- 1Center for Coastal and Ocean Mapping, University of New Hampshire, Durham, NH, United States
- 2NOAA Office of Ocean Exploration and Research, Silver Spring, MD, United States
- 3Department of Biology, University of Hawai‘i at Mānoa, Honolulu, HI, United States
Deep sea canyons and seamounts are topographically complex features that are considered to be biological hotspots. Anthropogenic pressures related to climate change and human activities are placing the species that inhabit these features at risk. Though studies have examined species composition on seamounts and canyons, few have compared communities between them, and even fewer studies have examined how species’ abundances correlate with environmental conditions or geomorphology. Consequently, this study compares species composition, community structure, and environmental variables between Northwest Atlantic continental margin canyons and seamounts along the New England Seamount Chain. Geoforms were also related to the occurrence of phyla and biodiversity. Overall, there was a significant difference in species composition between canyons and seamounts with sponges, corals, sea urchins and seastars contributing heavily to observed differences. Environmental conditions of temperature and salinity and the seafloor property slope contributed significantly to communities observed on seamounts, while substrate, depth and salinity contributed significantly to canyon communities. Abundances were significantly higher in canyons, but taxonomic richness, evenness, and diversity were all greater on seamounts. In an era where climate change and human activity have the potential to alter environmental parameters in the deep sea, it is important to examine factors that influence the spatial distribution of deep-sea benthic communities.
Introduction
Biological communities in the deep sea can be heavily impacted by commercial fishery trawling (Althaus et al., 2009; Hiddink et al., 2017), are predicted to experience climate-related stress (Lazier, 1988; Dickson et al., 2002; Levin and Le Bris, 2015; Danovaro et al., 2017), and face future potential threats from deep sea mining. Indeed, recent studies indicate that water column properties of the deep-sea are changing in response to a changing climate (Purkey and Johnson, 2010; Bindoff et al., 2019; Brito-Morales et al., 2020). The deep sea has been warming over the past decades and it is predicted this trend will continue with on-going changes in climate (Bindoff et al., 2019). The proposed rate of warming of 0.01–0.1°C per decade (Purkey and Johnson, 2010) in the deep ocean (1,000–4,000 m) may also accelerate due to climate change (Brito-Morales et al., 2020). In addition to warming, dissolved oxygen (DO) has declined over the past few decades and this trend is predicted to continue (Joos et al., 2003; Schmidtko et al., 2017). Species that inhabit the deep sea have evolved in relatively stable environmental conditions (Ayala et al., 1975), in which small environmental changes may negatively affect the physiology and growth of deep-sea species (Pörtner and Farrell, 2008; Dijkstra et al., 2021). Anthropogenic stressors associated to changes in climate may lead to changes in the distribution and abundance of deep-sea benthic communities.
Water temperatures are expected to increase and dissolved oxygen concentrations are expected to decline around the seamounts and submarine canyons in the Northwestern Atlantic (Bindoff et al., 2019). Submarine canyons and seamounts are ecologically and economically important features that support a high biodiversity and biomass of cold water corals, sponges and bryozoan reefs (Rowden et al., 2010; Vetter et al., 2010; Lapointe et al., 2020). They are also habitat for fishes, some of which are commercially important (Miller et al., 2015; Quattrini et al., 2015). Though canyons and seamounts serve as hotspots for benthic faunal diversity and abundance, their contrasting geomorphological and environmental variables can differentially influence associated species assemblages (Schlacher et al., 2007; Cordes et al., 2010; Quattrini et al., 2015, 2017; Gallo et al., 2020). Valleys in the continental slope often constrain accelerated currents that transport nutrients and debris down slope (Vetter and Dayton, 1999; De Leo et al., 2010) to create complex circulation patterns that facilitate high secondary production (Shepard et al., 1979; Mortensen and Buhl-Mortensen, 2005). Due to their rugged topography, canyons are not typically trawled and can therefore provide refuge for commercially harvested species (Yoklavich et al., 2000). Seamounts are undersea mountains rising from the abyssal plains. Similar to canyons, their topography disrupts ocean circulation, causing localized changes in currents that at times remove soft sediment (Koslow et al., 2016). Seamounts are thought to serve as stepping stones for larval dispersal (Rowden et al., 2010) and their unique topography combined with associated up-or-down-welling enhances biodiversity (Roden and Taft, 1985; Genin et al., 1986; Levin and Sibuet, 2012).
Seamount and submarine canyon features influence the distribution and abundance of organisms and can increase regional biodiversity (Rowden et al., 2010). Hard-bottoms within these features facilitate dispersal and the maintenance of inhabitant populations by serving as favorable substrates for species such as corals and sponges (Rowden et al., 2010; Dijkstra et al., 2021). Although many studies have characterized communities in submarine canyons (e.g., Hargrave et al., 2004; Kelly et al., 2010; Quattrini et al., 2015) and on seamounts (e.g., McClain et al., 2009; Rowden et al., 2010; Lapointe et al., 2020), few have compared communities on seamounts to those observed in canyons (e.g., Kelly et al., 2010; Rowden et al., 2010). Further, many studies obtain presence/absence data either to establish a species inventory or due to the difficulties in obtaining accurate counts of organisms per unit area (Hargrave et al., 2004; Mortensen and Buhl-Mortensen, 2005), which limits the usefulness of the data for detecting areas of high abundances or biodiversity for management applications (Gonzalez-Mirelis et al., 2021). Using species abundance data can provide a more comprehensive representation of benthic communities that can be used to improve models designed to understand species distribution patterns under a changing climate (Morato et al., 2020) or improve species distribution models. In this study, we use the abundance of individual taxa to (1) compare assemblages observed in canyons and on seamounts of the Northwest Atlantic continental margin and New England Seamount Chain, respectively, (2) determine taxa that are common to these assemblages, (3) examine community-environment relationships in canyons and seamounts, and (4) examine relationships between geomorphology and biodiversity.
Materials and Methods
Study Site and Data Collection
Remotely-operated vehicle (ROV) underwater video footage from five canyons and five seamount benthic communities (Figure 1) along the Northwest Atlantic continental margin and New England Seamount Chain were compared (see Figure 2 for site descriptions). One ROV transect was collected per site with the exception of McMaster Canyon in which two shorter ROV transects were collected. Depth of ROV dives ranged between 410 to 2,741 m with bottom time ranging from 4 to 8 h (see Table 1). The video footage was collected using the ROV Deep Discoverer (D2) aboard the NOAA Ship Okeanos Explorer. D2 is equipped with a CTD that records temperature, salinity, dissolved oxygen, and depth. It also has two lasers that are spaced 0.1 m apart. The location of D2 throughout the ROV dive is determined by an ultra-short baseline (USBL) positioning system (see Kennedy et al., 2019 for more information on ROV navigation).
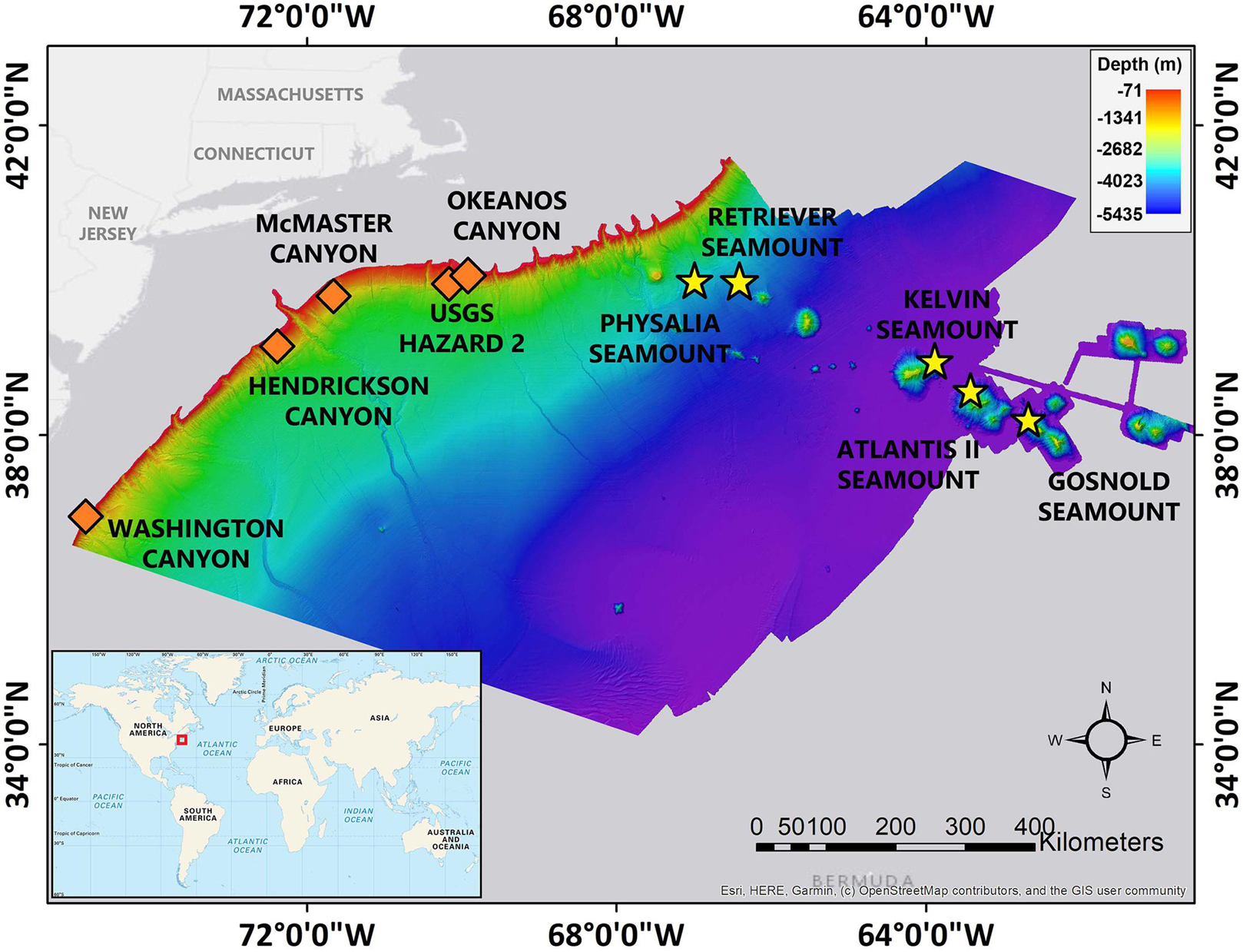
Figure 1. Location of study sites along the Northwest Atlantic continental margin and New England Seamount Chain. The canyon sites are marked with the orange diamonds and the seamount sites are marked with the yellow stars. Figure modified from Dijkstra et al. (2021).
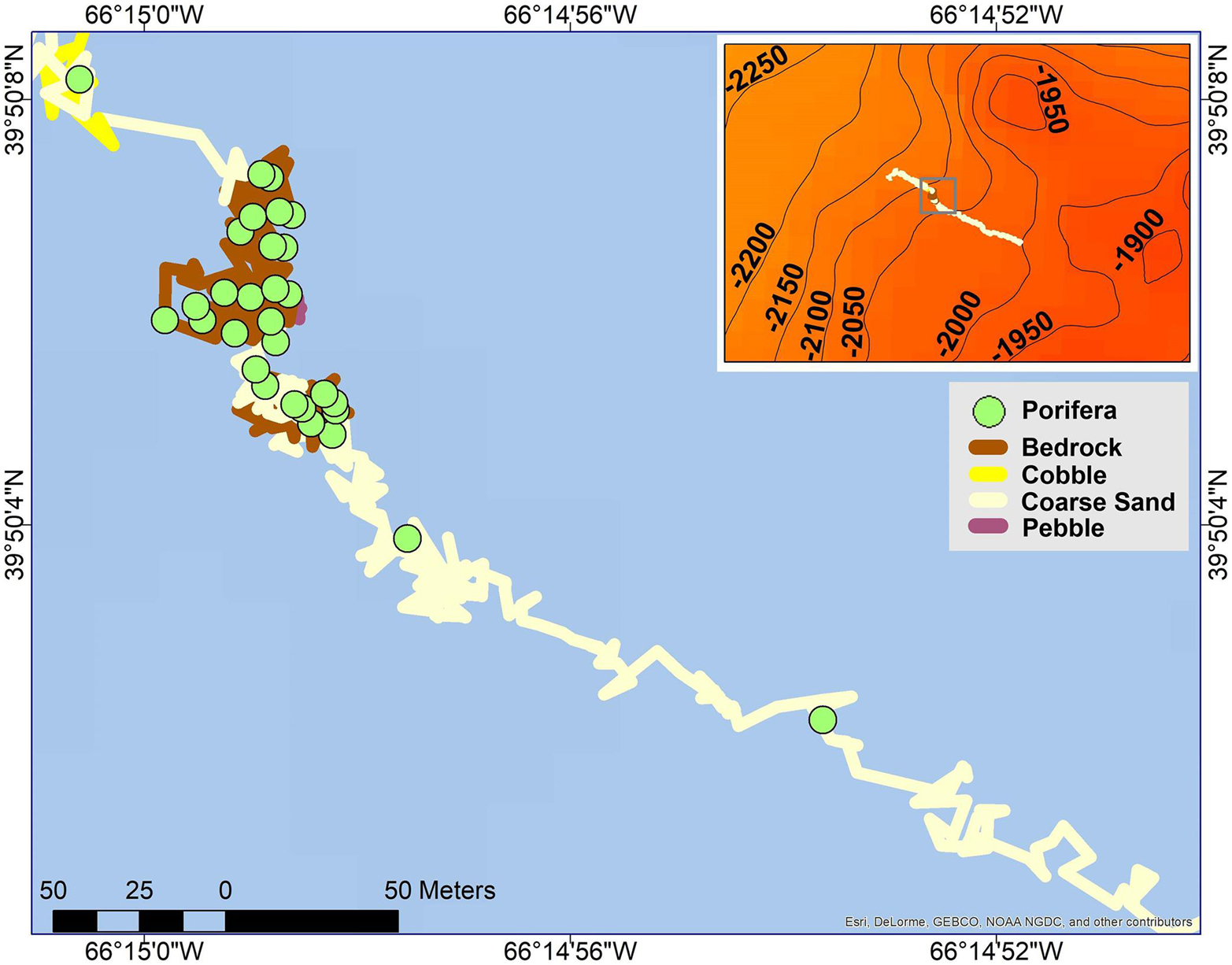
Figure 2. Individuals from the phylum Porifera plotted along a segment of the ROV track on Retriever Seamount. Top right corner: full ROV track plotted over 50 m contour lines and bathymetry.
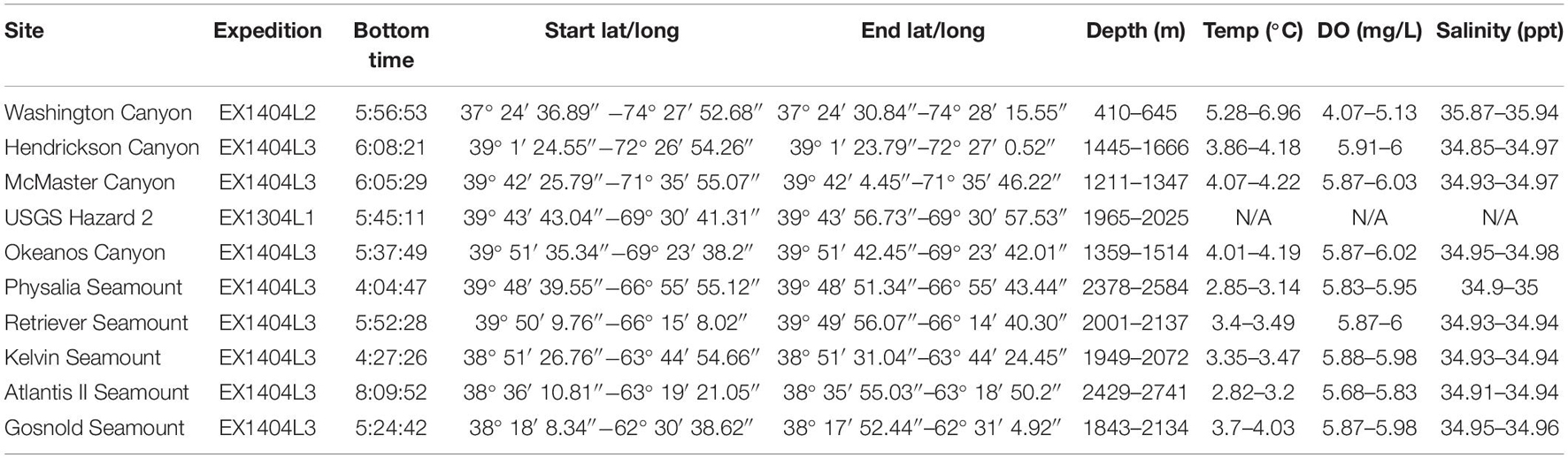
Table 1. Description of all sites analyzed in this study along with ranges of depth (m), temperature (°C), dissolved oxygen (mg/L), salinity (ppt) observed at each dive site.
Video Analysis
Approximately 57 h of video of the seafloor was analyzed by one observer. Each ROV track was divided into 50 m segments. Within each segment, organisms were identified to the lowest taxonomic level possible using a combination of the recorded auditory and written events log for each dive. Identifications were also verified using identification guides (National Oceanic and Atmospheric Administration, Office of Ocean Exploration and Research, 2020). Corals of the New England Seamount Chain have been extensively identified using physical samples (reviewed in Watling et al., 2011; Lapointe et al., 2020), however, our identification was made based on video imagery as the ROV was not equipped to collect voucher specimens during these expeditions. Individuals that could not be identified to species, genus or family level were identified at the class or higher level. Higher identification level occurred when there was insufficient morphological detail of an individual for genera or family level identification. Once the video was annotated, annotations were then synchronized to the navigation and CTD files using a customized Python script.
Organismal abundances were obtained by annotating organisms observed within the 0.1 m laser dots with an additional 0.2 m analyzed on either side of the laser points for a total width of 0.5 m. For portions of the video in which the lasers were turned off, organisms were placed in bins of abundances of 1, 2–4, 5–9, 10–14, and 15–19 with corresponding values of 1, 3, 7, 12, and 17 used for further analysis. Segments with organismal abundance higher than 20 were binned by groups of 10 (e.g., 21–30, 31–40 etc.) and were assigned the average (see Dijkstra et al., 2021 for more information). Segments in which the organisms could not be identified owing to the altitude of the ROV or when the camera was zoomed in were not annotated. Temperature, salinity, dissolved oxygen, depth, and slope were averaged for each 50 m segment. All organism annotations were plotted along the ROV track using ArcGIS (for an example see Figure 2). Primary substrates were classified for each segment using the Coastal and Marine Ecological Classification Standard (CMECS, FGDC, 2012). Ten primary substrate classes were identified: bedrock, silty bedrock, boulder, pebble, cobble, coarse sand, muddy sand, silty sand, clayey sand, and coral rubble.
Statistical Analysis: Community Composition and Environmental Variables
To examine divergence in species assemblages between canyons and seamounts, a Bray-Curtis resemblance matrix was constructed using fourth-root transformed abundances observed in each 50 m segment of the ROV track (total number of segments was 1,796 with 1,105 segments that had benthic species, which equates to ∼27,625 m2). A two-way nested Analysis of Similarities (ANOSIM) with site nested within seafloor feature (i.e., canyon or seamount) was used to determine differences in faunal composition between canyons and seamounts. To determine if our shallowest site (410–645 m), Washington Canyon, significantly contributed to the differences in species composition, we also performed a two-way nested ANOSIM comparing canyons and seamounts excluding this canyon. Discriminator taxa were calculated using SIMPER (SIMilarities PERcentages) with the PRIMER 7.0 package. Discriminator taxa, those with high and even distributions in most segments observed in canyons, but rare or unevenly in segments on seamounts, were determined. Discriminator taxa were calculated using square-root transformed Bray-Curtis similarities by first computing the average dissimilarity in species composition between canyons and seamounts (Clarke and Ainsworth, 1993). The overall average dissimilarity was then broken down into separate contributions from each species and the consistency with which each species contributed to the overall dissimilarity. A good discriminator species not only contributes to the overall dissimilarity, but does so consistently (Clarke and Warwick, 2001).
To examine which suite of environmental variables (see Table 1 for environmental variables) characterized canyon and seamount species assemblages, we correlated log transformed and normalized environmental variables to the corresponding Bray-Curtis similarity matrix calculated using the BEST procedure with Spearman rank correlation coefficients (Clarke and Ainsworth, 1993). Site “USGS Hazard2” was excluded from this analysis as the CTD sensors did not function properly and consequently only 915 segments were used for this analysis. Temperature and depth were highly correlated (99%) in canyons so only depth was used in the analysis. In contrast, all seamount environmental variables were used in the BEST analysis. Spearman rank correlations with 99 permutations tested for the significance of the correlations with p = 0.01 as a significance level due to the spatial autocorrelation in the environmental data among segments within a ROV track. All of the above analyses were performed in the statistical software program PRIMER-E 7.0.
Taxonomic richness, abundance, Shannon-Wiener diversity index (H′) and Pielou’s evenness index (J′) were calculated for each 50 m ROV segment (1,105 segments in all). The non-parametric Wilcoxon-Test was then used to determine differences in average richness, abundance, H′, and J′ between seamounts and canyons as variables were not homogeneous and did not conform to the assumptions of parametric statistics.
Geomorphic Features
Geomorphic features were classified using a semi-automated terrain analysis technique (Masetti et al., 2018) applied to a full coverage bathymetric grid of the study region synthesized from publicly available quality-controlled multibeam sonar surveys. Bathymetry was classified into four simple geomorphic landform classes: flats, ridges, slopes, and valleys. Landform classes were converted to proposed CMECS geoform classes primarily by re-naming them as appropriate for the marine setting in which the units occurred (seamount, continental slope, and abyssal plain), yielding fourteen possible distinct geoform types. The geoform classes for this study were limited to features discernible from a 100 m resolution bathymetric grid and were thus broad-scale in size. Fine-scale geomorphic features that are visually apparent in ROV video (and that may be an important contributor to habitat suitability for benthic fauna) are not captured by the geoform classes. Every segment in each of the study sites was categorized into one of 4 different geoform classes: Continental Slope Slope or Continental Slope Valley (located within the canyons region), and Seamount Ridge or Seamount Slope (located within the seamounts region). Geoform classes represent existing and provisional units derived in accordance with NOAA’s Coastal and Marine Ecological Classification Standard (CMECS). Diversity and abundances of phyla across geoforms did not conform to the assumptions of parametric statistics; therefore, the non-parametric test, Kruskal-Wallis, was used to determine differences in diversity and abundances of phyla among geoforms. If significant differences in abundances were observed within phyla, non-parametric Wilcoxon-pair tests were used to determine differences in abundances among geoforms within phyla.
Results
Ten primary substrates were identified in which 6 were considered hard and 4 considered soft substrate. Seamount transects were found to cover a greater percentage of hard substrate than those in the canyons (Figure 3).
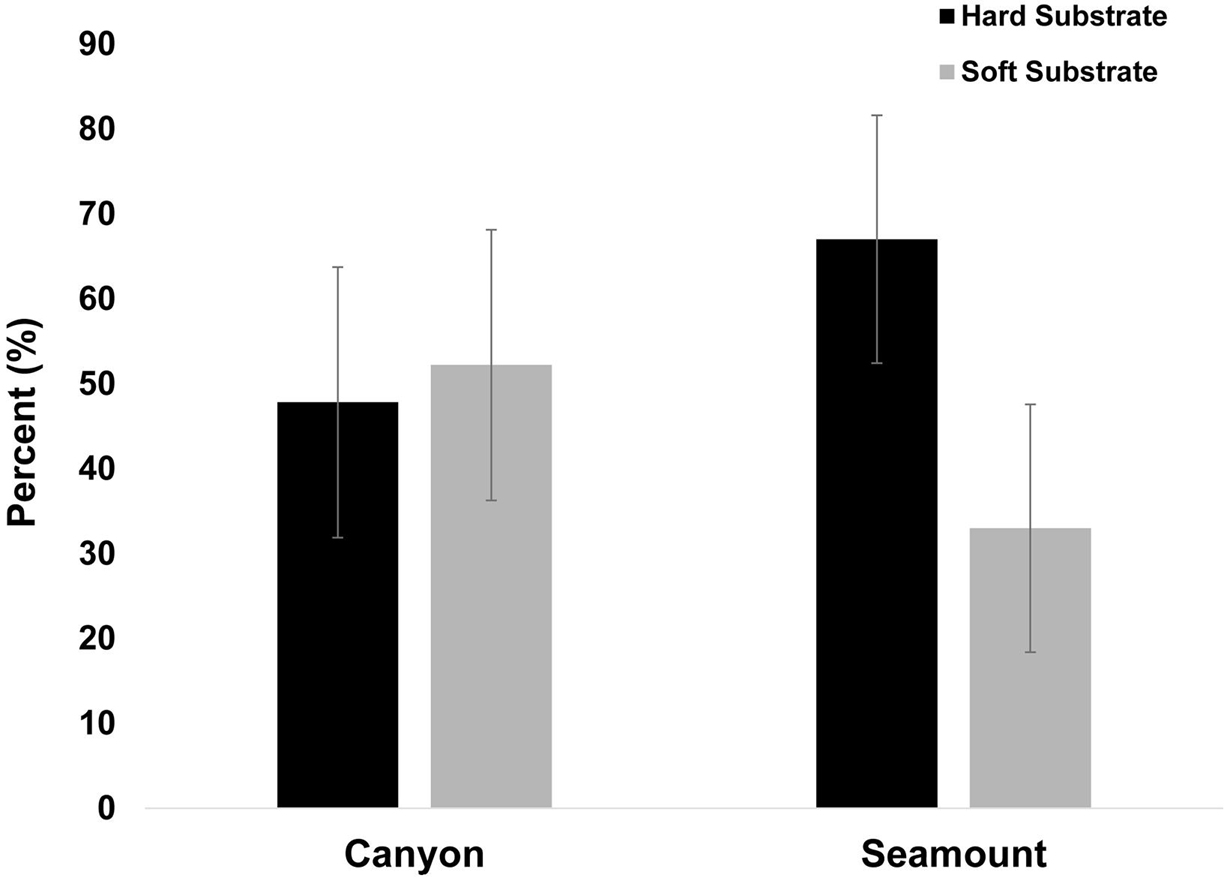
Figure 3. Percent of hard and soft substrates observed in canyons and seamounts (n = 1,796). Hard substrate was observed more often on seamounts.
Overall, there was a significant difference in taxonomic composition between canyon and seamount species assemblages (R = 0.34, p = 0.0008). Interestingly, exclusion of Washington Canyon resulted in slightly lower differences in species composition (R = 0.39, p = 0.0001) between canyons and seamounts. Taxa that consistently contributed 70% to the dissimilarities between canyon and seamount assemblages were sponges (Porifera sp. 2 and the genus, Farrea spp.), Echinoidea, the cup coral Desmophyllum dianthus, the precious coral Corallium spp., Actiniidea, the foraminifera Xenophyophorea, and the deep-sea crab Chaceon quinquedens (Table 2).
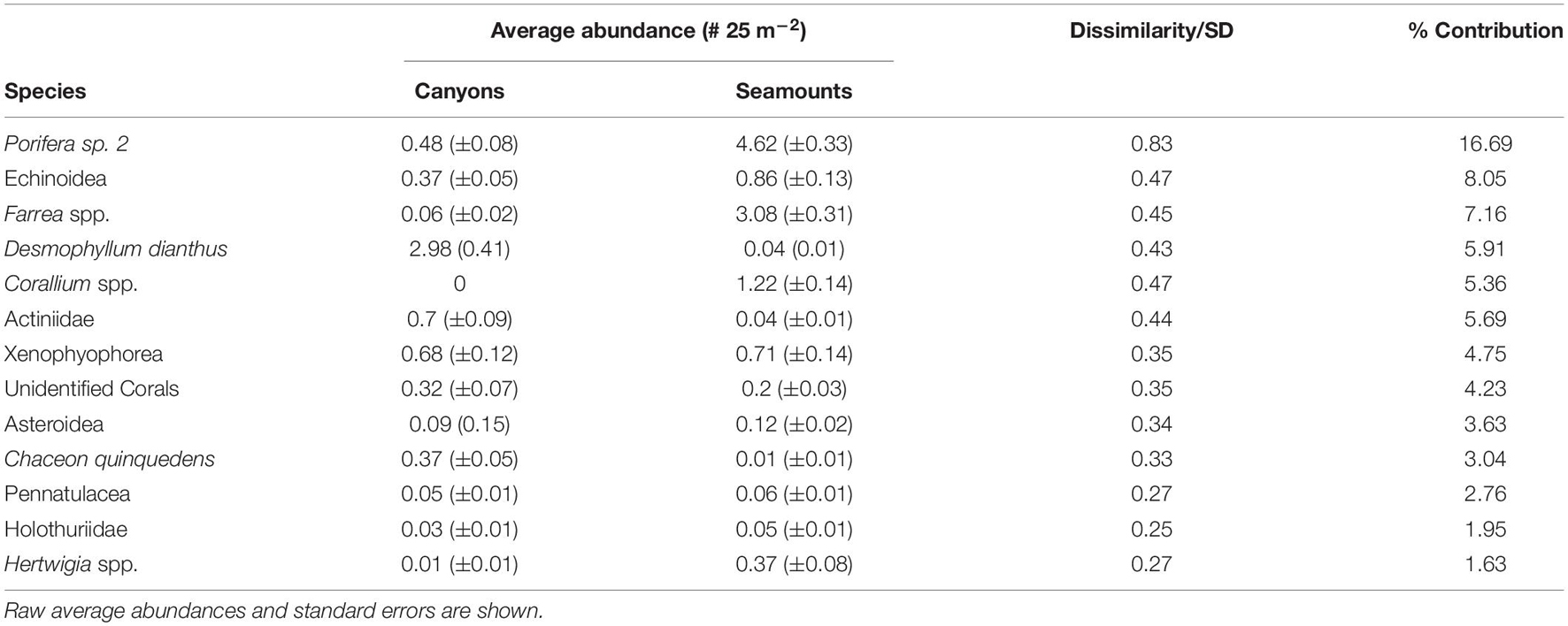
Table 2. SIMPER (similarity percentage) results showing which taxa made the greatest contribution to the dissimilarity between canyon and seamount communities (n = 1,105 segments).
Taxonomic richness, abundance, and diversity were greatest on seamounts [X2 (1) = 54.7, p < 0.001, X2 (1) = 34.2, p < 0.001, X2 (1) = 46.3, p < 0.001, respectively; Figure 4].
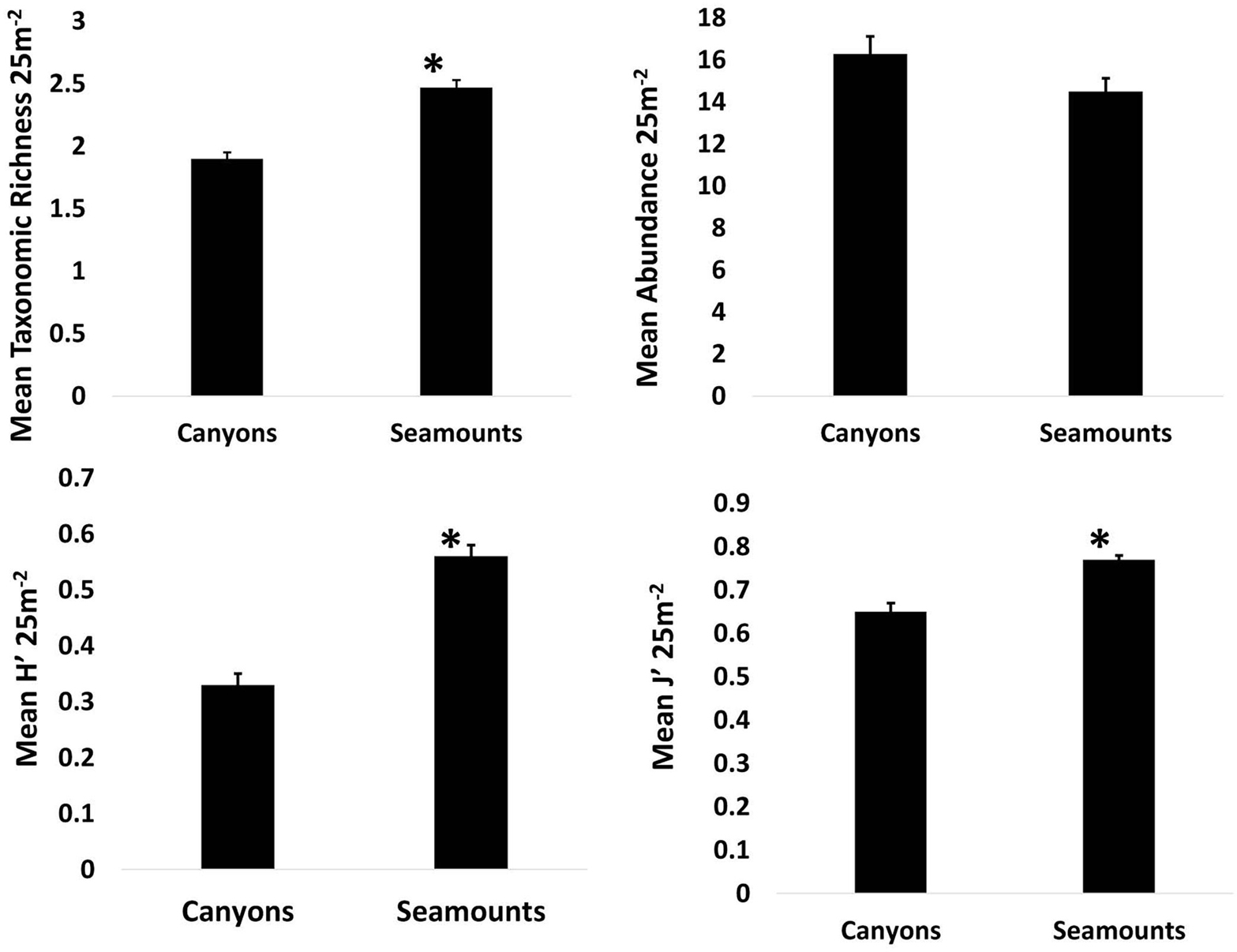
Figure 4. Mean taxonomic richness, abundance, diversity (H′), and evenness (J′) for segments within canyons (n = 533) and segments on seamounts (n = 572). Error bars are standard error. Asterisks denote significant differences.
Environmental variables that correlated most strongly with seamount assemblages were temperature, salinity, and slope (R = 0.26, p = 0.01). Substrate, depth, and salinity correlated most strongly to canyon communities (R = 0.27, p = 0.01).
Four geoform features were classified at our study locations: Continental Slope Slope, Continental Slope Valley, Seamount Ridge, and Seamount Slope. Washington Canyon was outside of the geoform analysis study area and therefore was not classified. Seamount Ridge had the highest diversity while Continental Slope Slope had the lowest (Figure 5). Kruskal-Wallis test revealed significant differences in diversity among geoforms [X2 (3) = 84.2, p < 0.001]. Non-parametric Wilcoxon pair-wise comparisons revealed significant differences in diversity among all geoforms (p < 0.017), but not between Continental Slope Valley and Continental Slope Slope (p < 0.845). Kruskal-Wallis test revealed no significant differences in abundances of arthropods among geoforms [X2 (3) = 2.24, p < 0.5]. Significant differences in abundances within phyla as a function of geoform were found for Foraminifera [X2 (3) = 17.5, p < 0.001], Echinodermata [X2 (3) = 88.5, p < 0.001], Mollusca [X2 (3) = 55.4, p < 0.001], Porifera [X2 (3) = 406.1, p < 0.001], and Cnidaria [X2 (3) = 20.4, p < 0.001]. Non-parametric Wilcoxon pair-wise comparisons revealed that abundances of phyla varied with the geomorphology of the seafloor (Figure 6). High abundances of the phyla, Echinodermata, Mollusca, and Foraminifera were found on Continental Slope Valley. The highest abundances of the phylum Porifera were found on Seamount Ridges while abundances of Cnidaria were slightly lower on Seamount Ridges and Seamount Slopes, respectively, than the other geoforms.
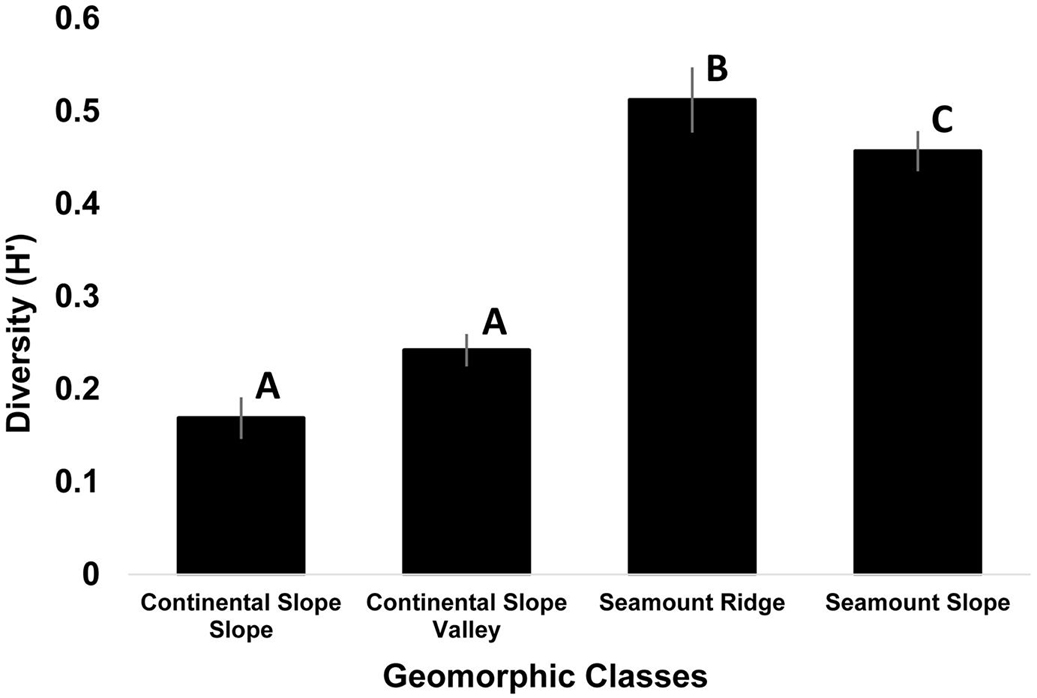
Figure 5. Diversity as a function of geomorphology (n = 1,105). Washington Canyon was excluded as bathymetry was not collected at this site. Highest diversity was observed on seamounts, specifically seamount ridges. Diversity was significantly different among all geoforms, except for Continental Slope Slope and Continental Slope Valley in which no differences were observed. Error bars represent standard error and different letters denote significant differences.
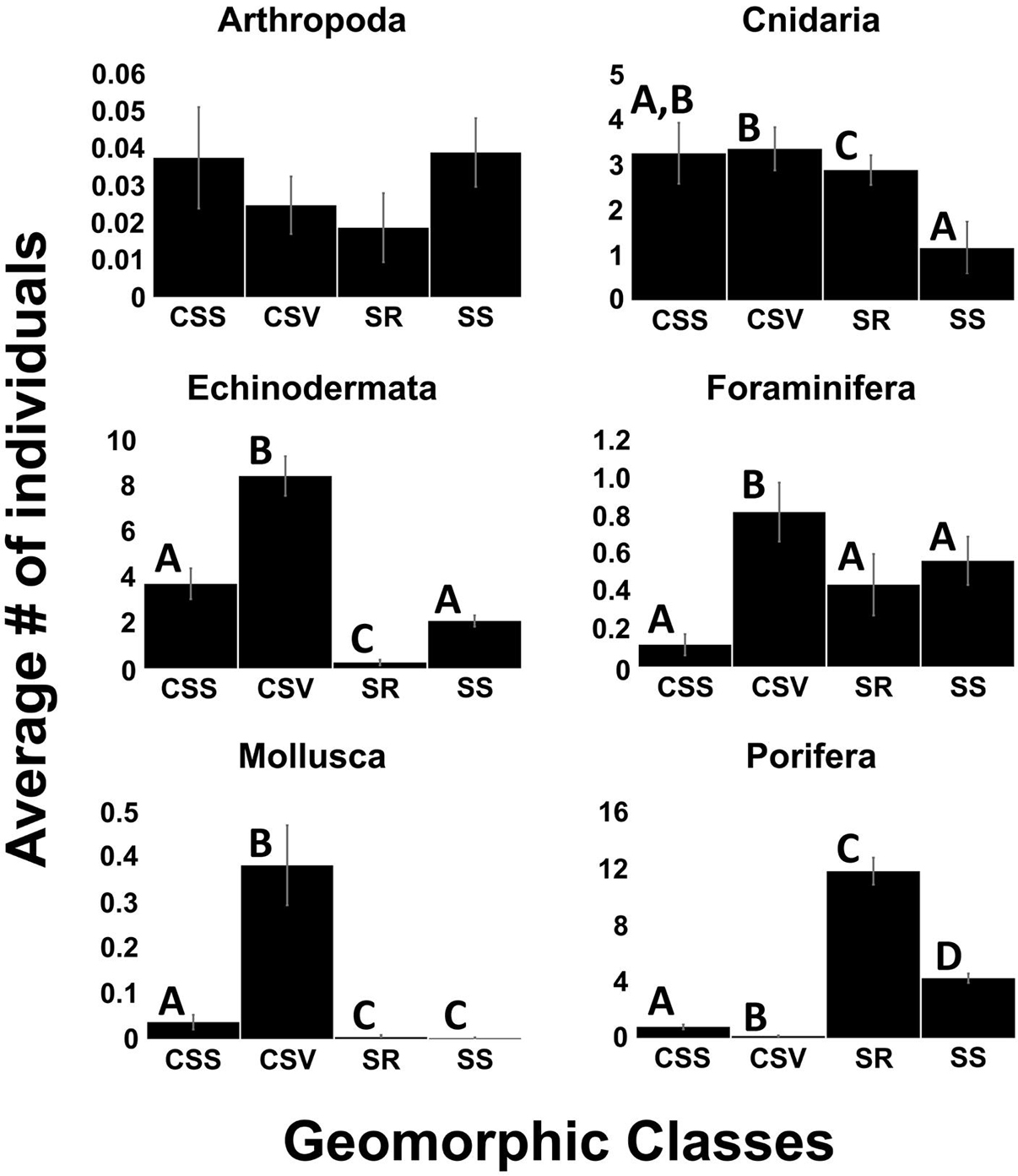
Figure 6. Abundances of phyla as a function of geoformorphic classes Continental Slope Slope, Continental Slope Valley, Seamount Ridge, and Seamount Slope (n = 1,105). Error bars are standard error. Different letters within phyla denote significant differences in abundances.
Discussion
This study demonstrates that there are significant differences in species composition between canyon and seamount communities that are driven by the abundances of sponges, sea urchins and corals. Abundances of sponges and the coral Corallium spp. were higher on seamounts than in canyons, while abundances of the cup coral Desmophyllum dianthus were much greater in canyons. The cup coral prefers sediment free vertical surfaces for settlement (Cairns, 1995; Fillinger and Richter, 2013), which were often observed in the canyon study sites. Actinarians drove the high abundances of cnidarians in canyons, specifically on the geoform Continental Slope Valley, while the branching corals, Corallium spp. and unidentified branching corals were most often observed on seamounts. Similarly, sponges were mainly observed on Seamount Ridges and Seamount Slopes. Greater abundances of sponges on seamounts may result from greater oxygen and food availability as previous studies have shown that current speed over seamount peaks can be double that of wide, sloping areas at the same depth, leading to greater food availability on peaks (Genin et al., 1986). Areas with high currents are generally well oxygenated and combined with high food availability may bolster population growth. Interestingly, abundances of the squat lobster, Gastroptychus formosus, were greatest on Seamount Slopes. Adults are known to have strong habitat associations with branching corals (Guilloux et al., 2009), whose abundances are highest on seamounts (Dijkstra et al., 2021). Associations between corals and other invertebrates are not uncommon in the deep sea. Indeed, a number of species from the class Ophiuroidea in this study were associated with the branching coral Paramuricea spp., which was more often observed on seamounts than in canyons (Dijkstra et al., 2021). Ophuroids not associated with corals were observed, along with bivalves, in high abundances on the geoform Continental Slope Valley. Ophuroids are known to concentrate in areas of high carbon export such as canyons where materials from the continental shelf filter down the valleys to the seafloor (Woolley et al., 2016). Bivalves, on the other hand, anchor on the hard substrate protruding from the valley floor or on vertical ledges using byssal threads.
Temperature, salinity and slope correlated with seamount communities. The complex topography of seamounts are known to produce localized physical oceanographic environments that can be associated with strong currents that prevent a build-up of sediment and exposes bare substrate for settlement by sessile filter feeders such as corals and sponges that require a hard stable surface for attachment (Lopes et al., 2011; Beazley et al., 2013; Kazanidis et al., 2019). Similar to seamounts the seafloor topography can create localized currents that lead to greater nutrient and dissolved oxygen concentrations, which may enable species to allocate more energy to development and enhance local population growth. High nutrient and dissolved oxygen concentrations combined with a greater amount of sediment-free hard substrate may facilitate local population growth as species that have lecithotrophic larvae will find suitable settlement sites close to the parent. Strong currents that produce sediment-free substrate around seamounts may not change, however, temperature is predicted to increase (Bindoff et al., 2019) and salinity is expected to decrease (Dickson et al., 2002). Though the predictions for temperature and salinity change appear small, deep-sea species have evolved in relatively stable environments and small changes that exceed mean environmental conditions may create a sub-optimal habitat that may lead to reduced survivorship and growth, particularly for sessile filter feeders (Hughes et al., 2019).
Salinity, depth and substrate characterized the canyon communities. Depth is a well-known factor that shapes community composition with differences in species assemblages naturally occurring with depth (Cordes et al., 2010), including those of octocoral and sponge assemblages (Schlacher et al., 2007; Quattrini et al., 2015, 2017). Salinity also strongly influences the composition of species. The deep North Atlantic Ocean, the body of water that influences the New England Seamount communities and canyons (Lapointe et al., 2020), has become slightly less saline over the past 40 years (Dickson et al., 2002), and this trend is expected to continue. Biological responses to small deviations from current ambient levels of salinity are poorly understood, but they may elicit sub-lethal changes in their physiological functioning (e.g., DeBiasse et al., 2018), akin to the sub-lethal effects of temperature deviations (e.g., Hughes et al., 2019).
High habitat heterogeneity observed in canyons has led to the hypothesis that they support high levels of biodiversity (Vetter and Dayton, 1999; De Leo et al., 2010; Fernandez-Arcaya et al., 2017). Interestingly, our study indicates that diversity, evenness, and taxonomic richness were higher on seamounts than in canyons with diversity greatest on the geoform Seamount Ridges. While deep sea canyons are known to have many different habitats and are productive owing to the strong currents and subsequent elevated concentrations of food particles, suspension feeders will concentrate on exposed hard substrate that is found as intermittent precipitous rock walls, boulders and bedrock. In contrast, the geology and hydrodynamics surrounding seamounts result in greater spatially consistent exposed hard substrate that facilitate settlement and growth of filter feeders (Genin et al., 1986; López-Garrido et al., 2020). This may result in a more even distribution of species and individuals as observed in this study.
Documenting species assemblages that inhabit canyons and seamounts provides baseline data that can be used to detect temporal changes in seafloor communities caused by anthropogenic stressors. Temperature, dissolved oxygen and salinity are predicted to change with the on-going effects of climate change. Mean changes in seawater properties may disproportionately affect sessile species such as corals and sponges that when established are unable to move to a more favorable environment. These sessile species act as habitat for fish and other species that use them to seek refuge or food, or as substrate by other filter feeding organisms (Mah, 2015; Ross et al., 2015), and climate induced changes in their composition may propagate through the food chain. Crabs and other mobile species have the ability to move to more favorable environments. However, studies evaluating movement of mobile benthic deep-sea species in response to changing environmental conditions are needed.
Using biological abundance data, rather than presence/absence data, retains information about abundances within spatially-explicit segments observed in this study. This makes the community-environment relationship model more sensitive to variation among communities (Cushman and McGarigal, 2004) which was recently exemplified in a study that compared the usefulness of presence/absence and species abundance data for determination of areas that may require management attention (Gonzalez-Mirelis et al., 2021). They found presence/absence to be insufficient for applied management as modeled presence/absence data highlighted areas that were either too large or too small for their purposes. However, incorporating abundances into their model enabled the detection of specific locations of manageable size that were of interest for conservation. Therefore, incorporating species abundances into models of associated environmental and physical variables will provide better predictions on the effects of climate change on deep-sea communities and improve the detection and refinement of the spatial extent of vulnerable marine habitats.
Data Availability Statement
Raw data used in this study from NOAA expeditions (EX1304L1: Northeast U.S. Canyons Exploration; EX1404L2: Our Deepwater Backyard: Exploring the Atlantic Canyons and Seamounts and EX1404L3: Northeast Seamounts and Canyons) are publicly available and can be accessed via the OER Digital Atlas (https://www.ncei.noaa.gov/maps/oer-digital-atlas/mapsOE.htm, last accessed December 2020). To search, preview, and download the dive video for Okeanos Explorer, go to the OER Video Portal (https://www.nodc.noaa.gov/oer/video/). Coral and sponge data generated for this study can be found in the OBIS database at https://doi.org/10.15468/3u8vf7. Further inquiries can be directed to the corresponding author.
Author Contributions
KM-R, JD, DS, LAM, and MM contributed to the design of the study. KM-R obtained and analyzed the underwater video footage. JD provided the statistical analysis. KM-R initiated the writing with input from JD. All authors contributed to the revision of the manuscript and approved the final submission.
Funding
Funding for this study was provided by NOAA grant NA15NOS4000200. The funding entity did not contribute to this study.
Conflict of Interest
The authors declare that the research was conducted in the absence of any commercial or financial relationships that could be construed as a potential conflict of interest.
Publisher’s Note
All claims expressed in this article are solely those of the authors and do not necessarily represent those of their affiliated organizations, or those of the publisher, the editors and the reviewers. Any product that may be evaluated in this article, or claim that may be made by its manufacturer, is not guaranteed or endorsed by the publisher.
Acknowledgments
The authors would like to thank Semme Dijkstra for the MATLAB code that parsed the ROV video footage into segments and NOAA GRANT NA15NOS4000200 for funding the study. The authors would like to thank the NOAA Office of Ocean Exploration and the scientists, officers, and crew onboard NOAA ship Okeanos Explorer expeditions EX1304L1: Northeast U.S. Canyons Exploration; EX1404L2: Our Deepwater Backyard: Exploring the Atlantic Canyons and Seamounts and EX1404L3: Northeast Seamounts and Canyons for collecting the underwater video imagery and CTD Data. Finally, the authors would like to thank the two reviewers for their comments which greatly improved the manuscript. This is contribution number 122 of the School of Life Sciences, University of Hawai‘i at Mānoa.
References
Althaus, F., Williams, A., Schlacher, T. A., Kloser, R. J., Green, M. A., Barker, B. A., et al. (2009). Impacts of bottom trawling on deep-coral ecosystems of seamounts are long-lasting. Mar. Ecol. Prog. Ser. 397, 279–294.
Ayala, F. J., Valentine, J. W., Hedgecock, D., and Barr, L. G. (1975). Deep-sea asteroids: high genetic variability in a stable environment. Evolution 29, 203–212. doi: 10.2307/2407208
Beazley, L. I., Kenchington, E. L., Murillo, F. J., and Sacau, M. (2013). Deep-sea sponge grounds enhance diversity and abundance of epibenthic megafauna in the Northwest Atlantic. ICES J. Mar. Sci. 70, 1471–1490. doi: 10.1093/icesjms/fst124
Bindoff, N. L., Cheung, W. W. L., Kairo, J. G., Aristegui, J., Guinder, V. A., Hallberg, R., et al. (2019). “Changing ocean, marine ecosystems, and dependent communities,” in The Ocean and Cryosphere in a Changing Climate, eds H.-O. Portner, D. C. Roberts, V. Masson-Delmotte, P. Zhai, M. Tignor, E. Poloczanska, et al. (Geneva: IPCC Special Report on the OCean and Cryosphere in a Changing Climate).
Brito-Morales, I., Schoeman, D. S., Molinos, J. G., Burrows, M. T., Klein, C. J., Arafeh-Dalmau, N., et al. (2020). Climate velocity reveals increasing exposure of deep-ocean biodiversity to future warming. Nat. Clim. Change 10, 576–581.
Cairns, S. D. (1995). The marine fauna of New Zealand: Scleractinia (Cnidaria: Anthozoa). New Zeal. Oceanogr. Inst. Mem. 103:139.
Clarke, K. R., and Ainsworth, M. (1993). A method of linking multivariate community structure to environmental variables. Mar. Ecol. Prog. Ser. 92, 205–219. doi: 10.3354/meps092205
Clarke, K. R., and Warwick, R. M. (2001). Change in Marine Communities: An Approach to Statistical Analysis and Interpretation, 2nd Edn. Plymouth: PRIMER-E Ltd.
Cordes, E. E., Becher, E. L., Hourdez, S., and Fisher, C. R. (2010). Influence of foundation species, depth, and location on diversity and community composition at Gulf of Mexico lower-slope cold seeps. Deep Sea Res. II 57, 1870–1881. doi: 10.1016/j.dsr2.2010.05.010
Cushman, S. A., and McGarigal, K. (2004). Patterns in the species-environment relationship depend on both scale and choice of response variables. Oikos 105, 117–124. doi: 10.1111/j.0030-1299.2004.12524.x
Danovaro, R., Corinaldesi, C., Dell’Anno, A., and Snelgrove, P. V. R. (2017). The deep-sea under global change. Curr. Biol. 27, R431–R510.
De Leo, F. C., Smith, C. R., Rowden, A. A., Bowden, D. A., and Clark, M. R. (2010). Submarine canyons: hotspots of benthic biomass and productivity in the deep sea. Proc. Biol. Sci. 277, 2783–2792. doi: 10.1098/rspb.2010.0462
DeBiasse, M. B., Kawji, Y., and Kelly, M. W. (2018). Phenotypic and transcriptomic responses to salinity stress across genetically and geographically divergent Tigriopus californicus populations. Mol. Ecol. 27, 1621–1632. doi: 10.1111/mec.14547
Dickson, B., Yashayaev, I., Meincke, J., Turrell, B., Dye, S., and Holfort, J. (2002). Rapid freshening of the deep North Atlantic Ocean over the past four decades. Nature 416, 832–837. doi: 10.1038/416832a
Dijkstra, J. A., Mello, K., Sowers, D., Malik, M., Watling, L., and Mayer, L. A. (2021). Fine-scale mapping of deep sea habitat-forming species abundances reveals taxonomic species environmental drivers. Glob. Ecol. Biogeogr. 30, 1286–1298. doi: 10.1111/geb.13285
Fernandez-Arcaya, U., Ramirez-Llodra, E., Aguzzi, J., Allcock, A. L., Davies, J. S., Dissanayake, A., et al. (2017). Ecological role of submarine canyons and need for canyon conservation: a review. Front. Mar. Sci. 4:5. doi: 10.3389/fmars.2017.00005
FGDC (2012). Coastal and Marine Ecological Classification Standard FGDC-STD-018-2012. Reston, VA: Federal Geographic Data Committee.
Fillinger, L., and Richter, C. (2013). Vertical and horizontal distribution of Desmophyllum dianthus in Comau Fjord, Chile: a cold-water coral thriving at low pH. PeerJ 1:e194.
Gallo, N. D., Beckwith, M., Wei, C. L., Levin, L. A., Kuhnz, L., and Barry, J. P. (2020). Dissolved oxygen and temperature best predict deep-sea fish community structure in the Gulf of California with climate change implications. Mar. Ecol. Prog. Ser. 63, 159–180. doi: 10.3354/meps13240
Genin, A., Dayton, P. K., Lonsdale, P. F., and Spiess, F. N. (1986). Corals on seamount peaks provide evidence of current acceleration over deep-sea topography. Nature 322, 59–61. doi: 10.1038/322059a0
Gonzalez-Mirelis, G., Ross, R. E., Albretsen, J., and Buhl-Mortensen, P. (2021). Modeling the distribution of habitat-forming, deep-sea sponges in the Barents Sea: the value of data. Front. Mar. Sci. 7:496688. doi: 10.3389/fmars.2020.496688
Guilloux, E. L., Hall-Spencer, J. M., Söffker, M. K., and Olu, K. (2009). Association between the squat lobster Gastroptychus formosus and cold-water corals in the North Atlantic. J. Mar. Biol. Assoc. U. K. 90, 1363–1369. doi: 10.1017/S0025315410000524
Hargrave, B. T., Kostylev, V. E., and Hawkins, C. M. (2004). Benthic epifauna assemblages, biomass and respiration in the Gully region on the Scotian Shelf, NW Atlantic Ocean. Mar. Ecol. Prog. Ser. 270, 55–70. doi: 10.3354/meps270055
Hiddink, J. G., Jennings, S., Sciberras, M., Szostek, C. L., Hughes, K. M., Ellis, N., et al. (2017). Global analysis of depletion and recovery of seabed biota after bottom trawling disturbance. Proc. Natl. Acad. Sci. 114, 8301–8306. doi: 10.1073/pnas.1618858114
Hughes, A. R., Hanley, T. C., Moore, A. F. P., Ramsay-Newton, C., Zerebecki, R. A., and Sotka, E. E. (2019). Predicting the sensitivity of marine populations to rising temperatures. Front. Ecol. Environ. 17:17–24. doi: 10.1002/fee.1986
Joos, F., Plattner, G. K., Stocker, T. F., Körtzinger, A., and Wallace, D. W. (2003). Trends in marine dissolved oxygen: implications for ocean circulation changes and the carbon budget. Eos Trans. Am. Geophys. Union 84, 197–201. doi: 10.1029/2003eo210001
Kazanidis, G., Vad, J., Henry, L.-A., Neat, F., Berx, B., Georgoulas, K., et al. (2019). Distribution of deep-sea sponge aggregations in an area of multisectoral activities and changing oceanic conditions. Front. Mar. Sci. 6:163. doi: 10.3389/fmars.2019.00163
Kelly, N. E., Shea, E. K., Metaxas, A., Haedrich, R. L., and Auster, P. J. (2010). Biodiversity of the deep-sea continental margin bordering the gulf of maine (NW Atlantic): relationships among sub-regions and to shelf systems. PLoS One 5:e13832. doi: 10.1371/journal.pone.0013832
Kennedy, B. R. C., Cantwell, K., Malik, M., Kelley, C., Potter, J., Elliot, K., et al. (2019). The unknown and the unexplored: insights into the Pacific deep-sea following NOAA CAPSTONE expeditions. Front. Mar. Sci. 6:480. doi: 10.3389/fmars.2019.00827
Koslow, J. A., Auster, P., Bergstad, O. A., Roberts, J. M., Rogers, A., Vecchione, M., et al. (2016). “Biological communities on seamount and other submarine features potentially threatened by disturbance,” in The First Integrated Marine Assessment: World Ocean Assessment I, eds L. Innuss and A. Simcock (New York, NY: United Nations General Assembly), 26.
Lapointe, A. E., Watling, L., France, S. C., and Auster, P. J. (2020). Megabenthic assemblages in the lower bathyal (700-3000 m) on the New England and Corner Rise Seamounts, Northwest Atlantic. Deep Sea Res. Part I Oceanogr. Res. Papers 165:103366. doi: 10.1016/j.dsr.2020.103366
Lazier, J. R. N. (1988). Temperature and salinity in the deep Labrador Sea, 1962-1986. Deep Sea Res. 35, 1247–1253. doi: 10.1016/0198-0149(88)90080-5
Levin, L. A., and Le Bris, N. (2015). The deep ocean under climate change. Science 350, 766–768. doi: 10.1126/science.aad0126
Levin, L. A., and Sibuet, M. (2012). Understanding Continental margin biodiversity: a new imperative. Ann. Rev. Mar. Sci. 4, 79–112. doi: 10.1146/annurev-marine-120709-142714
Lopes, D. A., Hajdu, E., and Reiswig, H. M. (2011). Taxonomy of Farrea (Porifera, Hexactinellida, Hexactinosida) from the southwestern Atlantic, with description of a new species and a discussion on the recognition of subspecies in Porifera. Can. J. Zool. 89, 169–189. doi: 10.1139/z10-105
López-Garrido, P. H., Barry, J. P., González-Gordillo, J. I., and Escobar-Briones, E. (2020). ROV’s video recordings as a tool to estimate variation in megabenthic epifauna diversity and community composition in the Guaymas Basin. Front. Mar. Sci. 7:154. doi: 10.3389/fmars.2020.00154
Mah, C. L. (2015). A new Atlantic species of Evoplosoma with taxonomic summary and in situ observations of Atlatnic deep-sea corallivorous Goniasteridae (Valvatida: Asteroidea). Mar. Biodivers. 8:e5.
Masetti, G., Mayer, L. A., and Ward, L. G. (2018). A bathymetry- and reflectivity based approach for seafloor segmentation. Geosciences 8:14. doi: 10.3390/geosciences8010014
McClain, C. R., Lundsten, L., Ream, M., Barry, J., and DeVogelaere, A. (2009). Endemicity, Biogeography, composition, and community structure on a Northeast Pacific Seamount. PLoS One 4:e4141. doi: 10.1371/journal.pone.0004141
Miller, R. J., Juska, C., and Hocevar, J. (2015). Submarine canyons as coral and sponge habitat on the eastern Bering Sea slope. Glob. Ecol. Conserv. 4, 85–94. doi: 10.1016/j.gecco.2015.05.009
Morato, T., Gonzalez-Irusta, J.-M., Dominguez-Carrió, C., Wei, C.-L., Davies, A., Sweetman, A. K., et al. (2020). Climate-induced changes in the suitable habitat of cold-water corals and commercially important deep-sea fishes in the North Atlantic. Glob. Change Biol. 26, 2181–2201.
Mortensen, P. B., and Buhl-Mortensen, L. (2005). “Deep-water corals and their habitats in the Gully, a submarine canyon off Atlantic Canada,” in Cold-Water Corals and Ecosystems. Erlangen Earth Conference Series, eds A. Freiwald and J. M. Roberts (Berlin: Springer), doi: 10.1007/3-540-27673-4_12
National Oceanic and Atmospheric Administration, Office of Ocean Exploration and Research (2020). Benthic Deepwater Animal Identification Guide V.3. Available online at: https://oceanexplorer.noaa.gov/okeanos/animal_guide/animal_guide.html
Purkey, S. G., and Johnson, G. C. (2010). Warming of global abyssal and deep Southern Ocean waters between the 1990s and 2000s: contributions to global heat and sea level rise budgets. J. Clim. 23, 6336–6351. doi: 10.1175/2010jcli3682.1
Quattrini, A. M., Gómez, C. E., and Cordes, E. E. (2017). Environmental filtering and neutral processes shape octocoral community assembly in the deep sea. Oecologia 183, 221–236. doi: 10.1007/s00442-016-3765-4
Quattrini, A. M., Nizinski, M. S., Chaytor, J. D., Demopoulos, A. W. J., Roark, E. B., France, S. C., et al. (2015). Exploration of the canyon-incised continental margin of the Northeastern United States reveals dynamic habitats and diverse communities. PLoS One 10:e0139904. doi: 10.1371/journal.pone.0139904
Roden, G. I., and Taft, B. A. (1985). Effect of the emperor seamounts on the mesoscale thermohaline structure during the summer of 1982. J. Geophys. Res. 90, 839–855. doi: 10.1029/JC090iC01p00839
Ross, W. W., Rhode, M., and Quattrini, A. M. (2015). Demersal fish distribution and habitat use within and near Baltimore and Norfolk Canyons, U.S. middle Atlantic slope. Deep Sea Res. Part I 54, 975–1007.
Rowden, A. A., Schlacher, T. A., Williams, A., Clark, M. R., Stewart, R., Althaus, F., et al. (2010). A test of the seamount oasis hypothesis: seamounts support higher epibenthic megafaunal biomass than adjacent slopes. Mar. Ecol. 31, 95–106. doi: 10.1111/j.1439-0485.2010.00369.x
Schlacher, T. A., Schlacher-Hoenlinger, M. A., Williams, A., Althaus, F., Hooper, J. N. A., and Kloser, R. (2007). Richness and distribution of sponge megabenthos in continental margin canyons off southeastern Australia. Mar. Ecol. Prog. Ser. 340, 73–88. doi: 10.3354/meps340073
Schmidtko, S., Stramma, L., and Visbeck, M. (2017). Decline in global oceanic oxygen content during the past five decades. Nature 542, 333–339.
Shepard, F. P., Marshall, N. F., McLoughlin, P. A., and Sullivan, G. G. (1979). Currents in submarine canyons and other seavalleys. Am. Assoc. Pet. Geol. Stud. Geol. 8. doi: 10.1306/st8406
Vetter, E. W., and Dayton, P. K. (1999). Organic enrichment by macrophyte detritus, and abundance patterns of megafaunal populations in submarine canyons. Mar. Ecol. Prog. Ser. 186, 137–148. doi: 10.3354/meps186137
Vetter, E. W., Smith, C. R., and De Leo, F. C. (2010). Hawaiian hotspots: enhanced megafauna abundance and diversity in submarine canyons on the oceanic islands of Hawaii. Mar. Ecol. 31, 183–199. doi: 10.1111/j.1439-0485.2009.00351.x
Watling, L., France, S. C., Pante, E., and Simpson, A. (2011). Biology of deep-water octocorals. Adv. Mar. Biol. 60, 41–122. doi: 10.1016/B978-0-12-385529-9.00002-0
Woolley, S. N. C., Tittensor, D. P., Dunstan, P. K., Guillera-Arroita, G., Lahoz-Monfort, J. J., Wintle, B. A., et al. (2016). Deep sea diversity patterns are shaped by energy availability. Nature 533, 393–396. doi: 10.1038/nature17937
Keywords: climate change, deep sea canyons, seamounts, communities, corals, sponges, North Atlantic
Citation: Mello-Rafter K, Sowers D, Malik M, Watling L, Mayer LA and Dijkstra JA (2021) Environmental and Geomorphological Effects on the Distribution of Deep-Sea Canyon and Seamount Communities in the Northwest Atlantic. Front. Mar. Sci. 8:691668. doi: 10.3389/fmars.2021.691668
Received: 06 April 2021; Accepted: 24 August 2021;
Published: 20 September 2021.
Edited by:
Erik Cordes, Temple University, United StatesReviewed by:
Myriam Lacharité, University of Tasmania, AustraliaRachel Przeslawski, Geoscience Australia, Australia
Copyright © 2021 Mello-Rafter, Sowers, Malik, Watling, Mayer and Dijkstra. This is an open-access article distributed under the terms of the Creative Commons Attribution License (CC BY). The use, distribution or reproduction in other forums is permitted, provided the original author(s) and the copyright owner(s) are credited and that the original publication in this journal is cited, in accordance with accepted academic practice. No use, distribution or reproduction is permitted which does not comply with these terms.
*Correspondence: Jennifer A. Dijkstra, amRpamtzdHJhQGNjb20udW5oLmVkdQ==