Supplementing Chitosan Oligosaccharide Positively Affects Hybrid Grouper (Epinephelus fuscoguttatus ♀ × E. lanceolatus ♂) Fed Dietary Fish Meal Replacement With Cottonseed Protein Concentrate: Effects on Growth, Gut Microbiota, Antioxidant Function and Immune Response
- 1Laboratory of Aquatic Animal Nutrition and Feed, College of Fisheries, Guangdong Ocean University, Zhanjiang, China
- 2Aquatic Animals Precision Nutrition and High Efficiency Feed Engineering Research Center of Guangdong, Zhanjiang, China
- 3Key Laboratory of Aquatic, Livestock and Poultry Feed Science and Technology in South China, Ministry of Agriculture, Zhanjiang, China
Oligosaccharides have recently received much attention from researchers owing to their multiple biological activities. This study was conducted to investigate the effects of a diet with reduced fish meal and chitosan oligosaccharide (COS) supplementation on a hybrid grouper (Epinephelus fuscoguttatus ♀ × E. lanceolatus ♂). Seven isonitrogenous and isolipidic types of diet were formulated to feed the groupers for 56 days. To prepare the feed, a reference diet (FM group) containing 55% fish meal was used. Concentrated cottonseed protein (CPC) was used to replace 45% of the fish meal protein, and different COS supplementation levels (0, 0.2%, 0.4, 0.6, 0.8, and 1%) were added. After an 8-week breeding trial, Vibrio harveyi bacteria were injected into the groupers for a 7-day challenge test. The results showed that the FM and COS0.4 groups showed the best growth performance among the groups (p < 0.05); however, there was no significant difference in the survival rate (p > 0.05). Unlike in the FM group, adhesion and breakage of the intestinal plica occurred in the COS0 group. The height and width of the gut fold reached maximum values in the COS0.4 group (p < 0.05). Microbiome sequencing suggested that there was a stable microbiota core in the gut of the groupers. With increasing COS levels, the abundance of both beneficial bacteria and conditional pathogens increased; the activities of serum glucose oxidase, catalase, and total superoxide dismutase also increased (p < 0.05). In the gut tissue, the activities of glutathione peroxidase, glutathione reductase, and glutamine increased first but then decreased (p < 0.05); the contents of lysozyme, acid phosphatase, complements C3 and C4, and IgM showed upward trends (p < 0.05). Compared with that in the FM group, the expression of IL-1β and TNF-α in the COS0 group was upregulated. Gene expression levels of TLR22, TGF-β, and Nrf2 increased first but then decreased with COS supplementation levels (p < 0.05). COS supplementation reduced the cumulative mortality of the groupers in the challenge test (p < 0.05). In general, the results of this study demonstrated that dietary COS supplementation enhanced growth performance, intestinal health, and antioxidant and immune responses of groupers fed with a low-fish meal diet. The optimal and acceptable levels of COS supplement were 0.45 and 0.4–0.6%, respectively; these values can provide a reference for developing aquatic prebiotics.
Introduction
The grouper (Epinephelus fuscoguttatus ♀ × E. lanceolatus ♂) is a new hybrid fish species that has a fast growth rate, enhanced nutritional value, and powerful stress resistance (Zhou et al., 2019). This species is widely cultivated in Southeast Asia and China, and it has maintained bright commercial prospects (Li et al., 2018b; Ye et al., 2020).
In recent years, aquaculture has become a rapidly growing industry that is essential in terms of global food security, because it provides high-quality protein to the public (Zeng et al., 2017). However, fish meal production cannot meet the demands of the aquatic industry (Hardy and Tacon, 2002; Hardy, 2006). In this context, replacing fish meal with plant protein is a feasible approach for sustainable aquaculture (Carter and Hauler, 2000; Daniel, 2018). As a new type of plant protein, cottonseed protein concentrate (CPC) has recently received much attention from researchers (Wan et al., 2018; Ye et al., 2020). Accordingly, the experimental replacement of fish meal with CPC has been conducted for certain aquaculture species, such as hybrid grouper (Chen et al., 2020), juvenile golden pompano (Trachinotus ovatus) (Shen et al., 2020), and pacific white shrimp (Litopenaeus vannamei) (Wan et al., 2018). However, researchers have found that replacing fish meal with high levels of CPC can decrease growth performance and cause intestinal inflammation issues in hybrid groupers (Yin et al., 2018).
In the past, antibiotics have been used as feed supplements to treat fish gut inflammation. More recently, prebiotics have become an environmentally friendly alternative, as they do not cause environmental pollution or public health hazards (Song et al., 2014; Carbone and Faggio, 2016). For example, chitosan oligosaccharide (COS), a novel prebiotic, is prepared by enzymatic degradation, chemical derivatization, and chromatographic separation to obtain chitin from marine shells (Wei et al., 2020). Studies have found that COS supplementation can have multiple physiological benefits, such as antioxidant and anti-inflammatory effects, as well as immune stimulation (Liaqat and Eltem, 2018; Naveed et al., 2019). Other benefits of COS supplementation have been observed for terrestrial animals, such as improvement of fetal survival and reproductive performance in sows (Sus scrofa domesticus) (Wan et al., 2016), adjustment of gut microbiota and metabolites in weaned piglets (Kong et al., 2014), and enhancement of growth performance and serum immunity in chickens (Gus gallus) (Li et al., 2007). COS is a preferred feed additive for livestock, because it acts as an immunostimulant (Swiatkiewicz et al., 2015). In aquaculture, studies have found that dietary COS supplementation can strengthen growth performance, innate immunity, and disease resistance in turbot (Scopthalmus maximus) (Cui et al., 2013), koi (Cyprinus carpio koi) (Lin et al., 2012), and juvenile rainbow trout (Oncorhynchus mykiss) (Luo et al., 2009).
In this context, COS could be provided as an oral immunostimulant to more fish species. However, the role of COS is affected by many factors, such as fish species, body size, and breeding environment (Wei et al., 2020). Therefore, it is worth exploring the effects of prebiotic supplementation after fish meal substitution (Bai et al., 2017; Torrecillas et al., 2018). Currently, COS application is still under development in aquaculture (Zhang, 2019).
To investigate the improvements induced by COS supplementation in hybrid groupers subjected to fish meal replacement with CPC, this study focused on growth, gut microbiota, antioxidant function, and immune response. Additionally, this study evaluated a suitable level of dietary COS supplementation, which will be useful for providing insight into grouper feed development.
Materials and Methods
Experimental Diets
Chitosan oligosaccharide with a purity of >90% was obtained from Bozhi Huili Biotechnology Co., Ltd. (Qingdao, China). Fish meal, CPC, starch, wheat gluten, casein, and gelatin were used as protein sources. Lipids were provided in the form of fish oil and phospholipids. Seven isonitrogenous and isolipidic feeds were prepared to feed the grouper. Based on a previous study (Yin et al., 2018), feed with 55% fish meal was designated as the reference diet (FM). For the test diets, 45% of the fish meal protein was replaced with CPC under uniform conditions. COS supplementation was conducted at different concentrations (0, 0.2, 0.4, 0.6, 0.8, and 1%).
The raw materials were fully mixed and extruded into a hard pellet with a diameter of approximately 2.5 mm. The semi-finished feed was dried at room temperature (25°C) to a 10% moisture content. The finished product was stored at −20°C for later use. The ingredients and approximate nutrient compositions of the test diets are shown in Table 1. The essential amino acid profiles of the test diets are shown in Table 2.
Feeding Management and Challenge Test
This experiment was approved by the Animal Care and Ethics Committee of Guangdong Ocean University. Experimental fish were obtained from East Island, Zhanjiang. A feeding experiment was conducted at the Marine Science and Technology Park of Zhanjiang (Guangdong, China). Seven treatment groups were used in this study, and each treatment group was established in triplicate. The volume of each breeding tank was 0.3 m3, where 30 hybrid groupers (initial weight = 13.19 ± 0.02 g) were cultured. The groupers were acclimated to the laboratory environment for 1 week prior to the experiment. The experimental breeding time was 8 weeks. Feeding was performed at 8:00 and 17:00 daily, and the fish were fully satiated. Each day, 90% of the water in each tank was changed. During breeding, the water temperature ranged from 28.4 to 31.6°C, and salinity was 30 ± 2%0. The average dissolved oxygen content exceeded 7 mg L−1, the pH ranged from 7.8 to 8.1, and the ammonia nitrogen content was below 0.03 mg L−1.
After the 8-week breeding experiment, 30 fish were randomly selected from each treatment group (10 fish from each tank) for a challenge test. Vibrio harveyi bacteria were obtained from the Key Laboratory of Aquatic Economic Animal Disease Control of Guangdong Provincial Colleges and Universities (Zhanjiang, China). According to the method of Liu et al. (2013), 300 μl of a 5 × 108 live V. harveyi bacteria suspension was injected into the base of the pectoral fin of each grouper using a 1-ml syringe. The cumulative mortality of the groupers was obtained for 7 days after injection.
Sample Collection
Before sampling, the groupers were fasted for 24 h counted, and weighed, and their body lengths were measured. Ten fish from each tank were randomly selected and sacrificed. First, blood was drawn from the fish and then centrifuged at 4,000 rpm for 10 min. The serum was stored at −20°C for later use. The visceral mass was separated, and then the visceral mass, liver, and intestine were weighed separately. Intestinal tissue was washed with physiological saline (0.65%) on a sterile operating table, and then it was stored at −80°C for later enzyme activity analysis, microbiome sequencing, and mRNA expression analysis.
Additionally, the intestines of three other fish per tank were separated, washed with sterile saline (0.65%), and preserved in a 4% formaldehyde solution. Intestinal AB–PAS (alcian blue-periodic acid sthiff) sectioning slices were prepared by Seville Biological Technology Co., Ltd. (Guangzhou, China). Histological observations were conducted using a Leica DM6000 optical microscope (Leica, Wetzlar, Germany), and the LAS 3.8 (Leica, Wetzlar, Germany) software (in Windows) was used for taking photographs. Ten visions from each slice were randomly selected for observation.
Growth Parameters and Biochemical Analysis
The parameters of growth performance were calculated as follows:
The standard procedures of the Association of Official Analytical Chemists were adopted to detect the approximate composition of feed (Chemists, 2005).
Determination of Enzyme Activity and Biochemical Indices
According to the methods of Ayiku et al. (2020), glucose oxidase (GLU), alanine aminotransferase (ALT), aspartate aminotransferase (AST), triglyceride (TG), catalase (CAT), total superoxide dismutase (T-SOD), and malondialdehyde (MDA) in serum were detected using a microplate photometer (Multiskan™ Go, Thermo Fisher Scientific, Waltham, MA, United States), using commercial kits obtained from Jiancheng Bioengineering Institute (Nanjing, China).
The intestinal tissue was washed with pre-chilled phosphate-buffered saline (PBS) (0.01 M, pH = 7.4), and then a PBS solution (1:9) was added. The gut tissue was then ground on ice. After homogenization, the homogenate was centrifuged at 5,000 rpm for 5 min. The supernatant was extracted for further testing. The methods for measuring the intestinal immune indices (such as lysozyme, acid phosphatase, complement proteins 3 and 4, and immunoglobulin M) were based on Ye et al. (2020), using enzyme-linked immunosorbent assay (ELISA) detection kits from Meilian Biotechnology Co., Ltd. (Shanghai, China) according to the instructions of the manufacturer. The activities of glutathione peroxidase (GSHPx), glutathione reductase (GR), and glutamine (Gln) were determined using the methods described by Chen et al. (2009). Nitric oxide (NO) content analysis was carried out using the method of Wink et al. (1993). Each sample was tested in triplicate.
Intestinal Microbiome Analysis
According to the methods of Chen et al. (2020), microbiome sequencing technology was used to detect the hybrid grouper intestinal microbiota, with the assistance of Biomarker Technologies Co., Ltd. (Beijing, China). The microbiome analyses of the samples of each treatment were performed in triplicate. Microbiota DNA was extracted using the Soil DNA Kit (Magen Co. Ltd., Guangzhou, China) according to the instructions of the manufacturer. The 16S rRNA V3 + V4 amplified areas of the ribosomal RNA gene were amplified by PCR. PCR was performed as follows: 95°C for 2 min, followed by 27 cycles of 98°C for 10 s, 62°C for 30 s, and 68°C for 30 s, and the final step at 68°C for 10 min. The primer sequences were 5′-ACTCCTACGGGAGGCAGCA-3′ (338F) and 5′-GGACTACHVGGGTWTCTAAT-3′ (806R). The amplicons were separated on a 2% agarose gel and then purified using the AxyPrep DNA Gel Extraction Kit (Axygen Biosciences, Union City, CA, United States). The amplicons were quantified using the ABI StepOnePlus real-time PCR system (Life Technologies, Carlsbad < CA, United States). Later, the purified amplicons were pooled in equimolar amounts, and paired-end sequencing (2 × 250) was carried out on the Illumina platform (Hiseq 2,500, Illumina, San Diego, CA, United States) based on standard protocols. The raw reads generated by the microbiome were deposited into the Sequence Read Archive of the National Center of Biotechnology Information (NCBI) database with the accession number PRJNA728963.
After filtering for noisy sequences and checking for the presence of chimeras, the data were clustered at the 97% similarity threshold (VSEARCH, version 10.0) (Edgar, 2013). The data were assigned to the same operational taxonomic units (OTUs) (Bokulich et al., 2013). To evaluate the sequencing depth, sparse curves were drafted by plotting the number of OTUs against the total number of sequences. Between-group Venn analysis was performed to identify unique and shared OTUs using the microbial indicative analysis function of the BMKCloud platform (http://www.biocloud.net/). The alpha indices (such as the coverage and the ACE, Chao1, Shannon, and Simpson indices) were calculated using the Mothur software (version v.1.30, http://www.mothur.org/) (Schloss et al., 2009; Aßhauer et al., 2015). Additionally, a comparison of the overall bacterial composition (beta analysis) was performed by partial least squares discrimination analysis in the BMKCloud platform, to establish a model of the relationship between sample categories and reduce the dimensionality of the data. Microbial species annotation was conducted using RDP Classifier (version 2.2, http://sourceforge.net/projects/rdpclassifier/) (Wang et al., 2007) based on the SILVA database (https://www.arb-silva.de/).
Real-Time Quantitative PCR Analysis
The TRI Reagent solution (Invitrogen, Carlsbad, CA, United States) was used to extract total RNA from three groupers per experimental group. The quality and quantity of RNA were analyzed by 1% agarose gel electrophoresis and spectrophotometry (A260: 280 nm). First-strand cDNA synthesis was conducted using PrimeScript™ RT-PCR kits obtained from Takara (Kusatsu, Japan), following the instructions of the manufacturer. Real-time quantitative PCR was conducted using quantitative thermal LightCycler480 (Roche Diagnostics, Basel, Switzerland). Amplification was performed in a mixture of 10 μl total volume, which included 5 μl TB Green™ Premix Ex Taq™ II (Takara, Kusatsu, Japan), 0.4 μl of each primer, 3.2 μl RNase-free water, and 1 μl cDNA mix. The steps for the real-time PCR program were as follows: one cycle of 95°C for 30 s, followed by 40 cycles of 95°C for 5 s, and then 60°C for 30 s, followed by a cycle of 95°C for 5 s, 60°C for 60 s, and then 95°C for 5 s, and finally a cycle of 50°C for 30 s. The specific primers used in this study are listed in Table 3. Based on previous results, β-actin was used as the reference gene for the groupers (Yin et al., 2020).
All real-time PCRs were conducted using an Applied Biosystems 7,500 real-time PCR system (Life Technologies, Carlsbad, CA, United States). Melting curve analysis of the amplification products was conducted at the end of each PCR to verify that a single PCR product was generated. Standard curves were obtained using four different dilutions of the cDNA samples in triplicate, and amplification efficiency was calculated using the formula:
The 2−ΔΔCT method was used to calculate the gene expression results using the method described by Dvinge and Bertone (2009).
Statistical Analysis
Statistical analysis was performed using SPSS Statistics 19.0 (IBM, Armonk, NY, United States). All the data were expressed as the mean ± standard error of the mean (SEM). The Shapiro–Wilk and Levene's tests were performed for data normality and homogeneity of variance, respectively. Two analysis methods were used in this experiment: (1) the FM group and each COS-containing group (namely, COS0, COS0.2, COS0.4, COS0.6, COS0.8, and COS1) were analyzed by t-test; (2) one-way ANOVA was performed to analyze the COS-containing groups, and the Tukey method was used for multiple comparisons between groups. Statistical significance was set at p < 0.05.
Ethics Statement
The experimental procedures were approved by the Animal Care and Ethics Committee of Guangdong Ocean University.
Results
Growth Performance and Morphological Indices
As shown in Table 4, there is no significant difference in IW (initial weight) and SR among all the treatments (p > 0.05). FW (final weight), WG, and SGR reached maximum values in the COS0.4 group, and the values are significantly greater than those of the COS0 and COS1 groups (p < 0.05). However, there were no significant differences in FW, WG, and SGR between the COS0.4 and FM groups (p > 0.05). Among the COS-containing groups, FCR was lowest in the COS0.4 group (p < 0.05). However, there was no significant difference in FCR between the COS0.4 and FM groups (p > 0.05). Regarding morphological indices, HSI was highest in the COS0.4 group out of all the treatments (p < 0.05). VSI in the COS0.4 group was higher compared with that in the other COS-containing groups (p < 0.05), but it was not significantly different from that in the FM group (p > 0.05). CF first increased and then decreased in all the different groups. It was highest in the COS0.6 group out of all the groups (p < 0.05). Based on the polynomial curve analysis of SGR for the COS-containing groups, Figure 1 shows that the optimal level of COS supplementation for groupers was 0.45%.
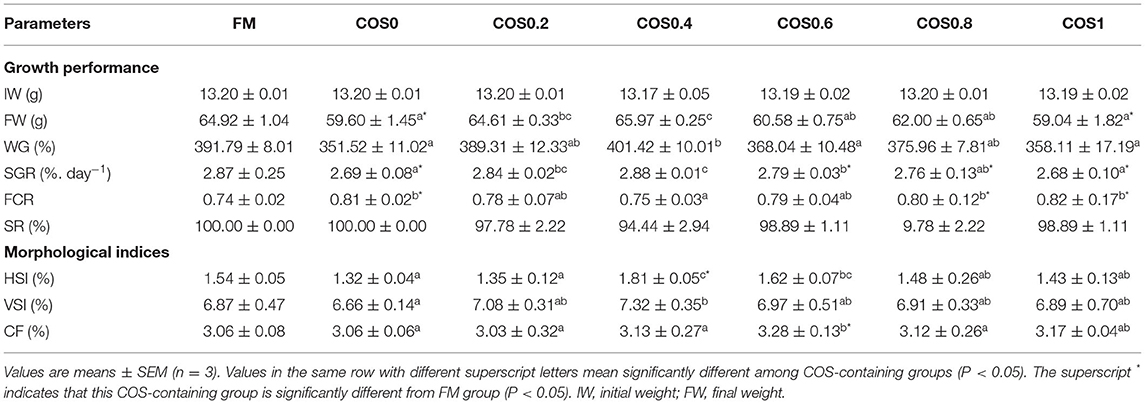
Table 4. Growth performance and morphological indices of hybrid grouper fed with experimental diets for 8 weeks.
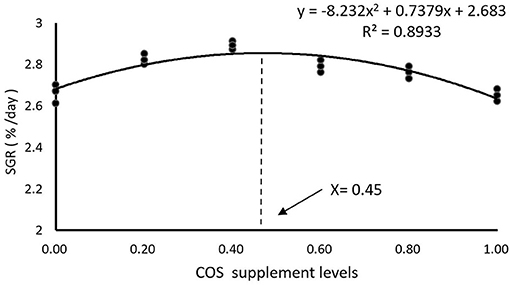
Figure 1. Polynomial curve model analysis of SGR in hybrid grouper fed with experimental diets for 8 weeks.
Intestinal Morphology
Figure 2 shows the intestinal morphology of the hybrid grouper. In the COS0, COS0.8, and COS1 groups, plica adhesion was observed with increase in the level of COS supplementation. In addition, plica breakage appeared in the COS0 and COS1 groups. More vacuolation of epithelial cells was observed in the COS0.6 group than in the other groups. The plica was most uniform in the FM and COS0.4 groups, whereas it had a poor uniformity and decreased density in the COS0 group.
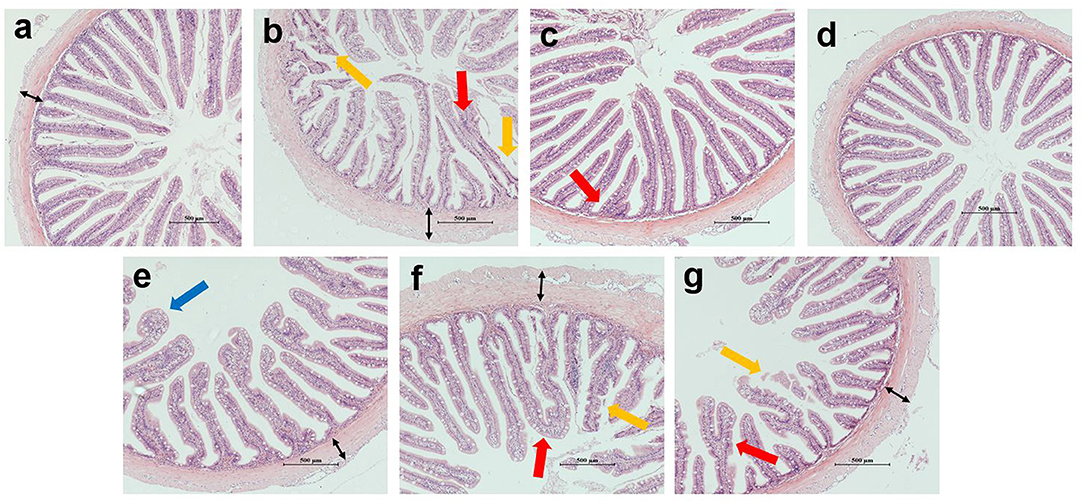
Figure 2. Intestinal morphology of grouper (magnification × 100). (a–g) correspond to FM, COS0, COS0.2, COS0.4, COS0.6, COS0.8 and COS1 groups, respectively. Red arrow: plica adhesion; yellow arrow: plica breakage; blue arrow: vacuolation of epithelial cells; black arrow: thickness of lamina propria. Scale size is 500 μm.
As shown in Figure 3, there are significant differences in the measurements of intestinal morphology. The height and width of the plica first increased and then decreased with increasing COS supplementation, both reaching the maximum in the COS0.4 group and the minimum in the COS0 group (p < 0.05). The plica height and width of the FM group were significantly higher than those of the COS0 and COS1 groups (p < 0.05). In contrast, the trend of lamina propria thickness was opposite to that of plica height and width, as it was at a minimum in the COS0.4 group (p < 0.05). Lamina propria thickness was greatest in the COS0 group out of all the groups (p < 0.05).
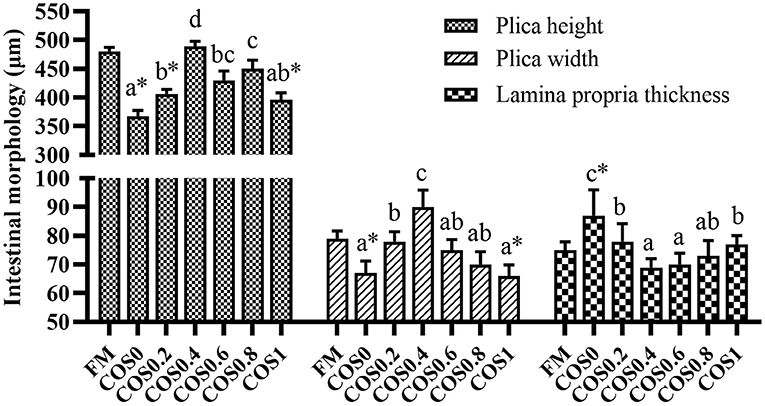
Figure 3. Intestinal morphology measurement of grouper(μm). Randomly selected 10 folds and lamina propria in each slice for measurement. Values are means ± SEM (n = 3). Values in the same row with different superscript letters mean significantly different among COS-containing groups (P < 0.05). The superscript * indicates that this COS-containing group is significantly different from FM group (P < 0.05).
Intestinal Microbiota Analysis
Richness, Diversity, and Composition of Microbiota
As shown in Figure 4, there are 1,161–1,380 OTUs in each group, and there are 1,445 core OTUs. The proportion of shared OTUs within each group (COS0, COS0.2, COS0.4, COS0.6, COS0.8, and COS1) was 53.16, 55.45, 53.14, 52.72, 53.03, 52.89, and 51.15%, respectively. As these results suggested that the intestinal microbiota of each group was similar, it was concluded that there was a stable microbiota core in the intestines of all tested groupers.
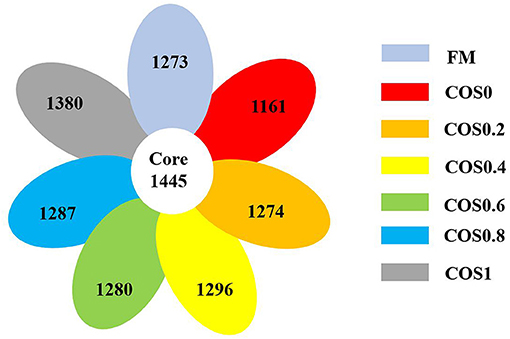
Figure 4. Shared OTUs analysis of intestinal microbiota in hybrid grouper fed with experimental diets for 8 weeks.
Figure 5 shows the alpha diversity indices of the intestinal microbiota. The sample saturation curves of each treatment group tended to be stable, indicating that the amount of sequencing had reached saturation. Additionally, the coverage was >99% for each treatment group, which suggested that the microbiome sequencing results reflected the gut microbiota composition of the hybrid groupers.
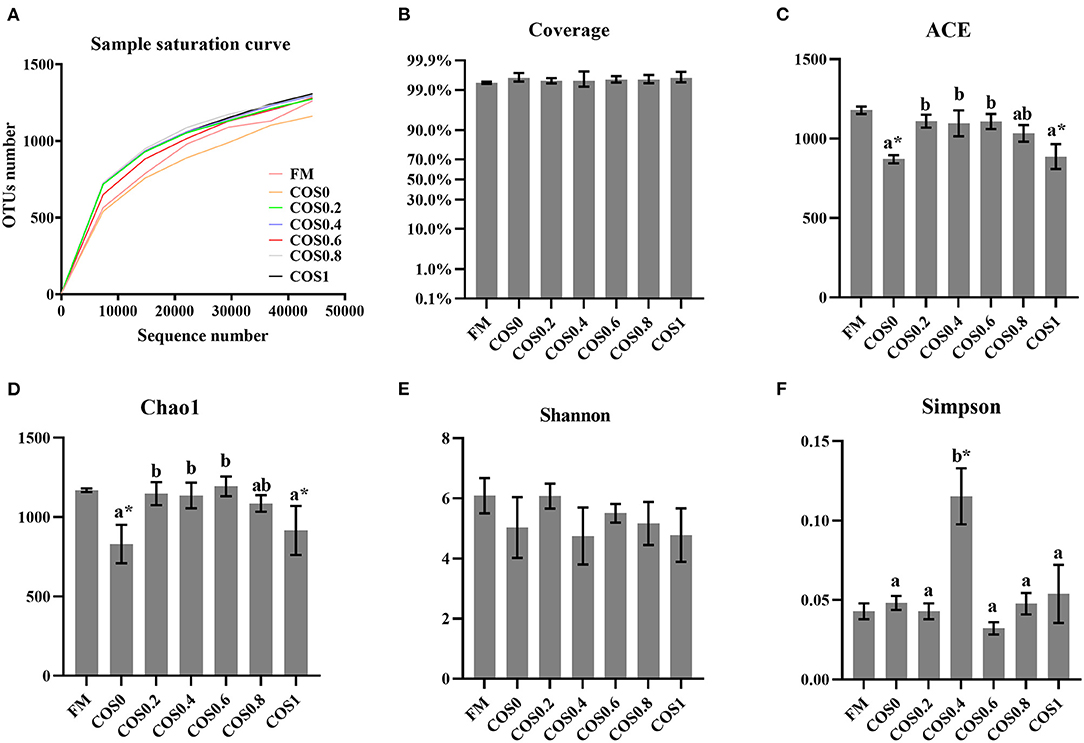
Figure 5. (A) Sample saturation curve analysis of intestinal microbiota in hybrid grouper fed with experimental diets for 8 weeks. (B–F) Alpha diversity indices of intestinal microbiota in hybrid grouper fed with experimental diets for 8 weeks. Values are means ± SEM (n = 3). Values in the same row with different superscript letters mean significantly different among COS-containing groups (P < 0.05). The superscript * indicates that this COS-containing group is significantly different from FM group (P < 0.05).
Among the alpha diversity indices, the ACE and Chao1 indices of the different groups both rose and then fell with increasing COS levels (p < 0.05), suggesting that microbiota richness was highest in the FM, COS0.4, and COS0.6 groups. There were no significant differences between the Shannon index values of the different groups (p > 0.05). However, the Simpson index was highest in the COS0.4 group, where the lowest diversity of microbiota was observed (p < 0.05).
As shown in Figure 6, there are clear similarities of the overall bacterial composition between treatments. The aggregations of the different groups of each treatment were relatively concentrated, suggesting that the microbiome had good reproducibility. The processing distance of each COS-containing group was similar (blue frame), indicating the bacterial composition of COS-containing groups. In addition, the layouts of the FM group (green frame) and the COS0 group (red frame) were clearly separated from the COS-containing groups. This phenomenon reflected the structural difference in bacterial composition between the groups that included COS supplementation and those that did not (FM and COS0).
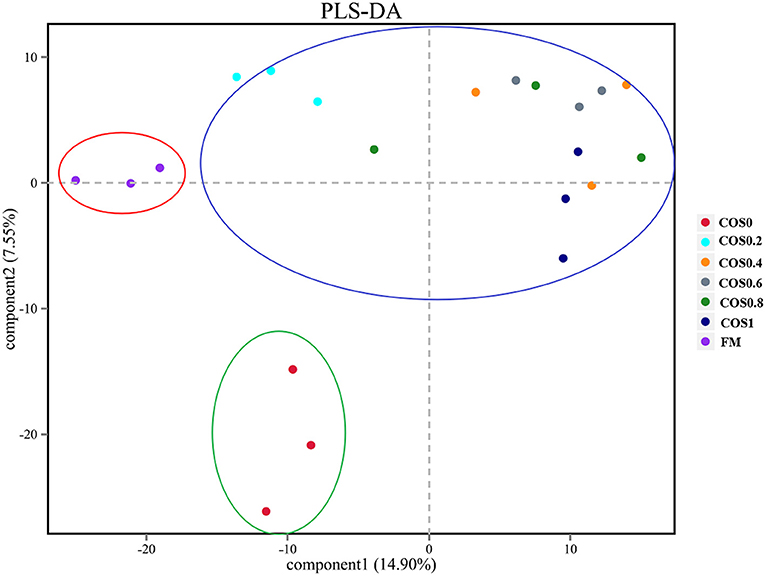
Figure 6. Comparison of overall bacterial composition between treatments by partial least squares discrimination analysis (PLS-DA) in grouper intestinal microbiota.
Community Analysis of Microbiota
Figure 7A displays the composition and relative abundance of the major bacterial phyla in the grouper intestinal microbiome. Firmicutes, Proteobacteria, Bacteroidetes, Actinobacteria, Acidobacteria, and Verrucomicrobia were the dominant bacteria at the phylum level. The relative abundance of Firmicutes, Bacteroidetes, and cyanobacteria in the COS-containing groups was higher than of the FM group, and it had an upward trend with increasing COS supplementation. The abundance of Chloroflexi and Rokubacteria showed a downward trend, with the high-COS groups (including COS0.6, COS0.8, and COS1) having significantly lower abundance than the low-COS and FM groups (p < 0.05). The abundance of Proteobacteria, Acidobacteria, and Gemmatimonadetes showed a relatively decreasing trend with increasing COS supplementation (p > 0.05).
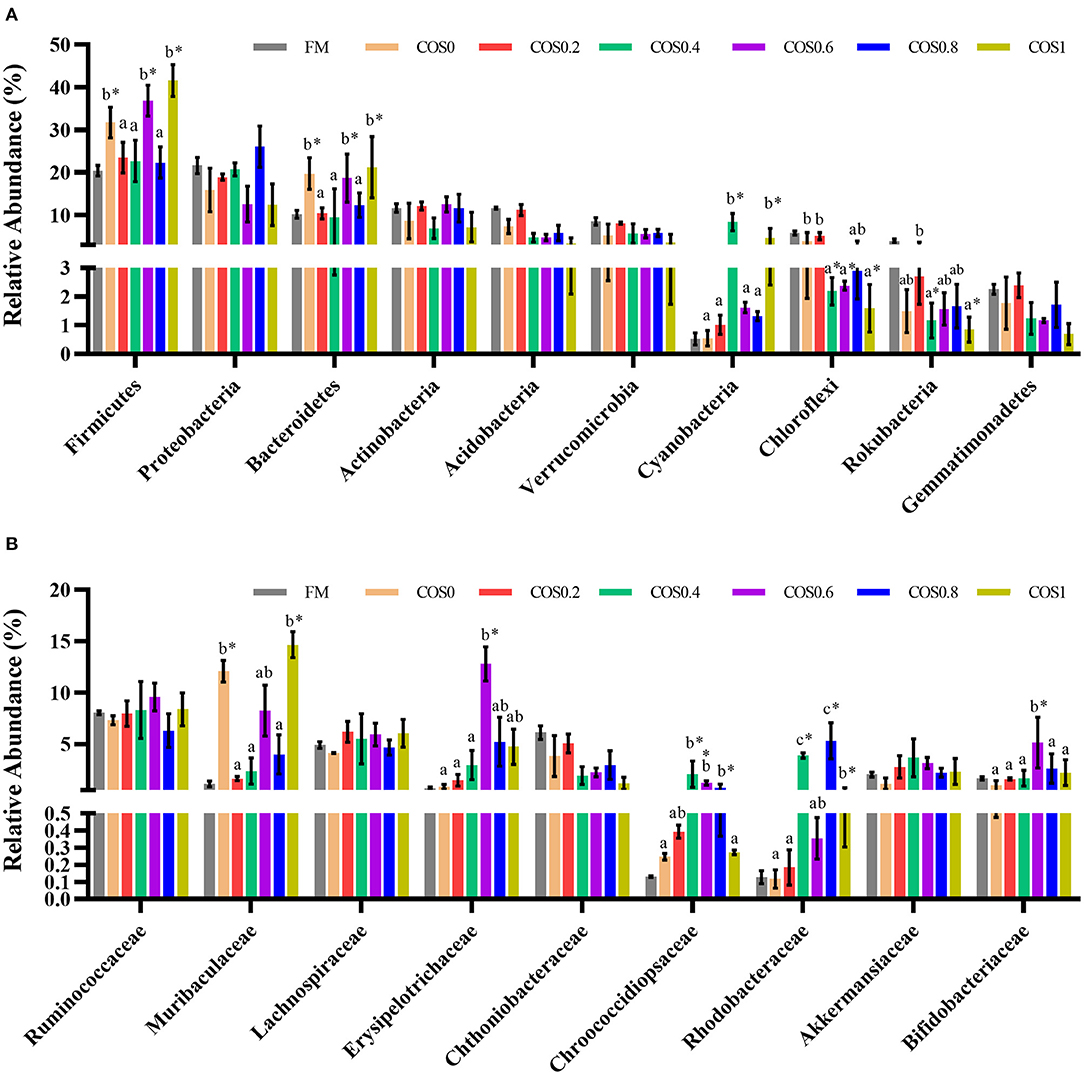
Figure 7. (A) Relative abundance of major bacterial phylum in grouper intestinal microbiota. (B) Relative abundance of major bacterial family in grouper intestinal microbiota. Values are means ± SEM (n = 3). Values in the same row with different superscript letters mean significantly different among COS-containing groups (P < 0.05). The superscript * indicates that this COS-containing group is significantly different from FM group (P < 0.05).
The relative abundance of the major bacterial families comprising the grouper intestinal microbiome is shown in Figure 7B. The dominant taxa at the family level were relatively similar across the treatment groups. There were no significant differences in the abundance of Ruminococcaceae, Lachnospiraceae, and Akkermansiaceae across the treatments (p > 0.05) but tended to increase first and then decrease. The abundance of Muribaculaceae in the COS0 and COS1 groups was significantly higher than that in the other groups, such as the FM group (p < 0.05). The abundance of Erysipelotrichaceae and Bifidobacteriaceae is highest in the COS0.6 group (p < 0.05), and it tended to increase first and then decrease in all the groups. The abundance of Chthoniobacteraceae showed a decreasing trend with increasing COS supplementation (p > 0.05). The abundance of Chroococcidiopsaceae and Rhodobacteraceae in the COS0.4 and COS0.8 groups is significantly higher than that in the other treatment groups (p < 0.05), and the abundance increased first and then decreased.
Serum and Intestinal Antioxidant Indices
Table 5 shows the serum and intestinal antioxidant indices of the hybrid grouper. In all the groups, the amount of GLU in the serum increased first and then decreased, and that in the COS0.6 group was significantly higher than that in the other groups (p < 0.05). The ALT level of the COS0.6 group was the lowest among all the groups, including such as the FM group (p < 0.05). AST was the lowest in the COS0.2 and COS0.8 groups (p < 0.05). There were no significant differences in TG among the groups (p > 0.05). CAT was significantly higher in the COS0.4 group than in the other groups, including such as the FM group (p < 0.05), whereas it was the lowest in the COS0 group (p < 0.05). The T-SOD level of the COS0.4 and COS0.6 groups was significantly higher than that of the other treatment groups (p < 0.05). MDA level was the highest in the COS0 group (p < 0.05), whereas the other groups showed no significant differences in MDA levels (p > 0.05).
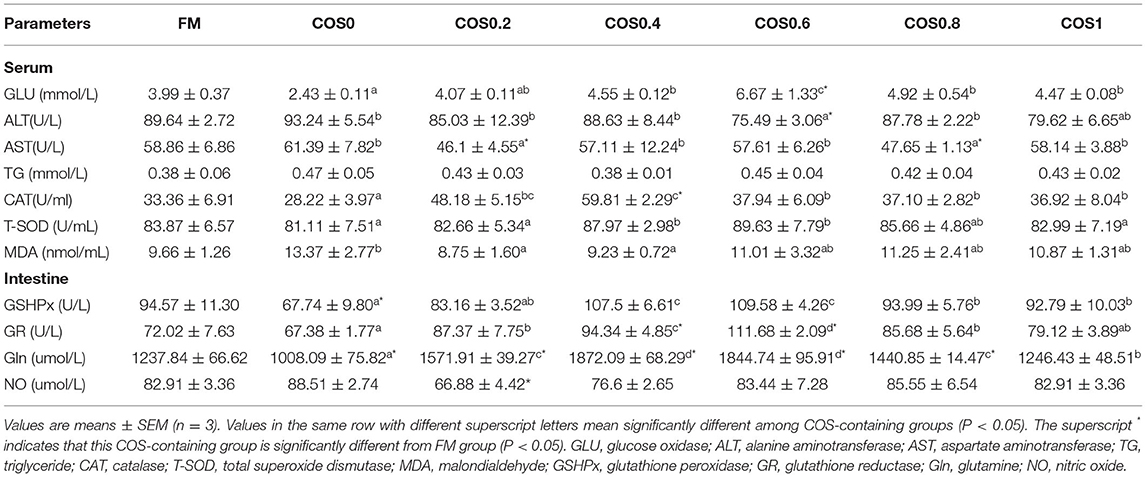
Table 5. Serum and intestinal antioxidant indices of hybrid grouper fed with experimental diets for 8 weeks.
In the intestinal tissue, GSHPx was lowest in the COS0 group when compared with that in all the other groups such as the FM group (p < 0.05); however, GSHPx was significantly higher in the COS0.4 and COS0.6 groups than in the other groups (p < 0.05). GR activity increased first and then decreased; the maximum value appeared in the COS0.6 group, and the lowest value appeared in the COS0 group (p < 0.05). Gln was significantly higher in the COS0.4 group than in all the other groups, such as the FM group (p < 0.05), whereas it was significantly lowest in the COS0 group (p < 0.05). There were no significant differences in NO across the COS-containing groups (p > 0.05), but the COS0.2 group had a significantly lower NO level than the FM group (p < 0.05).
Intestinal Non-specific Immunity Indices
As shown in Table 6, there are significant differences in the intestinal non-specific immunity indices between the hybrid grouper groups. The LYS (lysozyme) of the COS0.6 group was significantly higher than that of all other groups (p < 0.05), whereas the COS0 group had the least significant value (p < 0.05). The ACP (acid phosphatase) was highest in the COS0.4 group compared with that in all the other groups (p < 0.05), and it was least in the COS0 group (p < 0.05). C3 (complement protein 3) was lower in the FM group than in the COS-containing groups (p < 0.05), whereas it was highest in the COS0.4 group (p < 0.05). C4 (complement protein 4) was lowest in the FM group compared with that in all the other treatments, and it was significantly highest in the COS0.4 group compared with that in the other groups (p < 0.05). IgM (immunoglobulin M) increased first and then decreased, with the COS0.6 group having a significantly higher value than the other groups and the COS0 group having the least IgM (p < 0.05).
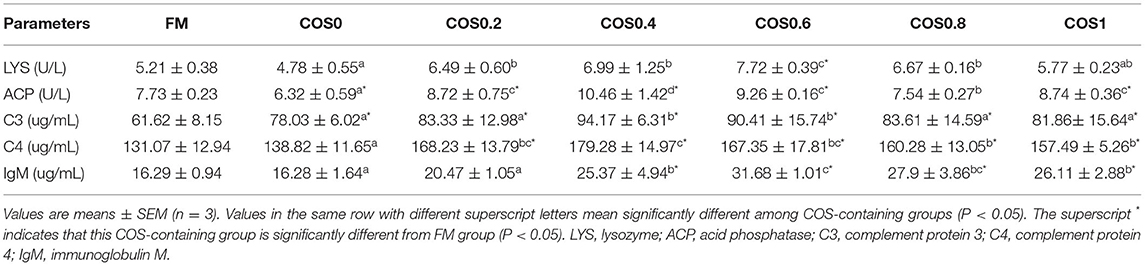
Table 6. Intestinal non-specific immunity indices of hybrid grouper fed with experimental diets for 8 weeks.
Intestinal Immunity Gene Expression
Intestinal immune gene expression is shown in Figure 8. The IL-1β mRNA level reached the highest significant value in the COS0 group (p < 0.05), whereas there was no significant difference in the other groups, such as the FM group (p > 0.05). The IL-10 mRNA level was significantly lower in the FM group than in the COS-containing groups (p < 0.05); however, there was no significant difference between the COS-containing groups (p > 0.05). There was no significant difference in MyD88 mRNA levels among the treatments (p > 0.05). The TLR22 mRNA level was upregulated in the COS0 group, and it was significantly higher than that in the other groups (p < 0.05); however, there was no significant difference in the FM group (p > 0.05). TGF-β mRNA levels tended to increase first and then decrease, with levels being significantly higher in the COS0.2 and COS0.4 groups than in the FM, COS0, and COS0.8 groups (p < 0.05). The mRNA expression of TNF-α showed a downward trend among the COS-containing groups. TNF-α mRNA was upregulated in the COS0 group, and it was significantly higher than that in the other treatment groups, such as the FM group (p < 0.05). The Nrf2 mRNA level was lowest in the COS0 group, whereas the Nrf2 mRNA expression in the COS0.4 group was upregulated and reached the highest value (p < 0.05).
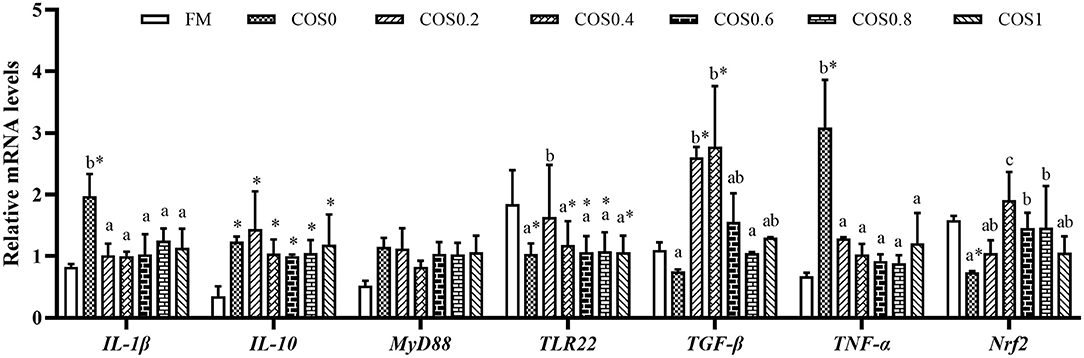
Figure 8. Intestinal immunity gene expression of hybrid grouper fed with experimental diets for 8 weeks. Values are means ± SEM (n = 3). Values in the same row with different superscript letters mean significantly different among COS-containing groups (P < 0.05). The superscript * indicates that this COS-containing group is significantly different from FM group (P < 0.05).
Challenge Test
Figure 9 shows the cumulative mortality of the groupers that were challenged with V. harveyi for 7 days. The grouper disease resistance was significantly different between the treatment groups post V. harveyi challenge. However, there were no significant differences in disease resistance between the FM and COS-containing groups (p > 0.05). Among the COS-containing groups, the cumulative mortality decreased first and then increased, with the highest mortality occurring in the COS0 group and the lowest occurring in the COS0.4 group (p < 0.05).
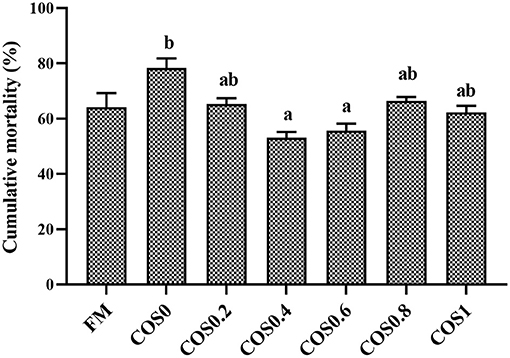
Figure 9. Cumulative mortality of experimental hybrid grouper challenged with Vibrio harveyi for 7 days. Values are means ± SEM (n = 3). Values in the same row with different superscript letters mean significantly different among COS-containing groups (P < 0.05).
Discussion
Generally, replacing fish meal with plant protein can adversely affect aquatic animals (Wu et al., 1999). For example, the substitution of fish meal with at least 34% CPC can hinder grouper growth and cause intestinal inflammation (Yin et al., 2018). The results of this study showed that FW, WG, and SGR had small values in the COS0 group, which was fed with an experimental diet, with CPC replacing fish meal. This suggests that plant protein replacement has an adverse effect on grouper growth, which may be caused by amino acid imbalance (Belghit et al., 2014; Skiba-Cassy et al., 2016). This is consistent with the result of this study, as the threonine and isoleucine contents of the COS-containing groups were lower than those of the FM group. Thus, the lack of dietary essential amino acids can adversely affect grouper growth. However, COS can have a growth-promoting effect on aquatic animals, such as hybrid tilapia (Oreochromis niloticus ♀ × Oreochromis aureus ♂) (Chubin et al., 2014), koi (Cyprinus carpio koi) (Lin et al., 2012), and Penaeus monodon (Jin et al., 2013). In this study, groupers in the COS0.4 group had higher FW, WG, and SGR values than those in the FM group. This illustrates that COS supplementation positively affected hybrid grouper growth, with an optimum effect occurring with COS supplementation of 0.45%. COS may have a growth-promoting effect, because it is fermented in the fish gut (Cha et al., 2008; Ring et al., 2010). The acidic substances produced from COS fermentation can lower the pH value in the gut and enhance digestive enzyme activity. This effect has been observed with mannose oligosaccharides, whose fermentation improved protease and lipase activities in crayfish (Cherax destructor Clark) (Sang et al., 2015). Therefore, oligosaccharides fermented in the intestine improve digestive ability and ultimately lead to better growth performance.
The intestine plays an important role in digestion and nutrient absorption in fish (Baker et al., 2014). Normal gut morphology, particularly the fold shape, is the basis for sufficient nutrient absorption and intestinal homeostasis. Optimizing the intestinal morphology can increase the gut absorption area, which is conducive to adequate nutrient absorption (Refstie et al., 2006; Geda et al., 2012). In this study, some undesirable changes occurred to the grouper gut morphology in the CPC-containing groups, such as fold breakage and morphological disorders. This may be explained by an imbalance in dietary amino acids caused by the replacement of fish meal with plant protein (Wanga et al., 2018). In carnivorous marine fish, larvae are sensitive to amino acid composition during the growth stage (Aragão et al., 2004). Dietary amino acids are mainly used to meet growth requirements and regulate fish body tissue construction. Thus, malnutrition caused by amino acid imbalance may lead to dysplasia in fish intestinal morphology (Yamamoto et al., 2012; Adriana et al., 2020). In this experiment, the fold height and width, and the lamina propria thickness were improved by the COS-containing treatments, especially in the COS0.2 and COS0.4 groups. These results were similar to those of an experiment conducted on turbot (Scophthalmus maximus) supplemented with mannan oligosaccharides (Bai et al., 2017). COS protects the gut by optimizing intestinal morphology and acting as a prebiotic. Feeding prebiotic supplements to fish can have a positive effect on enhancing intestinal morphology, as has been shown in Sparus aurata (Dimitroglou et al., 2010), juvenile pacu (Piaractus mesopotamicus) (Ricardo et al., 2014), Oncorhynchus mykiss (Dimitroglou et al., 2009), and larval cobia (Rachycentron canadum) (Salze et al., 2008). High-affinity ligands, prebiotics such as oligosaccharides, can provide binding sites that specifically match bacterial lectins, which could block the binding between pathogens and intestinal mucosal epithelial cells. In addition, prebiotics can promote the proliferation of beneficial bacteria, allowing them to attach more widely to the surface of the intestinal wall, thereby reducing the contact of conditional pathogens with the intestinal wall and protecting the intestines (Ofek et al., 1977; Dimitroglou et al., 2009; Rajendran et al., 2017).
The gut ameliorating effect of COS is closely related to its influence on the intestinal microbiota. The intestinal microbiota plays an indispensable role in maintaining fish intestinal homeostasis. There is lower microbiota diversity in the gut of fish than in that of terrestrial animals. Major bacterial species that are stable after colonization differ depending on fish feeding habits (Coscas et al., 2010; Tarnecki et al., 2017). Furthermore, the gut microbiota community structure is affected by the fish cultivation environment and available dietary nutrients (Li et al., 2018a). Related studies have reported that replacing fish meal with plant protein changes the fish intestinal microbiota community, and a high proportion of fish meal replacement may have adverse effects on the fish microbiota (Gy et al., 2020; Shen et al., 2020). However, oligosaccharide supplementation can adjust the balance between probiotics and conditional pathogens, thereby balancing the intestinal microflora (Mussatto and Mancilha, 2007). In this study, the ACE index and Chao1 index increased first and then decreased with an increase in COS supplementation. Therefore, it can be inferred that COS can enhance the abundance of intestinal microbiota in this grouper species. The comparison of overall bacterial composition revealed that the microbiota in the COS-containing groups were analogous or closely related. In addition, there were clear differences in the microbiota community between the treatments without COS supplementation (FM and COS0) and the COS-containing groups. It is worth noting that oligosaccharide supplementation is only beneficial when a moderate amount is provided. Excess oligosaccharides are excessively fermented by intestinal microorganisms, causing over-proliferation and intestinal microenvironment imbalance. This phenomenon causes the digestive capacity of fish intestines to decrease. In contrast, functional oligosaccharides have suboptimal physical and chemical properties, such as high viscosity and strong hygroscopicity (Mussatto and Mancilha, 2007). Therefore, high oligosaccharide supplementation lowers the palatability of feed, which is not conducive to fish growth; however, within a reasonable range of supplementation, oligosaccharides play a positive role in intestinal functioning. This may explain why 0.4–0.6% COS supplementation was optimal in this experiment.
The results indicated that the relative abundance of beneficial bacteria species, such as Firmicutes and Bacteroides, at the phylum level, and Lactobacteriaceae and Bifidobacterium, at the family level, increased among the COS-containing groups. Related studies have shown that Firmicutes and Bacteroides play important roles in the healthy physiological and metabolic activities of the host. For example, they can produce short-chain fatty acids by fermenting indigestible carbohydrates to resist pathogenic bacteria and further promote intestinal health of the host (Lara et al., 2017; Waite et al., 2017). In addition, beneficial bacteria that colonize the gut, such as Lactobacteriaceae and Bifidobacterium, can secrete enzymes that open β-1,4 glycosidic bonds and then use COS to produce succinic acid and lactic acid, further lowering the pH and maintaining gut stability (Liu et al., 2008). Interestingly, the abundance of conditional pathogens, such as Proteobacteria (phylum level) and Erysipelotrichaceae (family level), also increased with increasing COS supplementation. Proteobacteria is the largest phylum containing many conditional pathogens, such as Escherichia coli, Salmonella, Vibriocholerae, and Helicobacterpylori (Waite et al., 2017), and Erysipelotrichaceae is closely related to inflammation-related enteropathy and metabolic disorders (Kaakoush, 2015). The simultaneous increase in the abundance of probiotics and conditional pathogens in this study may have been related to the indigenous bacterial composition of the water environment (Cornejo-Granados et al., 2018). Despite this simultaneous increase, the physiology of the groupers, such as their growth and gut morphology, was not adversely affected. This suggests that COS supplementation enhances grouper intestinal homeostasis and promotes stability of the microbiota habitat, as even the increase in conditional pathogen abundance did not cause intestinal disorders. Mahious et al. (2006) found that 2% oligofructose supplementation promoted intestinal health in turbot (Psetta maxima), similar to prebiotics. Furthermore, Qingsen et al. (2016) explored dietary fucoidan supplementation as a prebiotic that can promote intestinal health. The findings suggested that dietary prebiotic supplementation reduced host antigen load and inflammatory response by maintaining a balanced composition of intestinal microbiota, which may be achieved by reducing serum lipopolysaccharide-binding protein levels.
Antioxidant function is an important means of maintaining homeostasis in fish (Pérez-Jiménez et al., 2014). Previous studies have found that dietary fish meal replacement with plant proteins hinders the antioxidant functions in marine fish, such as grouper (Yin et al., 2018), golden pompano (Trachinotus ovatus) (Shen et al., 2020), and totoaba juveniles (Totoaba macdonaldi). The liver is the main organ involved in TG metabolism. When abnormal hepatic cells cause TG metabolism disorder, serum TG content increases and further affects energy metabolism (Wu et al., 2011). Lipid peroxidation causes cell damage and produces MDA as the final oxidation product. Thus, the MDA content in the serum can indirectly reflect the degree of cell damage (Box et al., 2020). When the FM group was compared with the COS0 group, it was found that the COS0 group contained higher serum TG and MDA levels. Therefore, the COS0 group had a certain degree of oxidation disorder. Glucose is the main source of energy in fish, and increased GLU activity facilitates energy utilization and physiological efficiency (Li et al., 2020). As one of the key enzymes in the biodefense system, CAT breaks down hydrogen peroxide into water and oxygen, preventing cells from being damaged by hydrogen peroxide (Cai et al., 2017). T-SOD is an important antioxidant enzyme in fish that effectively scavenges free radicals and reduces the production of oxidative stress (Yu et al., 2011). ALT and AST are two important transaminases that play vital roles in the metabolism of amino acids and the interconversion of proteins, lipids, and glucose; serum ALT and AST levels can reflect liver function impairment (Wu et al., 2020). Glutathione is an important antioxidant, and Gln plays a crucial role in glutathione synthesis. GSHPx and GR are two important peroxidases that catalyze glutathione conversion and reduce toxic peroxides to non-toxic hydroxyl compounds, thus protecting the cell membrane from interference and damage by peroxides (Bilzer et al., 2002; Yan and Xiao, 2006; Yin et al., 2018). In this experiment, serum GLU, CAT, and T-SOD activities increased in the COS-containing groups with increasing COS supplementation levels. Conversely, ALT and AST activities decreased. In the gut, the activities of GSHPx, GR, and Gln tended to increase first and then decrease, reaching an optimum in the COS0.4 or the COS0.6 group. These results suggest that COS supplementation can alleviate the antioxidant burden induced by plant protein substitution, thereby refining the antioxidant capacity of this grouper species. Previous studies have suggested that, in addition to its antioxidant properties, COS has a variety of biological activities such as scavenging free radicals and inducing the expression of antioxidant enzymes and genes (Joodi et al., 2011; Sun et al., 2011; Vieira et al., 2020). The amino, primary, and secondary hydroxyl groups in the COS molecule are the structural bases for its antioxidant function (Tao et al., 2007). The molecular structure of COS contains reducing end-groups, whose reducing properties can scavenge or inhibit free radicals, thus maintaining the relative antioxidant stability of the internal environment (Xie et al., 2001). Furthermore, the antioxidant potency of prebiotics is related to their molecular weight and solubility, which may be influenced by differences in the species and habitat. Therefore, the antioxidant effects of prebiotics in aquaculture deserve further research attention (Palframan et al., 2002; Gomez et al., 2010; Mei et al., 2011).
As vertebrates, fish have a relatively well-developed immune system. The non-specific immune defense mechanism is the first barrier and the main protector of fish against pathogens (Alonso and Leong, 2002). The main mechanisms of non-specific immune response include phagocytic activity, respiratory bursts, complement system functioning, and production of lysozyme and cytokines (Little et al., 2005). ACP, a major indicator of fish growth and immune function, plays an important role in cellular regulation and nutrient transport. In addition, ACP is closely related to lysozyme, a cationic protein synthesized in the liver. It is responsible for bacteriolytic, regulatory, and immune responses, as well as antimicrobial activity (Saurabh and Sahoo, 2010; Gudmundsdottir et al., 2014). C3 and C4 are important components of the immune complement system. They can kill pathogens by making small holes in the pathogen cell membrane, thus supplementing the role of antibodies (Cuesta et al., 2016). IgM is an important immune protein that has powerful effects of bactericidal activity, complement activation, immune regulation, and agglutination (Krogdahl et al., 2015). In this experiment, the activities of LYS and ACP showed an upward trend with increased COS supplementation levels. In addition, the C3, C4, and IgM contents in the gut tissue increased first and then decreased. This result is similar to that obtained for Sparus aurata and Dicentrarchus labrax (Carbone and Faggio, 2016), suggesting that COS supplementation improves grouper health by enhancing lysozyme activity and the complement system.
Previous studies have shown that replacing fish meal with plant protein can induce intestinal inflammatory factor expression, activate inflammatory pathways, and ultimately cause intestinal inflammation in marine fish. This may be related to the unreasonable composition of amino acids and the presence of anti-nutritional factors in plant proteins (Yin et al., 2018, 2020). IL-1β is an important mediator of inflammation in infection response (Holland et al., 2002). TNF-α is also one of the main mediators of inflammation (Hideaki et al., 2005). The results of this study showed that the expression of IL-1β and TNF-α was upregulated in the COS0 group, which suggests that dietary fish meal replacement with CPC triggered an inflammatory response in the grouper gut. As an important anti-inflammatory cytokine, IL-10 participates in inflammatory response and relieves inflammation. It has immunomodulatory effects on teleost fish (Wei et al., 2013). Upregulated IL-10 expression helps to alleviate inflammation and inhibits T cells from producing excessive IL-6, IL-1β, and other inflammatory cytokines, thereby preventing excessive immune responses from harming the body (Xiaoyi et al., 2008). TLRs play vital roles in the immune system and are widely present in marine fish. TLR22 is an important inflammatory regulator because of its recognition of dsRNA, recruitment of TRIF signaling molecules, and activation of the I-interferon response (Sahoo et al., 2015). TGF-β is an important regulatory hub involved in signal transduction (at the stage of transcription phosphorylation) in cellular inflammatory pathways (Liu et al., 2017). Nrf2 is an important transcription factor that regulates the oxidative stress response of cells; it is also a central regulator that maintains cell redox homeostasis (Hui and Yang, 2018). In this experiment, the expression of TLR22 and TGF-β tended to be upregulated first and then be downregulated, and they had a high expression level in the COS0.2 and COS0.4 groups. This indicates that the intestinal inflammatory response of the groupers was regulated and activated by COS supplementation. Moreover, IL-10 expression level was higher in the COS-containing groups than in the FM group, suggesting that COS supplementation alleviates intestinal inflammation in the groupers. This aligns with the observed improvement in intestinal morphology. In addition, Nrf2 expression was upregulated as COS supplementation was increased. This shows that COS supplementation reduced oxidative damage to the grouper intestinal cells, maintaining a steady state of the gut environment. The conclusions of this study are consistent with those of a study carried out on carp (Cyprinus carpio L.), which found that COS supplementation can increase their antioxidant and immune responses (Dautremepuits et al., 2004). The results of this study showed that the resistance of the groupers against V. harveyi was enhanced with increased dietary COS supplementation. This may be because oligosaccharides are positively charged polymer substances. The structure of their positively charged and polymerized molecules is the basis for their antibacterial and bactericidal abilities (Anderson and Siwicki, 1994; Talpur et al., 2014). In summary, oligosaccharide prebiotics are immunogenic and stimulate immune responses of the body (Ghosh and Mehla, 2012; Si et al., 2017), thereby improving non-specific immunity (Maqsood et al., 2010; Huo et al., 2015), anti-inflammatory activity (Azuma et al., 2015), and disease resistance (Zhang et al., 2012) in fish species.
Conclusion
The results of this study demonstrated that chitosan oligosaccharide supplementation can improve the growth, intestinal morphology, microbiota composition, antioxidant capacity, and immune response of hybrid groupers under the adverse effects of fishmeal replacement with CPC. Hence, COS can be used as a potential enhancer to fortify grouper fed a low-fishmeal diet.
Data Availability Statement
The datasets presented in this study can be found in online repositories. The names of the repository/repositories and accession number(s) can be found at: NCBI (accession: PRJNA728963).
Ethics Statement
The animal study was reviewed and approved by Animal Care and Ethics Committee of Guangdong Ocean University.
Author Contributions
HL, BT, and XD were involved in conceptualization. GC, BY, QY, SC, and SZ designed the experiments. BY provided the necessary assistance. GC were involved in data analysis and writing of the original draft. GC and HL reviewed and edited the manuscript. All the authors approved the final version of the manuscript for submission.
Funding
This work was supported by the National Key RD Program of China (2019YFD0900200) and the National Natural Science Foundation of China (No.31772864), Natural Science Foundation of Guangdong Province (2018A030313154; 2020A1515011129).
Conflict of Interest
The authors declare that the research was conducted in the absence of any commercial or financial relationships that could be construed as a potential conflict of interest.
Publisher's Note
All claims expressed in this article are solely those of the authors and do not necessarily represent those of their affiliated organizations, or those of the publisher, the editors and the reviewers. Any product that may be evaluated in this article, or claim that may be made by its manufacturer, is not guaranteed or endorsed by the publisher.
Acknowledgments
We thank Bozhi Huili Biotechnology Co., Ltd. for providing chitosan oligosaccharide.
References
Adriana, M., Tania, G., Villalobos, Y. D., Hugo, B., Htoo, J. K., González-Vega, J. C., et al. (2020). Dietary protein-bound or free amino acids differently affect intestinal morphology, gene expression of amino acid transporters, and serum amino acids of pigs exposed to heat stress. J. Anim. Ence 98:skaa056. doi: 10.1093/jas/skaa056
Alonso, M., and Leong, J. A. (2002). Suppressive subtraction libraries to identify interferon-inducible genes in fish. Marine Biotech. 4, 74–80. doi: 10.1007/s10126-001-0001-9
Anderson, D. P., and Siwicki, A. K. (1994). Duration of protection against aeromonas salmonicida in brook trout immunostimulated with glucan or chitosan by injection or immersion. Progr. Fish-Culturist 56, 258–261. doi: 10.1577/1548-8640(1994)056<0258:DOPAAS>2.3.CO;2
Aragão, C., Concei, O. L., Dinis, M. T., and Fyhn, H. J. (2004). Amino acid pools of rotifers and Artemia under different conditions: nutritional implications for fish larvae. Aquaculture 234, 429–445. doi: 10.1016/j.aquaculture.2004.01.025
Aßhauer, K. P., Wemheuer, B., Daniel, R., and Meinicke, P. (2015). Tax4Fun: predicting functional profiles from metagenomic 16S rRNA data. Bioinformatics 31, 2882–2884. doi: 10.1093/bioinformatics/btv287
Ayiku, S., Jianfei, S., Tan, B.-P., Dong, X.-H., and Liu, H.-Y. (2020). Effects of dietary yeast culture on shrimp growth, immune response, intestinal health and disease resistance against Vibrio harveyi. Fish Shellfish Immunol. 102, 286–295. doi: 10.1016/j.fsi.2020.04.036
Azuma, K., Osaki, T., Minami, S., and Okamoto, Y. (2015). Anticancer and anti-inflammatory properties of chitin and chitosan oligosaccharides. J. Funct. Biomater. 6, 33–49. doi: 10.3390/jfb,6010033
Bai, N., Gu, M., Xu, X., Xu, B., and Krogdahl, A. (2017). Protective effects of mannan oligosaccharides on turbot Scophthalmus maximus suffering from soy enteropathy. Aquaculture 476, 141–151. doi: 10.1016/j.aquaculture.2017.04.005
Baker, R., Buckland, A., and Sheaves, M. (2014). Fish gut content analysis: robust measures of diet. Fish Fish. 15, 170–177. doi: 10.1111/faf.12026
Belghit, I., Skiba-Cassy, S., Geurden, I., Dias, K., Surget, A., Kaushik, S., et al. (2014). Dietary methionine availability affects the main factors involved in muscle protein turnover in rainbow trout (Oncorhynchus mykiss). Br. J. Nutr. 112, 493–503. doi: 10.1017/S0007114514001226
Bilzer, M., Baron, A., Schauer, R., Steib, C., and Ebensberger, S. (2002). Glutathione treatment protects the rat liver against injury after warm ischemia and kupffer cell activation. Digest. –Basel London 66, 49–57. doi: 10.1159/000064415
Bokulich, N. A., Subramanian, S., Faith, J. J., Gevers, D., Gordon, J. I., Knight, R., et al. (2013). Quality-filtering vastly improves diversity estimates from Illumina amplicon sequencing. Nat. Methods 10, 57–59. doi: 10.1038/nmeth.2276
Box, A., Capó, X., Tejada, S., Sureda, A., Mejías, L., and Valencia, J. M. (2020). Perkinsus mediterraneus infection induces oxidative stress in the mollusc Mimachlamys varia. J. Fish Dis. 43, 1–7. doi: 10.1111/jfd.13085
Cai, H. R., Yang, Q. H., Tan, B. P., Dong, X. H., Chi, S. Y., Liu, H. Y., et al. (2017). Effects of dietary manganese source and supplemental levels on growth performance, antioxidant enzymes activities, tissue Mn concentrations and cytosolic manganese superoxide dismutase (cMnSOD) mRNA expression level of juvenile Litopenaeus vannamei. Aquacult. Nutri. 23, 603–610. doi: 10.1111/anu.12427
Carbone, D., and Faggio, C. (2016). Importance of prebiotics in aquaculture as immunostimulants. effects on immune system of Sparus aurata and Dicentrarchus labrax. Fish Shellfish Immunol. 54, 172–178. doi: 10.1016/j.fsi.2016.04.011
Carter, C., and Hauler, R. (2000). Fish meal replacement by plant meals in extruded feeds for Atlantic salmon, Salmo salar L. Aquaculture 185, 299–311. doi: 10.1016/S0044-8486(99)00353-1
Cha, S. H., Lee, J. S., Song, C., Lee, K. J., and Jeon, Y. J. (2008). Effects of chitosan-coated diet on improving water quality and innate immunity in the olive flounder, Paralichthys olivaceus. Aquaculture 278, 110–118. doi: 10.1016/j.aquaculture.2008.01.025
Chen, G., Yin, B., Liu, H., Tan, B., Dong, X., Yang, Q., et al. (2020). Effects of fishmeal replacement with cottonseed protein concentrate on growth, digestive proteinase, intestinal morphology and microflora in pearl gentian grouper (♀ Epinephelus fuscoguttatus×♂ Epinepheluslanceolatu). Aquac. Res. 51, 2870–2884. doi: 10.1111/are.14626
Chen, J., Zhou, X.-Q., Feng, L., Liu, Y., and Jiang, J. (2009). Effects of glutamine on hydrogen peroxide-induced oxidative damage in intestinal epithelial cells of Jian carp (Cyprinus carpio var. Jian). Aquaculture 288, 285–289. doi: 10.1016/j.aquaculture.2008.10.053
Chubin, Q., Yuting, Z., Wenshu, L., Li, X., and Yang, Y. (2014). Effects of chito-oligosaccharides supplementation on growth performance, intestinal cytokine expression, autochthonous gut bacteria and disease resistance in hybrid tilapia Oreochromis niloticus ♀ × Oreochromis aureus ♂. Fish Shellfish Immunol. 40, 267–274. doi: 10.1016/j.fsi.2014.07.010
Cornejo-Granados, F., Gallardo-Becerra, L., Leonardo-Reza, M., Ochoa-Romo, J. P., and Ochoa-Leyva, A. (2018). A meta-analysis reveals the environmental and host factors shaping the structure and function of the shrimp microbiota. PeerJ. 6:e5382. doi: 10.7717/peerj.5382
Coscas, G., Quentel, G., Pinon, F., and Soubrane, G. (2010). The ultrastructure of the intestinal epithelium in fishes with different types of feeding. J. Fish Biol. 44, 181–193. doi: 10.1111/j.1095-8649.1994.tb01197.x
Cuesta, A., Brinchmann, M., Morcillo, F., Patricia, C., Hector, E., and Maria, A. (2016). Differential proteome profile of skin mucus of gilthead seabream (Sparus aurata) after probiotic intake and/or overcrowding stress. J. Proteomics 132, 41–50. doi: 10.1016/j.jprot.2015.11.017
Cui, L., Xu, W., Ai, Q., Wang, D., and Mai, K. (2013). Effects of dietary chitosan oligosaccharide complex with rare earth on growth performance and innate immune response of turbot, S cophthalmus maximus L. Aquac. Res. 44, 683–690. doi: 10.1111/j.1365-2109.2011.03072.x
Daniel, N. (2018). A review on replacing fish meal in aqua feeds using plant protein sources. Int. J. Fish. Aquat. Stud 6, 164–179. Available online at: https://www.researchgate.net/publication/324006059
Dautremepuits, C., Betoulle, S., Paris-Palacios, S., and Vernet, G. (2004). Humoral immune factors modulated by copper and chitosan in healthy or parasitised carp (Cyprinus carpio L.) by Ptychobothrium sp. (Cestoda). Aqua. Toxicol. 68, 325–338. doi: 10.1016/j.aquatox.2004.04.003
Dimitroglou, A., Merrifield, D. L., Moate, R., Davies, S. J., Spring, P., Sweetman, J., et al. (2009). Dietary mannan oligosaccharide supplementation modulates intestinal microbial ecology and improves gut morphology of rainbow trout, Oncorhynchus mykiss (Walbaum). J. Anim. ence 87, 3226–3234. doi: 10.2527/jas.2008-1428
Dimitroglou, A., Merrifield, D. L., Spring, P., Sweetman, J., Moate, R., and Davies, S. J. (2010). Effects of mannan oligosaccharide (MOS) supplementation on growth performance, feed utilisation, intestinal histology and gut microbiota of gilthead sea bream (Sparus aurata). Aquaculture 300, 182–188. doi: 10.1016/j.aquaculture.2010.01.015
Dvinge, H., and Bertone, P. (2009). HTqPCR: High-throughput analysis and visualization of quantitative real-time PCR data in R. Bioinformatics 25, 3325–3326. doi: 10.1093/bioinformatics/btp578
Edgar, R. C. (2013). UPARSE: highly accurate OTU sequences from microbial amplicon reads. Nat. Methods 10:996. doi: 10.1038/nmeth.2604
Geda, F., Rekecki, A., Decostere, A., Bossier, P., Wuyts, B., Kalmar, I. D., et al. (2012). Changes in intestinal morphology and amino acid catabolism in common carp at mildly elevated temperature as affected by dietary mannanoligosaccharides. Anim. Feed Sci. Technol. 178, 95–102. doi: 10.1016/j.anifeedsci.2012.09.008
Ghosh, S., and Mehla, R. K. (2012). Influence of dietary supplementation of prebiotics (mannanoligosaccharide) on the performance of crossbred calves. Trop. Anim. Health Prod. 44, 617–622. doi: 10.1007/s11250-011-9944-8
Gomez, E., Tuohy, K. M., Gibson, G. R., Klinder, A., and Costabile, A. (2010). In vitro evaluation of the fermentation properties and potential prebiotic activity of Agave fructans. J. Appl. Microbiol. 108, 2114–2121. doi: 10.1111/j.1365-2672.2009.04617.x
Gudmundsdottir, B. K., Gudmundsdottir, S., and Magnadottir, B. (2014). Yersiniosis in Atlantic cod, Gadus morhua (L.), characterization of the infective strain and host reactions. J. Fish Dis. 37, 511–519. doi: 10.1111/jfd.12139
Gy, A., Xdab, C., Qyab, C., Scab, C., Hlab, C., Hzb, D., et al. (2020). Dietary replacement of fish meal with peanut meal in juvenile hybrid grouper (Epinephelus fuscoguttatus ♀ × Epinephelus lanceolatus ♂): Growth performance, immune response and intestinal microbiota. Aquac. Rep. 17:100327. doi: 10.1016/j.aqrep.2020.100327
Hardy, R. W., and Tacon, A. G. (2002). Fish meal: historical uses, production trends and future outlook for sustainable supplies. Respon. Marine Aquacult. 1, 311–325. doi: 10.1079/9780851996042.0311
Hardy, R. W. (2006). Worldwide fish meal production outlook and the use of alternative protein meals for aquaculture. Avances en Nutrición Acuicola 15–17.
Hideaki, K., Shi-Ichi, H., Shin, M., and Lufen, M. (2005). Reactive oxygen species promote TNFα-induced death and sustained JNK activation by inhibiting MAP kinase phosphatases. Cell 5, 649–661. doi: 10.1016/j.cell.2004.12.041
Holland, J. W., Pottinger, T. G., and Secombes, C. J. (2002). Recombinant interleukin-1beta activates the hypothalamic-pituitary-interrenal axis in rainbow trout, Oncorhynchus mykiss. J. Endocrinol. 175, 261–267. doi: 10.1677/joe.0.1750261
Hui, L. I., and Yang, L. (2018). Molecular regulatory mechanism of Nrf2 antioxidant. Chin. J. Bioinform. 16, 1–6. doi: 10.3969/j.issn.1672-5565.201708001
Huo, P., Pan, J., Han, Y., Su, P., and Jiang, Z. (2015). Effects of alginic acid oligosaccharides on growth performance, hematological indexes and non-specific immunity of juvenile turbot (Scophthalmus maximus). J. Guangdong Ocean Univ. 35, 10–16. doi: 10.3969/j.issn.1673-9159.2015.04.002
Jin, N., Hei-Zhao, L., Shi-Gui, J., Xu, C., and Kai-Chang, W. (2013). Comparison of effect of chitin, chitosan, chitosan oligosaccharide and N-acetyl-d-glucosamine on growth performance, antioxidant defenses and oxidative stress status of Penaeus monodon. Aquaculture 372, 1–8. doi: 10.1016/j.aquaculture.2012.10.021
Joodi, G., Ansari, N., and Khodagholi, F. (2011). Chitooligosaccharide-mediated neuroprotection is associated with modulation of Hsps expression and reduction of MAPK phosphorylation. Int. J. Biol. Macromol. 48, 726–735. doi: 10.1016/j.ijbiomac.2011.02.011
Kaakoush, N. O. (2015). Insights into the role of erysipelotrichaceae in the human host. Front. Cell. Infect. Microbiol. 5:84. doi: 10.3389/fcimb.2015.00084
Kong, X., Zhou, X., Lian, G., Blachier, F., Liu, G., Tan, B., et al. (2014). Dietary supplementation with chitooligosaccharides alters gut microbiota and modifies intestinal luminal metabolites in weaned Huanjiang mini-piglets. Livest. Sci. 160, 97–101. doi: 10.1016/j.livsci.2013.11.023
Krogdahl, A., Bakke-McKellep, A. M., Roed, K. H., and Baeverfjord, G. (2015). Feeding Atlantic salmon Salmo salar L. soybean products: effects on disease resistance (furunculosis), and lysozyme and IgM levels in the intestinal mucosa. Aquacult. Nutri. 6, 77–84. doi: 10.1046/j.1365-2095.2000.00129.x
Lara, C., Romina, M., Barbara, F., and Nicol,ò, M. (2017). Impact of Omega-3 fatty acids on the gut microbiota. Int. J. Molecul. ences 18:2645. doi: 10.3390/ijms18122645
Li, E., Xu, C., Wang, X., Wang, S., Zhao, Q., Zhang, M., et al. (2018a). Gut microbiota and its modulation for healthy farming of pacific white shrimp Litopenaeus vannamei. Rev. Fish. ence Aquacult. 26, 381–399. doi: 10.1080/23308249.2018.1440530
Li, S., Sang, C., Zhang, J., Li, Z., and Chen, N. (2018b). Molecular cloning, expression profiling of adipose triglyceride lipase (ATGL) and forkhead box O1 (FoxO1), and effects of dietary carbohydrate level on their expression in hybrid grouper (Epinephelus fuscoguttatus♀ × E. lanceolatus♂). Aquaculture 492, 103–112. doi: 10.1016/j.aquaculture.2018.03.062
Li, X., Piao, X., Kim, S., Liu, P., Wang, L., Shen, Y., et al. (2007). Effects of chito-oligosaccharide supplementation on performance, nutrient digestibility, and serum composition in broiler chickens. Poult. Sci. 86, 1107–1114. doi: 10.1093/ps/86.6.1107
Li, X., Zheng, S., Han, T., Song, F., and Wu, G. (2020). Effects of dietary protein intake on the oxidation of glutamate, glutamine, glucose and palmitate in tissues of largemouth bass (Micropterus salmoides). Amino Acids 52, 1491–1503. doi: 10.1007/s00726-020-02907-3
Liaqat, F., and Eltem, R. (2018). Chitooligosaccharides and their biological activities: a comprehensive review. Carbohydr. Polym. 184, 243–259. doi: 10.1016/j.carbpol.2017.12.067
Lin, S., Mao, S., Guan, Y., Luo, L., Luo, L., and Pan, Y. (2012). Effects of dietary chitosan oligosaccharides and Bacillus coagulans on the growth, innate immunity and resistance of koi (Cyprinus carpio koi). Aquaculture 342, 36–41. doi: 10.1016/j.aquaculture.2012.02.009
Little, T. J., Hultmark, D., and Read, A. F. (2005). Invertebrate immunity and the limits of mechanistic immunology. Nat. Immunol. 6, 651–654. doi: 10.1038/ni1219
Liu, B., Xu, L., Ge, X., Xie, J., Xu, P., Zhou, Q., et al. (2013). Effects of mannan oligosaccharide on the physiological responses, HSP70 gene expression and disease resistance of Allogynogenetic crucian carp (Carassius auratus gibelio) under Aeromonas hydrophila infection. Fish Shellfish Immunol. 34, 1395–1403. doi: 10.1016/j.fsi.2013.02.028
Liu, J. X., Xu, Q. H., Li, S., Yu, X. D., Liu, W. Y., Ouyang, G., et al. (2017). Transcriptional factors Eaf1/2 inhibit endoderm and mesoderm formation via suppressing TGF-β signaling. Biochimica et Biophysica Acta (BBA)— Gene Regul. Mechan.1860, 1103–1116. doi: 10.1016/j.bbagrm.2017.09.001
Liu, P., Piao, X. S., Kim, S. W., Wang, L., Shen, Y. B., Lee, H. S., et al. (2008). Effects of chito-oligosaccharide supplementation on the growth performance, nutrient digestibility, intestinal morphology, and fecal shedding of Escherichia coli and Lactobacillus in weaning pigs. J. Anim. ence 86, 2609–2618. doi: 10.2527/jas.2007-0668
Luo, L., Cai, X., He, C., Xue, M., Wu, X., and Cao, H. (2009). Immune response, stress resistance and bacterial challenge in juvenile rainbow trouts Oncorhynchus mykiss fed diets containing chitosan-oligosaccharides. Curr. Zool. 55, 416–422. doi: 10.1093/czoolo/55.6.416
Mahious, A. S., Gatesoupe, F. J., Hervi, M., Metailler, R., and Ollevier, F. (2006). Effect of dietary inulin and oligosaccharides as prebiotics for weaning turbot, Psetta maxima (Linnaeus, C. 1758). Aquacult. Int. 14, 219–229. doi: 10.1007/s10499-005-9003-4
Maqsood, S., Singh, P., Samoon, M. H., and Balange, A. K. (2010). Effect of dietary chitosan on non-specific immune response and growth of Cyprinus carpio challenged with Aeromonas hydrophila. J. Card. Fail. 2, 77–85. Available online at: https://www.researchgate.net/publication/286150928
Mei, G. Y., Carey, C. M., Tosh, S., and Kostrzynska, M. (2011). Utilization of different types of dietary fibres by potential probiotics. Can. J. Microbiol. 57:857. doi: 10.1139/w11-077
Mussatto, S. I., and Mancilha, I. M. (2007). Non-digestible oligosaccharides: a review. Carbohydr. Polym. 68, 587–597. doi: 10.1016/j.carbpol.2006.12.011
Naveed, M., Phil, L., Sohail, M., Hasnat, M., Baig, M. M. F. A., Ihsan, A. U., et al. (2019). Chitosan oligosaccharide (COS): an overview. Int. J. Biol. Macromol. 129, 827–843. doi: 10.1016/j.ijbiomac.2019.01.192
Ofek, I., Mirelman, D., and Sharon, N. (1977). Adherence of Escherichia coli to human mucosal cells mediated by mannose receptors. Nature 265, 623–625. doi: 10.1038/265623a0
Palframan, R. J., Gibson, G. R., and Rastall, R. A. (2002). Effect of pH and dose on the growth of gut bacteria on prebiotic carbohydrates in vitro. Anaerobe 8, 287–292. doi: 10.1006/anae.2002.0434
Pérez-Jiménez, A., Bañuelos-Vargas, I., López, L. M., and Peres, H. (2014). Effect of fishmeal replacement by soy protein concentrate with taurine supplementation on hepatic intermediary metabolism and antioxidant status of totoaba juveniles (Totoaba macdonaldi). Comp. Biochem. Physiol. Part B Biochem. Molecul. Biol. 170, 18–25. doi: 10.1016/j.cbpb.2014.01.003
Qingsen, S., Xindi, S., Chao, C., Jiejie, H., and Guoyun, L. (2016). Dietary fucoidan modulates the gut microbiota in mice by increasing the abundance of Lactobacillus and Ruminococcaceae. Food Funct. 7, 3224–3232. doi: 10.1039/C6FO00309E
Rajendran, S., Okolie, C. L., Udenigwe, C. C., and Mason, B. (2017). Structural features underlying prebiotic activity of conventional and potential prebiotic oligosaccharides in food and health. J. Food Biochem. 41:e12389. doi: 10.1111/jfbc.12389
Refstie, S., Landsverk, T., Bakke-Mckellep, A. M., Ring, E., Sundby, A., Shearer, K. D., et al. (2006). Digestive capacity, intestinal morphology, and microflora of 1-year and 2-year old Atlantic cod (Gadus morhua) fed standard or bioprocessed soybean meal. Aquaculture 261, 269–284. doi: 10.1016/j.aquaculture.2006.07.011
Ricardo, S., Alvaro, B., and José, E. (2014). Growth and intestinal morphology of juvenile pacu Piaractus mesopotamicus (Holmberg 1887) fed dietary prebiotics (mannanoligosaccharides—MOS). Anais da Academia Brasileira de Ciências 86, 1517–1524. doi: 10.1590/0001-3765201420130088
Ring, E., Olsen, R. E., Gifstad, T., Dalmo, R. A., and Bakke, A. M. (2010). Prebiotics in aquaculture: a review. Aquacult. Nutrit. 16, 117–136. doi: 10.1111/j.1365-2095.2009.00731.x
Sahoo, B. R., Dikhit, M. R., Bhoi, G. K., Maharana, J., Lenka, S. K., Dubey, P. K., et al. (2015). Understanding the distinguishable structural and functional features in zebrafish TLR3 and TLR22, and their binding modes with fish dsRNA viruses: an exploratory structural model analysis. Amino Acids 47, 381–400. doi: 10.1007/s00726-014-1872-2
Salze, G., Mclean, E., Schwarz, M. H., and Craig, S. R. (2008). Dietary mannan oligosaccharide enhances salinity tolerance and gut development of larval cobia. Aquaculture 274, 148–152. doi: 10.1016/j.aquaculture.2007.11.008
Sang, H. M., Fotedar, R., and Filer, K. (2015). Effects of dietary mannan oligosaccharide on the survival, growth, immunity and digestive enzyme activity of freshwater crayfish, Cherax destructor Clark (1936). Aquacult. Nutrit. 17, e629–e635. doi: 10.1111/j.1365-2095.2010.00812.x
Saurabh, S., and Sahoo, P. K. (2010). Lysozyme: an important defence molecule of fish innate immune system. Aquac. Res. 39, 223–239. doi: 10.1111/j.1365-2109.2007.01883.x
Schloss, P. D., Westcott, S. L., Ryabin, T., Hall, J. R., Hartmann, M., Hollister, E. B., et al. (2009). Introducing mothur: open-source, platform-independent, community-supported software for describing and comparing microbial communities. Appl. Environ. Microbiol. 75, 7537–7541. doi: 10.1128/AEM.01541-09
Shen, J., Liu, H., Tan, B., Dong, X., Yang, Q., Chi, S., et al. (2020). Effects of replacement of fishmeal with cottonseed protein concentrate on the growth, intestinal microflora, haematological and antioxidant indices of juvenile golden pompano (Trachinotusovatus). Aquacult. Nutrit. 26, 1119–1130. doi: 10.1111/anu.13069
Si, B., Wang, Y., Bao, N., Chen, F., Wang, L., Sun, M., et al. (2017). Effects of coated chitosan oligosaccharides on growth performance and immune response of sea cucumber (Stichopus japonicus). J. Guangdong Ocean Univ. 37, 28–34. doi: 10.3969/j.issn.1673-9159.2017.01.005
Skiba-Cassy, S., Geurden, I., Panserat, S., and Seiliez, I. (2016). Dietary methionine imbalance alters the transcriptional regulation of genes involved in glucose, lipid and amino acid metabolism in the liver of rainbow trout (Oncorhynchusmykiss). Aquaculture 454, 56–65. doi: 10.1016/j.aquaculture.2015.12.015
Song, S. K., Beck, B. R., Kim, D., Park, J., Kim, J., Kim, H. D., et al. (2014). Prebiotics as immunostimulants in aquaculture: a review. Fish Shellfish Immunol. 40, 40–48. doi: 10.1016/j.fsi.2014.06.016
Sun, L. W., Wen, H., Jiang, M., Fan, W. U., Liu, W., Tian, J., et al. (2011). Effect of dietary chitosan on growth performance, non-specific immunity and hematological parameters of juvenile GIFT Tilapia, Oreochromisniloticus. J. Guangdong Ocean Univ. 31, 43–49. Available online at: http://en.cnki.com.cn/Article_en/CJFDTOTAL-SHDX201103009.htm
Swiatkiewicz, S., Swiatkiewicz, M., Arczewska-Wlosek, A., and Jozefiak, D. (2015). Chitosan and its oligosaccharide derivatives (chito-oligosaccharides) as feed supplements in poultry and swine nutrition. J. Anim. Physiol. Anim. Nutr. 99, 1–12. doi: 10.1111/jpn.12222
Talpur, A. D., Munir, M. B., Mary, A., and Hashim, R. (2014). Dietary probiotics and prebiotics improved food acceptability, growth performance, haematology and immunological parameters and disease resistance against Aeromonas hydrophila in snakehead (Channastriata) fingerlings. Aquaculture 426, 14–20. doi: 10.1016/j.aquaculture.2014.01.013
Tao, F., Du, Y., Li, J., Wei, Y., and Yao, P. (2007). Antioxidant activity of half N-acetylated water-soluble chitosan in vitro. Euro. Food Res. Technol. 225, 133–138. doi: 10.1007/s00217-006-0391-0
Tarnecki, A. M., Burgos, F. A., Ray, C. L., and Arias, C. R. (2017). Fish intestinal microbiome: diversity and symbiosis unravelled by metagenomics. J. Appl. Microbiol. 123, 2–17. doi: 10.1111/jam.13415
Torrecillas, S., Rivero-Ramírez, F., Izquierdo, M., Caballero, M., Makol, A., Suarez-Bregua, P., et al. (2018). Feeding European sea bass (Dicentrarchuslabrax) juveniles with a functional synbiotic additive (mannan oligosaccharides and Pediococcus acidilactici): an effective tool to reduce low fishmeal and fish oil gut health effects? Fish Shellfish Immunol. 81, 10–20. doi: 10.1016/j.fsi.2018.07.007
Vieira, T. F., Corrêa, R., Peralta, R. A., Peralta-Muniz-Moreira, R. F., Adelar, B., and Peralta, R. M. (2020). An overview of structural aspects and health beneficial effects of antioxidant oligosaccharides. Curr. Pharm. Des. 26, 1759–1777. doi: 10.2174/1381612824666180517120642
Waite, D. W., Inka, V., Christian, R., Parks, D. H., Ying, Z., Ken, T., et al. (2017). Comparative genomic analysis of the class epsilonproteobacteria and proposed reclassification to epsilonbacteraeota (phyl. nov.). Front. Microbiol. 8:19. doi: 10.3389/fmicb.2017.00682
Wan, J., Yang, K., Xu, Q., Chen, D., Yu, B., Luo, Y., et al. (2016). Dietary chitosan oligosaccharide supplementation improves foetal survival and reproductive performance in multiparous sows. RSC Adv. 6, 70715–70722. doi: 10.1039/C6RA13294D
Wan, M., Yin, P., Fang, W., Xie, S., Chen, S. J., Tian, L. X., et al. (2018). The effect of replacement of fishmeal by concentrated dephenolization cottonseed protein on the growth, body composition, haemolymph indexes and haematological enzyme activities of the Pacific white shrimp (Litopenaeusvannamei). Aquacult. Nutri. 24, 1845–1854. doi: 10.1111/anu.12823
Wang, Q., Garrity, G. M., Tiedje, J. M., and Cole, J. R. (2007). Naive Bayesian classifier for rapid assignment of rRNA sequences into the new bacterial taxonomy. Appl. Environ. Microbiol. 73, 5261–5267. doi: 10.1128/AEM.00062-07
Wanga, K. Z., Jianga, W. D., Wua, P., Liua, Y., and Jianga, J. (2018). Gossypol reduced the intestinal amino acid absorption capacity of young grass carp (Ctenopharyngodonidella). Aquaculture 492, 46–58. doi: 10.1016/j.aquaculture.2018.03.061
Wei, G., Cai, S., Wu, Y., Ma, S., and Huang, Y. (2020). Immune effect of Vibrio harveyi formalin-killed cells vaccine combined with chitosan oligosaccharide and astragalus polysaccharides in♀ Epinephelusfuscoguttatus×♂ Epinepheluslanceolatus. Fish Shellfish Immunol. 98, 186–192. doi: 10.1016/j.fsi.2020.01.015
Wei, H., Yang, M., Zhao, T., Wang, X., and Zhou, H. (2013). Functional expression and characterization of grass carp IL-10: An essential mediator of TGF-β1 immune regulation in peripheral blood lymphocytes. Mol. Immunol. 53, 313–320. doi: 10.1016/j.molimm.2012.08.021
Wink, D. A., Darbyshire, J. F., Nims, R. W., Saavedra, J. E., and Ford, P. C. (1993). Reactions of the bioregulatory agent nitric oxide in oxygenated aqueous media: Determination of the kinetics for oxidation and nitrosation by intermediates generated in the nitric oxide/oxygen reaction. Chem. Res. Toxicol. 6, 23–27. doi: 10.1021/tx00031a003
Wu, C., Lu, B., Wang, Y., Jin, C., and Ye, J. (2020). Effects of dietary vitamin D3 on growth performance, antioxidant capacities and innate immune responses in juvenile black carp Mylopharyngodonpiceus. Fish Physiol. Biochem. 46, 2243–2256. doi: 10.1007/s10695-020-00876-8
Wu, Y. V., Tudor, K. W., Brown, P. B., and Rosati, R. R. (1999). Substitution of Plant Proteins or Meat and Bone Meal for Fish Meal in Diets of Nile Tilapia. North Am. J. Aquaculture 61, 58–63. doi: 10.1577/1548-8454(1999)061<0058:SOPPOM>2.0.CO;2
Wu, M. S. B., Singh, J., Wang, C., and Chung, C. (2011). Antidiabetic and antisteatotic effects of the selective fatty acid synthase (FAS) inhibitor platensimycin in mouse models of diabetes. Proc. Nat. Acad. Sci. 108, 5378–5383. doi: 10.1073/pnas.1002588108
Xiaoyi, S., Hua, Y., Nose, K., Satoko, H., and Emir, Q. (2008). Decline in intestinal mucosal IL-10 expression and decreased intestinal barrier function in a mouse model of total parenteral nutrition. Am. J. Physiol. Gastrointest. Liver Physiol. 57, 139–147. doi: 10.1152/ajpgi.00386.2007
Xie, W., Xu, P., and Liu, Q. (2001). Antioxidant activity of water-soluble chitosan derivatives. Bioorgan. Med. Chem. Lett. 11, 1699–1701. doi: 10.1016/S0960-894X(01)00285-2
Yamamoto, T., Matsunari, H., Sugita, T., Furuita, H., Masumoto, T., Iwashita, Y., et al. (2012). Optimization of the supplemental essential amino acids to a fish meal-free diet based on fermented soybean meal for rainbow trout Oncorhynchusmykiss. Fish. Sci. 78, 359–366. doi: 10.1007/s12562-011-0456-2
Yan, L., and Xiao, Q. Z. (2006). Dietary glutamine supplementation improves structure and function of intestine of juvenile Jian carp (Cyprinuscarpiovar. Jian)—sciencedirect. Aquaculture 256, 389–394. doi: 10.1016/j.aquaculture.2006.02.011
Ye, G., Dong, X., Yang, Q., Chi, S., Liu, H., Zhang, H., et al. (2020). Low-gossypol cottonseed protein concentrate used as a replacement of fish meal for juvenile hybrid grouper (Epinephelusfuscoguttatus♀ × Epinepheluslanceolatus♂): Effects on growth performance, immune responses and intestinal microbiota. Aquaculture 524:735309. doi: 10.1016/j.aquaculture.2020.735309
Yin, B., Liu, H., Tan, B., Dong, X., Chi, S., Yang, Q., et al. (2018). Cottonseed protein concentrate (CPC) suppresses immune function in different intestinal segments of hybrid grouper♀ Epinephelusfuscoguttatus×♂ Epinepheluslanceolatu via TLR-2/MyD88 signaling pathways. Fish Shellfish Immunol. 81, 318–328. doi: 10.1016/j.fsi.2018.07.038
Yin, B., Liu, H., Tan, B., Dong, X., Chi, S., Yang, Q., et al. (2020). Preliminary study of mechanisms of intestinal inflammation induced by plant proteins in juvenile hybrid groupers (♀ Epinephelus fuscoguttatus × ♂ E. lanceolatu). Fish and Shellfish Immunol. 106, 341–356. doi: 10.1016/j.fsi.2020.07.026
Yu, Z., He, X., Fu, D., and Zhang, Y. (2011). Two superoxide dismutase (SOD) with different subcellular localizations involved in innate immunity in Crassostreahongkongensis. Fish Shellfish Immunol. 31, 533–539. doi: 10.1016/j.fsi.2011.06.022
Zeng, Q., Tian, X., and Wang, L. (2017). Genetic adaptation of microbial populations present in high-intensity catfish production systems with therapeutic oxytetracycline treatment. Sci. Rep. 7, 1–13. doi: 10.1038/s41598-017-17640-3
Zhang, B. (2019). Dietary chitosan oligosaccharides modulate the growth, intestine digestive enzymes, body composition and nonspecific immunity of loach Paramisgurnusdabryanus. Fish Shellfish Immunol. 88, 359–363. doi: 10.1016/j.fsi.2019.03.006
Zhang, R. B., Cao, J. M., Huang, Y. H., Wang, G. X., Chen, X. Y., Yan, J., et al. (2012). Effect of dietary xylooligosaccharides on intestinal histology and microflora of tilapia(Oreochromisniloticus×O.aureus) and resistance against Aeromonashydrophila. J. Shanghai Ocean Univ. 21, 233–240. doi: 10.1007/s11783-011-0280-z
Zhou, S., Song, D., Zhou, X., Mao, X., Zhou, X., Wang, S., et al. (2019). Characterization of Bacillus subtilis from gastrointestinal tract of hybrid Hulong grouper (Epinephelusfuscoguttatus× E.lanceolatus) and its effects as probiotic additives. Fish Shellfish Immunol. 84, 1115–1124. doi: 10.1016/j.fsi.2018.10.058
Keywords: chitosan oligosaccharide (cos), prebiotics, fishmeal replacement, intestinal microbiota, immune response
Citation: Chen G, Yin B, Liu H, Tan B, Dong X, Yang Q, Chi S and Zhang S (2021) Supplementing Chitosan Oligosaccharide Positively Affects Hybrid Grouper (Epinephelus fuscoguttatus ♀ × E. lanceolatus ♂) Fed Dietary Fish Meal Replacement With Cottonseed Protein Concentrate: Effects on Growth, Gut Microbiota, Antioxidant Function and Immune Response. Front. Mar. Sci. 8:707627. doi: 10.3389/fmars.2021.707627
Received: 10 May 2021; Accepted: 21 July 2021;
Published: 18 August 2021.
Edited by:
Jin Niu, Sun Yat-Sen University, ChinaReviewed by:
Yun-Zhang Sun, Jimei University, ChinaDeiene Rodriguez Barreto, University of La Laguna, Spain
Copyright © 2021 Chen, Yin, Liu, Tan, Dong, Yang, Chi and Zhang. This is an open-access article distributed under the terms of the Creative Commons Attribution License (CC BY). The use, distribution or reproduction in other forums is permitted, provided the original author(s) and the copyright owner(s) are credited and that the original publication in this journal is cited, in accordance with accepted academic practice. No use, distribution or reproduction is permitted which does not comply with these terms.
*Correspondence: Hongyu Liu, liuhyu@gdou.edu.cn; Beiping Tan, bptan@126.com