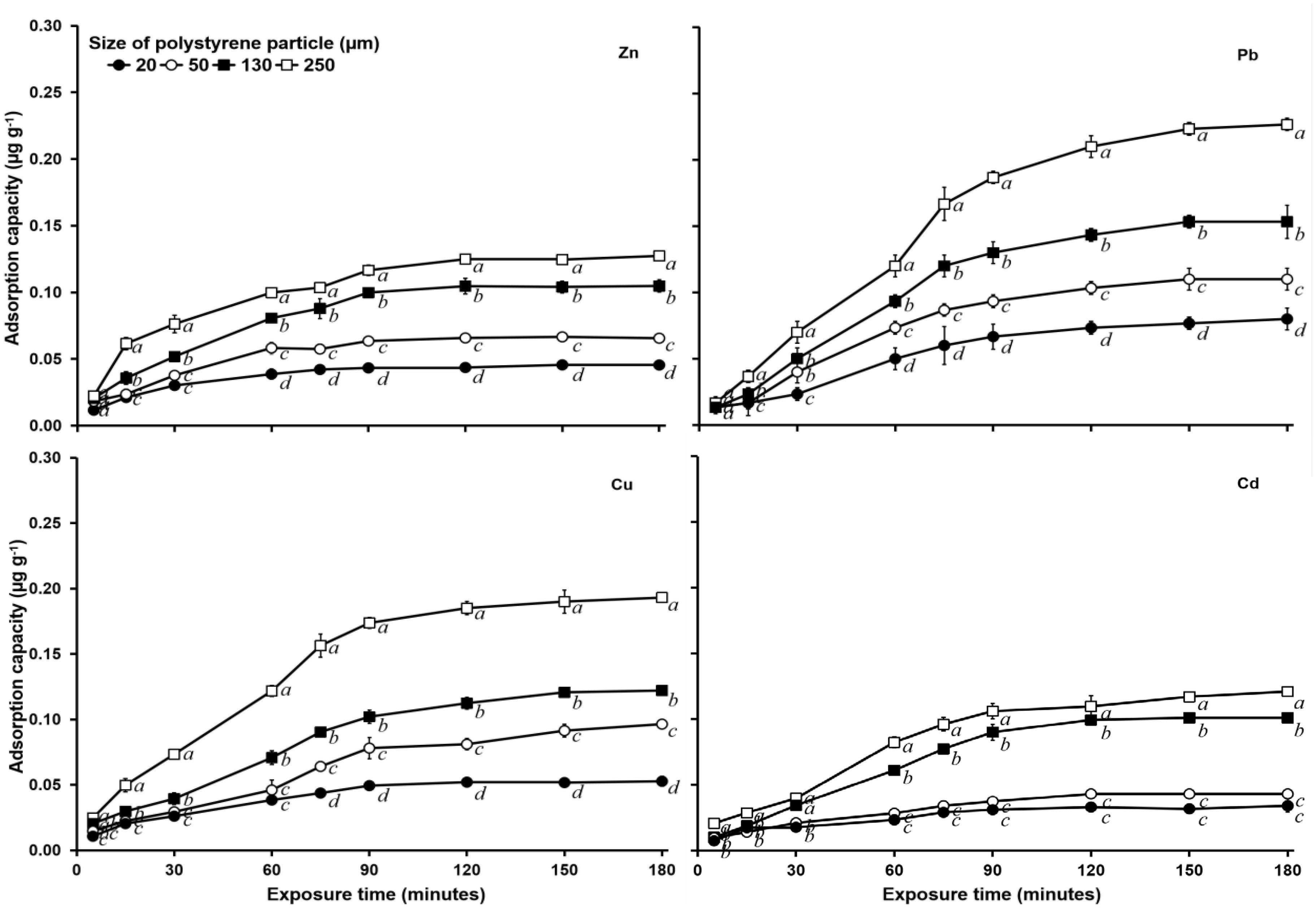
Figure 3. Heavy metal (Zn, Pb, Cu, and Cd) adsorption capacity (μg g–1) of PS of different sizes (20, 50, 130, and 250 μm) with a 1 mg L–1 heavy metal concentration and salinity of 30‰. See Figure 1 for statistical information.
The adsorption rate (%) of all heavy metals at a salinity of 0‰ was significantly higher (p < 0.05) than that at a salinity of 15‰, and the adsorption rate of heavy metals at a salinity of 15‰ was significantly higher (p < 0.05) than that at a salinity of 30‰ (Figure 4).
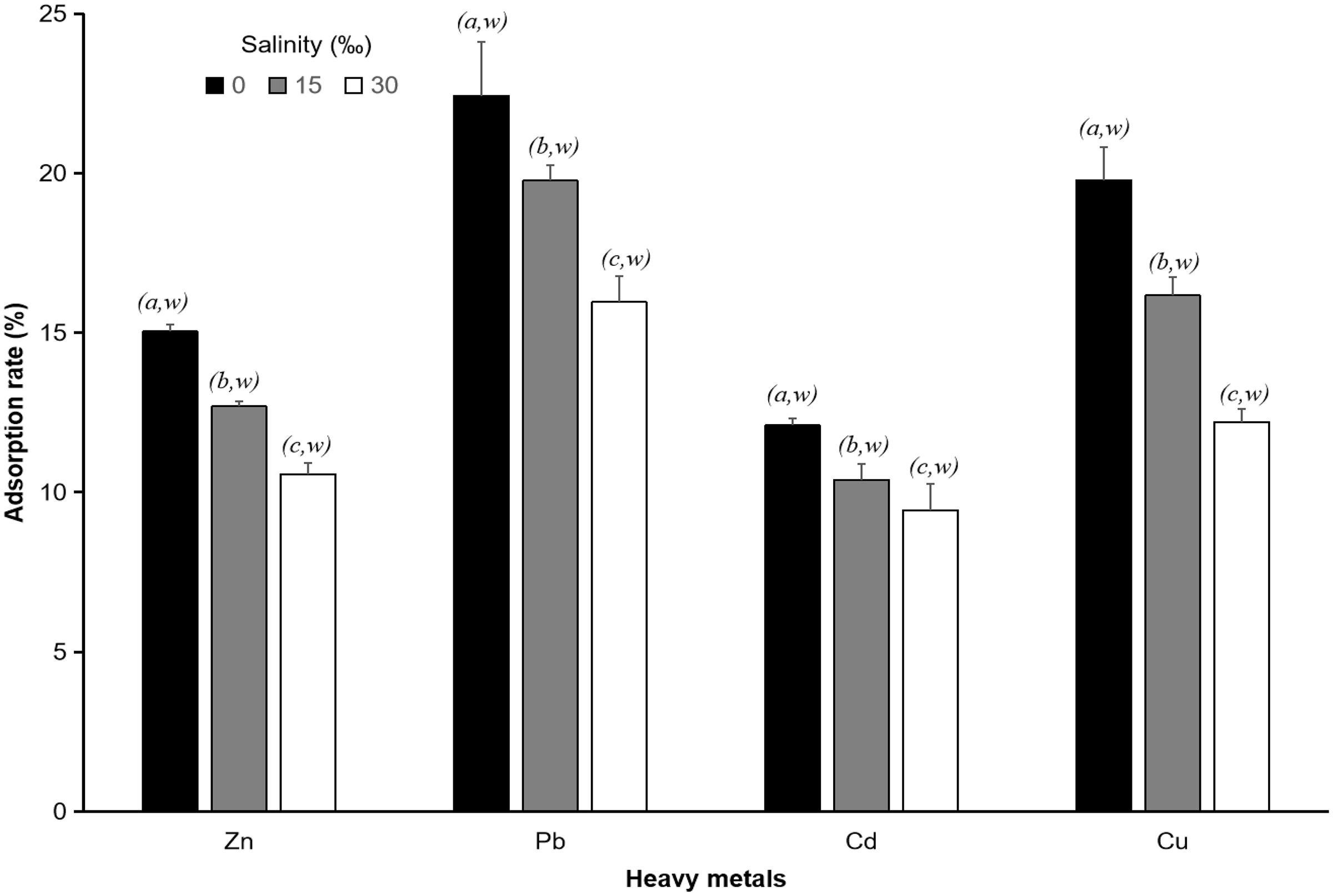
Figure 4. Heavy metal (Zn, Pb, Cu, and Cd) equilibrium adsorption rate of 250 μm PS with a 1 mg L–1 heavy metal concentration and different salinities (0, 15, 30‰). Data for the adsorption rate (%) of the same heavy metal with different letters (a, b, c, and d) are significantly different (p < 0.05) between each heavy metal and each salinity.
In all experiments with different salinities, the adsorption rate of heavy metals differed significantly (p < 0.05) between all types of heavy metals in this study. The highest adsorption rate was found for Pb, followed by Cu, Zn, and Cd. At a salinity of 0‰, the equilibrium adsorption rate of Pb by 250 μm PS particles was 22.41 ± 1.69%, that of Cu was 19.77 ± 1.02%, that of Zn was 15.03 ± 0.21%, and that of Cd was 12.09 ± 0.22%. At a salinity of 15‰, the adsorption rate of Pb was 19.76 ± 0.46%, that of Cu was 16.16 ± 0.57%, that of Zn was 12.68 ± 0.15%, and that of Cd was 10.40 ± 0.50%, whereas at a salinity of 30‰, the adsorption rate of Pb was 15.95 ± 0.82%, that of Cu was 12.23 ± 0.41%, that of Zn was 10.56 ± 0.36%, and that of Cd was 9.43 ± 0.82%. These results indicated that salinity and the type of heavy metal have important roles in the adsorption of heavy metals on the surface of PS particles.
Heavy Metal Release
After obtaining adsorption results at all initial heavy metal concentrations, we assessed the adsorption capacity at an initial concentration of 5 mg L–1. From observations at various exposure times, an adsorption capacity of approximately 0.1 mg L–1 was found at approximately 15 min of exposure time for PS particles of size 130 and 250 μm, while for PS particles of 20 and 50 μm, this value was reached at 30 min of exposure time. We chose these exposure times so that the initial amount of heavy metals that was adsorbed on the surface of the PS particles was approximately the same.
The release of heavy metals varied based on the particle size and type of heavy metal. Larger particles released more heavy metals than smaller particles. PS with a size of 250 μm released most of heavy metals, followed by PS with sizes of 130, 50, and 20 μm. An increase in the salinity of the solution caused the value of heavy metal release to be lower and the time needed to achieve equilibrium to be longer.
Statistical analysis showed that the particle size and salinity had a significant effect on the release amount at equilibrium of all heavy metals in this study. The relationships between the rate of release (Rls. Rate) of each heavy metal in this study and the particle size (Sz) and salinity (S) are as follows:
Rls. Rate Zn = 90.918 + 3.416 Sz − 4.620 S (R2 = 0.785)
Rls. Rate Pb = 85.915 + 5.404 Sz − 5.263 S (R2 = 0.863)
Rls. Rate Cd = 68.082 + 6.707 Sz − 5.404 S (R2 = 0.892)
Rls. Rate Cu = 56.562 + 3.768 Sz − 5.407 S (R2 = 0.828)
At a salinity of 0‰, all particle sizes reached equilibrium release efficiencies at the same exposure time of 90 min, and then the release curve flattened out. For Pb, Cu, and Cd, the release efficiency of 250 μm PS particles at 90 min exposure differed significantly (p < 0.05) from those of the other three sizes. The release efficiency of 130 μm PS particles was not significantly different (p > 0.05) from that of 50 μm particles but was significantly different (p < 0.05) from that of 20 μm particles. Whereas, the release of Zn from 250 μm PS was not significantly different (p > 0.05) from that with 130 μm PS but was significantly different (p < 0.05) from those with 50 and 20 μm PS (Figure 5).
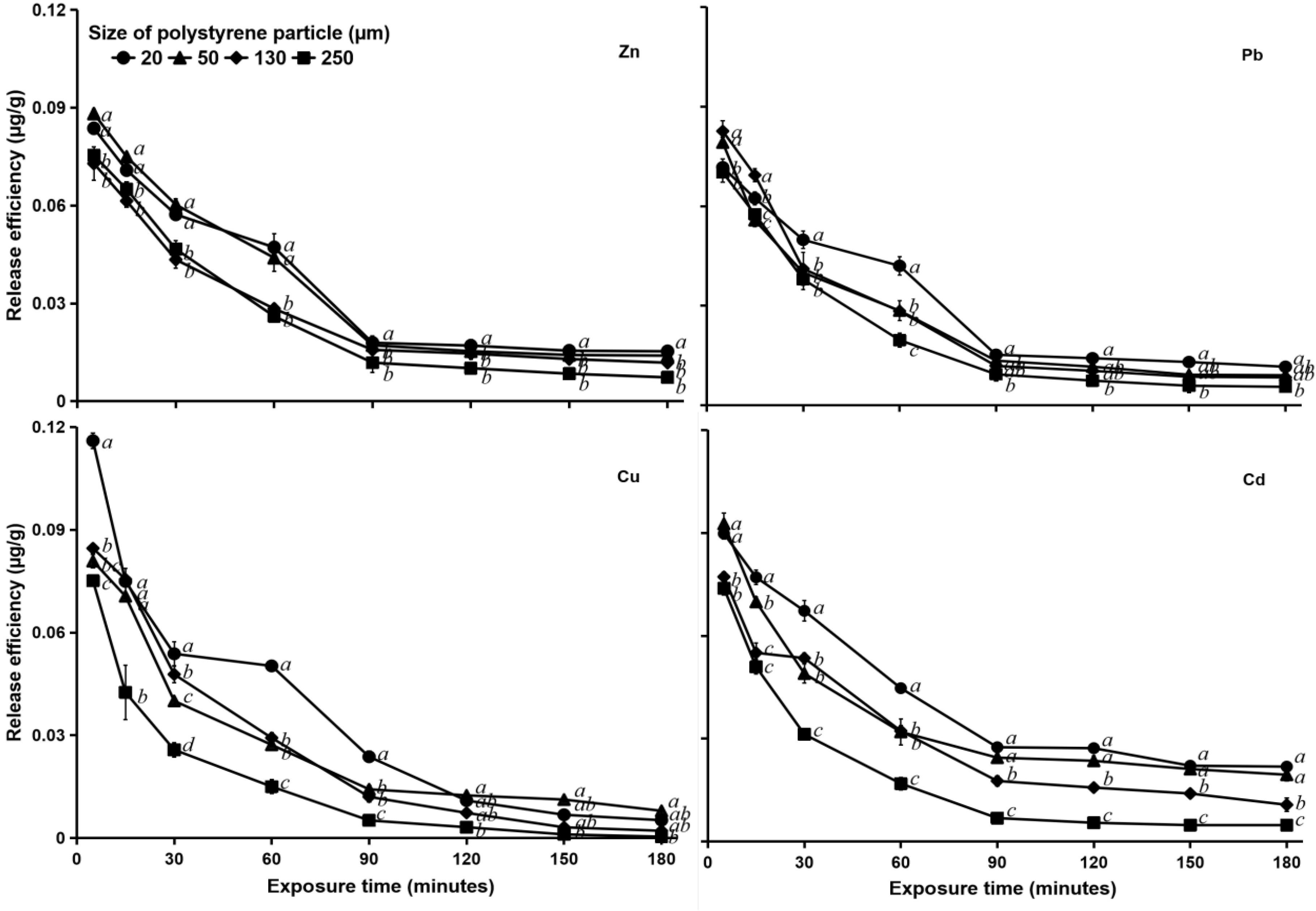
Figure 5. Heavy metal (Zn, Pb, Cu, and Cd) release efficiency (μg g–1) in MPs of different sizes (20, 50, 130, and 250 μm) in water with a salinity of 0‰. Data for the release efficiency (μg g–1) of the same heavy metal with different letters (a, b, c, and d) are significantly different (p < 0.05) between each PS size.
Figure 6 shows that at a salinity of 15‰, the release of heavy metals in particles of smaller size (20 and 50 μm) was slower than that at a salinity of 0‰. The larger particles (130 and 250 μm) achieved equilibrium release of Pb, Zn, and Cu at 90 min exposure, and then the release curves flattened, whereas the smaller PS particles (20 and 50 μm) reached equilibrium at 120 min exposure, and then the release curves flattened. At this salinity, the difference in release efficiency was more pronounced among the larger PS particles than the smaller ones. For all heavy metals, the release efficiencies from larger PS particles differed significantly (p < 0.05) from those of smaller particles at 90 min exposure until the last observation (Figure 6).
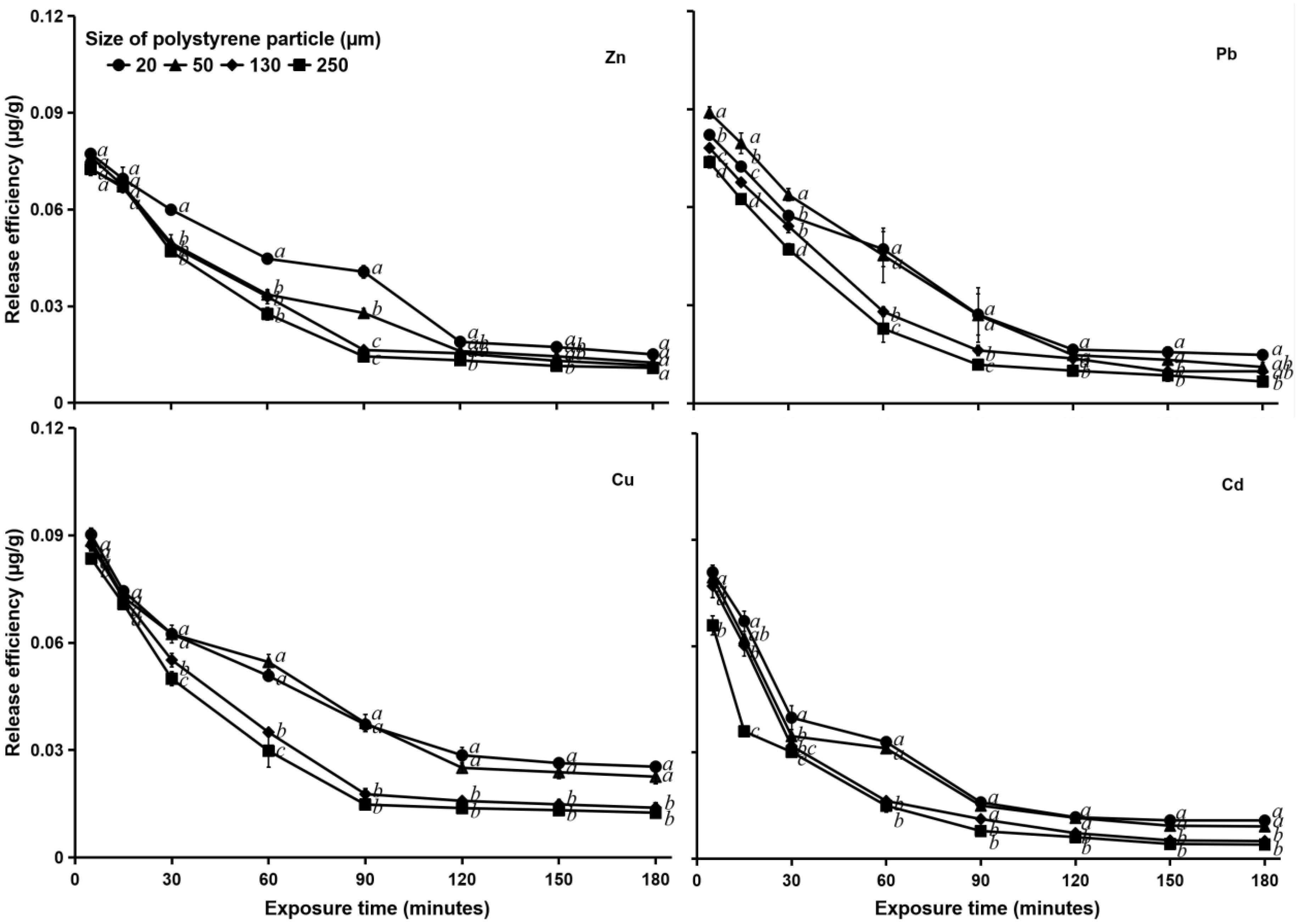
Figure 6. Heavy metal (Zn, Pb, Cu, and Cd) release efficiency (μg g–1) in different sizes (20, 50, 130, and 250 μm) of MPs in water with a salinity of 15‰. See Figure 5 for statistical information.
At a salinity of 30‰, for Pb, Zn, and Cu, the larger PS particles (130 and 250 μm) reached equilibrium sooner, released heavy metals more rapidly and had higher release values than the smaller PS particles (20 and 50 μm). Particles with sizes of 130 and 250 μm reached equilibrium release of Pb, Zn, and Cu at 120 min of exposure time, while particles with sizes of 20 and 50 μm reached equilibrium at 150 min exposure (Figure 7). This result indicated that salinity is an important factor in the release of heavy metals from the surface of PS particles.
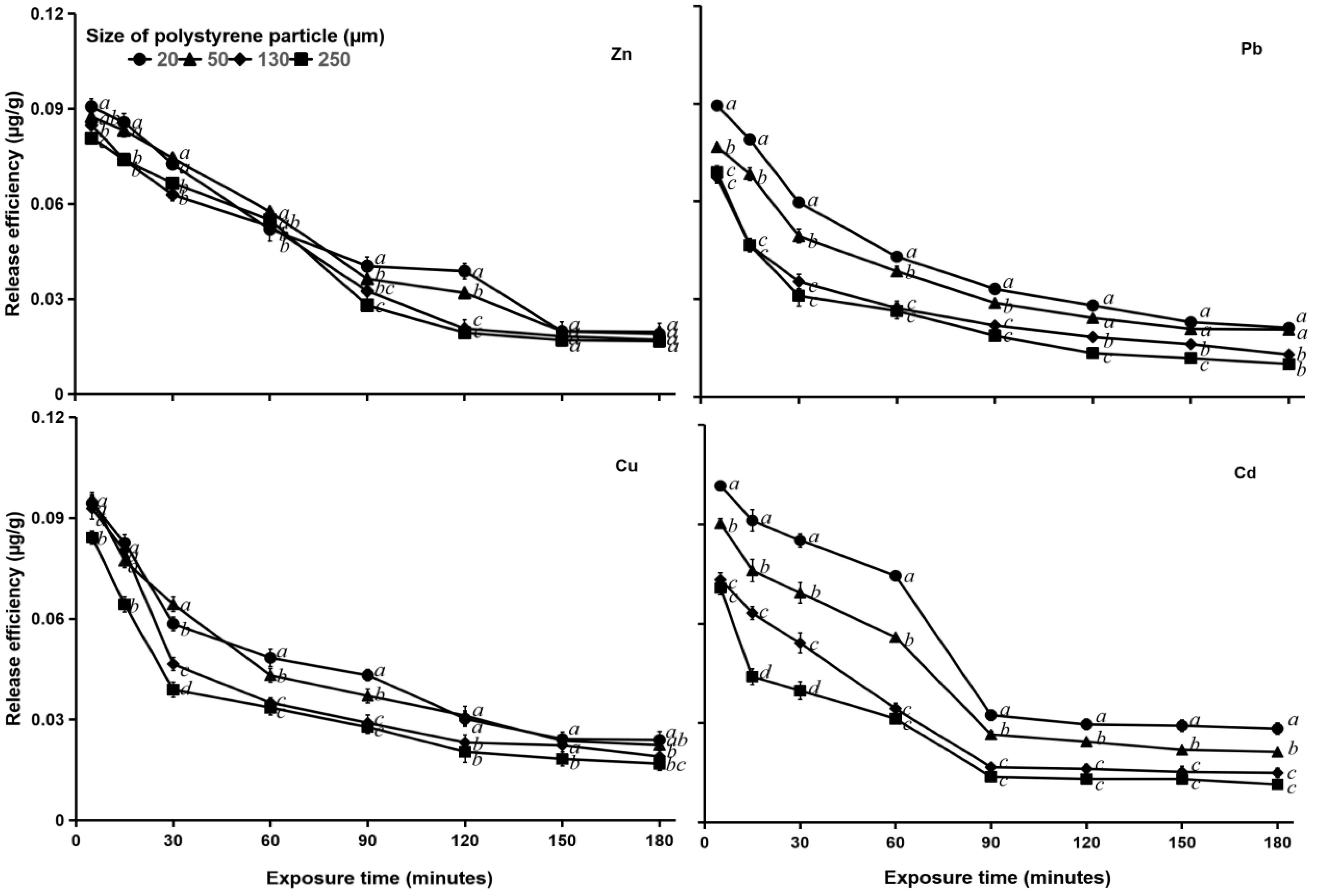
Figure 7. Heavy metal (Zn, Pb, Cu, and Cd) release efficiency (μg g–1) in MPs of different sizes (20, 50, 130, and 250 μm) in water with a salinity of 30‰. See Figure 5 for statistical information.
We compared the equilibrium release rates of 250 μm PS particles to clearly show the effect of salinity on the release rate of heavy metals. The results clearly show that for all heavy metals, the release rate at a salinity of 0‰ was significantly higher (p < 0.05) than those at the other two salinities. The release rate at a salinity of 15‰ was also significantly higher (p < 0.05) than that at a salinity of 30‰ (Figure 8).
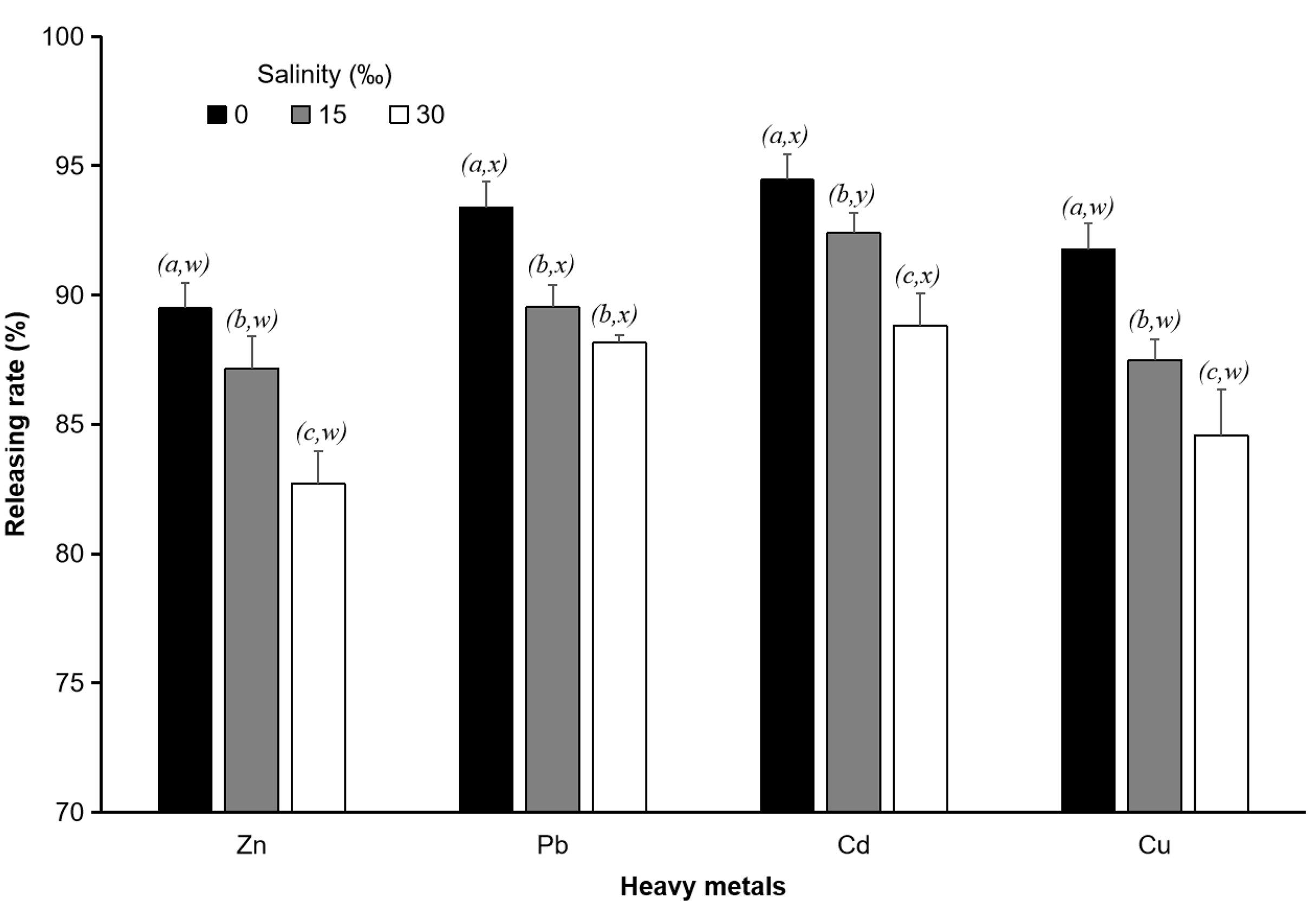
Figure 8. Heavy metal (Zn, Pb, Cu, and Cd) release rates in water with different salinities (0, 15, 30‰). Data for the release rate (%) of the same heavy metal with different letters (a, b, c, and d) are significantly different (p < 0.05) between each heavy metal and each salinity.
Figure 8 also shows that each heavy metal had a different level of release. In the experiment with the three salinity values, the highest level of heavy metal release was found for Cd, followed by Pb, Cu, and Zn. At a salinity of 0‰, the release rate of Zn was not significantly different (p > 0.05) from that of Cu, but both were significantly lower (p < 0.05) than those of Pb and Cd. The levels of Pb and Cd release did not differ significantly (p > 0.05). At equilibrium, the release rate of Cd was 94.45 ± 0.77%, that of Pb was 93.40 ± 1.41%, that of Cu was 91.75 ± 0.97%, and that of Zn was 89.48 ± 2.46%. At a salinity of 15‰, the release rate of h Zn was not significantly different (p > 0.05) from that of Cu. The release rate of Cd was significantly higher (p < 0.05) than that of Pb, and both were significantly higher (p < 0.05) than those of Zn and Cu (Figure 8). At equilibrium, with 250 μm PS particles, the release rate of Cd was 92.40 ± 0.78%, that of Pb was 89.52 ± 0.86%, that of Cu was 87.49 ± 0.82%, and that of Zn was 87.16 ± 1.25%. At a salinity of 30‰, the differences in the release rates among the four heavy metals were similar to those at a salinity of 0‰. The release rate of Cd was 88.81 ± 1.25%, that of Pb was 88.16 ± 0.29%, that of Cu was 84.57 ± 1.76% and that of Zn was 82.72 ± 1.25% (Figure 8).
Overall, the results of the three different salinity experiments above indicate that the heavy metal with the highest release rate was Cd, followed by Pb, Cu and Zn. These results indicated that the type of heavy metals also affects the release rate heavy metals.
Discussion
Plastic comes from synthetic materials and additives and is lightweight and durable; accordingly, the use of plastic continues to increase, but its nature makes it difficult to decompose in both terrestrial and aquatic environments (Law and Thompson, 2014). Galgani et al. (2015) reported that the plastic decomposition process takes place very slowly, and it can take up to hundreds of years for plastic to be degraded into MPs through various physical, chemical, and biological processes. MPs also have the potential to be carriers of other pollutants, such as heavy metals, to aquatic ecosystems.
This study is concerned with analyzing short-term interactions between micro PS particles and heavy metals with respect to different sizes of PS particles, salinities, heavy metals, and heavy metal concentrations. The interactions, in this case, were assessed by analyzing the adsorption and release efficiency of heavy metals on the surface of PS particles. Such assessment is important because it can show opportunities for MPs to act as pollution carriers, especially for heavy metals. Vieira et al. (2021) stated that there was an indication of a correlation between heavy metal content and particle MPs in oyster bodies. Heavy metal concentrations were found to be higher in oysters contaminated with MPs.
In this study, we used PS with relatively very small sizes, namely, 20, 50, 130, and 250 μm. Smaller MP particles would be more dangerous if they entered the bodies of organisms (Wang et al., 2019). MP particles with very small sizes can reduce the swimming performance of fish (Puga et al., 2016; Barboza et al., 2018a). Moreover, very small MP particles have a more adverse effect on organisms when combined with heavy metals (Barboza et al., 2018b).
Our results indicated that PS particle size is an important factor in the adsorption of heavy metals. Fred-Ahmadu et al. (2019) said that MP particle size is a factor that affects the adsorption of pollutants. PS particle sizes of 130 and 250 μm reached adsorption equilibrium after 60 min, whereas particle sizes of 20 and 50 μm reached adsorption equilibrium after 45 min. This finding is similar to Oz et al. (2019), who reported that heavy metals (Pb and Al) need 60 min to reach adsorption equilibrium on the surface of MP particles. Larger PS particles have a greater surface area for the adsorption of heavy metals, so the time needed to cover the surface is also longer than that needed for smaller particles. This result is supported by Wang et al. (2018), who stated that smaller MPs reached adsorption equilibrium with various contaminants more easily and quickly than larger particles.
In this study, PS particles with a diameter of 250 μm adsorbed most of heavy metals. Particles with a larger diameter will provide a greater adsorption area for heavy metals. This greater particle surface area will also cause the time to adsorption equilibrium to be longer. This relationship means that larger particles adsorb more heavy metals. This result was consistent with previous studies (Rochman et al., 2013; Holmes et al., 2014). These results are supported by the results of correlation analysis, which showed that the adsorption capacities for the four heavy metals in this study had a strong and positive correlation with the PS particle size. The larger the particle was, the higher the adsorption capacity for heavy metals (Figure 1). However, Gao et al. (2019) showed the opposite result: larger MPs adsorbed lower amounts of heavy metals due to the diminished specific surface area, resulting in a decrease in adsorption sites.
Generally, PS and polyethylene (PE) have higher sorption capacities than other plastic types under laboratory conditions (Khalid et al., 2020). Several studies have demonstrated the interaction between various types of MPs and heavy metals under various conditions (Table 1). Holmes et al. (2014) reported that virgin and beached PE pellets can adsorb approximately 0.19–2.73 μg g–1 Pb in filtered river water and seawater. PP and polyvinyl chloride (PVC) particles adsorbed approximately 1.3 μg g–1 more Pb than Cu (0.2 μg g–1) and Cd (0.10 μg g–1) in a seawater environment (Gao et al., 2019). The physical and chemical characteristics of plastics, for example, their small size, crystallinity, and surface area-to-volume ratio, help in the accumulation of chemical ions on plastics and can affect the fate of these contaminants in aquatic settings (Filella and Turner, 2018; Wang et al., 2018).
Differences in the initial heavy metal concentration also caused the adsorption capacity for the heavy metals to be different. A higher initial concentration of heavy metals caused the adsorption capacity to be higher but the adsorption rate to be lower. Hodson et al. (2017) reported that at relatively high initial concentrations of Zn (0.1–100 mg L–1), the adsorption capacities reached 236–7,171 mg g–1, much larger than those observed at low initial concentrations (Holmes et al., 2012; Turner and Holmes, 2015). In general, heavy metal adsorption by all particle sizes of PS at 1 mg L–1 was significantly different from that at 2 mg L–1, whereas the adsorption rate at an initial concentration of 2 mg L–1 was not significantly different from that at 5 mg L–1. Furthermore, the adsorption rate at an initial concentration of 5 mg L–1 was significantly higher than the adsorption rate at 10 mg L–1. The highest adsorption rate of heavy metals was found at the lower concentration (1 mg L–1) because low concentrations of heavy metal solution could provide multiple adsorption sites for MP particles. Conversely, when the concentration was higher, the adsorption rate decreased, probably because the surface reached a certain coverage rate, affecting the subsequent adsorption rate (Gao et al., 2019). The results above clearly show that the concentration of heavy metals in solution was a factor that greatly influenced the adsorption value of heavy metals on the surface of the PS particles. Oz et al. (2019) said that differences in initial heavy metal concentrations caused differences in the adsorption capacity of MP particles.
Previous studies reported that salinity affects the adsorption behavior between MPs and contaminants (Zhan et al., 2016; Hu et al., 2017). Our results showed that salinity also has an effect on heavy metal adsorption. A higher salinity of the heavy metal solution resulted in a slower adsorption time and lower adsorption capacity. For all types of heavy metals, the adsorption rate at a salinity of 0‰ was significantly higher than that at a salinity of 15‰, and the adsorption rate at a salinity of 15‰ was significantly higher than that at a salinity of 30‰. Adsorption can decrease due to cation competition (Li et al., 2018; Liu et al., 2018).
These results are consistent with Holmes et al. (2014), who reported that the adsorption of the heavy metals Cd, Co, and Ni decreased with increasing salinity. Free ions have the most interactions with the particle surface, and increasing competition for adsorption sites on the particle surface is an important factor.
The type of heavy metal was also an important factor in the adsorption of heavy metals on the surface of MPs. In general, the heavy metals with the highest adsorption amount in this study was Pb, followed by Cu, Zn, and Cd. Figure 4 shows that Pb adsorption was significantly higher than that of Cu, Cu adsorption was significantly higher than that of Zn, and Zn adsorption was significantly higher than that of Cd. These results were similar to those reported by Turner and Holmes (2015) and Gao et al. (2019). Gao et al. (2019) reported that in both freshwater and seawater, Pb had a higher adsorption capacity than the other three heavy metals. This result means that Pb has more affinity with all types and sizes of particles and may have a higher chance of becoming a pollutant combined with MPs. The difference in the adsorption amount of each type of heavy metal may be related to several relevant metal properties, such as hydrolysis value, ionic radius and softness (Covelo et al., 2011; Hu et al., 2018). The value of the ionic radius is inversely proportional to the adsorption affinity for a given metal with the same charge (Saha et al., 2002; Covelo et al., 2011). The hydrated ionic radii of Cu2+, Cd2+, and Pb2+ are 0.419, 0.426, and 0.401 nm, respectively. The order of adsorption affinity was consistent with the order of the hydrated ionic radius, which suggests that electrostatic interactions determine metal adsorption on MPs to some extent, particularly for Pb2+, which has the lowest hydrated ionic radius and the largest electrostatic interaction with MPs among the considered heavy metals. The higher the electrostatic strength of a heavy metal is, the more likely it will be for the heavy metal to be adsorbed on the surface of PS particles. Zou et al. (2020) reported that Pb had stronger adsorption than Cu and Cd on two PE plastic particles, PVC and chlorinated polyethylene (CPE). The high electrostatic strength of Pb played a crucial role in this adsorption. Each PS size adsorbs a different amount of heavy metal. A larger diameter will result in a greater surface area as a medium for the adsorption of heavy metals. The higher the salinity of a solution is, the higher the competition for free metals (Holmes et al., 2014; Hu et al., 2017; Li et al., 2018; Liu et al., 2018). These factors caused the adsorption capacity to be lower and the time needed to reach adsorption equilibrium to be longer.
Polystyrene particles that have adsorbed heavy metals have an opportunity to release heavy metals again if they come into contact with solutions that do not contain heavy metals. Previous studies reported that acidic solutions were used to acidify and release heavy metals from the surface of MPs (Rochman et al., 2013; Holmes et al., 2014). In this study, to examine the level of heavy metal release, we used artificial seawater with salinities of 0, 15, and 30‰ and ensured that the water was free from heavy metal contamination. The results showed that after contact with the water, heavy metals were released from the surface of the PS particles, even though the release rate was slower than those in acidic solutions. The rate of release also differs depending on the size of the PS particles. In general, almost all tests in this study revealed that larger MPs release more heavy metals than smaller particles. The results also showed that the level of heavy metal release was strongly influenced by the PS particle size. Larger particles released heavy metals faster than smaller particles in pure water. This result may be because smaller particles have a higher particle density than larger particles; accordingly, heavy metals adsorbed on the particles would be bound more tightly and would be more difficult to release. Figure 5 shows that at a salinity of 0‰, the release efficiency of Pb, Cd, and Cu at 60 mins exposure by 20 μm PS was significantly lower than that by 50 and 130 μm PS, and the release efficiency by 250 μm PS was significantly higher than that by 50 and 130 μm PS. This result means that the surface area affected not only the adsorption capacity for heavy metals but also the time required for heavy metal release.
Our results also show that salinity can influence the release of heavy metals from PS particles. In general, an increase in the salinity of the liquid in direct contact with particles that have adsorbed heavy metals causes the release time to be slower and the release efficiency to be lower (Figures 6, 7). This effect may be closely related to free ion competition. Solutions that have a higher salt content have higher competition and a higher ion density (Holmes et al., 2014; Li et al., 2018; Liu et al., 2018), which causes heavy metal ions that are adsorbed on the surface of the PS particles to be more difficult to release.
The type of heavy metal was also an important factor in the release rate of heavy metals from the surface of MPs. In general, the heavy metal with the greatest release amount at the equilibrium in this study was Cd, followed by Pb, Cu, and Zn. This situation was found in three different salinity tests. This result was also related to the ionic radius of each heavy metal. Cd2+ has a larger ion radius than the other three heavy metals. The larger the radius of a heavy metal ion is, the weaker the bonds between the ion and the surface of PS particles (Saha et al., 2002; Covelo et al., 2011). These results were similar to Brennecke et al. (2016). Brennecke et al. (2016) reported that a PVC plate (5 × 10 cm) with two additional layers of copper and zinc released more Cu than Zn after contact with solution. This process can also be a serious threat to the water environment. MPs are an important intermediary for the transportation of metals in aquatic environments, and they display a higher adsorption affinity for heavy metals in freshwater than seawater environments (Holmes et al., 2014). MP particles containing heavy metals will be transported to clean waters and eaten by organisms. Aquatic organisms cannot degrade these contaminants through digestion, which leads the heavy metals to bioaccumulate in the food chain, potentially reaching higher trophic levels.
Our findings clearly showed the interactions between MPs and heavy metals and strongly support recent findings that plastic can play a key role as a vector for heavy metals in aquatic systems. It should be noted that this study was limited to a laboratory scale with short-term observations.
Conclusion
Our research indicated that all the variables used in this study influenced the interaction of PS particles with heavy metals in terms of both adsorption capacity and release efficiency. Each heavy metal had a different adsorption and release efficiency due to differences in electrostatic strength. PS particle size was also an important factor in the interactions between heavy metals and PS particles. The larger the particle size was, the higher the adsorption capacity. The release of heavy metals from larger particles was also higher. Salinity also plays an important role in the adsorption capacity and release of heavy metals on the surface of PS particles. The higher the salinity value of the heavy metal solution is, the smaller the adsorption capacity and the longer the time to reach adsorption equilibrium. Likewise, in the release of heavy metals, the higher the salinity value of the solution in contact with the surface of the PS particles is, the lower the release efficiency and the longer it takes to achieve equilibrium. An increase in the concentration of heavy metals in the solution caused the adsorption amount on the surface of the PS particles to also increase, but the adsorption rate decreased. These results clearly showed that PS particles have strong interactions with heavy metals in aquatic systems, influenced by environmental conditions.
Data Availability Statement
The raw data supporting the conclusions of this article will be made available by the authors, without undue reservation.
Author Contributions
KC, MC, and S-YC contributed to conception and design of the study. BB and KC wrote the first draft of the manuscript. RL, HC, and CL wrote the sections of the manuscript. BB and JW organized the database. All authors contributed to manuscript revision, read, and approved the submitted version.
Funding
This study was funded by the National Key Research and Development Program of China (2017YFC1404803), National Natural Science Foundation of China (41576180, 41976216, and U2005207), Ministry of Science and Technology, Taiwan (Grant No. 106-2611-M-019-009), and the Natural Science Foundation of Fujian, China (2020J0141).
Conflict of Interest
The authors declare that the research was conducted in the absence of any commercial or financial relationships that could be construed as a potential conflict of interest.
Acknowledgments
We thank to Z. Y. Cyue, P. H. Chou and H. R. Hsieh for their help with the analyses, and we also thank to P. Y. Chen for his assistance for English improvement.
References
Andrady, A. L. (2017). The plastic in microplastics: a review. Mar. Pollut. Bull. 119, 12–22. doi: 10.1016/j.marpolbul.2017.01.082
Ashton, K., Holmes, L., and Turner, A. (2010). Association of metals with plastic production pellets in the marine environment. Mar. Pollut. Bull. 60, 2050–2055. doi: 10.1016/j.marpolbul.2010.07.014
Barboza, L. G. A., Vieira, L. R., Branco, V., Figueiredo, N., Carvalho, F., Carvalho, C., et al. (2018a). Microplastics cause neurotoxicity, oxidative damage and energy-related changes and interact with the bioaccumulation of mercury in the European seabass, Dicentrarchus labrax (Linnaeus, 1758). Aquat. Toxicol. 195, 49–57. doi: 10.1016/j.aquatox.2017.12.008
Barboza, L. G. A., Vieira, L. R., and Guilhermino, L. (2018b). Single and combined efects of microplastics and mercury on juveniles of the European seabass (Dicentrarchus labrax): changes in behavioural responses and reduction of swimming velocity and resistance time. Environ. Pollut. 236, 1014–1019. doi: 10.1016/j.envpol.2017.12.082
Brandts, I., Teles, M., Goncalves, A. P., Barreto, A., Franco-Martinez, L., Tvarijonaviciute, A., et al. (2018). Effects of nanoplastics on Mytilus galloprovincialis after individual and combined exposure with carbamazepine. Sci. Total Environ. 643, 775–784. doi: 10.1016/j.scitotenv.2018.06.257
Brennecke, D., Duarte, B., Paiva, F., Caçador, I., and Clode, J. (2016). Microplastics as vector for heavy metal contamination from the marine environment. Estuar. Coast. Shelf Sci. 178, 189–195. doi: 10.1016/j.ecss.2015.12.003
Covelo, E. F., Couce, M. L. A., and Vega, F. A. (2011). Competitive adsorption and desorption of cadmium, chromium, copper, nickel, lead, and zinc by humic umbrisols. Commun. Soil Sci. Plant Anal. 35, 2709–2729. doi: 10.1081/css-200036421
Desforges, J. P. W., Galbraith, M., Dangerfield, N., and Ross, P. S. (2014). Widespread distribution of microplastics in subsurface seawater in the NE Pacific Ocean. Mar. Pollut. Bull. 79, 94–99. doi: 10.1016/j.marpolbul.2013.12.035
Edmunds, W. M., Shand, P., Hart, P., and Ward, R. S. (2003). The natural (baseline) quality of ground water in England and Wales: a UK pilot study. Sci. Total Environ. 310, 25–355. doi: 10.1016/s0048-9697(02)00620-4
Filella, M., and Turner, A. (2018). Observational study unveils the extensive presence of hazardous elements in beached plastics from Lake Geneva. Front. Environ. Sci. 6:1. doi: 10.3389/fenvs.2018.00001
Fred-Ahmadu, O., Bhagwat, G., Idowu, O., and Benson, N. (2019). Interaction of chemical contaminants with microplastics: principles and perspectives. Sci. Total Environ. 706:135978. doi: 10.1016/j.scitotenv.2019.135978
Galgani, F., Hanke, G., and Maes, T. (2015). “Global distribution, composition and abundance of marine litter,” in Marine Anthropogenic Litter, eds M. Bergmann, L. Gutow, and M. Klages (Cham: Springer International Publishing), 29–56. doi: 10.1007/978-3-319-16510-3_2
Galloway, T. S. (2015). “Micro- and nano-plastics and human health,” in Marine Anthropogenic Litter, eds M. Bergmann, L. Gutow, and M. Klages (Cham: Springer International Publishing), 343–366. doi: 10.1007/978-3-319-16510-3_13
Gambardella, C., Morgana, S., Ferrando, S., Bramini, M., Piazza, V., Costa, E., et al. (2017). Effects of polystyrene microbeads in marine planktonic crustaceans. Ecotoxicol. Environ. Safety 145, 250–257. doi: 10.1016/j.ecoenv.2017.07.036
Gao, F., Li, J., Sun, C., Zhang, L., Jiang, F., Cao, W., et al. (2019). Study on the capability and characteristics of heavy metals enriched on microplastics in marine environment. Mar. Pollut. Bull. 144, 61–67. doi: 10.1016/j.marpolbul.2019.04.039
GESAMP (Joint Group of Experts on the Scientific Aspects of Marine Environmental Protection) (2015). Sources, Fate and Effects of Microplastics in the Marine Environment: A Global Assessment (Kershaw, P. J., ed.). (IMO/FAO/UNESCO-IOC/UNIDO/WMO/IAEA/UN/UNEP/UNDP Joint Group of Experts on the Scientific Aspects of Marine Environmental Protection). Reports and Studies GESAMP. 90. London: GESAMP, 96.
Gu, Y. G., Lin, Q., Wang, X. H., Du, F. Y., Yu, Z. L., and Huang, H. H. (2015). Heavy metal concentrations in wild fishes captured from the South China Sea and associated health risks. Mar. Pollut. Bull. 96, 508–512. doi: 10.1016/j.marpolbul.2015.04.022
Hidalgo-Ruz, V., Gutow, L., Thompson, R. C., and Thiel, M. (2012). Microplastics in the marine environment: a review of the methods used for identification and quantification. Environ. Sci. Technol. 46, 3060–3075. doi: 10.1021/es2031505
Hodson, M. E., Duffus-Hodson, C. A., Clark, A., Prendergast-Miller, M. T., and Thorpe, K. L. (2017). Plastic bag derived-microplastics as a vector for metal exposure in terrestrial invertebrates. Environ. Sci. Technol. 51, 4714–4721. doi: 10.1021/acs.est.7b00635
Holmes, L. A., Turner, A., and Thompson, R. C. (2012). Adsorption of trace metals to plastic resin pellets in the marine environment. Environ. Pollut. 160, 42–48. doi: 10.1016/j.envpol.2011.08.052
Holmes, L. A., Turner, A., and Thompson, R. C. (2014). Interactions between trace metals and plastic production pellets under estuarine conditions. Mar. Chem. 167, 25–32. doi: 10.1016/j.marchem.2014.06.001
Hu, J. Q., Yang, S. Z., Guo, L., Xu, X., Yao, T., and Xie, F. (2017). Microscopic investigation on the adsorption of lubrication oil on microplastics. J. Mol. Liquids 227, 351–355. doi: 10.1016/j.molliq.2016.12.043
Hu, S., Zhou, Y., Zhou, L., Huang, Y., and Zeng, Q. (2018). Study on the adsorption behavior of cadmium, copper, and lead ions on the crosslinked polyethylenimine dithiocarbamate material. Environ. Sci. Pollut. Res. 27, 2444–2454. doi: 10.1007/s11356-018-3536-3
Jin, Y., Xia, J., Pan, Z., Yang, J., Wang, W., and Fu, Z. (2018). Polystyrene microplastics induce microbiota dysbiosis and inflammation in the gut of adult zebrafish. Environ. Pollut. 235, 322–329. doi: 10.1016/j.envpol.2017.12.088
Khalid, N., Aqeel, M., Noman, A., Hashem, M., Mostafa, Y. S., Alhaithloul, H. A. S., et al. (2020). Linking effects of microplastics to ecological impacts in marine environments. Chemosphere 264:128541. doi: 10.1016/j.chemosphere.2020.128541
Kik, K., Bukowska, B., and Sicinska, P. (2020). Polystyrene nanoparticles: sources, occurrence in the environment, distribution in tissues, accumulation and toxicity to various organisms. Environ. Pollut. 262:114297. doi: 10.1016/j.envpol.2020.114297
Law, K. L., and Thompson, R. C. (2014). Microplastics in the seas. Science 345, 144–145. doi: 10.1126/science.1254065
Li, J., Zhang, K., and Zhang, H. (2018). Adsorption of antibiotics on microplastics. Environ. Pollut. 237, 460–467. doi: 10.1016/j.envpol.2018.02.050
Li, W., Lo, H. S., Wong, H. M., Zhou, M., Wong, C. Y., Tam, N. F. Y., et al. (2020). Heavy metals contamination of sedimentary microplastics in Hong Kong. Mar. Pollut. Bull. 153:110977. doi: 10.1016/j.marpolbul.2020.110977
Lithner, D., Larsson, A., and Dave, G. (2011). Environmental and health hazard ranking and assessment of plastic polymers based on chemical composition. Sci. Total Environ. 409, 3309–3324. doi: 10.1016/j.scitotenv.2011.04.038
Liu, G., Zhu, Z., Yang, Y., Sun, Y., Yu, F., and Ma, J. (2018). Sorption behavior and mechanism of hydrophilic organic chemicals to virgin and aged microplastics in freshwater and seawater. Environ. Pollut. 246, 26–33. doi: 10.1016/j.envpol.2018.11.100
Lu, Y., Zhang, Y., Deng, Y., Jiang, W., Zhao, Y., and Geng, J. (2016). Uptake and accumulation of polystyrene microplastics in zebrafish (Danio rerio) and toxic effects in liver. Environ. Sci. Technol. 50:4054. doi: 10.1021/acs.est.6b00183
Marengo, E., Gennaro, M. C., Robotti, E., Rossanigo, P., Rinaudo, C., and Roz-Gastaldi, M. (2016). Investigation of anthropic effects connected with metal ions concentration, organic matter and grain size in Bormida river sediments. Anal. Chim. Acta 560, 172–183. doi: 10.1016/j.aca.2005.11.086
Mattsson, K., Hansson, L. A., and Cedervall, T. (2015). Nano-plastics in the aquatic environment. Environ. Sci. Process. Impacts 17, 1712–1721. doi: 10.1039/c5em00227c
Mohsen, M., Sun, L., Zhang, L., and Lin, C. (2020). Microplastic fibers transfer from the water to the internal fluid of the sea cucumber Apostichopus japonicus. Environ. Pollut. 27:113606. doi: 10.1016/j.envpol.2019.113606
Oz, N., Kadizade, G., and Yurtsever, M. (2019). Investigation of Heavy Metal Adsorption on Microplastics. Appl. Ecol. Environ. Res. 17, 7301–7310.
Pedà, C., Caccamo, L., Fossi, M. C., Gai, F., Andaloro, F., Genovese, L., et al. (2016). Intestinal alterations in European sea bass Dicentrarchus labrax (Linnaeus, 1758) exposed to microplastics: preliminary results. Environ. Pollut. 212, 251–256. doi: 10.1016/j.envpol.2016.01.083
Puga, S., Pereira, P., Pinto-Ribeiro, F., O’Driscoll, N. J., Mann, E., Barata, M., et al. (2016). Unveiling the neurotoxicity of methylmercury in fish (Diplodus sargus) through a regional morphometric analysis of brain and swimming behaviour assessment. Aquat. Toxicol. 180, 320–333. doi: 10.1016/j.aquatox.2016.10.014
Rios, L. M., Moore, C., and Jones, P. R. (2007). Persistent organic pollutants carried by synthetic polymers in the ocean environment. Mar. Pollut. Bull. 54, 1230–1237. doi: 10.1016/j.marpolbul.2007.03.022
Rochman, C. M., Hoh, E., Hentschel, B. T., and Kaye, S. (2013). Long-term field measurement of sorption of organic contaminants to five types of plastic pellets: implications for plastic marine debris. Environ. Sci. Technol. 47, 1646–1654.
Saha, U. K., Taniguchi, S., and Sakurai, K. (2002). Simultaneous adsorption of cadmium, zinc, and lead on hydroxyaluminum- and hydroxyaluminosilicatemontmorillonite complexes. Soil Sci. Soc. Am. J. 66, 117–128. doi: 10.2136/sssaj2002.0117
Sjollema, S. B., Redondo-Hasselerharm, P., Leslie, H. A., Kraak, M. H. S., and Vethaak, A. D. (2016). Do plastic particles affect microalgal photosynthesis and growth? Aquat. Toxicol. 170, 259–261. doi: 10.1016/j.aquatox.2015.12.002
Tang, S., Lin, L., Wang, X., Feng, A., and Yu, A. (2020). Pb (II) uptake onto nylon microplastics: interaction mechanism and adsorption performance. J. Hazard. Materials 386:121960. doi: 10.1016/j.jhazmat.2019.121960
Thompson, R. C., Olsen, Y., Mitchell, R. P., Davis, A., Rowland, S. J., John, W. G., et al. (2004). Lost at sea: where is all the plastic? Science 304:838. doi: 10.1126/science.1094559
Turner, A., and Holmes, L. A. (2015). Adsorption of trace metals by microplastic pellets in fresh water. Environ. Chem. 12, 600–610. doi: 10.1071/en14143
Vieira, K. S., Neto, J. A. B., Crapez, M. A. C., Gaylarde, C., Pierri, B. S., Saldana-Serrano, M., et al. (2021). Occurrence of microplastics and heavy metals accumulation in native oysters Crassostrea Gasar in the Paranaguá estuarine system, Brazil. Mar. Pollut. Bull. 166:112225. doi: 10.1016/j.marpolbul.2021.112225
Wang, F., Wang, F., and Zeng, E. Y. (2018). “Sorption of toxic chemicals on microplastics,”in Microplastic Contamination in Aquatic Environments. ed. E. Y. Zeng (Amsterdam: Elsevier), 225–247. doi: 10.1016/b978-0-12-813747-5.00007-2
Wang, Q., Zhang, Y., Wangjin, X., Wang, Y., Meng, G., and Chen, Y. (2020). The adsorption behavior of metals in aqueous solution by microplastics effected by UV radiation. J. Environ. Sci. 87, 272–280. doi: 10.1016/j.jes.2019.07.006
Wang, W., Gao, H., Jin, S., Li, R., and Na, G. (2019). The ecotoxicological effects of microplastics on aquatic food web, from primary producer to human: a review. Ecotoxicol. Environ. Safety 173, 110–117. doi: 10.1016/j.ecoenv.2019.01.113
Wright, S. L., Thompson, R. C., and Galloway, T. S. (2013). The physical impacts of microplastics on marine organisms: a review. Environ. Pollut. 178, 483–492. doi: 10.1016/j.envpol.2013.02.031
Xu, B., Liu, F., Brookes, P. C., and Xu, J. (2018). Microplastics play a minor role in tetracycline sorption in the presence of dissolved organic matter. Environ. Pollut. 240, 87–94. doi: 10.1016/j.envpol.2018.04.113
Yooeun, C., Dokyung, K., Shin, W. K., and Youn-Joo, A. (2018). Trophic transfer and individual impact of Nano-sized polystyrene in a four-species freshwater food chain. Sci. Rep. 8:284.
Yousefzadeh, H., Salarian, A. A., and Kalal, H. S. (2018). Study of Pb (II) adsorption from aqueous solutions by TiO2 functionalized with hydroxide ethyl aniline (PHEA/n-TiO2). J. Mol. Liquids 263, 294–302. doi: 10.1016/j.molliq.2018.03.023
Zhan, Z., Wang, J., Peng, J., Xie, Q., Huang, Y., and Gao, Y. (2016). Sorption of 3,3’,4,4’- tetrachlorobiphenyl by microplastics: a case study of polypropylene. Mar. Pollut. Bull. 110, 559–563. doi: 10.1016/j.marpolbul.2016.05.036
Zhang, G., Pan, Z., Hou, X., Wang, X., and Li, X. (2014). Distribution and bioaccumulation of heavy metals in food web of Nansi Lake, China. Environ. Earth Sci. 73, 2429–2439. doi: 10.1007/s12665-014-3592-z
Keywords: polystyrene, adsorption capacity, heavy metal, release rate, salinity
Citation: Barus BS, Chen K, Cai M, Li R, Chen H, Li C, Wang J and Cheng S-Y (2021) Heavy Metal Adsorption and Release on Polystyrene Particles at Various Salinities. Front. Mar. Sci. 8:671802. doi: 10.3389/fmars.2021.671802
Received: 24 February 2021; Accepted: 29 April 2021;
Published: 21 June 2021.
Edited by:
Haibo Zhang, Zhejiang Agriculture and Forestry University, ChinaCopyright © 2021 Barus, Chen, Cai, Li, Chen, Li, Wang and Cheng. This is an open-access article distributed under the terms of the Creative Commons Attribution License (CC BY). The use, distribution or reproduction in other forums is permitted, provided the original author(s) and the copyright owner(s) are credited and that the original publication in this journal is cited, in accordance with accepted academic practice. No use, distribution or reproduction is permitted which does not comply with these terms.
*Correspondence: Kai Chen, a2NoZW5AeG11LmVkdS5jbg==; Sha-Yen Cheng, ZXJpY0BtYWlsLm50b3UuZWR1LnR3