- 1Université Grenoble Alpes, CNRS, IRD, Grenoble INP, IGE, Grenoble, France
- 2Institute for Marine and Atmospheric Research, Utrecht University, Utrecht, Netherlands
- 3Coastal Risks and Climate Change Unit, Risk and Prevention Department, French Geological Survey, BRGM, Orléans, France
- 4Department of Geography, King’s College London, London, United Kingdom
- 5British Antarctic Survey, Cambridge, United Kingdom
- 6Institute of Geography and MARUM, Center for Marine Environmental Sciences, University of Bremen, Bremen, Germany
- 7Danish Meteorological Institute, Copenhagen, Denmark
- 8Tyndall Centre for Climate Change Research, University of East Anglia, Norwich, United Kingdom
- 9Laboratoire de Glaciologie, Université Libre de Bruxelles, Brussels, Belgium
- 10Department of Geography, University of Zurich, Zurich, Switzerland
- 11Department of Estuarine and Delta Systems, NIOZ Royal Netherlands Institute for Sea Research, Yerseke, Netherlands
- 12Potsdam Institute for Climate Impact Research (PIK), Member of the Leibniz Association, Potsdam, Germany
- 13Institute of Physics and Astronomy, University of Potsdam, Potsdam, Germany
- 14Faculty of Aerospace Engineering, Delft University of Technology, Delft, Netherlands
Coastal areas are highly diverse, ecologically rich, regions of key socio-economic activity, and are particularly sensitive to sea-level change. Over most of the 20th century, global mean sea level has risen mainly due to warming and subsequent expansion of the upper ocean layers as well as the melting of glaciers and ice caps. Over the last three decades, increased mass loss of the Greenland and Antarctic ice sheets has also started to contribute significantly to contemporary sea-level rise. The future mass loss of the two ice sheets, which combined represent a sea-level rise potential of ∼65 m, constitutes the main source of uncertainty in long-term (centennial to millennial) sea-level rise projections. Improved knowledge of the magnitude and rate of future sea-level change is therefore of utmost importance. Moreover, sea level does not change uniformly across the globe and can differ greatly at both regional and local scales. The most appropriate and feasible sea level mitigation and adaptation measures in coastal regions strongly depend on local land use and associated risk aversion. Here, we advocate that addressing the problem of future sea-level rise and its impacts requires (i) bringing together a transdisciplinary scientific community, from climate and cryospheric scientists to coastal impact specialists, and (ii) interacting closely and iteratively with users and local stakeholders to co-design and co-build coastal climate services, including addressing the high-end risks.
Introduction
Coastal zones (regions less than 10 m above mean sea level) are ecologically rich, diverse and productive, but highly sensitive to changes in sea level (Wong et al., 2014; Oppenheimer et al., 2019). They concentrate population, being home to more than 600 million people, of which about 300 million live in flood-prone areas. Coastal zones are key engines of the global economy and sites of significant urbanization, hosting 65% of the world’s largest and numerous smaller cities. If current trends continue, the coastal population will roughly double by 2060 (Neumann et al., 2015), while the coastal economy will grow even more – plausibly by an order of magnitude – greatly increasing the exposure of humans and infrastructure to the hazards caused by sea-level rise (SLR). From an environmental and socio-economic point of view, improving decadal to centennial projections of regionally resolved SLR and making them available to coastal stakeholders has the highest priority.
This study aims to consider how we can most effectively enhance global understanding of sea level, and translate this into coastal climate services to provide useful information for local impact and adaptation needs assessment. This involves considering the science of global sea-level change, translation of global information to potential local sea-level change, as well as user and stakeholder engagement to co-produce projections appropriate to the decisions being made. These three points are outlined in more detail below and in the following sections.
In the 20th century, SLR was mainly caused by mass loss of glaciers and ice caps, henceforth simply referred to as glaciers,1 and by ocean thermal expansion (IPCC, 2019, 2021). However, since 1990, both the Antarctic Ice Sheet (AIS) and Greenland Ice Sheet (GrIS), which combined represent a sea-level rise potential of ∼65 m, have increasingly contributed to SLR (Bamber et al., 2018; Shepherd et al., 2018, 2021). As highlighted in the last three assessment reports of the Intergovernmental Panel on Climate Change (IPCC) (IPCC, 2007, 2013, 2021) and the Special Report on the Ocean and Cryosphere in a Changing Climate (BR001), the main uncertainty in projections of future SLR is the limited ability to model the future melt dynamics of the AIS and GrIS. Uncertainty quantification is further complicated by the fact that both ice sheets have been estimated to have tipping points at, or slightly above, 1.5–2.0°C warming compared to the pre-industrial epoch (Pattyn et al., 2018; Pattyn and Morlighem, 2020; Noël et al., 2021). Crossing these tipping points might lead to self-sustained demise of large parts of both ice sheets with a multi-meter contribution to SLR within a couple of centuries as a consequence. Though the relative contribution of ice sheet mass loss to SLR is expected to increase further over the 21st Century (Marzeion et al., 2018; Goelzer et al., 2020; Seroussi et al., 2020), glaciers will continue to contribute significantly to SL, a contribution that must also be better quantified (IPCC, 2021). Clearly, future SLR is a global problem that is mainly controlled by the future losses of land ice masses. This aspect is further detailed in Section “Components of Global Mean Sea-Level Rise.”
It is important to recognize that SLR is highly non-uniform in space and time and this needs to be understood to provide useful sea-level scenarios and coastal climate services. While in mitigation we consider global changes, impacts and adaptation need to reflect local changes. Ocean warming causes thermal expansion which leads to an increase in ocean volume. Water exchange between the land and the ocean, for instance by the melting of land ice, causes a change in ocean mass. Superimposed on these global processes are regional variations resulting from changes in ocean currents and density, as well as gravitational, rotational and deformational effects resulting from changes in the loading of ice and water masses and all components of vertical land movement. To make an inventory of potential coastal impacts and adaptation needs thus requires SLR projections that are essentially regional (Slangen et al., 2014) and local (Wöppelmann and Marcos, 2016; Woodworth et al., 2019; Nicholls et al., 2021a). This is detailed in Section “Sea-Level Rise Impacts: The Need for Local Information.”
There are multiple approaches to quantify SLR uncertainty, yet none of these can fully embrace the wide variety of user needs for sea level information. Individual user needs depend strongly on the value of exposed assets, their level of risk aversion, the relevant timescale and the wider context set by other stakeholders such as national governments, regional/local authorities, coastal conservation agencies, insurance/investment companies, etc (Hinkel et al., 2019; Kopp et al., 2019; Nicholls et al., 2021a). As a consequence, improving and adjusting SLR projections to the needs of our society can only be achieved through a broad and multidisciplinary collaboration in close and iterative interaction with users and stakeholders. This is discussed in Section “Interactions With Stakeholders and Co-design of Sea-Level Rise Projections.”
Components of Global Mean Sea-Level Rise
Sea-level rise contributions from thermal expansion (due to ocean warming) and changes in ocean dynamics (due to changes in ocean currents and density) can be quantified by global climate and related models. Between 2006 and 2018, thermal expansion contributed to increase mean SLR by 1.39 mm/yr and for 2100 is projected to contribute to SLR in the range between 0.12 (0.09–0.15) m under the high mitigation SSP1-1.9 scenario to 0.30 (0.24–0.36) m under the highly pessimistic SSP5-8.5 scenario, accounting for 30–40% of the total SLR (Fox-Kemper et al., 2021). Thermal expansion is a relatively well understood process, and uncertainties mainly reflect differences between scenarios.
The remainder of the projected 21st century total SLR mainly constitutes the melting of land ice. Apart from glaciers and increasingly the GrIS, this represents continued mass loss from specific regions in Antarctica, notably the West Antarctic Ice Sheet and the Antarctic Peninsula. Evidence of mass loss in these regions of the AIS has become clear with the advent of widespread remote sensing measurements in the early 1990s, and their rate of mass loss has steadily increased since the early 2000s. In contrast, the mass balance of the much bigger East Antarctic Ice Sheet remains highly uncertain (Shepherd et al., 2018; Rignot et al., 2019). Overall, Antarctica contributed 0.37 (0.24–0.50) mm/yr to SLR between 2006 and 2018 (Fox-Kemper et al., 2021). The mass balance of the AIS currently has two major components: a positive contribution made by the annual accumulation of ∼2500 Gt of snow at the ice sheet surface and a negative contribution of similar magnitude made by the flow of ice streams and outlet glaciers toward the ocean, where they form extensive floating ice shelves which lose mass by basal melt at the ocean-ice interface and iceberg calving at the ice shelf front. Assumed smaller (<10%) but poorly understood contributions to the AIS mass balance are basal melt at the ice-bedrock interface, (drifting snow) sublimation and the runoff of meltwater (van Wessem et al., 2018; Agosta et al., 2019).
The primary driver of current AIS mass loss is the acceleration of outlet glaciers, notably those feeding the Amundsen Sea Embayment (ASE) in West Antarctica. Here, ice discharge increased by about 77% from 1973 to 2013 (Mouginot et al., 2014). Acceleration of ice flow in the ASE occurs in response to incursions of warm Circumpolar Deep Water, thinning the ice shelves from below (Dutrieux et al., 2014). The thinning of ice shelves reduces the buttressing they exert on upstream grounded glaciers and consequently enhances the ice flow, causing mass loss of grounded ice and SLR (Gagliardini et al., 2010). The ASE is of particular concern because the grounding line, the transition between grounded and floated ice, rests on bedrock generally sloping downwards toward the interior of the ice sheet (Morlighem et al., 2020). In such a topographic configuration, the grounding line may be unstable whereby an initial retreat reinforces the ice outflow, leading to thinning and further retreat, etc. This process, known as the Marine Ice Sheet Instability (MISI), has been discussed for decades (Weertman, 1974; Vaughan, 2008) and it is nowadays acknowledged that unconfined ice shelves are indeed unstable (Schoof, 2007), although ice-shelf buttressing and/or rapid bedrock uplift might in some situations stabilize the glacier (Gudmundsson et al., 2012; Barletta et al., 2018). It has been proposed that MISI could be engaged in the ASE once a 1.5–2°C global warming compared to preindustrial has been exceeded (Pattyn et al., 2018; Rosier et al., 2021).
The retreat of Antarctic outlet glaciers that are currently confined by ice shelves might be further accelerated if intense surface melting leads to meltwater ponding and subsequent ice shelf disintegration. This process of hydro-fracturing is thought to be responsible for the catastrophic disintegration of Larsen A (1995), Larsen B (2002), and Wilkins (2008) ice shelves in the Antarctic Peninsula (Scambos et al., 2009). It has also been proposed that the collapse of ice shelves could expose ice cliffs high enough to produce stresses exceeding the strength of ice and leading to consecutive ice cliff collapses and rapid retreat of the ice shelf front (DeConto and Pollard, 2016). This process, named Marine Ice Cliff Instability (MICI), remains as yet unproven (Edwards et al., 2019). Current median projections of the contribution of the AIS to SLR from 1995–2014 baseline to 2,100 range from 0.10 to 0.12 m independent of the considered Shared Socioeconomic Pathways (SSP) (Fox-Kemper et al., 2021). However, the poorly understood MISI and MICI and the subsequent dynamical responses could introduce a considerably higher contribution (Bamber et al., 2019; DeConto et al., 2021; Fox-Kemper et al., 2021).
The mass balance of the GrIS is very different from that of the AIS. Apart from iceberg calving in narrow fjords -the GrIS does not have extensive ice shelves-, an additional significant negative mass balance component is meltwater runoff. Until the mid 1990s, mass losses from the GrIS were probably modest (Mouginot et al., 2019), but a series of strong melt years since the mid 2000s, together with the rapid acceleration and retreat of multiple major outlet glaciers (King et al., 2020), tipped the annual ice sheet budget into a persistent negative state (Shepherd et al., 2018). With a contemporary mass loss equivalent of 0.63 (0.51–0.74) mm per year between 2006 and 2018, the GrIS is currently the largest single net contributor to increased ocean mass leading to SLR (Fox-Kemper et al., 2021). At the same time, interannual variability of mass loss also appears to have increased (Simonsen et al., 2021), mainly owing to highly variable surface processes. The increase in surface melt and runoff since the late 1990s is in part due to the increased occurrence and persistence of atmospheric blocking over Greenland, leading to clear skies, higher air temperatures and higher melt rates notably on the southwestern GrIS (Hanna et al., 2018). However, this effect varies between years.
By storing and refreezing liquid water, the layer of firn (compressed snow) that covers 90% of the GrIS currently prevents nearly half of the percolating meltwater to run off. Strong melt events, particularly in the summers of 2012 and 2019, measurably increased the extent of impermeable ice layers that reduce percolation into the deeper firn in subsequent years, enhancing runoff (MacFerrin et al., 2019). The increase in GrIS mass loss from acceleration and increased submarine melting of marine-terminating outlet glaciers was likely, at least in part, initiated by increased ocean temperatures (Straneo and Heimbach, 2013). Notably, the retreat of glaciers in southeast and west Greenland concurred with warm ocean waters becoming entrained into the fjord environments and eventually melting the glacier fronts. In spite of the continuously improved understanding of dynamic behavior of marine-terminating outlet glaciers, the wide range of factors that determine this behavior, including bed topography, makes it complicated to generalize the response of outlet glacier dynamics to future climate warming.
In a long term perspective, the GrIS might enter a self-sustained melting state, due to positive feedbacks such as the melt-elevation feedback (a lower ice sheet surface faces a warmer atmosphere and melts even faster, etc.) and the melt-albedo feedbacks (as the ice sheet melts its surface gets darker and melts even faster). Once meltwater runoff exceeds the accumulation of snow, mass loss becomes quasi-irreversible; Pattyn et al. (2018) estimated that such a threshold could be crossed at about 2°C warming above pre-industrial, which could be reached already around 2055 under a high-end warming scenario (Noël et al., 2021). Current main projections of the contribution of the GrIS to SLR from 1995–2014 baseline to 2100 range from 0.05 (0.00–0.09) m (SSP1-19) to 0.13 (0.09–0.18) m (SSP5-85) (Fox-Kemper et al., 2021).
While glaciers store less than 1% of the global ice mass (Farinotti et al., 2019), their increasing mass loss rates (Zemp et al., 2019; Hugonnet et al., 2021) exceeded those of the GrIS or AIS in recent years. For the period 2006 to 2018, Fox-Kemper et al. (2021) estimated the glacier contribution (excluding glaciers in the periphery of the ice sheets) to SLR to be 0.62 (0.57–0.68) mm/yr. In the past two decades, all regions that harbor glaciers have contributed to SLR (Hock et al., 2019a; IPCC, 2019). Because the geometric adjustment of glacier extent in response to climate change is delayed, glacier mass loss during the first half of the 21st century is already committed and to a large degree independent of future greenhouse gas emissions (Marzeion et al., 2018). The evolution of glacier mass during the second half of the 21st century depends strongly on the considered emission scenario, but also on the combination of climate and glacier models used for the projection (Hock et al., 2019b; Marzeion et al., 2020). Current main projections of the contribution of the glaciers to SLR from 1994–2015 baseline to 2100 range from 0.08 (0.06–0.10) m (SSP1-19) to 0.18 (0.15–0.20) m (SSP5-85) (Fox-Kemper et al., 2021).
Given (i) the leading contribution to SLR of land ice melt and the associated future SLR commitment, (ii) its huge potential to further contribute to future SLR with tipping points that could be crossed in the coming decades by both the AIS and the GrIS, combined with (iii) the deep uncertainties attached to poorly understood processes controlling the possible self-sustained demise of large regions of both ice sheets, we must intensify in-depth research into glacier and ice sheet mass balance. The complexity of the system under study requires an interdisciplinary team with numerical/observational experts from the following research disciplines: (i) meteorology and surface mass balance of ice sheets and glaciers, (ii) oceanography and ice shelf basal melting, (iii) ice sheet and glacier processes (including calving) and dynamics, (iv) the coupling of these components, and (v) uncertainty quantification.
Sea-Level Rise Impacts: The Need for Local Information
Although global-mean SLR is a useful climate metric, multiple processes cause large spatial and temporal variations in sea-level change on a wide range of scales, which must be considered if we wish to develop information relevant to impacts, adaptation needs, and wider climate services in sea level (Figure 1). At the regional scale (∼100 km), changes in dynamic sea level reflect changes in ocean water density (temperature and salinity) as well as changes in the wind-driven and density-driven ocean circulation, e.g., forced by El Niño Southern Oscillation. A second driver for regional sea-level changes are the gravitational, rotational and deformational effects resulting from mass redistribution between land and ocean. When an ice sheet loses mass, its gravitational pull on the ocean is reduced, leading to a sea-level fall close to the ice sheet itself, but greater than average SLR in the far field, i.e., more than 2,000 km away from the mass source (Mitrovica et al., 2001). The resulting pattern is often referred to as a sea-level ‘fingerprint’ and typically shows an above-average SLR in the Northern Hemisphere for mass loss from the AIS, and an above-average SLR in the Southern Hemisphere for mass loss from the GrIS. Vertical land movement is also of particular importance, as in some coastal locations its magnitude is equal to or even larger than the long-term SLR, amplifying local relative SLR (Nicholls et al., 2021b). Possible causes are natural or human-induced subsidence (through e.g., compaction of sedimentary layers due to groundwater extraction), glacial isostatic adjustment, and tectonics. Glacial isostatic adjustment is estimated using global models (e.g., Peltier et al., 2015), while other components can be derived from in situ measurements such as in deltas and cities (Nicholls et al., 2021b) and at tide gauges (e.g., Kopp et al., 2014).
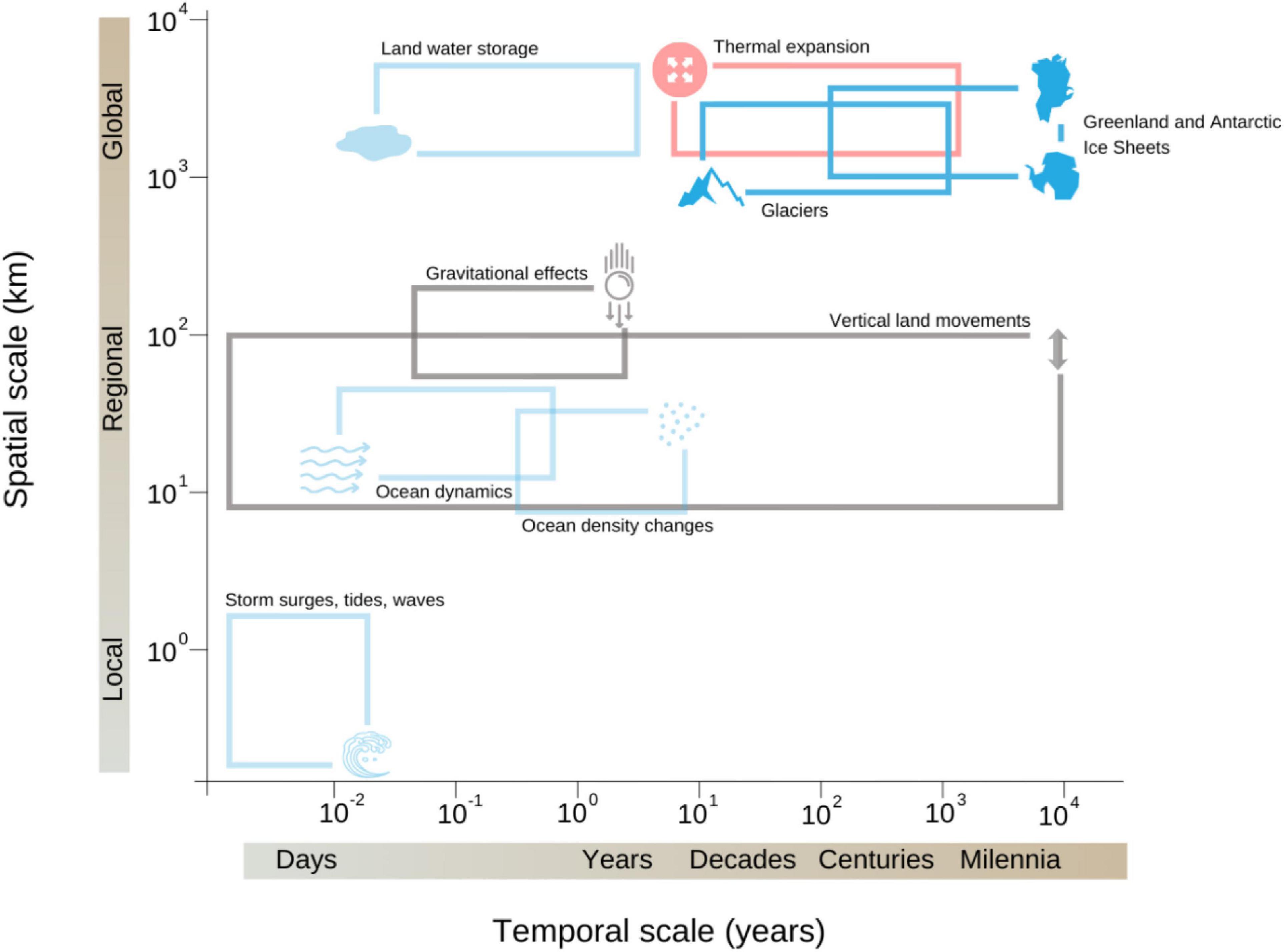
Figure 1. Illustration of the processes that contribute to sea level change with respect to their temporal and spatial scales.
Sea-level rise is also affected by processes acting on a local scale (∼10 km or less) such as ocean and coastal dynamic (geomorphological) processes (Zhang et al., 2017). Of particular relevance to coastal risk management are changes in the frequency and height of sea-level extremes, for instance from tides, storm surges or waves and their combinations, including mean SLR (Allison et al., 2021). Although these processes typically occur on short timescales (hours to days) and only have a local to regional effect on the coast, their impacts are magnified by long-term SLR (Oppenheimer et al., 2019). There is also evidence that tide and surge propagation is being affected by SLR, as well as human modifications to bathymetry, and this has the potential to enhance extreme events more than SLR alone (Arns et al., 2015; Haigh et al., 2020; Talke and Jay, 2020). Further, such extreme events cause most floods and damage, so stakeholders strongly focus on adaptation to them while often raising the consideration of adaptation to mean SLR as well (e.g., Rosenzweig et al., 2011; Ranger et al., 2013). Adding appropriate allowance, Figure 1 summarizes crucial processes influencing change in sea level and their associated spatial and temporal scales.
As SLR will have a local impact, and decisions on adaptation and mitigation are also made on a local scale, it is essential that coastal climate services provide local sea-level projections that include the downscaling of all relevant processes; considering only global mean projections will seriously misdirect adaptation efforts and may lead to maladaptation. Therefore, producing reliable and sensible SLR projections requires that climate and sea-level scientists interact with specialists in the field of geodesy, earth science and ocean dynamics. Further, the context of use and application of the local (or relative) sea-level rise information needs to be considered so the relevant coastal impacts, adaptation needs and societal context needs to be considered as discussed in the next section.
Interactions With Stakeholders and Co-Design of Sea-Level Rise Projections
The processes and methods described above contain uncertainties that differ in both nature and magnitude (e.g., Bakker et al., 2017), accumulate across scales (e.g., Rohmer et al., 2019) and ultimately affect coastal impact and adaptation assessments (Toimil et al., 2020; Rohmer et al., 2021). Historically, the IPCC has delivered sea-level projections with uncertainties described using quantitative metrics (likelihood) associated with qualitative confidence statements reflecting the quality of the evidence and the degree of agreement (Mastrandrea et al., 2011). This may not be sufficient from an adaptation perspective, particularly where users have a high risk aversion (Hinkel et al., 2019). In these cases, the emerging practice is to deliver a high-end scenario together with likely or probable scenarios (Ranger et al., 2013; Stammer et al., 2019; Nicholls et al., 2021a). High-end in this context means a scenario well above the likely projected SLR that cannot be excluded with present (limited) understanding of ice-sheet mass loss processes. Other uncertainty frameworks which consider probabilistic models or semi-quantitative information (Le Cozannet et al., 2017) might also be considered to deliver consistent sea-level information suited to the needs of various users. In its latest assessment report (AR6), the IPCC for the first time presented a “low likelihood high impact” scenario attached to the SSP5-8.5, showing that a sea level rise of 1.7 m by 2100 and more than 15 m by 2300 could not be excluded under high emissions (Fox-Kemper et al., 2021).
The impacts caused by SLR are wide-ranging – increased flooding, erosion, salinization and ultimately submergence – and there are many approaches to adaptation from planned or forced retreat, accommodation, hard and/or soft protection and even advance (Oppenheimer et al., 2019). The selected adaptation strategy will depend strongly on the local situation and decision context (Hinkel et al., 2019; Nicholls et al., 2021a). Some decisions such as beach nourishment operate at a decadal timescale and are easily adjusted over time, e.g., adding more or less sand (assuming sand resources are readily available). Hence these approaches can be easily adjusted to improved understanding as sea levels rise. In contrast, decisions such as building a nuclear power station or raising low-lying islands for urban development have long lead times, i.e., a century or more, and high risk aversion means there is strong interest in high-end SLR projections (e.g., Wilby et al., 2011; Brown et al., 2020): as the rate of SLR considered increases, the ability to protect may be overwhelmed for extreme cases linked to ice sheet collapse (Haasnoot et al., 2020). There are so many local situations that we do not a priori know the best approach to adaptation or the SLR information needs: the sea-level and adaptation scientists need to work together to understand the adaptation problem and what SLR science can presently provide that is relevant. Hence, the most useful SLR projections should be co-designed with users. This implies an iterative process in which all parties have to understand the needs, the possibilities to address these needs and the range of potential changes, and then jointly envision a future coastal adaptation service before elaborating projections (Figure 2).
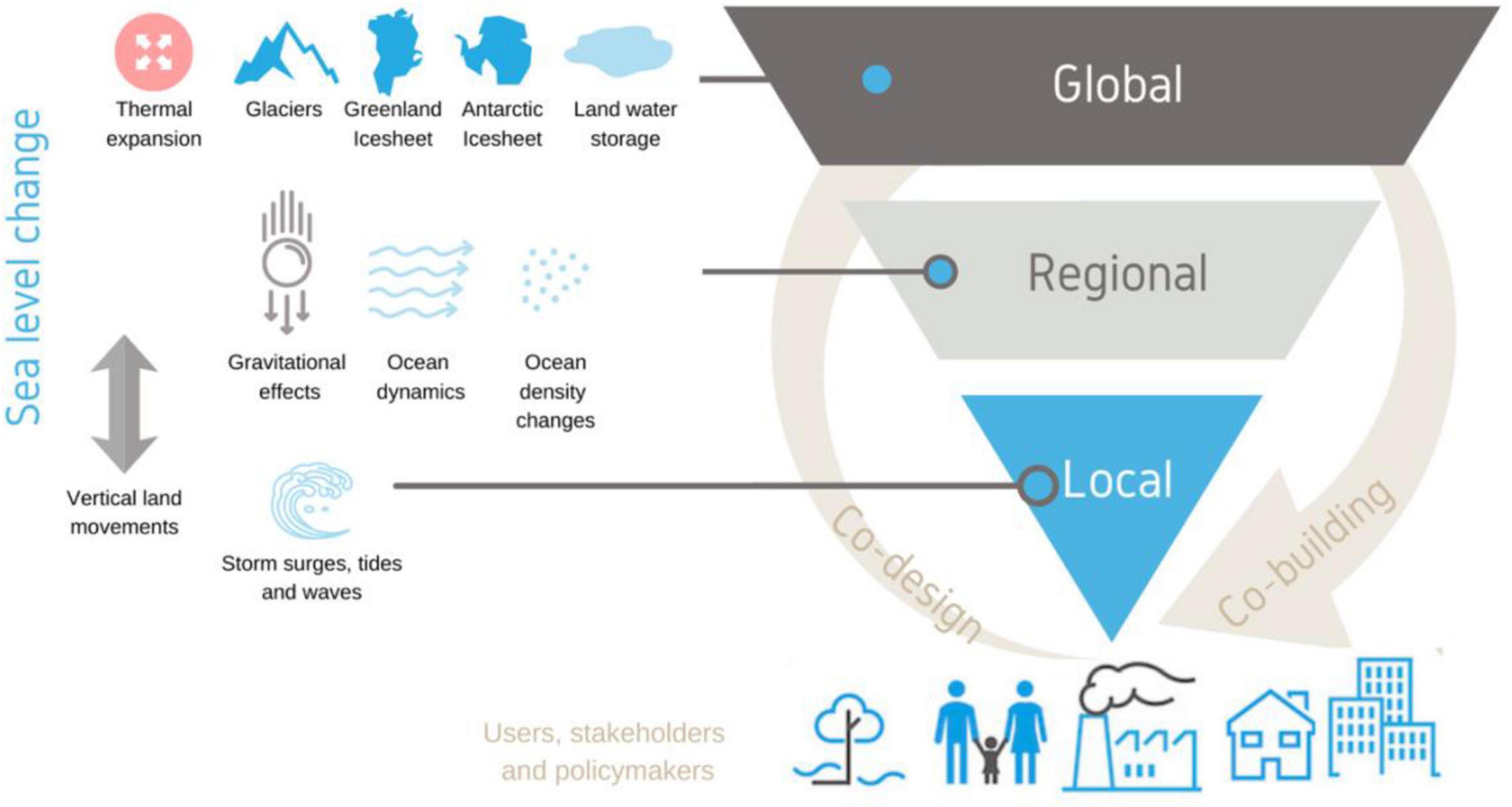
Figure 2. Providing reliable coastal adaptation services requires considering a large variety of processes on a wide range of scales. It should be iteratively co-designed and built with local users stakeholders, and policymakers.
Conclusion
This paper has considered how we can most effectively translate global understanding of sea level, including the major ice sheets and their uncertainties, to effective local climate services and coastal adaptation. It can be concluded that there are two important factors to consider: (i) to provide local sea-level projections, information from many different research disciplines needs to be transparently exchanged and combined, and (ii) to ensure usability for local stakeholders, sea-level information needs to be tailored to local requirements. Following these principles, as also adopted in the EU project “Projecting sea-level rise: from projections to local implications” (PROTECT), we encourage the formation of research consortia that cover the entire knowledge chain, ranging from ice sheet and ocean modeling, global, regional and extreme SLR projections, through to the analysis of SLR risks and adaptation needs and the co-design of sea-level information across the range of stakeholder needs. In this way global sea-level science can be linked to effective coastal climate services at the scale of risk and adaptation.
Data Availability Statement
The original contributions presented in the study are included in the article, further inquiries can be directed to the corresponding author.
Author Contributions
All authors contributed to the establishment of the concept defended here and applied in the PROTECT project. They also contributed to the writing of the manuscript and the design of the figures.
Funding
This publication was supported by PROTECT. This project has received funding from the European Union’s Horizon 2020 Research and Innovation Programme under grant agreement No 869304, PROTECT contribution number 27.
Conflict of Interest
The authors declare that the research was conducted in the absence of any commercial or financial relationships that could be construed as a potential conflict of interest.
Publisher’s Note
All claims expressed in this article are solely those of the authors and do not necessarily represent those of their affiliated organizations, or those of the publisher, the editors and the reviewers. Any product that may be evaluated in this article, or claim that may be made by its manufacturer, is not guaranteed or endorsed by the publisher.
Footnotes
- ^ Here, we follow the definition of glaciers as used in the 5th assessment report of the Intergovernmental Panel on Climate Change (IPCC, 2013): “A glacier is a perennial mass of land ice that originates from compressed snow, shows evidence of past or present flow (through internal deformation and/or sliding at the base) and is constrained by internal stress and friction at the base and sides. A glacier is maintained by accumulation of snow at high altitudes, balanced by melting at low altitudes and/or discharge into the sea. An ice mass of the same origin as glaciers, but of continental size, is called an ice sheet. For the purpose of simplicity, all ice masses other than ice sheets are referred to as glaciers.”
References
Agosta, C., Amory, C., Kittel, C., Orsi, A., Favier, V., Gallée, H., et al. (2019). Estimation of the Antarctic surface mass balance using the regional climate model MAR (1979–2015) and identification of dominant processes. Cryosphere 13, 281–296. doi: 10.5194/tc-13-281-2019
Allison, I., Paul, F., Colgan, W., and King, M. (2021). “Chapter 20 – Ice sheets, glaciers, and sea level,” in Snow and Ice-Related Hazards, Risks, and Disasters (Second Edition), eds W. Haeberli and C. Whiteman (New York, NY: Elsevier), 707–740. doi: 10.1016/B978-0-12-817129-5.00013-5
Arns, A., Wahl, T., Dangendorf, S., and Jensen, J. (2015). The impact of sea level rise on storm surge water levels in the northern part of the german bight. Coast. Eng. 96, 118–131. doi: 10.1016/j.coastaleng.2014.12.002
Bakker, A. M. R., Louchard, D., and Keller, K. (2017). Sources and implications of deep uncertainties surrounding sea-level projections. Climat. Change 140, 339–347. doi: 10.1007/s10584-016-1864-1
Bamber, J. L., Oppenheimer, M., Kopp, R. E., Aspinall, W. P., and Cooke, R. M. (2019). Ice sheet contributions to future sea-level rise from structured expert judgment. Proc. Natl. Acad. Sci. U.S.A. 116, 11195–11200. doi: 10.1073/pnas.1817205116
Bamber, J. L., Westaway, R. M., Marzeion, B., and Wouters, B. (2018). The land ice contribution to sea level during the satellite era. Environ. Res. Lett. 13:063008. doi: 10.1088/1748-9326/aac2f0
Barletta, V. R., Bevis, M., Smith, B. E., Wilson, T., Brown, A., Bordoni, A., et al. (2018). Observed rapid bedrock uplift in amundsen sea embayment promotes ice-sheet stability. Science 360, 1335–1339. doi: 10.1126/science.aao1447
Brown, S., Wadey, M. P., Nicholls, R. J., Shareef, A. A., Khaleel, Z., Hinkel, J., et al. (2020). Land raising as a solution to sea-level rise: an analysis of coastal flooding on an artificial Island in the Maldives. J. Flood Risk Manag. 13:e12567. doi: 10.1111/jfr3.12567
DeConto, R. M., and Pollard, D. (2016). Contribution of Antarctica to past and future sea-level rise. Nature 531, 591–597. doi: 10.1038/nature17145
DeConto, R. M., Pollard, D., Alley, R. B., Velicogna, I., Gasson, E., Gomez, N., et al. (2021). ‘The Paris climate agreement and future sea-level rise from Antarctica’. Nature 593, 83–89. doi: 10.1038/s41586-021-03427-0
Dutrieux, P., De Rydt, J., Jenkins, A., Holland, P. R., Ha, H. K., Lee, S. H., et al. (2014). Strong sensitivity of pine island ice-shelf melting to climatic variability. Science 343, 174–178. doi: 10.1126/science.1244341
Edwards, T. L., Brandon, M. A., Durand, G., Edwards, N. R., Golledge, N. R., Holden, P. B., et al. (2019). Revisiting Antarctic ice loss due to marine ice-cliff instability. Nature 566, 58–64. doi: 10.1038/s41586-019-0901-4
Farinotti, D., Huss, M., Fürst, J. J., Landmann, J., Machguth, H., Maussion, F., et al. (2019). A consensus estimate for the ice thickness distribution of all glaciers on earth. Nat. Geosci. 12, 168–173. doi: 10.1038/s41561-019-0300-3
Fox-Kemper, B., Hewitt, H. T., Xiao, C., Aðalgeirsdóttir, G., Drijfhout, S. S., Edwards, T. L., et al. (2021). Ocean, Cryosphere and Sea Level Change. Climate Change 2021: The Physical Science Basis. Contribution of Working Group I to the Sixth Assessment Report of the Intergovernmental Panel on Climate Change, Cambridge University Press.
Gagliardini, O., Durand, G., Zwinger, T., Hindmarsh, R. C. A., and Le Meur, E. (2010). Coupling of ice-shelf melting and buttressing is a key process in ice-sheets dynamics. Geophys. Res. Lett. 37:L14501. doi: 10.1029/2010GL043334
Goelzer, H., Nowicki, S., Payne, A., Larour, E., Seroussi, H., Lipscomb, W. H., et al. (2020). The future sea-level contribution of the greenland ice sheet: a multi-model ensemble study of ISMIP6. Cryosphere 14, 3071–3096. doi: 10.5194/tc-14-3071-2020
Gudmundsson, G. H., Krug, J., Durand, G., Favier, L., and Gagliardini, O. (2012). The stability of grounding lines on retrograde slopes. Cryosphere 6, 1497–1505. doi: 10.5194/tc-6-1497-2012
Haasnoot, M., Kwadijk, J., van Alphen, J., Le Bars, D., van den Hurk, B., Diermanse, F., et al. (2020). Adaptation to uncertain sea-level rise; how uncertainty in Antarctic mass-loss impacts the coastal adaptation strategy of the Netherlands. Environ. Res. Lett. 15:034007. doi: 10.1088/1748-9326/ab666c
Haigh, I. D., Pickering, M. D., Green, J. A. M., Arbic, B. K., Arns, A., Dangendorf, S., et al. (2020). The tides they are A-Changin’: a comprehensive review of past and future nonastronomical changes in tides, their driving mechanisms, and future implications. Rev. Geophys. 58:e2018RG000636. doi: 10.1029/2018RG000636
Hanna, E., Fettweis, X., and Hall, R. J. (2018). Brief communication: recent changes in summer greenland blocking captured by none of the CMIP5 models. Cryosphere 12, 3287–3292. doi: 10.5194/tc-12-3287-2018
Hinkel, J., Church, J. A., Gregory, J. M., Lambert, E., Le Cozannet, G., and Lowe, J. (2019). Meeting user needs for sea level rise information: a decision analysis perspective. Earths Future 7, 320–337. doi: 10.1029/2018EF001071
Hock, R., Bliss, A., Marzeion, B., Giesen, R. H., Hirabayashi, Y., Huss, M., et al. (2019a). GlacierMIP – a model intercomparison of global-scale glacier mass-balance models and projections. J. Glaciol. 65, 453–467. doi: 10.1017/jog.2019.22
Hock, R., Rasul, G., Adler, C., Cáceres, B., Gruber, S., Hirabayashi, Y., et al. (2019b). High Mountain Areas: IPCC Special Report on the Ocean and Cryosphere in a Changing Climate. The Intergovernmental Panel on Climate Change. Geneva: IPCC, 2019.
Hugonnet, R., McNabb, R., Berthier, E., Menounos, B., Nuth, C., Girod, L., et al. (2021). Accelerated global glacier mass loss in the early twenty-first century. Nature 592, 726–731. doi: 10.1038/s41586-021-03436-z
IPCC (2007). Climate Change 2007: The Physical Science Basis. Contribution of Working Group I to the Fourth Assessment Report of the Intergovernmental Panel on Climate Change. Cambridge: Cambridge University Press.
IPCC (2013). Climate Change 2013: The Physical Science Basis: Working Group I Contribution to the Fifth Assessment Report of the Intergovernmental Panel on Climate Change. Cambridge: Cambridge University Press, 2014.
IPCC (2019). “Polar Regions,” in IPCC Special Report on the Ocean and Cryosphere in a Changing Climate (Geneva: IPCC).
IPCC (2021). Climate Change 2021: The Physical Science Basis. Contribution of Working Group I to the Sixth Assessment Report of the Intergovernmental Panel on Climate Change. Cambridge: Cambridge University Press, 2021.
King, M. D., Howat, I. M., Candela, S. G., Jeong, S., Noh, M. J., Noël, B., et al. (2020). Dynamic ice loss from the Greenland ice sheet driven by sustained glacier retreat. Commun. Earth Environ. 1:1. doi: 10.1038/s43247-020-0001-2
Kopp, R. E., Gilmore, E. A., Little, C. M., Lorenzo-Trueba, J., Ramenzoni, V. C., and Sweet, W. V. (2019). Usable science for managing the risks of sea-level rise. Earths Future 7, 1235–1269. doi: 10.1029/2018EF001145
Kopp, R. E., Horton, R. M., Little, C. M., Mitrovica, J. X., Oppenheimer, M., Rasmussen, D. J., et al. (2014). Probabilistic 21st and 22nd century sea-level projections at a global network of tide gauge sites. Earths Future 2, 383–406. doi: 10.1002/2014EF000239
Le Cozannet, G. L., Manceau, J., and Rohmer, J. (2017). Bounding probabilistic sea-level projections within the framework of the possibility theory. Environ. Res. Lett. 12:014012. doi: 10.1088/1748-9326/aa5528
MacFerrin, M., Machguth, H., van As, D., Charalampidis, C., Stevens, C. M., Heilig, A., et al. (2019). Rapid expansion of Greenland’s low-permeability ice slabs. Nature 573, 403–407. doi: 10.1038/s41586-019-1550-3
Marzeion, B., Hock, R., Anderson, B., Bliss, A., Champollion, N., Fujita, K., et al. (2020). Partitioning the uncertainty of ensemble projections of global glacier mass change. Earths Future 8:e2019EF001470. doi: 10.1029/2019EF001470
Marzeion, B., Kaser, G., Maussion, F., and Champollion, N. (2018). Limited influence of climate change mitigation on short-term glacier mass loss. Nat. Climate Change 8, 305–308. doi: 10.1038/s41558-018-0093-1
Mastrandrea, M. D., Mach, K. J., Plattner, G. K., Edenhofer, O., Stocker, T. F., Field, C. B., et al. (2011). The IPCC AR5 guidance note on consistent treatment of uncertainties: a common approach across the working groups. Climat. Change 108:675. doi: 10.1007/s10584-011-0178-6
Mitrovica, J. X., Tamisiea, M. E., Davis, J. L., and Milne, G. A. (2001). Recent mass balance of polar ice sheets inferred from patterns of global sea-level change. Nature 409, 1026–1029. doi: 10.1038/35059054
Morlighem, M., Rignot, E., Binder, T., Blankenship, D., Drews, R., Eagles, G., et al. (2020). Deep glacial troughs and stabilizing ridges unveiled beneath the margins of the Antarctic Ice Sheet. Nat. Geosci. 13, 132–137. doi: 10.1038/s41561-019-0510-8
Mouginot, J., Rignot, E., Bjørk, A. A., van den Broeke, M., Millan, R., Morlighem, M., et al. (2019). Forty-six years of Greenland ice sheet mass balance from 1972 to 2018. Proc. Natl. Acad. Sci. U.S.A. 116, 9239–9244. doi: 10.1073/pnas.1904242116
Mouginot, J., Rignot, E., and Scheuchl, B. (2014). Sustained increase in ice discharge from the Amundsen Sea embayment, West Antarctica, from 1973 to 2013. Geophys. Res. Lett. 41, 1576–1584. doi: 10.1002/2013GL059069
Neumann, B., Vafeidis, A. T., Zimmermann, J., and Nicholls, R. J. (2015). Future coastal population growth and exposure to sea-level rise and coastal flooding – a global assessment. PLoS One 10:e0118571. doi: 10.1371/journal.pone.0118571
Nicholls, R. J., Hanson, S. E., Lowe, J. A., Slangen, A. B. A., Wahl, T., Hinkel, J., et al. (2021a). Integrating new sea-level scenarios into coastal risk and adaptation assessments: an ongoing process. WIREs Climate Change 12:e706. doi: 10.1002/wcc.706
Nicholls, R. J., Lincke, D., Hinkel, J., Brown, S., Vafeidis, A. T., Meyssignac, B., et al. (2021b). A global analysis of subsidence, relative sea-level change and coastal flood exposure. Nat. Climate Change 11, 338–342. doi: 10.1038/s41558-021-00993-z
Noël, B., van Kampenhout, L., Lenaerts, J. T. M., van de Berg, W. J., and van den Broeke, M. R. (2021). A 21st century warming threshold for sustained greenland ice sheet mass loss. Geophys. Res. Lett. 48:e2020GL090471. doi: 10.1029/2020GL090471
Oppenheimer, M., Glavovic, B. C., Hinkel, J., van de Wal, R., Magnan, A. K., Abd-Elgawad, A., et al. (2019). Sea Level Rise and Implications for Low-Lying Islands, Coasts and Communities. IPCC Special Report on the Ocean and Cryosphere in a Changing Climate, Vol. 126, Geneva: IPCC.
Pattyn, F., and Morlighem, M. (2020). The Uncertain Future of the Antarctic Ice Sheet. Science 367, 1331–1335. doi: 10.1126/science.aaz5487
Pattyn, F., Ritz, C., Hanna, E., Asay-Davis, X., DeConto, R., Durand, G., et al. (2018). ‘The Greenland and Antarctic ice sheets under 1.5 °C global warming. Nat. Climate Change 8, 1053–1061. doi: 10.1038/s41558-018-305-308
Peltier, W. R., Argus, D. F., and Drummond, R. (2015). Space geodesy constrains ice age terminal deglaciation: the global ICE-6G_C (VM5a) model. J. Geophys. Res. Solid Earth 120, 450–487. doi: 10.1002/2014JB011176
Ranger, N., Reeder, T., and Lowe, J. (2013). Addressing “Deep” uncertainty over long-term climate in major infrastructure projects: four innovations of the Thames Estuary 2100 Project. EURO J. Decis. Process. 1, 233–262. doi: 10.1007/s40070-013-0014-5
Rignot, E., Mouginot, J., Scheuchl, B., van den Broeke, M., van Wessem, M. J., and Morlighem, M. (2019). ‘Four decades of Antarctic Ice sheet mass balance from 1979–2017. Proc. Natl. Acad. Sci. U.S.A. 116, 1095–1103. doi: 10.1073/pnas.1812883116
Rohmer, J., Le Cozannet, G., and Manceau, J.-C. (2019). Addressing ambiguity in probabilistic assessments of future coastal flooding using possibility distributions. Climatic Change 155, 95–109. doi: 10.1007/s10584-019-02443-4
Rohmer, J., Lincke, D., Hinkel, J., Le Cozannet, G., Lambert, E., and Vafeidis, A. T. (2021). Unravelling the importance of uncertainties in global-scale coastal flood risk assessments under sea level rise. Water 13:774.
Rosenzweig, C., Solecki, W. D., Blake, R., Bowman, M., Faris, C., Gornitz, V., et al. (2011). Developing coastal adaptation to climate change in the New York City infrastructure-shed: process, approach, tools, and strategies. Climat. Change 106, 93–127. doi: 10.1007/s10584-010-0002-8
Rosier, S. H. R., Reese, R., Donges, J. F., De Rydt, J., Gudmundsson, G. H., and Winkelmann, R. (2021). The tipping points and early warning indicators for Pine Island Glacier, West Antarctica. Cryosphere 15, 1501–1516. doi: 10.5194/tc-15-1501-2021
Scambos, T., Fricker, H. A., Liu, C.-C., Bohlander, J., Fastook, J., Sargent, A., et al. (2009). Ice shelf disintegration by plate bending and hydro-fracture: satellite observations and model results of the 2008 Wilkins Ice shelf break-Ups. Earth Planet. Sci. Lett. 280, 51–60. doi: 10.1016/j.epsl.2008.12.027
Schoof, C. (2007). Ice sheet grounding line dynamics: steady states, stability, and hysteresis. J. Geophys. Res. Earth Surf. 112:F03S28. doi: 10.1029/2006JF000664
Seroussi, H., Nowicki, S., Payne, A. J., Goelzer, H., Lipscomb, W. H., Abe-Ouchi, A., et al. (2020). ISMIP6 Antarctica: a multi-model ensemble of the Antarctic Ice sheet evolution over the 21st Century. Cryosphere 14, 3033–3070. doi: 10.5194/tc-14-3033-2020
Shepherd, A., Ivins, E., Rignot, E., Smith, B., van den Broeke, M., Velicogna, I., et al. (2018). Mass balance of the Antarctic Ice sheet from 1992 to 2017. Nature 558, 219–222. doi: 10.1038/s41586-018-0179-y
Shepherd, A., Ivins, E., Rignot, E., Smith, B., van den Broeke, M., Velicogna, I., et al. (2021). Antarctic and Greenland Ice Sheet Mass Balance 1992-2020 for IPCC AR6’. Text/Plain,Text/CSV. London: UK Polar Data Centre, Natural Environment Research Council, UK Research & Innovation. doi: 10.5285/77B64C55-7166-4A06-9DEF-2E400398E452
Simonsen, S. B., Barletta, V. R., Colgan, W. T., and Sørensen, L. S. (2021). Greenland Ice sheet mass balance (1992–2020) from calibrated radar altimetry. Geophys. Res. Lett. 48:e2020GL091216. doi: 10.1029/2020GL091216
Slangen, A. B. A., Carson, M., Katsman, C. A., van de Wal, R. S. W., Köhl, A., Vermeersen, L. L. A., et al. (2014). Projecting twenty-first century regional sea-level changes. Climat. Change 124, 317–332. doi: 10.1007/s10584-014-1080-9
Stammer, D., van de Wal, R. S. W., Nicholls, R. J., Church, J. A., Le Cozannet, G., Lowe, J. A., et al. (2019). Framework for high-end estimates of sea level rise for stakeholder applications. Earths Future 7, 923–938. doi: 10.1029/2019EF001163
Straneo, F., and Heimbach, P. (2013). North Atlantic warming and the retreat of Greenland’s outlet Glaciers. Nature 504, 36–43. doi: 10.1038/nature12854
Talke, S. A., and Jay, D. A. (2020). Changing tides: the role of natural and anthropogenic factors. Annu. Rev. Mar. Sci. 12, 121–151. doi: 10.1146/annurev-marine-010419-010727
Toimil, A., Camus, P., Losada, I. J., Le Cozannet, G., Nicholls, R. J., Idier, D., et al. (2020). Climate change-driven coastal erosion modelling in temperate sandy beaches: methods and uncertainty treatment. Earth Sci. Rev. 202:103110. doi: 10.1016/j.earscirev.2020.103110
van Wessem, J. M., van de Berg, W. J., Noël, B. P. Y., van Meijgaard, E., Amory, C., Birnbaum, G., et al. (2018). Modelling the climate and surface mass balance of polar Ice sheets using RACMO2 – Part 2: Antarctica (1979–2016). Cryosphere 12, 1479–1498. doi: 10.5194/tc-12-1479-2018
Vaughan, D. G. (2008). West Antarctic Ice sheet collapse – the fall and rise of a Paradigm. Climat. Change 91, 65–79. doi: 10.1007/s10584-008-9448-3
Weertman, J. (1974). Stability of the junction of an Ice Sheet and an Ice Shelf’. J. Glaciol. 13, 3–11. doi: 10.3189/S0022143000023327
Wilby, R. L., Nicholls, R. J., Warren, R., Wheater, H. S., Clarke, D., and Dawson, R. J. (2011). Keeping nuclear and other coastal sites safe from climate change. Proc. Inst. Civil Eng. 164, 129–136. doi: 10.1680/cien.2011.164.3.129
Wong, P. P., Losada, I. J., Gattuso, J.-P., Hinkel, J., Khattabi, A., McInnes, K. L., et al. (2014). Coastal systems and low-lying areas. Climate Change 2104, 361–409.
Woodworth, P. L., Melet, A., Marcos, M., Ray, R. D., Wöppelmann, G., Sasaki, Y. N., et al. (2019). Forcing factors affecting sea level changes at the coast. Surv. Geophys. 40, 1351–1397. doi: 10.1007/s10712-019-09531-1
Wöppelmann, G., and Marcos, M. (2016). Vertical land motion as a key to understanding sea level change and variability. Rev. Geophys. 54, 64–92. doi: 10.1002/2015RG000502
Zemp, M., Huss, M., Thibert, E., Eckert, N., McNabb, R., Huber, J., et al. (2019). Global glacier mass changes and their contributions to sea-level rise from 1961 to 2016. Nature 568, 382–386. doi: 10.1038/s41586-019-1071-0
Keywords: sea-level rise, Antarctic, Greenland, glaciers, local impact
Citation: Durand G, van den Broeke MR, Le Cozannet G, Edwards TL, Holland PR, Jourdain NC, Marzeion B, Mottram R, Nicholls RJ, Pattyn F, Paul F, Slangen ABA, Winkelmann R, Burgard C, van Calcar CJ, Barré J-B, Bataille A and Chapuis A (2022) Sea-Level Rise: From Global Perspectives to Local Services. Front. Mar. Sci. 8:709595. doi: 10.3389/fmars.2021.709595
Received: 14 May 2021; Accepted: 22 December 2021;
Published: 20 January 2022.
Edited by:
Riccardo Briganti, University of Nottingham, United KingdomReviewed by:
Francesco De Leo, Cal Poly San Luis Obispo College of Engineering, United StatesGiovanni Besio, University of Genoa, Italy
Copyright © 2022 Durand, van den Broeke, Le Cozannet, Edwards, Holland, Jourdain, Marzeion, Mottram, Nicholls, Pattyn, Paul, Slangen, Winkelmann, Burgard, van Calcar, Barré, Bataille and Chapuis. This is an open-access article distributed under the terms of the Creative Commons Attribution License (CC BY). The use, distribution or reproduction in other forums is permitted, provided the original author(s) and the copyright owner(s) are credited and that the original publication in this journal is cited, in accordance with accepted academic practice. No use, distribution or reproduction is permitted which does not comply with these terms.
*Correspondence: Gaël Durand, Z2FlbC5kdXJhbmRAdW5pdi1ncmVub2JsZS1hbHBlcy5mcg==