- 1Department of Physical Sciences, Virginia Institute of Marine Science, William & Mary, Gloucester Point, VA, United States
- 2Environmental Studies Program/Geology, Randolph-Macon College, Ashland, VA, United States
- 3Department of Biological Sciences, Columbian College of Arts & Sciences, George Washington University, Washington, DC, United States
- 4Stantec, Williamsburg, VA, United States
- 5Office of Research and Advisory Services, Virginia Institute of Marine Science, William & Mary, Gloucester Point, VA, United States
- 6Stantec, Rockledge, FL, United States
Barrier islands and their backbarrier saltmarshes have a reciprocal relationship: aeolian and storm processes transport sediment from the beaches and dunes to create and build marshes along the landward fringe of the island. In turn, these marshes exert a stabilizing influence on the barrier by widening the barrier system and forming a platform onto which the island migrates, consequently slowing landward barrier migration and inhibiting storm breaching. Here, we present a novel framework for applying these natural interdependencies to managing coastal systems and enhancing barrier-island resilience. Further, we detail application of these principles through a case study of the design of a marsh creation project that showcases the interdisciplinary engagement of scientists, engineers, stakeholders, and policymakers. Specifically, we describe: (1) the ecologic, sedimentologic, stratigraphic, and morphologic data obtained from the southern 4 km of Cedar Island (Virginia, United States) and nearby backbarrier tidal channels, tidal flats, and flood-tidal deltas, and (2) the use of those data to develop an engineering and design plan for the construction of a high (46 ha) and low (42 ha) fringing marsh platform located behind the island, proximal to a former ephemeral inlet. Additionally, we chronicle the process used to narrow five initial alternative designs to the optimal final plan. This process involved balancing best-available existing science and models, considering design and financial constraints, identifying stakeholder preferences, and maximizing restoration benefits of habitat provision and shoreline protection. Construction of this marsh would: (1) provide additional habitat and ecosystem benefits, (2) slow the rapid migration (up to 15 m/yr at present) of the barrier island, and (3) hinder island breaching. Ultimately, this project – presently at the final design and permitting stage – may enhance the storm and sea-level rise resilience of the island, backbarrier marshes and lagoons, and the mainland town community; and provide an example of a novel science-based approach to coastal resilience that could be applied to other global barrier settings.
Introduction
Coastal saltmarshes are long recognized for their ability to denitrify coastal waters; serve as habitat for birds, fish, and invertebrates; store coastal blue carbon; attenuate storm wave energy; reduce coastal erosion; and shelter mainlands from flooding (e.g., Howes et al., 1996; Pennings and Bertness, 2001; Shepard et al., 2011; Fagherazzi, 2014; Leonardi et al., 2018; Najjar et al., 2018). Backbarrier marshes, particularly those situated directly landward of barrier islands, provide an additional and largely underrecognized function: they help to naturally stabilize fronting islands. By filling accommodation (the three-dimensional and subaqueous volume between the lagoon floor and mean high water available for sediment to accumulate) landward of the island, saltmarshes reduce backbarrier tidal prism (FitzGerald et al., 2008, 2018) and provide a platform upon which beach and dune systems can migrate and perch without the loss of sand to lagoon infilling. In this manner, backbarrier saltmarshes conserve sand and widen the overall barrier-island system, slowing barrier-island migration (Walters et al., 2014; Lorenzo-Trueba and Mariotti, 2017) and protecting against storm breaching (Cañizares and Irish, 2008; Morton, 2008; Nienhuis et al., 2021; Figure 1). As such, backbarrier marshes play an important role in the resiliency of the fronting barrier islands to sea-level rise.
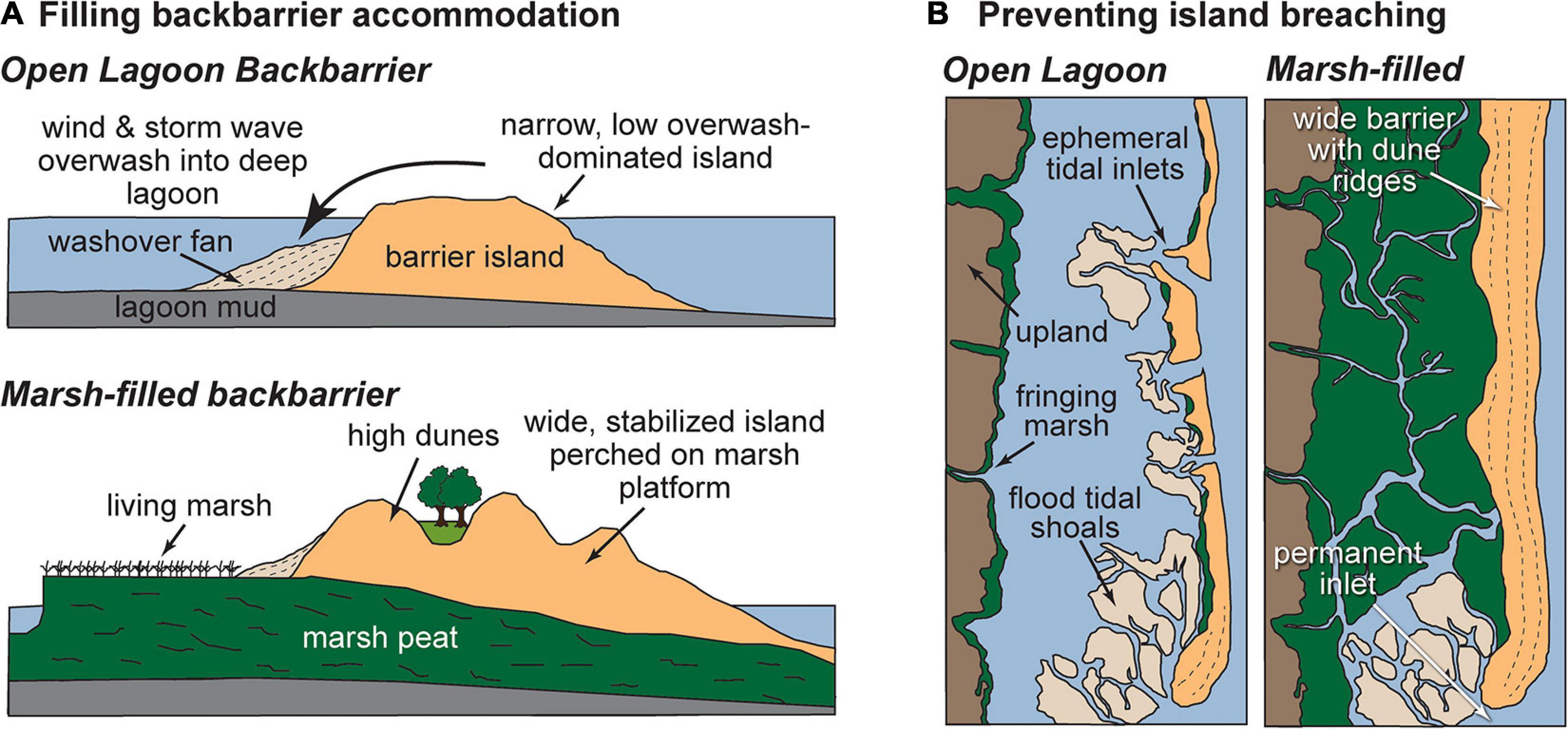
Figure 1. Conceptual model of barrier-saltmarsh couplings along a landward-migrating barrier island. (A) Marsh situated along the landward margins of barrier islands fills backbarrier accommodation, providing a platform upon which the barrier can perch, thereby slowing its migration and allowing sand that would have filled the adjacent lagoon through overwash to build the island vertically. (B) Backbarrier marsh reduces backbarrier tidal prism and widens the barrier-marsh system, reducing the likelihood of breaching and formation of ephemeral tidal inlets.
In a reciprocal fashion, barrier islands enhance backbarrier marsh resilience to sea-level rise. The backbarrier marsh receives barrier and nearshore sediment directly through aeolian (Rodriguez et al., 2013), tidal (Castagno et al., 2018), and wave-driven transport (overwash; Dolan and Godfrey, 1973; Walters and Kirwan, 2016). Specifically, storms may be responsible for contributing inorganic sediment to saltmarshes in thicknesses up to several times that of normal annual accumulation (Rejmanek et al., 1988; Cahoon et al., 1995; Tweel and Turner, 2014). This sediment can enhance marsh growth by providing a fresh supply of nutrients and new sediment for root occupation (Baustian and Mendelssohn, 2015). These conditions allow a fringing marsh landward of the island to better maintain its tidal elevation and, consequently, enhance resilience of the barrier-adjacent marsh to sea-level rise (Nyman et al., 1995).
Here, we propose a framework for applying these natural interdependent feedbacks to management of coupled saltmarsh-barrier-island systems. Specifically, we present a case study of the design and selection process of an innovative, science- and nature-based marsh construction project along the landward side of the southern 4 km of Cedar Island (Eastern Shore, Virginia, United States; Figure 2A). This vulnerable part of the island (shoreline reach) is offset landward by nearly 500 m as compared with the northern part of the island, reflecting more rapid landward migration along this reach during the last several decades (Figure 2B). This configuration mimics other islands in the Virginia Barrier Island chain (e.g., Metompkin), where the presence or absence of backbarrier fringing marsh influences shoreline offsets along individual islands (Walters et al., 2014). An additional driver of accelerated migration along southern Cedar Island at this location is repeated storm-induced breaching (Figure 3).
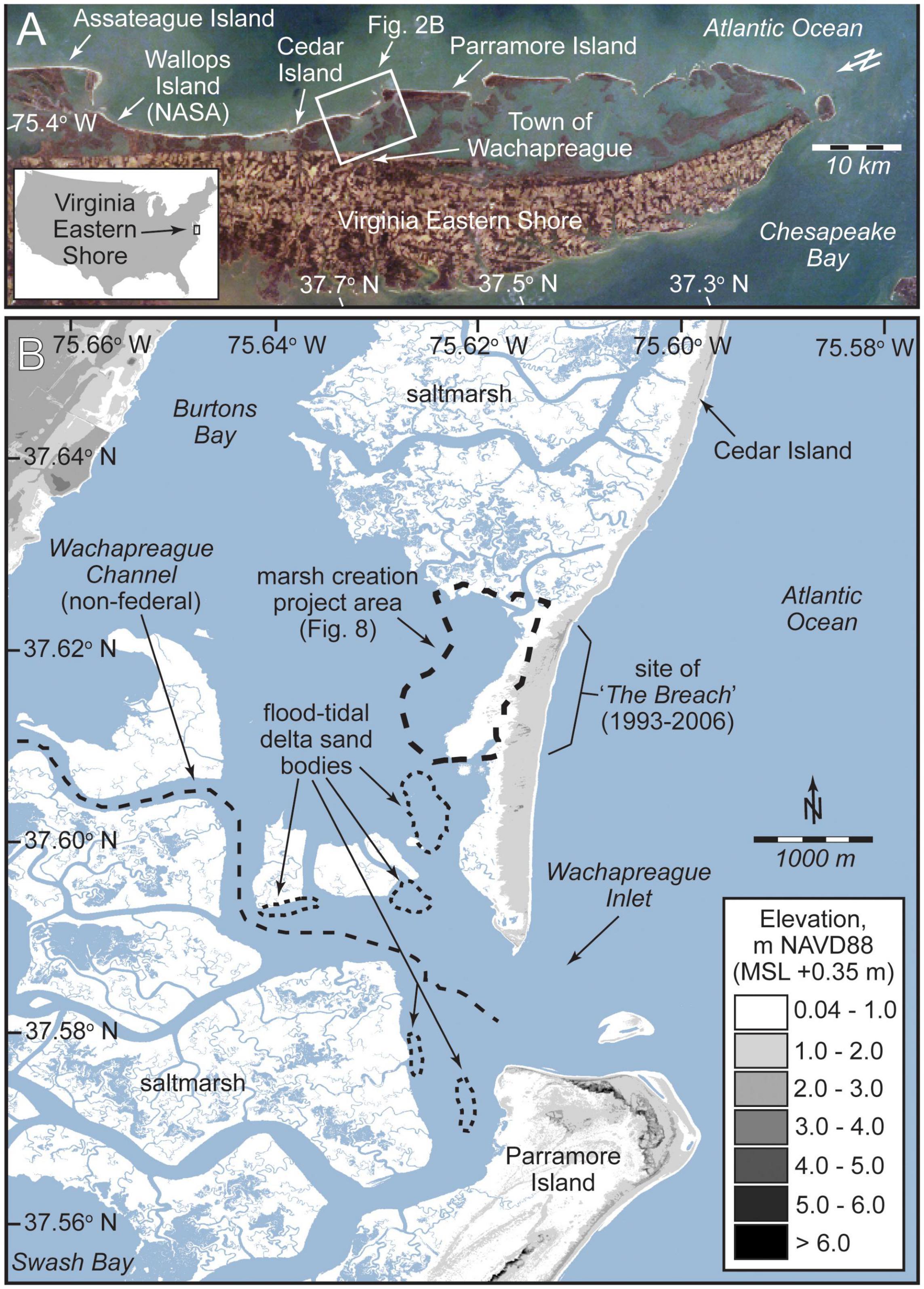
Figure 2. Project study site. (A) The Virginia Eastern Shore and its barrier islands. Modified from Deaton et al. (2017). (B) Southern Cedar Island and vicinity, showing key features, channels, flood-tidal delta shoals, and the marsh creation project area. Digital elevation model is from topographic and shallow bathymetric lidar [OCM (National Oceanic and Atmospheric Administration Office for Coastal Management) Partners, 2020c].
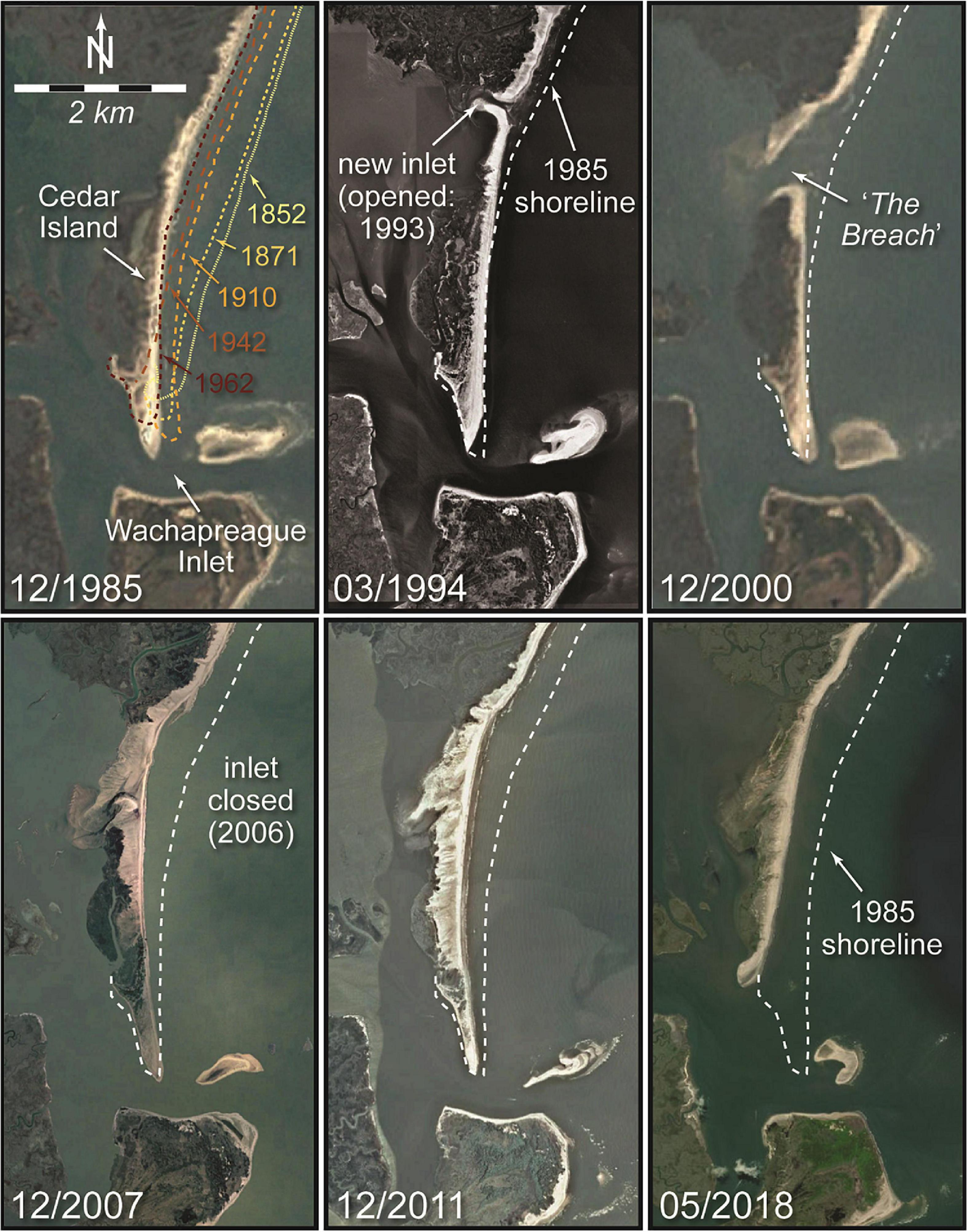
Figure 3. Historic satellite orthoimagery from southern Cedar Island, showing physical change over a period of 33 years (1985–2018). Note the combination of former island breaching (1993–2006) and more recent shortening due to widening of Wachapreague Inlet (2011–2018). The marsh restoration site is located landward of the former inlet (locally called “The Breach”). Imagery sources: Landsat (1985, 2000), US Geological Survey (1994), the Commonwealth of Virginia (2007) and the National Agriculture Imagery Program of the US Department of Agriculture’s Farm Service Agency (2011, 2018). Historical shorelines overlain on 1985 image are from historical maps and NOAA t-sheets, digitized by Himmelstoss et al. (2010).
We detail the conceptual foundation, design approach, and preliminary engineering design plans – along with the field and laboratory data collection and analysis that underpin these – for the creation of 88 ha of fringing marsh designed to prevent future erosion and breaching of this vulnerable reach. Serving as a full-scale validation of modeled island-marsh couplings (Walters et al., 2014; Lorenzo-Trueba and Mariotti, 2017; Lauzon et al., 2018), we designed the proposed marsh construction project to slow landward island migration and reduce the likelihood of future breaching. Stabilizing the barrier will have the synergistic effect of enhancing the mainland’s resiliency by providing a critical storm barrier for the mainland and decreasing mainland storm flooding.
In this paper, we first review the morphologic changes that have occurred historically along Cedar Island in response to storms and sea-level rise and introduce a framework for mitigating island degradation through marsh construction. We then present new ecologic, sedimentologic, stratigraphic, and morphologic data used to develop five concept design plans. Finally, we showcase the process of final design plan selection which involved balancing the best-available science, stakeholder needs, anticipated project effectiveness, and funding and permitting constraints. This process produced transferable and scalable lessons for nature-based, coupled marsh/barrier-system restoration.
Case Study: Cedar Island, Virginia, United States
Cedar Island is an undeveloped, approximately 11-km long, 200–250-m wide, mixed-energy barrier island (Shawler et al., 2019) located offshore of the southern Delmarva Peninsula (Mid-Atlantic Coast) (Figure 2). The low elevation (ranging from sea level to 20 m) Virginia portion of the Delmarva Peninsula is rural, with a population of ∼45,000 that is underserved and threatened by climate change, relative sea-level rise, and storms (A-NPDC, 2015). The 13 barrier islands that front the southern Delmarva comprise one of the fastest-changing barrier systems in the world: it experiences an average landward shoreline migration rate of 5–7 m yr–1 (Deaton et al., 2017) in response to an energetic storm regime (Fenster and Dolan, 1994; Fenster et al., 2003; Hayden and Hayden, 2003), high rates of relative sea-level rise (3.5–5.0 mm yr–1; Boon and Mitchell, 2015), and sediment supply (Shawler et al., 2021b). Twelve of these islands are presently undeveloped, and have no history of soft (e.g., beach nourishment) or hard (e.g., seawalls, bulkheads, groins or jetties) shoreline stabilization projects. These islands and the bays behind them serve as centers of commerce (aquaculture, tourism) and recreation. They have also been focal areas for ecological restoration and research through partnerships between academics, non-governmental organizations, and local and state governments. The Nature Conservancy, which owns all or parts of many of these islands, have protected ∼55,000 ha (including >110 km of beachfront) of the islands and backbarrier marshes, lagoons, creeks, and tidal flats. Combined efforts of non-governmental organizations, academia, and government have led to the restoration of approximately 24 ha of oyster reef; designation of 810 ha of oyster reef as sanctuaries; establishment of 3,367 ha of eelgrass meadows (the largest seagrass restoration project in the world; Orth et al., 2020); and the successful reintroduction of the bay scallop, a species that locally disappeared 80 years ago due to loss of seagrass habitat (Oreska et al., 2017).
A mixture of federal and state agencies, non-governmental organizations, and private landowners own and manage Cedar Island and nearby backbarrier marshes. Structures are limited to remnants of a US Coast Guard station active from the late 1800s to the mid-1900s near the northern end of the island. Along the southern 3 km of the island, several private homes were present from the 1980s to 2010s, but all have been destroyed by storms or preemptively removed by homeowners because of erosion threats. Cedar Island is among the most dynamic of the Delmarva Peninsula barrier islands: following a period of progradation along its northern end prior to ca. 1850 (Shawler et al., 2019), Cedar Island has undergone landward migration at an island-averaged rate of 5 m yr–1 from the 1850s through 2010, accelerating to 11 m yr–1 between 1980 and 2010 (Deaton et al., 2017). This has occurred predominantly through overwash, island breaching, and – to a lesser extent – aeolian transport through the island’s segmented foredune ridge. In particular, Nebel et al. (2013) observed that the southern 4 km of Cedar Island retreated by ∼25 m as a result of a single tropical storm (Ernesto) in 2013. Repeated breaching along this same island segment formed a series of ephemeral tidal inlets over the last 60 years (Hanley and McBride, 2011). While open, these inlets allowed for increased wave energy reaching the backbarrier, contributing to the loss of approximately 1,000 ha of saltmarsh during the last 150 years (Nebel et al., 2013; Deaton et al., 2017).
The youngest of these ephemeral tidal inlets, known locally as “The Breach,” first opened in 1993, closed for 6 months in 1997, and then reopened, migrated south, rotated counter-clockwise, and closed in 2006 (Moyer, 2007; Hanley et al., 2015; Figure 3). This breach and attendant formation of inlet shoals sequestered sediment moving in the southerly longshore transport system which, in turn, resulted in accelerated degradation, landward migration, and shortening of the southern end of the island (Figure 3). During this period, tidal flows were altered, and greater storm surges exacerbated mainland flooding. Increased wave energy entering through The Breach resulted in higher wave energy in the proximal backbarrier, further accelerating marsh-edge erosion. Conspicuously, The Breach formed at a location where no marsh existed (and still does not exist) directly behind the island, a configuration reflecting greater lagoonal accommodation resulting from deeper antecedent substrate (Shawler et al., 2021a) and/or erosion by earlier ephemeral tidal inlets in this location (Hanley and McBride, 2011). This area remains a likely location for a future breach.
Today, the southern ∼4 km of Cedar Island is offset farther landward from the northern end of the island by nearly 500 m (Figures 2B, 3). This condition likely reflects, in part, the presence of an extensive and largely contiguous marsh platform in the backbarrier directly behind the northern 8 km of the island, which widens the island system, thereby limiting inlet formation. Additionally, migration atop this marsh has provided this portion of the island with a natural perch, allowing sediment delivered to the beach and dunes to accumulate vertically, slowing its landward migration (Walters et al., 2014; Deaton et al., 2017). In contrast, the southern end of the island contains only fringing backbarrier saltmarsh, washover fans, and shallow subaqueous bars associated with relict flood-tidal deltas deposited by former ephemeral inlets (Figure 2B). During the last decade, widening of Wachapreague Inlet has resulted in additional erosion of the southern 25 ha of the island (Figure 3). Combined, these processes have led to rapid thinning, degradation, and landward migration of the island’s southern 4 km. Additionally, the widening of Wachapreague Inlet has led to increased backbarrier wave energy from greater exposure to open-ocean conditions. This process has accelerated marsh-edge erosion and realignment and partial shoaling of adjacent navigation channels.
Methods: Field Data Collection and Analysis
A combination of morphologic, stratigraphic, sedimentologic, and ecologic field data collection (Figure 4) allowed for detailed mapping of the marsh construction and restoration project site, quantification of ecologic and sedimentologic success targets, and identification of potential borrow sites. These data were then integrated and used to inform details of an engineering design plan and quantification of sediment needs and potential sources.
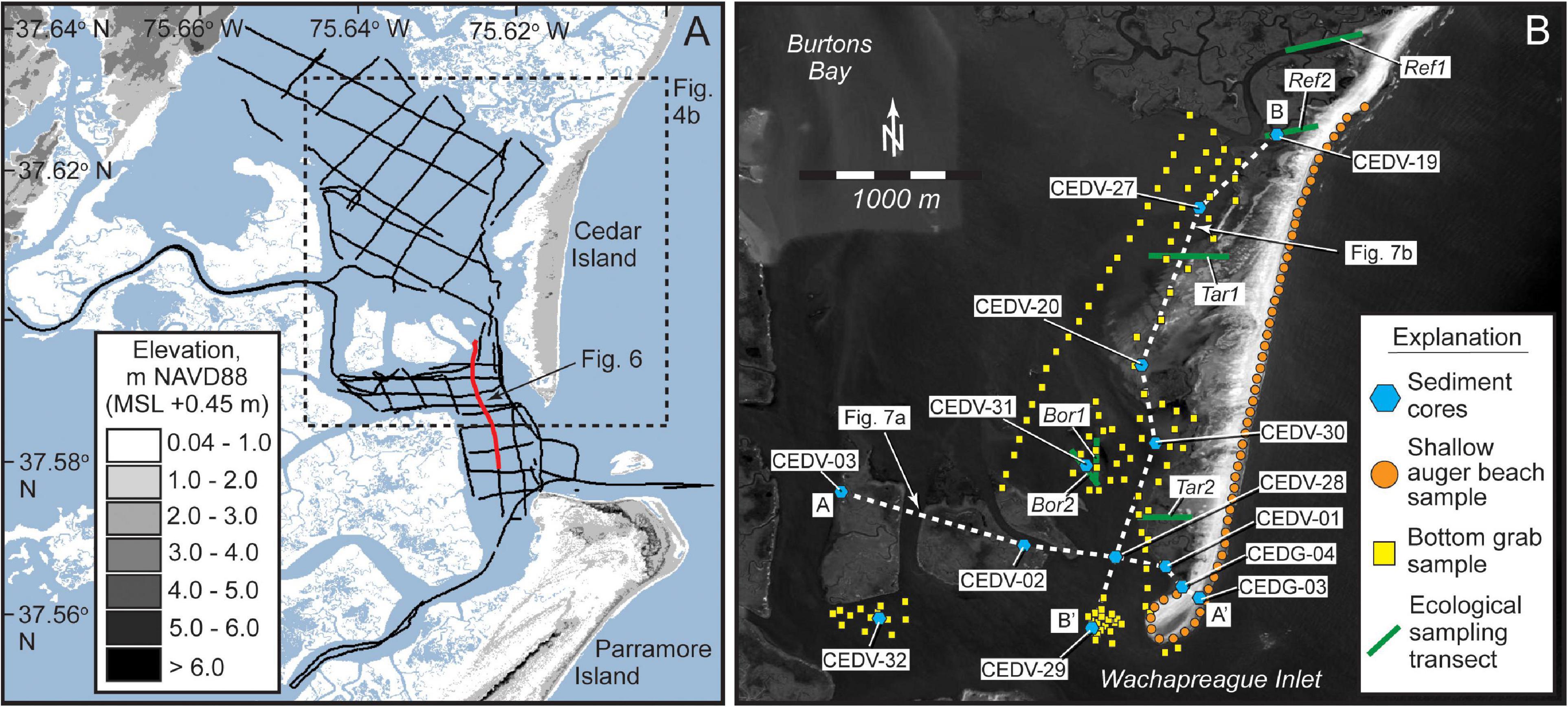
Figure 4. Map of southern Cedar Island and vicinity showing locations of field mapping and data collection. (A) Locations of ∼30 km of shallow-seismic profiles (black lines) overlain on digital elevation model (topographic and shallow bathymetric lidar; OCM (National Oceanic and Atmospheric Administration Office for Coastal Management) Partners, 2020c). (B) Sedimentologic and ecologic data collection. The former consists of 121 bottom grab samples, 57 shallow-auger beach sediment samples, 11 vibracores (labeled “CEDV-xx”), and two direct-push Geoprobe cores (labeled “CEDG-xx”). Ecological data were collected along six transects, with two each in reference marshes (labeled “Ref1” and “Ref2”), prospective project area marshes (labeled “Tar1” and “Tar2”), and a representative borrow site (labeled “Bor1” and “Bor2”). Background image is December 2018 orthophoto from the National Agriculture Imagery Program, administered by the US Department of Agriculture’s Farm Service Agency. Note difference in morphology of southern-most Cedar Island between images (approximately 2 years).
In order to produce a baseline map for use in design planning, we developed a seamless topographic-bathymetric (topo-bathy) map from existing and new field data. Existing topo-bathy data were compiled from a 2010 Eastern Shore digital elevation model (DEM) [OCM (National Oceanic and Atmospheric Administration Office for Coastal Management) Partners, 2020b], a 2016 US Army Corps of Engineers post-Matthew LiDAR survey [OCM (National Oceanic and Atmospheric Administration Office for Coastal Management) Partners, 2020a], a 2016 US Geological Survey Coastal National Elevation Database Topobathymetric Digital Elevation Model (CoNED TBDEM) [OCM (National Oceanic and Atmospheric Administration Office for Coastal Management) Partners, 2020c], and National Oceanic and Atmospheric Administration tidal datum data from at Wachapreague, VA (Station 8631044). New subaerial topographic and shallow bathymetric data were collected along southern Cedar Island and its backbarrier (Figure 5A) through use of high-resolution aerial imagery obtained in November of 2019 using unmanned aerial vehicles (drones). Imagery was collected with a SenseFly eBee drone with a global-positioning system with real-time kinematics (RTK-GPS), which yields centimeter-resolution (error: ∼3 cm horizontal, ∼5 cm vertical) GPS tags on each image. Survey missions were flown at 100 m above mean sea level, with 75% latitudinal and longitudinal overlap, yielding a ground resolution of 2.5 cm/pixel. Following Roze et al. (2014), we estimate a vertical uncertainty for individual DEM pixels of 1–3 times the ground sampling distance (2.5–7.5 cm in this case). Images were collected with ground-control points deployed on the beach as well, in case of RTK-GPS correction loss during flight. Images were analyzed using Agisoft Metashape photogrammetry software to produce orthomosaics (Figure 5B), DEMs (Figure 5C), three-dimensional models, and dense point clouds. Topographic and shallow bathymetric data were merged and used to: (1) develop seamless, updated topographic-bathymetric contours; (2) define and delineate existing high and low marsh areas as well as targeted post-project high and low marsh areas; and (3) compute fill height and final bottom elevations at each coordinate in the targeted project site.
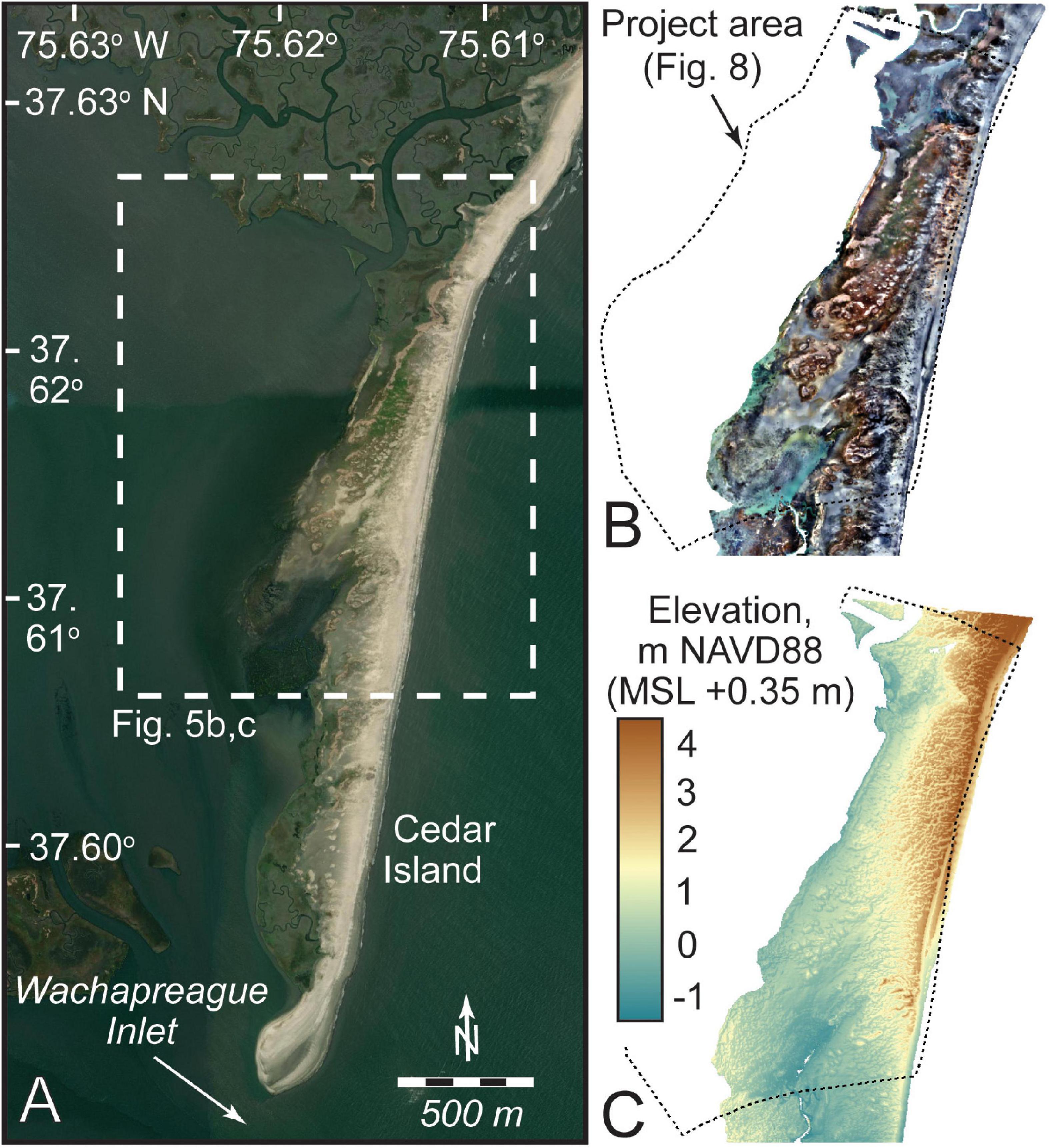
Figure 5. Results of drone-based topographic-bathymetric mapping of the southern Cedar Island project area. (A) Satellite image of southern Cedar Island (December 2018; from National Agriculture Imagery Program, administered by the US Department of Agriculture’s Farm Service Agency). (B) Orthoimagery data collected and mosaicked through structure-from-motion technology, converted into (C) a digital elevation model. Data collected November 2019 and processed by K. McPherran, University of Delaware.
Surface morphologic mapping data were supplemented with >30 km of shallow seismic data (Figure 4A), collected using an Applied Acoustics AA300 boomer seismic system operated at 150–300 J, with a CSP300 seismic energy source and 4.5 m long Applied Acoustics hydrophone streamer with eight elements. A Trimble DSM 132 differential GPS marine positioning receiver and antenna enabled merging real-time digital geographic positions to digital SEG-Y seismic data. This system provided submeter horizontal positional accuracy and up to 60 m of penetration based on an assumed sound velocity of 1,500 m/s. This depth penetration allowed for medium-resolution (1–2 m) imaging of major lithological units, and determination of sediment thickness and potential borrow sediment deposits under the project area (Figure 6), and associated sediment volumes through multiple crossing lines. Chesapeake Technologies’ SonarWiz software version 7 was used for data acquisition, processing, and interpretation. Tide-corrected analysis of two-way-travel time of the seismic signal between the vessel and seafloor allowed for high-precision mapping of bathymetry along seismic tracklines.
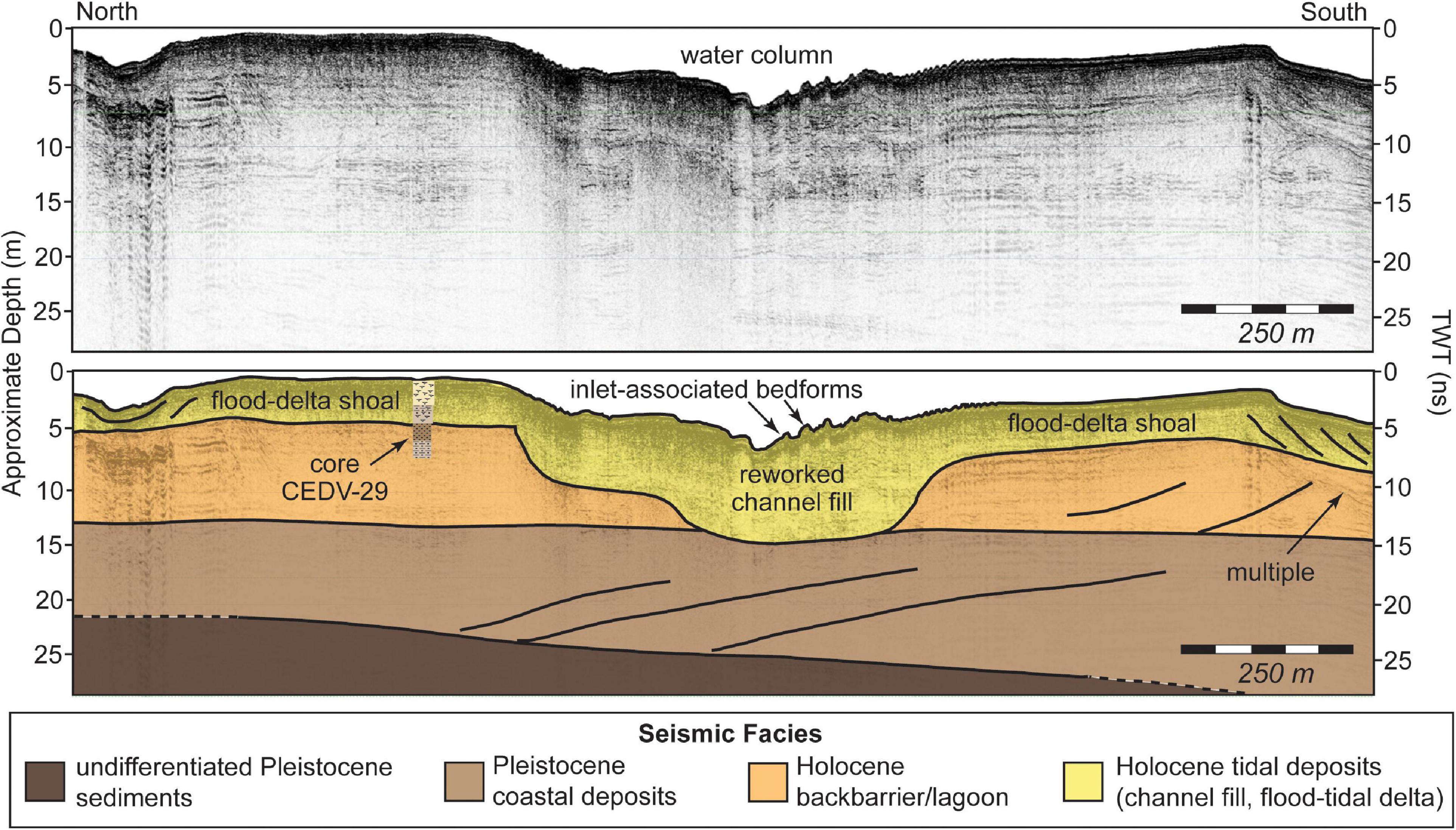
Figure 6. Example of processed (top) and interpreted (bottom) seismic data from Cedar Island backbarrier. Seismic profile line was collected along a north-south transect along the west side of a flood channel extending from Wachapreague Inlet (see location, Figure 4). Profile crosses over the location of sediment core CEDV-29 (core log shown on profile; for detail see Figures 7, 10), collected through target sand borrow site #1 (Figure 10).
Geophysical data were ground-truthed with 121 bottom grab samples collected with a ponar grab sampler, 57 shallow-auger (15 cm depth) beach sediment samples, 11 vibracores (4.5–5.0 m long, 7.6 cm in diameter), and two direct-push cores collected with a Geoprobe 66DT coring rig (17 and 24.5 m long; 5 cm in diameter) (Figure 4). Of these, data from seven cores were published previously by Shawler et al. (2019) and Shawler et al. (2021a); all other data are new to this study. Sediment cores were opened, photographed, described for texture (as compared to standards), mineralogy, and color (using a Munsell Soil Color Chart), interpreted for graphic core logs (Figure 7) and sampled for grain size. Aliquots of all surface, shallow auger, and core sediment samples were dried and analyzed for organic content (loss-on-ignition) through combustion at 650°C for ∼14 h and for calcium carbonate content through treatment with 10 M HCl. Grain-size analysis was conducted on wet sample aliquots using a Beckman Coulter LS 13320 (Beckman Coulter GmbH, Krefeld, Germany) laser diffraction particle size analyzer, equipped with an aqueous liquid module and a Polarization Intensity Differential Scatter unit. Grain-size distributions were calculated using the Fraunhofer optical model (see de Boer et al., 1987).
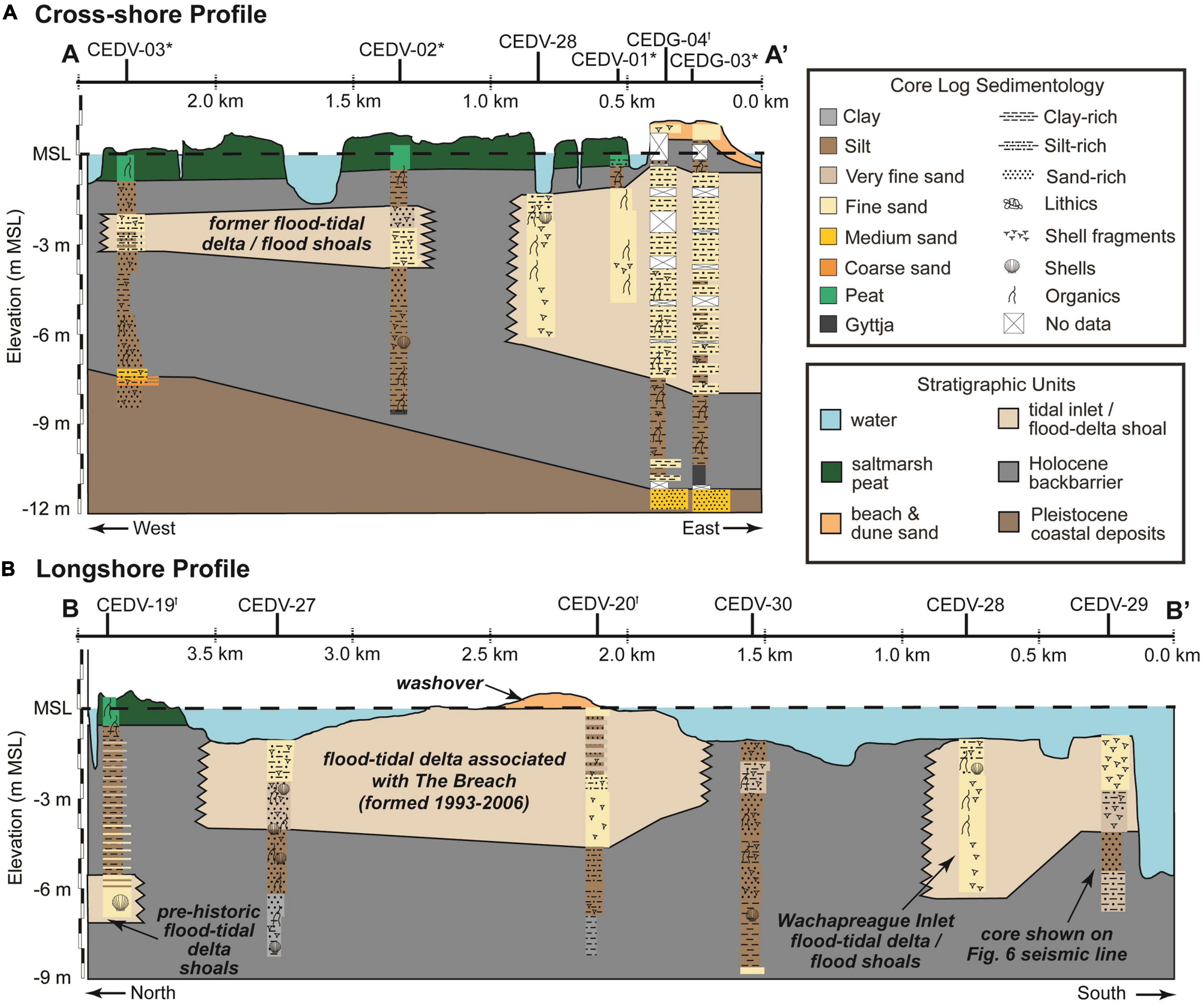
Figure 7. Stratigraphic sections developed from cores collected along (A) shore-normal and (B) shore-parallel transects west (landward) of southern Cedar Island (see location, Figure 4). Cores targeted the project site (CEDV-20, CEDV-27), flood-delta shoals (target borrow sites) (CEDV-28, CEDV-29), and reference island (CEDG-03, CEDG-04) and saltmarsh (e.g., CEDV-19, CEDV-02) locations. Core logs denoted with an asterisk (*) were originally published by Shawler et al. (2019); those denoted with a dagger (†) were originally published by Shawler et al. (2021a).
Finally, we collected a suite of marsh-site characteristic data in order to ensure created marsh within the target project area is compatible with local marsh types and characteristics. Specifically, we surveyed six transects (two each at construction, reference, and borrow site types; each 170–470 m long, Figure 4B) for ecological variables of plant species composition and percent cover and benthic macrofaunal densities. Transects ran from MLLW to MHW tidal datums. Access was restricted in areas of higher elevation close to dunes to protect nesting shorebirds. Transect surveys consisted of a 1×1 m quadrat every 10 m in which we quantified vegetation composition with percent foliar cover of all species within the plot, height of the dominant plant species, saltmarsh cordgrass (Spartina alterniflora), and benthic macrofaunal density in intertidal quadrats, and benthic macrofauna presence/absence in subtidal quadrats.
Results
Field, laboratory, and mapping data were analyzed and combined to develop five concept designs (Table 1), representing varying approaches to construction/restoration, and balancing between feasibility (based on estimated cost and needed fill material for construction) and expected benefits in terms of enhanced barrier-marsh system resiliency. Design concepts and the design-selection process – which considered factors such as stakeholder needs, cost, and permitting in addition to best-available science – are detailed in section “Science-Based Concept Design Development for Habitat Restoration and Coastal Resilience.” Here, we present results of our field data collection and analysis, and detail resulting preliminary plans for our chosen design (Concept 2) and proposed sediment borrow sites.
Preliminary Design Plans
Developed design plans are for a marsh construction project within a 160-ha area that is approximately 1,800 m long (north-south) and 630–1,150 m wide (east-west). Located ∼2 km north of Wachapreague Inlet, the project area would extend from the southern margin of the broad (3 km wide) marsh backing northern Cedar, across a shallow lagoon, and to south of the former flood-tidal delta of The Breach (Figure 8). This is an area characterized by low-profile (<2 m) coppice dunes fronting gently (0.15°–0.58°) westward-sloping supra- to inter-tidal marsh and tidal flats. Landward of the now-closed Breach, a sandy, ∼500 m wide platform – the flood-tidal delta of the former inlet – extends for nearly 1 km to the south-southwest from the island, at 0.5 to 2.0 m below mean high water (MHW) (Figure 5). This provides a shallow, relatively non-compactable surface upon which the new marsh can be constructed, minimizing needed fill, while also targeting a section of Cedar Island that is historically highly vulnerable to breaching.
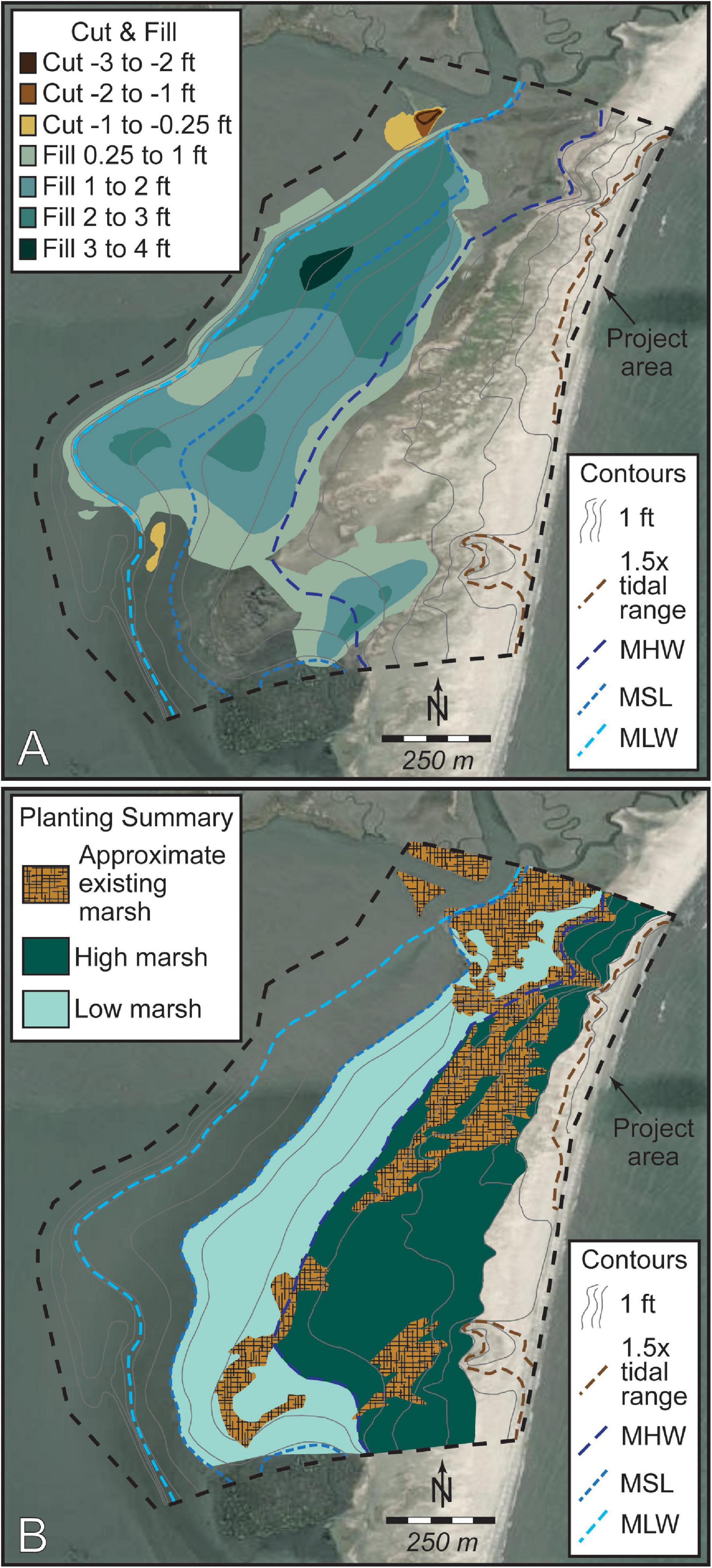
Figure 8. Project area map showing (A) cut and fill areas and proposed grading of existing marsh; and (B) low and high marsh planting plan. Engineering design drawings use Imperial units as required for permit application drawings. 1 ft = 0.3048 m. MHW, mean high water; MLW, mean low water; MSL, mean sea level.
Sediment textures within the project area correspond with distance from The Breach. Surficial sediments along and adjacent to the former flood-tidal delta are composed of fine to medium sand (median grain size: ∼0.21–0.29 mm) and become finer grained (muddy very fine to fine sand; median grain size: ∼0.09–0.14 mm) to the north and south. The finest sediments (largely sandy silt; median grain size: ∼20–60 μm) are found at the northern end of the project area, adjacent to the marsh, outside of the influence of the former tidal inlet.
A sediment core (CEDV-20) collected through the former location of the flood-tidal delta of The Breach revealed that inlet-associated deposits are nearly 3–4 m thick (Figure 7B). These are characterized by alternating very fine sand and sandy silt layers near the base; overtopped by 2 m of fine to medium, well-sorted and subrounded, mica- and heavy-mineral-rich sand with abundant shell hash and some rock fragments; and capped by interbedded sandy silt and silty very fine to fine sand. We interpret the latter to be barrier-proximal lagoon incorporating overwash and aeolian-transported beach and dune sand; flood-tidal delta and inlet channel fill deposits; and shallow sub-tidal to intertidal barrier-proximal lagoon facies, respectively. North of the former flood-tidal delta, CEDV-27 (Figure 7B) sampled ∼3.5 m of sandy clayey silt (shallow lagoon sediment) overtopping organic-rich, laminated silty-clay (deep, quiet-water lagoon deposits) and capped by mica-rich alternating layers of sandy silt and silty very fine to fine sand (likely wind-transported barrier beach and dune deposits). The stratigraphic successions captured in these sediment cores from within the project area contrast with that of core CEDV-19, collected through barrier-adjacent saltmarsh at the northern boundary of our project area (Figure 7B). Here, fine, mica- and heavy-mineral-rich sand is overlain by 5.5 m of interbedded clayey silt and clean, fine quartz sand, and capped by ∼1 m of saltmarsh peat. This facies succession is interpreted at the “natural” regressive-transgressive stratigraphy of Cedar Island (Shawler et al., 2019, 2021a). Consequently, our marsh construction project aims to replicate the gross characteristics of the upper sections of this vertical sequence.
This project will build 46 and 42 ha of high and low marsh, respectively, supplementing the existing 21 ha of saltmarsh that has built naturally upon flood-tidal delta and inlet-fill deposits associated with the former Breach. The proposed Grading Plan (sediment cut and fill) (Figure 8A) requires: (1) extending the fill area out to −5 ft NAVD88 (−1.98 m MHW) contour; (2) achieving a maximum fill thickness of 2 ft (0.61 m) at the −2 ft NAVD88 (−1.06 m MHW) contour; and (3) tapering the fill both shallower and deeper than −2 ft NAVD88 (−1.06 m MHW) so that the fill ties into existing contours. High marsh regions will be graded at a lagoon-ward (westward) slope of 1:750 and low marsh at a slope of 1:330 to 1:390. The low marsh will be stepped into the lagoon at a steeper grade of 1:50 to 1:70. These shallow slopes were selected to maximize the width of the final marsh. During and immediately following placement, the channel-ward margin of the sediment fill may require stabilization (e.g., coir logs or similar) and avian exclusion strategies (e.g., goose fencing) until planted vegetation is established.
The marshes at and around the project site display typical faunal and floral assemblages of Mid-Atlantic back-barrier marshes (Tyler and Zieman, 1999; Walsh, 1998), containing 50–90% vegetation cover of Spartina alterniflora and few other vascular plant species and common marsh invertebrates of fiddler crabs Uca spp., ribbed mussels Geukensia demissa, mud snails Tritia obsoleta, and periwinkle snails Littoraria irrorata. The northern reference and project area transects (Ref1 and Tar1; see locations in Figure 4B) are similar to the southern reference and project area transects (Ref2 and Tar2) (Figure 9). Northern transects (Ref1 and Tar1) had greater densities of fiddler crabs whereas southern transects (Ref2 and Tar2) had greater and more variable densities of mud snails (Figure 9). The highest density of ribbed mussels occurred at the northernmost transect (Ref1), whereas the highest density of periwinkle snails was at the southernmost transect (Tar2). Differences in the invertebrate community likely reflect substrate grain size differences that result from overwash events and location with respect to tidal creeks. Following these characteristics, design plans include planting of low marsh with Spartina alterniflora between mean sea level and mean high water. Designs also include planting of high marsh with Spartina patens between mean high water and 4.5 ft NAVD88 (0.9 m MHW), which is typical of the area (Tolley and Christian, 1999; Lonard et al., 2010) but was precluded from our field survey. Planted areas will be defined by the elevation of graded sediment, after the sediment has had sufficient time to dewater and settle, and the planted area will greatly exceed the area of the marsh disturbed by grading. Plants will be sourced as plugs and spaced ∼45 cm″ apart, planted using a power auger to drill holes, with manual plug placement. Plantings will be monitored during each growing season for a minimum of 3 years (and likely longer) and replanted as necessary to satisfy typical permit conditions, though scientific monitoring is expected to continue in perpetuity as part of on-going monitoring projects on the island, in part as a macrocosm test of marsh-barrier couplings.
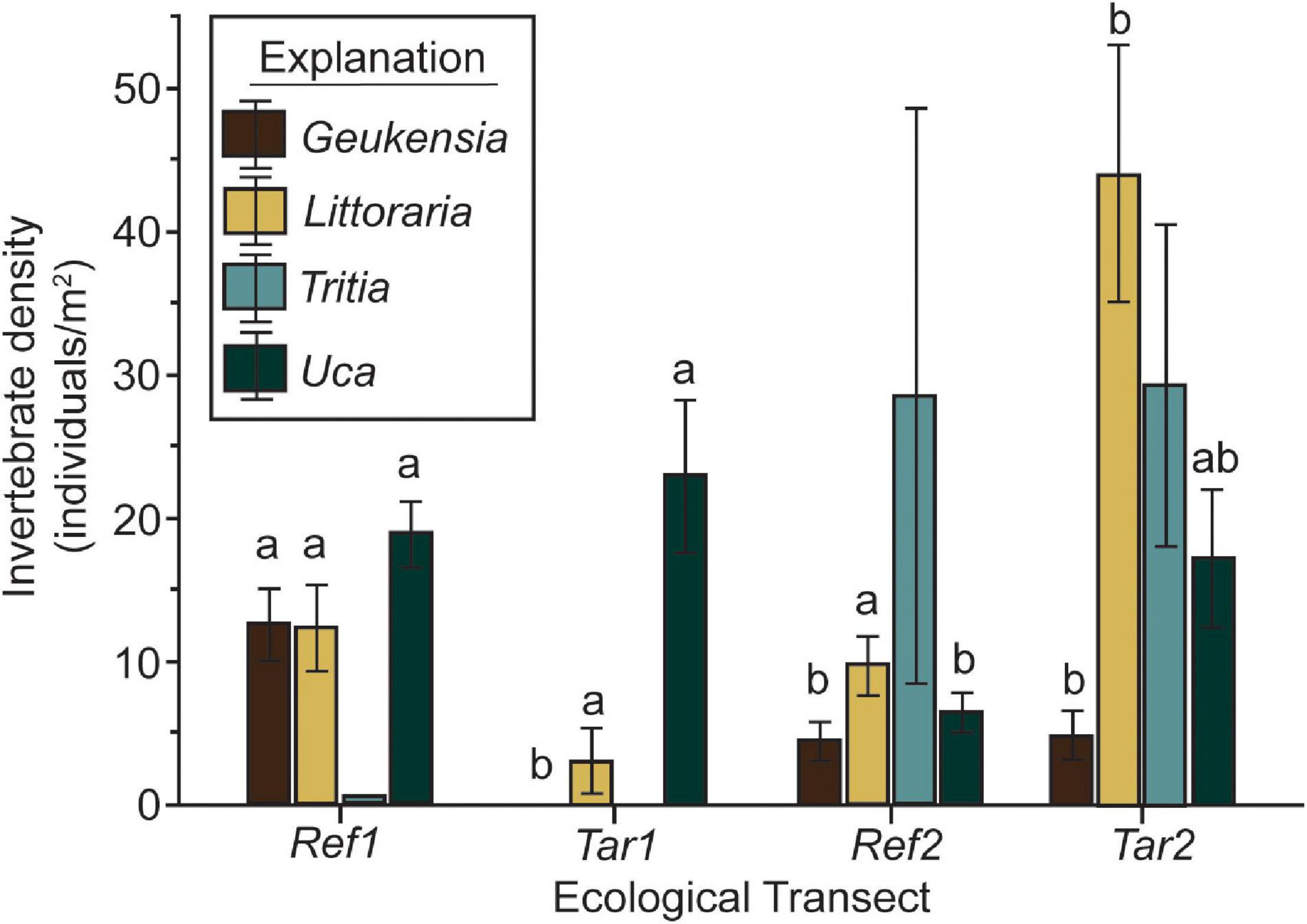
Figure 9. Results of invertebrate surveys from each reference (Ref1, Ref2) and project (Tar1, Tar2) marsh and tidal flat ecologic transects (see location: Figure 4B). Letters above bars denote significantly different groups in Tukey’s post hoc tests.
Proposed Borrow Sites
The project scope requires a net 285,603 m3 (5,119 m3 cut; 290,722 m3 fill) of source material as fill to elevate and grade backbarrier reaches and establish new high and low marsh along southern Cedar Island. In general, marshes range from fine- (clay) to coarse- (sand) grained sediments due to the biological trapping and tidal/storm processes. The first fundamental step toward restoration and new marsh creation is to (re-)establish saltmarsh structure and function, which includes stability of marsh sediments. To accomplish this, the grain size of the fill material is the dominant characteristic considered when designing a saltmarsh system. This must be consistent with the existing sediment currently found in the backbarrier marshes of southern Cedar Island. Therefore, we target predominantly silty sand – matching those proximal backbarrier sediments unaffiliated with the former Breach flood-tidal delta (Figure 7B) – for use as fill material to provide stability and biological function necessary for restoration. Candidates for this source material include: (1) shoals associated with the Wachapreague Inlet flood-tidal delta; and (2) dredge spoil from nearby backbarrier navigation channels.
Located at the southern terminus of Cedar Island, Wachapreague Inlet is the primary conduit for exchanging ∼55 × 106 m3 of water between the Atlantic Ocean and backbarrier bays during each tidal cycle (Fenster et al., 2011). The inlet is characterized by a single, stable, deep (∼20 m) channel, anchored in a Pleistocene stream valley (Morton and Donaldson, 1973). To the east (seaward) of this channel is a crescent-shaped ebb-tidal delta that is one of the largest along the Delmarva Peninsula; landward of the channel is a disparate, atypically shaped flood-tidal delta (Richardson et al., 2015). Sand bodies associated with this latter feature extend more than 1.5 km landward of the main ebb channel of Wachapreague Inlet. Seismic data, ground-truthed with sediment cores, reveal that flood-tidal delta shoals consist of short, discontinuous, and chaotic reflectors indicative of reworked material and overlie uniform-amplitude, continuous reflectors characteristic of finer-grained muds deposited in a lower-energy environment. Multiple high-amplitude reflectors are indicative of major erosion surfaces at the lower boundaries of these flood-tidal delta sands. Sand bodies are commonly found along the margins of major inlet-proximal tidal channels (Figure 2B) and are 2–3 m thick, on average (Figure 10). These sand bodies are depauperate and predominantly occupied by Agarophyton vermiculophyllum (previously Gracilaria vermiculophylla)-Diopatra cuprea polychaete associations (Thomsen et al., 2009). Besterman et al. (2020) have studied the value of this association in terms of biological resources for shorebirds, a major conservation concern for the region. The association is common in the Virginia coastal lagoons and provides prey resources to generalist shorebirds, but lower value resource quality for specialist foragers (Besterman et al., 2020). These data and analyses allowed us to identify three flood-tidal delta bodies located nearby to our project site that may be used as potential borrow sites (at locations of cores CEDV-29, −31, and −32; Figure 10). Together these total ∼790,000 m3 of sediment. Samples and cores reveal that these deposits are composed of 85–98% (predominantly fine) sand, with median grain sizes ranging from 0.1 to 0.25 mm (Figure 10), matching sand found within washover and former flood-tidal delta deposits at the project site (Figure 7).
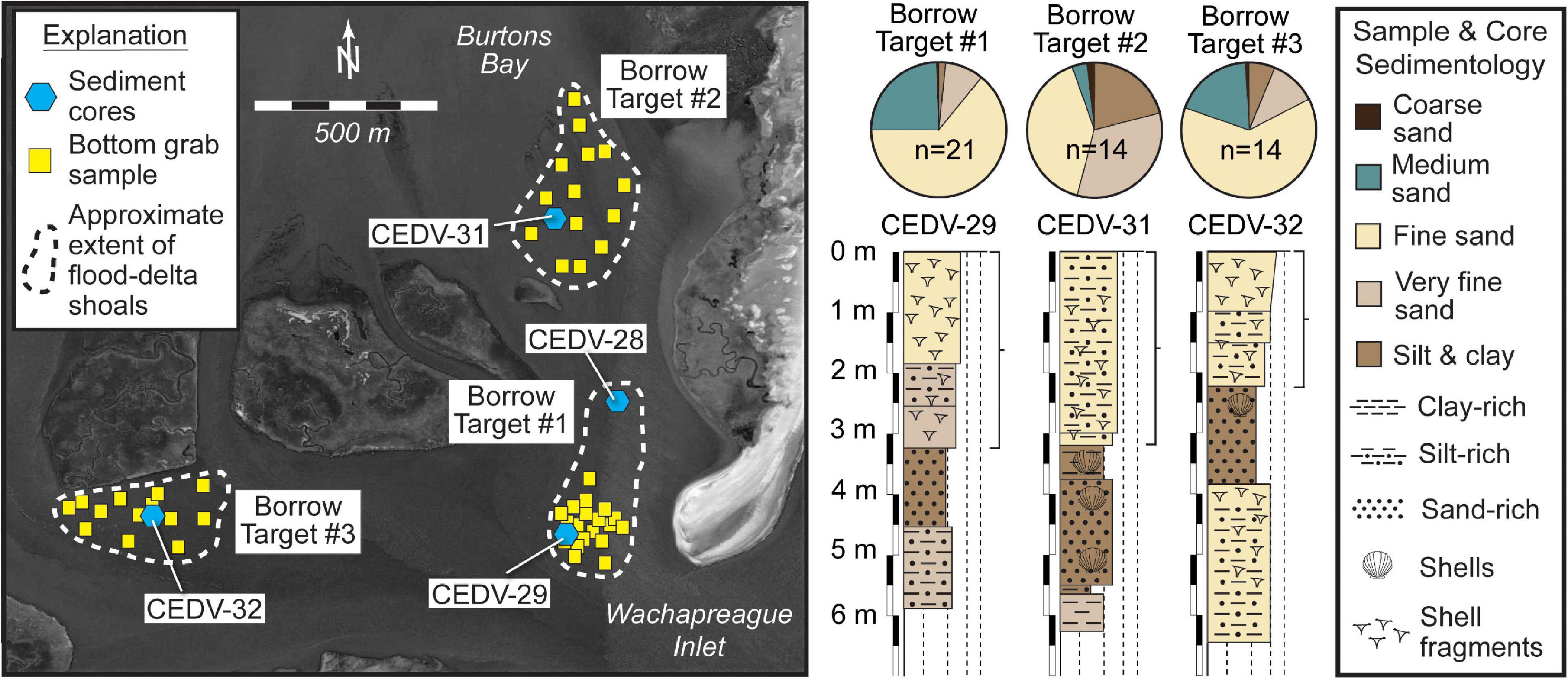
Figure 10. Approximate extents and sedimentology of discrete flood-tidal delta shoals located along the margins of major inlet-proximal tidal channels, and identified as potential sand reservoir borrow sites for marsh construction project. Pie charts show average grain-size distributions of all samples collected within a given target borrow site (total number given as n value within chart). Brackets to the right of core logs indicate the thickness sediments appropriate for borrow for the marsh construction project.
Dredge spoil from lagoonal navigation channels may provide additional sources of sediment to the project. In particular, the maintenance of channels leading from the mainland Wachapreague Harbor, which is home to a US Coast Guard station, tourism and aquatic-resource industries, and a scientific field, to Wachapreague Inlet and the Atlantic Ocean, provide likely sources. Wachapreague Harbor and Inlet are connected by the federal Bradford Bay and Finney Creek channels, as well as the non-federal Wachapreague Channel (Figure 2B). Dredging of the federal channels is conducted by the US Army Corps of Engineers approximately once every 7 years under the auspices of the US Water Resources Development Act of 1992, Continuing Authorities Program Section 204 (Beneficial Uses of Dredged Material). Dredging of the Bradford Bay and Finney Creek channels in summer 2020 yielded 94,014 m3 of sediment; no textural data are currently available [USACE (US Army Corps of Engineers), 2021]. One possible impediment to using as a source for our project sediments dredged in the future from these channels – even if of suitable texture for use as marsh construction fill – is that transporting dredge spoils a minimum of 4.5 km from the dredge site to the marsh re-creation project area may require booster pumps and therefore would be a higher-cost option. Further, use of the federal spoil is constricted by the timeline of federal dredging.
Hydrographic surveys are planned for summer 2021 for the 6.5-km long, non-federal Wachapreague Channel to determine dredge volume required to restore the channel to a width of at least 18.3 m and depth of 1.83 m. This channel is identified by the Eastern Shore of Virginia Regional Navigable Waterways Committee as a top-five dredging priority for Accomack County, Virginia. Here, dredging is hindered in part by the lack of dredge material disposal sites. Additionally, designing a beneficial placement site for dredged material poses a financial obstacle these rural localities. This scenario presents an opportunity for a collaborative effort for dredging and dredge disposal. However, this requires further analysis of channel sediment textures and hydrographic surveys of estimated sediment volumes; these activities are currently underway, led by the Accomack-Northampton Planning District Commission (A-NPDC).
Discussion
Science-Based Concept Design Development for Habitat Restoration and Coastal Resilience
Small- (Konisky et al., 2006), large- (Weinstein et al., 2001), and regional-scale (e.g., Chesapeake Bay Watershed Agreement, 2014) marsh-restoration programs have underscored the value of using tidal marshes as coastal resiliency promotors. Common approaches have included thin-layer deposition (e.g., Raposa et al., 2020), tidal-flow restoration (e.g., Warren et al., 2002; Konisky et al., 2006), wetland creation through beneficial use of dredge spoil (e.g., Cornwell et al., 2020), and others (c.f., Roman and Burdick, 2012; Herbert et al., 2015; Broome et al., 2019). However, rarely, if ever, have these efforts involved a synergistic, barrier-island systems approach that uses marsh/barrier-island bio-geomorphodynamics to attenuate accelerating barrier-system changes. Here, we detail the selection process used to develop a science-based marsh creation/restoration project concept designed to improve barrier-system resilience through marsh/barrier-island couplings. In particular, we highlight transferrable lessons associated with balancing design factors such as stakeholder needs, cost, and permitting, in addition to best-available science.
Southern Cedar Island, Virginia was chosen for this test design case because of its recent (60-year) history of breaching and overwashing, narrowing (erosion due to inlet widening), lowering, and landward migration (see section “Case Study: Cedar Island, Virginia, United States”). Marsh creation and expansion in our project area will fill accommodation behind the most vulnerable southern barrier reaches and consequently, mimic the northern, more resilient natural reaches. The low topography of Cedar Island topography maximizes the potential for sediment delivery to the constructed marsh through overwash and aeolian activity – processes known to maintain or increase marsh elevation in relation to the water level with accelerating sea-level rise (e.g., Rodriguez et al., 2013; Kirwan et al., 2016; Walters and Kirwan, 2016). We anticipate that the migration of Cedar Island onto the platform provided by the eastern (barrier-abutting) margin of our newly created marsh project will initially help to retain barrier sand in the subaerial barrier-system compartment, reduce the rate of island migration, and consequently, stabilize the island. Later, we expect the western edge of the marsh will form and grow at a pace commensurate with island rollover and eventually, sand will cover the entire construction project and begin to build on natural marsh. Further, widening the presently narrow island system with a newly constructed fringing marsh at the site of a former ephemeral tidal inlet – and subsequent island elevating through sand deposition on top of this platform – will reduce the likelihood of future storm breaching. Increased wave and tidal energy reaching the marshes behind Cedar Island during past phases of ephemeral inlet openings accelerated marsh-edge erosion along the eastern-facing marshes near the inlet (Erwin et al., 2004); indeed, edge erosion by wind waves is a primary cause of marsh losses throughout the Virginia Barrier Islands (Fagherazzi et al., 2013; McLoughlin et al., 2015; Sepanik and McBride, 2015; Deaton et al., 2017). Thus, mitigating future island breaching at this site will protect existing proximal marshes. Finally, this approach of coupling nature-based island stabilization with marsh creation is expected to have the added resiliency benefits of expanding marsh habitat and associated ecosystem services and ecological functions, such as fish, invertebrate, and avian habitat, and blue carbon storage (Erwin, 1996; Day et al., 2008; Mcleod et al., 2011; Kirwan and Mudd, 2012).
The approach presented here is founded on more than a decade of basic research into the interdependencies between barrier islands and adjacent backbarrier saltmarshes. Most importantly, it relies on numerical modeling experiments demonstrating that the presence of a backbarrier marsh may reduce the rate of island migration, reduce the likelihood of breaching, and help to stabilize the island naturally (Walters et al., 2014; Brenner et al., 2015; Lorenzo-Trueba and Mariotti, 2017; Nienhuis et al., 2021). However, no large-scale test of these couplings has been conducted to date; construction of a project that could be monitored over years to decades to validate existing models is a motivating objective of our design approach. Yet, any such real-world resiliency design project must also balance considerations beyond those which are informed only by science. This was reflected in our five design concepts (Table 1), which varied in scope and impact (both at the project and potential borrow sites) and reflected different balances among science, engineering, stakeholder needs, anticipated budget and permitting constraints, and maximizing benefits of habitat provision and shoreline protection.
For example, the Concept 1 design incorporated one-and-a-half times the marsh area (129 ha) as our chosen design (Concept 2), and thus would have provided a marsh platform for future migration of the entire southern ∼4 km of Cedar Island. Implementation of this design concept would seek to prevent future inlet formation at the site of The Breach and present the most comprehensive macro-scale test of modeled marsh-island couplings. In contrast, Concept 5 (i.e., Concept 1 backbarrier marsh construction combined with dune construction and beach nourishment) provided the most ambitious design, because it optimized island stability potential with a comprehensive marsh-, beach-, and dune-restoration effort. A similar project developed by Stantec in 2006 along Prime Hook National Wildlife Refuge (Delaware) provides an example of success for this design concept (Tabar, 2018). However, coupling marsh construction with additional soft island-stabilization techniques negates our ability to quantify the efficacy of constructed/restored backbarrier marshes specifically on slowing migration of Cedar Island, and to transfer findings from long-term monitoring efforts to other projects. This approach also requires both a backbarrier fill volume for marsh creation equivalent to that of Concept 2, and additional sediment for creating and nourishing 4 km of dune and beach. These requirements could be difficult to satisfy in sand-starved regions like the Virginia Barrier Islands and pose budgetary constraints.
Our final three conceptual designs reflected possible limitations associated with fill needs, cost, and permitting. Each of these incorporated components of the Concept 1 design: Concept 2 (our chosen design) emphasized the dual role of the created marsh in providing a platform for island migration and minimizing the prospect of future inlet breaching, but left the southern 2 km of Cedar Island vulnerable to continued erosion. Concept 3, requiring an order of magnitude less fill volume (∼23,000 m3) to create a marsh area (27 ha) approximately one-third that of Concept 2, benefitted from proximity to Borrow Target #1 (Figure 10), but would have created a marsh only half as wide as the island in that area; this narrow marsh would leave less of a platform for island migration as some of the other design concepts. To address this, Concept 4 (requiring 7× as much fill sediment as Concept 3) proposed reorienting a tidal channel located between southern Cedar and Borrow Target #1. This approach would allow for construction of a marsh more than twice as wide as that designed for Concept 3, but would have to be built across an existing channel and much of Borrow Target #1. Unlike Concept 2, which would be largely built onto flood-tidal delta sands, the remaining Concept 4 marsh (and the complete Concept 3 marsh) would be situated on compactable mud (Figure 7), which may dewater and cause more rapid subsidence. Concept 4 designs also extended marsh onto the Borrow Target #1 flood shoal, removing this shoal as a potential sand source. Finally, historical shoreline-change analyses showed that, while the marsh areas of Concepts 3 and 4 would likely receive the largest volumes of sediment via the adjacent Wachapreague Inlet (Castagno et al., 2018), further inlet widening (as is expected in a regime of accelerated sea-level rise and/or increased storminess; Fenster et al., 2016) would threaten any created marsh along the southernmost 1 km of Cedar Island.
Beyond these scientific and engineering factors, stakeholder needs, permitting considerations, and financial limitations of this rural locale all contributed to design decisions. For example, constraints associated with funding and permitting rank among the most acute hurdles to coastal restoration and resiliency projects, especially in the face of regulatory inflexibility associated with permitting requirements of and processes within federal, state, and local agencies (Ulibarri et al., 2020). Our approach engaged both local stakeholders and regional, state, and federal regulators at various stages of the design processes. We began by discussing our intent and project concept at the start of the design process with key stakeholders and regulators and followed those discussions by project mid-point with engineering concept design updates. After completing the field work, we presented a comparative analysis of our five design concepts to stakeholders at a specially called meeting at a time and location convenient to stakeholders. Based on stakeholder feedback from this meeting, we discounted the beach and dune restoration in Concept 5, in part, because some stakeholders (e.g., The Nature Conservancy) purposefully manage the southern Virginia Barrier Island beaches and dunes to retain a natural, dynamic state.
Based on these considerations, our team concluded, in consultation with local and regional stakeholders and regulators, that Concept 2 would provide the best balance between scope (cost, fill material required, completion timeliness, mitigation effectiveness, likelihood of permitting), and long-term resilience. Numerical hydrodynamic/sediment-transport modeling of Wachapreague Inlet and vicinity – currently underway as part of final project design and permitting – will quantify changes in circulation and morphological characteristics between existing and proposed conditions and help identify potential design modifications based on the hydrodynamic changes. Similar to many backbarrier bays and lagoons, the target project region is broad and shallow, limiting the required fill volume despite the large project area. Additionally, the aerial extent of the construction/restoration has been designed to account for both public shellfish grounds and private leases, thus avoiding a substantial regulatory hurdle. Nonetheless, estimated projected costs approach approximately US$10 million, presenting a financial hurdle to implementation. Finally, beneficial use of dredge spoil from backbarrier navigation channels provides one of the most likely sediment sources for the 285,000 m3 of fill material required for the Concept 2 project because of existing dredging needs and cost effectiveness. However, use of this material requires aligning project construction with the timing, funding, and priorities for regional (state-supported) or federal dredging.
Lessons on the Role of Stakeholder Engagement in Marsh Resilience Design
Residents of Virginia’s Eastern Shore have been visiting, inhabiting, and using the adjacent barrier islands for farming, hunting, fishing, and ranching since the mid-1600s, following centuries of use and occasional habitation by the native Accomac and Occohannock peoples (Barnes and Truitt, 1997). Like many coastal communities, they share a wide-spread recognition of the rapid changes occurring along this dynamic coast, and associated threats to the local environment, regional economy, and mainland physical infrastructure originating from habitat loss and the impacts of sea-level rise and increased storminess (Chen et al., 2020). The underrepresented, economically depressed, sparsely populated rural communities of Virginia’s Eastern Shore find themselves at a disadvantage in terms of competing for state and federal funding aimed at coastal resiliency efforts as compared to more populous localities (A-NPDC, 2015). The complex ownership of the Virginia Barrier Islands, and adjacent lagoons and marshes, by a network of public and private entities further complicates efforts at system-wide resiliency management level (A-NPDC, 2015). Additionally, the authors have observed, for more than a decade, a perception of “savior science” that exists among local residents. This apparently emanates from an impression that science has overpromised on efforts to improve the resilience of the natural/physical system in which they live and work. According to local residents’ comments, this perceived failure has its origin in the inability of experts to take into consideration local knowledge and desires or, at least, on the implications of those improvements for the livelihoods of residents. This perception has resulted in skepticism of science-based solutions among subsets of the local community, particularly those proposed by scientists from outside the community.
In such cases, early partnership in the conceptualization, design, and funding of a project of this nature is critical to identifying the priorities of the local community and achieving mutually desired outcomes with community support (Adamowicz and O’Brien, 2012; Portnoy, 2012). Here, that process included conducting informational, feedback-gathering, and planning sessions at key times in project conception and development; and communicating with the public through in-person engagement and the media, aiming not to “oversell” the project or its intended benefits for the local community. Future efforts require continued two-way education focused on the value of science-based decision-making that accounts for natural-system dynamics in concert with the interests of all involved to achieve resilience of a coupled natural and human system.
Summary and Recommendations
This case study chronicles the data and process used to develop an interdisciplinary, science-based engineering design for mitigating barrier-island deterioration and enhancing ecosystem functions and coastal resiliency through backbarrier saltmarsh construction. The design is founded on a conceptual and evidence-based scientific understanding of interdependent barrier-marsh couplings and relies upon ecologic, sedimentologic, stratigraphic, and morphologic field and lab data from the project site, adjacent reference saltmarshes, and prospective sediment borrow sites. If constructed, the resulting ∼100 ha of existing and constructed marsh would fill backbarrier accommodation at the site of a former ephemeral inlet on Cedar Island, Virginia. This effort would make southern Cedar Island more resilient to future breaching and by providing a platform upon which the island could migrate and aggrade. This approach to improved barrier-system resiliency is temporary: migration of undeveloped barrier islands is a long-term process that is likely to accelerate with sea-level rise (Mariotti, 2021). However, in the short term (several decades), a project of this scope is likely to slow barrier migration and deterioration as compared with the “do nothing” alternative. This approach can provide a temporary buffer for mainland communities and allow greater time to increase resiliency to climate change and its coastal impacts.
If successful, this design approach could be applied in other barrier settings in which unfilled backbarrier accommodation has left the fronting island vulnerable to sand loss or breaching. Examples include Pea Island along North Carolina’s Outer Banks (Montoya et al., 2018); Tom’s Cove Isthmus along southern Assateague Island, Virginia (Shawler et al., 2021b); southern Long Beach Island, New Jersey (Rogers et al., 2015), multiple vulnerable sites along Fire Island, New York (Hapke et al., 2013); and elsewhere along the Virginia Barrier Islands themselves (e.g., southern Metompkin Island; Walters et al., 2014). A single federal agency or non-governmental organizations (i.e., National Park Service, US Fish and Wildlife Service, The Nature Conservancy) own and manage each of these barriers; management, design, and permitting considerations are likely to be simplified as compared with Cedar Island.
The engineering design phase has allowed us to realize several transferable lessons related to design, funding, permitting, and stakeholder engagement:
1) Final project design selection from a suite of coastal resiliency alternatives requires attentiveness to multiple factors beyond scientific understanding of complex physical and biological systems, including (potentially complex) socio-economic, cultural, and policy considerations.
2) Opportunities exist at the nexus of ecology, geology, and engineering within the dynamic coastal zone. However, successful implementation of cross-disciplinary projects requires clear, regular, and timely communication; setting aside of professional and disciplinary biases; and a willingness to trust the expertise of colleagues.
3) Timely and regular engagement of regulatory agencies can guide project development and aid in project permitting.
4) Complex ownership of lands within and adjacent to the project area requires early engagement with, and full buy-in from, diverse stakeholders, who may have competing interests.
5) Navigation channel maintenance and beneficial use of dredged material provide opportunities for securing fill material for large projects at low cost and minimal environmental impact. However, the interaction of multiple, sometimes conflicting, interests and the uncertain and irregular timing of dredging operations can hinder this strategy.
6) Regular, precise communication and early engagement with the general public can help set expectations of project outcomes and implications for local communities in terms of physical resilience, while also educating residents on the economic, environmental, and community benefits of ecological restoration.
7) Local knowledge from stakeholders and community members can inform development of coastal management projects on topics ranging from geography and bio-physical changes to historic sites and economic considerations. Thus, engagement with stakeholders needs to occur as a dialogue wherein the project team and stakeholders learn from each other, and local information informs the final design. Doing so also builds trust.
Data Availability Statement
The raw data supporting the conclusions of this article will be made available by the authors, without undue reservation.
Author Contributions
CH, KG, and MF collected and analyzed all field data. JT and TD completed engineering design plans. CH and EH led organization of all stakeholder and regulator outreach, with contributions from the full project team. CH led manuscript preparation with contributions from all co-authors. All authors contributed to project design and securing funding.
Funding
This research was provided by the National Fish and Wildlife Foundation Coastal Resilience Grant Program FY19.
Conflict of Interest
JT and TD are employed by Stantec.
The remaining authors declare that the research was conducted in the absence of any commercial or financial relationships that could be construed as a potential conflict of interest.
Publisher’s Note
All claims expressed in this article are solely those of the authors and do not necessarily represent those of their affiliated organizations, or those of the publisher, the editors and the reviewers. Any product that may be evaluated in this article, or claim that may be made by its manufacturer, is not guaranteed or endorsed by the publisher.
Acknowledgments
We thank The Nature Conservancy’s Virginia Coast Reserve, the Commonwealth of Virginia, and numerous private landowners on Cedar Island provided access, sampling, and coring permissions. Virginia Institute of Marine Science (VIMS) and William & Mary staff and students Jennifer Connell, Kayla Cahoon, Sean Fate, Mahinaokalani Robbins, P. G. Ross, and Justin Shawler assisted with field data collection and laboratory processing and Charles Gowan from Randolph-Macon College assisted with the seismic surveys. George Washington University staff and students Jessica MacGregor, Man Qi, and Justus Jobe assisted with ecologic data collection. Daniel Proctor of Stantec provided abundant administrative support. We thank two reviewers for recommendations that improved this manuscript. Collaborating stakeholders include Shannon Alexander (A-NPDC); Curt Smith (formerly of A-NPDC); John Joeckel (Eastern Shore Regional Navigable Waterways Committee); Jill Bieri, Alexandra Wilke, and Susan Bates (The Nature Conservancy); Ruth Boettcher (Virginia Department of Wildlife Resources); Cora Baird (Virginia Coast Reserve LTER); Richard Snyder (VIMS Eastern Shore Lab); Sean Fate (VIMS Eastern Shore Lab and Wachapreague Fire Department); and Lyle Varnell (VIMS Office of Research and Advisory Service). This paper is Contribution No. 4042 of the Virginia Institute of Marine Science,William & Mary.
References
Adamowicz, S. C., and O’Brien, K. M. (2012). “Drakes Island tidal restoration,” in Tidal Marsh Restoration: a Synthesis of Science and Management, eds C. T. Roman and D. M. Burdick (Washington DC: Island Press), 315–332.
A-NPDC (2015). Commercial and Recreational Use Assessment Report–Seaside of Virginia’s Eastern Shore. Accomac, VA: Accomack-Northampton Planning District Commission.
Barnes, B. M., and Truitt, B. R. (1997). “A short history of the Virginia Barrier Islands,” in Seashore Chronicles: Three Centuries of the Virginia Barrier Islands, eds B. M. Barnes and B. R. Truitt (Charlottesville, VA: University of Virginia Press), 6–15.
Baustian, J. J., and Mendelssohn, I. A. (2015). Hurricane-induced sedimentation improves marsh resilience and vegetation vigor under high rates of relative sea level rise. Wetlands 35, 795–802. doi: 10.1007/s13157-015-0670-2
Besterman, A. F., Karpanty, S. M., and Pace, M. L. (2020). Impact of exotic macroalga on shorebirds varies with foraging specialization and spatial scale. PLoS ONE 15:e0231337. doi: 10.1371/journal.pone.0231337
Boon, J. D., and Mitchell, M. (2015). Nonlinear change in sea level observed at North American tide stations. J. Coast. Res. 31, 1295–1305. doi: 10.2112/JCOASTRES-D-15-00041.1
Brenner, O. T., Moore, L. J., and Murray, A. B. (2015). The complex influences of back-barrier deposition, substrate slope and underlying stratigraphy in barrier island response to sea-level rise: insights from the Virginia Barrier Islands, Mid-Atlantic Bight, USA. Geomorphology 246, 334–350. doi: 10.1016/j.geomorph.2015.06.014
Broome, S. W., Craft, C. B., and Burchell, M. R. (2019). “Tidal marsh creation,” in Coastal Wetlands: an Integrated Ecosystem Approach, eds G. M. E. Perillo, E. Wolanski, D. R. Cahoon, and C. S. Hopkinson (Amsterdam: Elsevier), 789–816.
Cahoon, D. R., Reed, D., Day, J. W., Steyer, D., Boumans, R. M., Lynch, C. J., et al. (1995). The influence of Hurricane Andrew on sediment distribution in Louisiana coastal marshes. J. Coastal Res. SI 21, 280–294.
Cañizares, R., and Irish, J. L. (2008). Simulation of storm-induced barrier island morphodynamics and flooding. Coast. Eng. 55, 1089–1101. doi: 10.1016/j.coastaleng.2008.04.006
Castagno, K. A., Jiménez-Robles, A. M., Donnelly, J. P., Wiberg, P. L., Fenster, M. S., and Fagherazzi, S. (2018). Intense storms increase the stability of tidal bays. Geophys. Res. Lett. 45, 5491–5500. doi: 10.1029/2018GL078208
Chen, Z., Swallow, S. K., and Yue, I. T. (2020). Non-participation and heterogeneity in stated: a double hurdle latent class approach for climate change adaptation plans and ecosystem services. Environ. Res. Econ. 77, 35–67. doi: 10.1007/s10640-020-00434-z
Chesapeake Bay Watershed Agreement (2014). Chesapeake Watershed Agreement. (Amended, January 2020). Available online at: https://www.chesapeakebay.net/documents/FINAL_Ches_Bay_Watershed_Agreement.withsignatures-HIres.pdf (accessed February 8, 2021)
Cornwell, J. C., Owens, M. S., and Staver, L. W. (2020). Tidal marsh restoration at Poplar Island I: transformation of estuarine sediments into marsh soils. Wetlands 40, 1673–1686. doi: 10.1007/s13157-020-01294-5
Day, J. W., Christian, R. R., Boesch, D. M., Yanez-Arancibia, A., Morris, J., Twilley, R. R., et al. (2008). Consequences of climate change on the ecogeomorphology of coastal wetlands. Estuaries Coasts 31, 477–491. doi: 10.1007/s12237-008-9047-6
de Boer, G. B. J., de Weerd, C., Thoenes, D., and Goossens, H. W. (1987). Laser diffraction spectrometry: Fraunhofer diffraction versus Mie scattering. Part. Part. Syst. Charact. 4, 14–19. doi: 10.1002/ppsc.19870040104
Deaton, C. D., Hein, C. J., and Kirwan, M. L. (2017). Barrier-island migration dominates ecogeomorphic feedbacks and drives salt marsh loss along the Virginia Atlantic Coast, USA. Geology 45, 123–126. doi: 10.1130/G38459.1
Dolan, R., and Godfrey, P. (1973). Effects of hurricane ginger on the barrier islands of North Carolina. Geol. Soc. Am. Bull. 84, 1329–1334. doi: 10.1130/0016-7606197384<1329:EOHGOT>2.0.CO;2
Erwin, R. M. (1996). Dependence of waterbirds and shorebirds on shallow-water habitats in the mid-Atlantic coastal region: an ecological profile and management recommendations. Estuaries 19, 213–219. doi: 10.2307/1352226
Erwin, R. M., Sanders, G. M., and Prosser, D. J. (2004). Changes in lagoonal marsh morphology at selected northeastern Atlantic coast sites of significance to migratory waterbirds. Wetlands 24, 891–903.
Fagherazzi, S., Mariotti, G., Wiberg, P. L., and McGlathery, K. J. (2013). Marsh collapse does not require sea level rise. Oceanography 26, 70–77.
Fenster, M. S., and Dolan, R. (1994). Large-scale reversals in shoreline trends along the U.S. mid-Atlantic coast. Geology 22, 543–546. doi: 10.1130/0091-76131994022<0543:LSRIST<2.3.CO;2
Fenster, M. S., Dolan, R., and Smith, J. J. (2016). Grain-size distributions and coastal morphodynamics along the southern Maryland and Virginia barrier islands. Sedimentology 63, 809–823. doi: 10.1111/sed.12239
Fenster, M. S., Honeycutt, M. G., and Gowan, C. (2003). “Impact of storms on shoreline change along the mid-Atlantic coast,” in Proceedings of the Coastal Sediments 2003, eds R. A. Davis, A. Sallenger, and P. Howd (Clearwater Beach, FL: American Association of Civil Engineers), 14. doi: 10.1142/5315
Fenster, M. S., McBride, R. A., Trembanis, A., Richardson, T., and Nebel, S. H. (2011). “A field test of the theoretical evolution of a mixed-energy barrier coast to a regime of accelerated sea-level rise,” in Proceedings of the Coastal Sediments 2011, eds J. Rosati, P. Wang, and T. M. Roberts (Miami, FL: American Association of Civil Engineers), 216–229. doi: 10.1142/8190
FitzGerald, D. M., Fenster, M. S., Argow, B. A., and Buynevich, I. V. (2008). Coastal impacts due to sea-level rise. Annu. Rev. Earth Planet. Sci. 36, 601–647. doi: 10.1146/annurev.earth.35.031306.140139
FitzGerald, D. M., Hein, C. J., Hughes, Z., Kulp, M., Georgiou, I., and Miner, M. (2018). “Runaway barrier island transgression concept: global case studies,” in Barrier Dynamics and Response to Changing Climate, eds L. Moore and A. B. Murray (Cham: Springer), 3–56. doi: 10.1007/978-3-319-68086-6_1
Hanley, J. T., and McBride, R. A. (2011). “Repetitive breaching on Cedar Island, Virginia, USA: history, geomorphology, and deposits,” in Proceedings of the Coastal Sediments 2011, eds J. Rosai, P. Wang, and T. M. Roberts (Miami, FL: American Association of Civil Engineers), 149–162.
Hanley, J. T., McBride, R. A., and Tedder, E. (2015). Ephemeral tidal inlets along southern Cedar Island, Virginia: geomorphic and geologic framework,” in Holocene barrier-island geology and morphodynamics of the Maryland and Virginia open-ocean coasts: Fenwick, Assateague, Chincoteague, Wallops, Cedar, and Parramore Islands, eds R. A. McBride, M. S. Fenster, C. T. Seminack, T. M. Richardson, J. M. Sepanik, J. T. Hanley, et al., in Tripping from the Fall Line: field excursions for the GSA Annual Meeting, Baltimore, 2015, eds D. K. Brezinski, J. P. Halka, R. A. Ortt Jr. (Boulder, CO: Geological Society of America). Field Guide 40, 408–418. doi: 10.1130/2015.0040(10)
Hapke, C. J., Brenner, O., Hehre, R., and Reynolds, B. J. (2013). Coastal Change from Hurricane Sandy and the 2012-13 Winter Storm Season. New York, NY: US Department of the Interior.
Hayden, B. P., and Hayden, N. R. (2003). “Decadal and century-long changes in storminess at long-term ecological research sites,” in Climate Variability and Ecosystem Response at Long-Term Ecological Research Sites, eds D. Greenland, D. G. Goodin, and R. C. Smith (New York, NY: Oxford University Press), 262–285.
Herbert, E. R., Marton, J. M., and Craft, C. B. (2015). “Tidal wetland restoration,” in Wetland Soils: Genesis, Hydrology, Landscapes, and Classification, eds J. L. Richardson and M. J. Vepraskas (Boca Raton, FL: CRC Press), 447–468.
Himmelstoss, E. A., Kratzmann, M., Hapke, C. J., Thieler, E. R., and List, J. H. (2010). The National Assessment of Shoreline Change: a GIS Compilation of Vector Shorelines and Associated Shoreline Change Data for the New England and Mid-Atlantic Coasts: U.S. Geological Survey Open-File Report 2010-1119. Reston, VA: US Department of the Interior.
Howes, B. L., Weiskel, P. K., Goehringer, D. D., and Teal, J. M. (1996). “Interception of freshwater and nitrogen transport from uplands to coastal waters: the role of saltmarshes,” in Estuarine Shores: Evolution, Environments, and Human Alterations, eds K. F. Nordstrom and C. T. Roman (New York, NY: Wiley), 287–310.
Kirwan, M. L., and Mudd, S. M. (2012). Response of salt-marsh carbon accumulation to climate change. Nature 489, 550–553. doi: 10.1038/nature11440
Kirwan, M. L., Temmerman, S., Skeehan, E. E., Guntenspergen, G. R., and Fagherazzi, S. (2016). Overestimation of marsh vulnerability to sea level rise. Nat. Clim. Change 6, 253–260. doi: 10.1038/nclimate2909
Konisky, R. A., Burdick, D. M., Dionne, M., and Neckles, H. A. (2006). A regional assessment of salt marsh restoration and monitoring in the Gulf of Maine. Restor. Ecol. 14, 516–525. doi: 10.1111/j.1526-100X.2006.00163.x
Lauzon, R., Murray, A. B., Moore, L. J., Walters, D., Kirwan, M., and Fagherazzi, S. (2018). Effects of marsh edge erosion in coupled barrier island-marsh systems and geometric constraints on marsh evolution. J. Geophys. Res. Earth Surf. 123, 1218–1234. doi: 10.1029/2017JF004530
Leonardi, N., Carnacina, I., Donatelli, C., Ganju, N. K., Plater, A. J., Schuerch, M., et al. (2018). Dynamic interactions between coastal storms and salt marshes: a review. Geomorphology 301, 92–107. doi: 10.1016/j.geomorph.2017.11.001
Lonard, R. I., Judd, F. W., and Stalter, R. (2010). The biological flora of coastal dunes and wetlands: Spartina patens (W. Aiton) G.H. Muhlenberg. J. Coast. Res. 26, 935–946. doi: 10.2112/JCOASTRES-D-09-00154.1
Lorenzo-Trueba, J., and Mariotti, G. (2017). Chasing boundaries and cascade effects in a coupled barrier-marsh-lagoon system. Geomorphology 290, 153–163. doi: 10.1016/j.geomorph.2017.04.019
Mariotti, G. (2021). Self-organization of coastal barrier systems during the holocene. J. Geophys. Res. Earth Surf. 126:e2020JF005867. doi: 10.1029/2020JF005867
Mcleod, E., Chmura, G. L., Bouillon, S., Salm, R., Björk, M., Duarte, C. M., et al. (2011). A blueprint for blue carbon: toward an improved understanding of the role of vegetated coastal habitats in sequestering CO2. Front. Ecol. Environ. 9:552–560. doi: 10.1890/110004
McLoughlin, S. M., Wiberg, P. L., Safak, I., and McGlathery, K. J. (2015). Rates and forcing of marsh edge erosion in a shallow coastal bay. Estuaries Coasts 38, 620–638. doi: 10.1007/s12237-014-9841-2
Montoya, L. V., Sciaudone, E. J., Mitasova, H., and Overton, M. F. (2018). Observation and modeling of the evolution of an ephemeral storm-induced inlet: Pea Island Breach, North Carolina, USA. Cont. Shelf Res. 156, 55–69. doi: 10.1016/j.csr.2018.02.002
Morton, R. A. (2008). Historical changes in the Mississippi-Alabama barrier-island chain and the roles of extreme storms, sea level, and human activities. J. Coast. Res. 24, 1587–1600. doi: 10.2112/07-0953.1
Morton, R. A., and Donaldson, A. C. (1973). Sediment distribution and evolution of tidal deltas along a tide-dominated shoreline, Wachapreague, Virginia. Sediment. Geol. 10, 285–299. doi: 10.1016/0037-0738(73)90053-5
Moyer, K. (2007). An Assessment of an Ephemeral Breach Along Cedar Island, Virginia. Ph. D thesis. Fairfax, VA: George Mason University.
Najjar, R. G., Herrmann, M., Alexander, R., Boyer, E. W., Burdige, D. J., Butman, D., et al. (2018). Carbon budget of tidal wetlands, estuaries, and shelf waters of eastern North America. Glob. Biogeochem. Cycles 32, 389–416. doi: 10.1002/2017GB005790
Nebel, S. H., Trembanis, A. C., and Barber, D. C. (2013). Shoreline analysis and barrier island dynamics: decadal scale patterns from Cedar Island. Virginia. J. Coast. Res. 280, 332–341. doi: 10.2112/JCOASTRES-D-10-00144.1
Nienhuis, J. H., Heijkers, L. G., and Ruessink, G. (2021). Barrier breaching versus overwash deposition: predicting the morphologic impact of storms on coastal barriers. J. Geophys. Res. Earth Surf. 126:e2021JF006066. doi: 10.1029/2021JF006066
Nyman, J. A., Crozier, C. R., and DeLaune, R. D. (1995). Roles and patterns of hurricane sedimentation in an estuarine marsh landscape. Estuar. Coast. Shelf Sci. 40, 665–679. doi: 10.1006/ecss.1995.0045
OCM (National Oceanic and Atmospheric Administration Office for Coastal Management) Partners (2020b). 2016 USACE Post-Matthew Topobathy Lidar: Southeast Coast (VA, NC, SC, GA and FL). Available online at: https://www.fisheries.noaa.gov/inport/item/49409 (accessed January 15, 2020)
OCM (National Oceanic and Atmospheric Administration Office for Coastal Management) Partners (2020a). 2010 VA Information Technologies Agency (VITA)/VA Geographic Information Network (VGIN) Lidar: Eastern Shore, VA (Accomack and Northampton Counties). Available online at: https://www.fisheries.noaa.gov/inport/item/50124 (accessed January 15, 2020)
OCM (National Oceanic and Atmospheric Administration Office for Coastal Management) Partners (2020c). 2016 USGS CoNED Topobathymetric Model (1859–2015): Chesapeake Bay Region. Available online at: https://www.fisheries.noaa.gov/inport/item/55321 (accessed January 15, 2020)
Oreska, M. P. J., Truitt, B., Orth, R. J., and Luckenbach, M. W. (2017). The bay scallop (Argopecten irradians) industry collapse in Virginia and its implications for the successful management of scallop-seagrass habitats. Mar. Pol. 75, 116–124. doi: 10.1016/j.marpol.2016.10.021
Orth, R. J., Wilcox, D. J., Whiting, J. R., Kenne, A. K., and Smith, E. R. (2020). 2019 Distribution of Submerged Aquatic Vegetation in Chesapeake Bay and Coastal Bays. Available online at: https://www.vims.edu/research/units/programs/sav/reports/2019/index.php (accessed May 24, 2021)
Pennings, S. C., and Bertness, M. D. (2001). “Salt marsh communities,” in Marine Community Ecology, eds M. D. Bertness, S. D. Gaines, and M. E. Hay (Sunderland, MA: Sinauer), 289–316.
Portnoy, J. W. (2012). “Salt marsh restoration at cape Cod National Seashore, Massachusetts,” in Tidal Marsh Restoration: a Synthesis of Science and Management, eds C. T. Roman and D. M. Burdick (Washington DC: Island Press), 299–314.
Raposa, K., Wasson, K., Nelson, J., Fountain, M., West, J., Endris, C., et al. (2020). Guidance for Thin-Layer Sediment Placement as a Strategy to Enhance Tidal Marsh Resilience to Sea-Level Rise. Silver Spring, MD: collaboration with the National Estuarine Research Reserve System Science Collaborative.
Rejmanek, M., Sasser, C. E., and Peterson, G. W. (1988). Hurricane-induced sediment deposition in a Gulf coast marsh. Estuar. Coast. Shelf Sci. 27, 217–222. doi: 10.1016/0272-7714(88)90091-1
Richardson, T. M., McBride, R. A., Fenster, M. S., and Seminack, C. T. (2015). Morphodynamic changes at Wachapreague Inlet, Virginia: a tide-dominated inlet system,” in Holocene barrier-island geology and morphodynamics of the Maryland and Virginia open-ocean coasts: Fenwick, Assateague, Chincoteague, Wallops, Cedar, and Parramore Islands, eds R. A. McBride, M. S. Fenster, C. T. Seminack, T. M. Richardson, J. M. Sepanik, J. T. Hanley, J. A. Bundick, E. Tedder, in Tripping from the Fall Line: field Excursions for the GSA Annual Meeting, Baltimore, 2015, eds D. K. Brezinski, J. P. Halka, R. A. Ortt Jr. (Boulder, Colorado: Geological Society of America). Field Guide 40, 385–392.
Rodriguez, A. B., Fegley, S. R., Ridge, J. T., VanDusen, B. M., and Anderson, N. (2013). Contribution of aeolian sand to backbarrier marsh sedimentation. Estuar. Coast. Shelf Sci. 117, 248–259. doi: 10.1016/j.ecss.2012.12.001
Rogers, L. J., Moore, L. J., Goldstein, E. B., Hein, C. J., Lorenzo-Trueba, J., and Ashton, A. D. (2015). Anthropogenic controls on overwash deposition: evidence and consequences. J. Geophys. Res. Earth Surf. 120, 2609–2624. doi: 10.1002/2015JF003634
Roman, C. T., and Burdick, D. M. (2012). Tidal Marsh Restoration: a Synthesis of Science and Management. Washington DC: Island Press.
Roze, A., Zufferey, J. C., Beyeler, A., and McClellan, A. (2014). eBee RTK accuracy assessment. Lausanne: senseFly.
Sepanik, T. M., and McBride, R. A. (2015). “Salt-marsh loss in a barrier-island system: Parramore and Cedar islands, Virginia” in Holocene barrier-island geology and morphodynamics of the Maryland and Virginia open-ocean coasts: Fenwick, Assateague, Chincoteague, Wallops, Cedar, and Parramore Islands, eds R. A. McBride, M. S. Fenster, C. T. Seminack, T. M. Richardson, J. M. Sepanik, J. T. Hanley, J. A. Bundick, E. Tedder, in Tripping from the Fall Line: field excursions for the GSA Annual Meeting, Baltimore, 2015, eds D. K. Brezinski, J. P. Halka, R. A. Ortt Jr. (Boulder, Colorado: Geological Society of America). Field Guide 40, 392–401.
Shawler, J. L., Ciarletta, D. J., Connell, J. E., Boggs, B. Q., Lorenzo-Trueba, J., and Hein, C. J. (2021a). Relative influence of antecedent topography and sea-level rise on barrier-island migration. Sedimentology 68, 639–669. doi: 10.1111/sed.12798
Shawler, J. L., Ciarletta, D. J., Lorenzo-Trueba, J., and Hein, C. J. (2019). “Drowned foredune ridges as evidence of pre-historical barrier-island state changes between migration and progradation,” in Proceedings of the Coastal Sediments 2019, eds J. Rosati, P. Wang, and M. Vallee (St. Petersburg, FL: American Association of Civil Engineers), 158–171.
Shawler, J. L., Hein, C. J., Obara, C. A., Robbins, M. G., Hout, S., and Fenster, M. S. (2021b). The effect of coastal landform development on decadal- to millennial-scale longshore sediment fluxes: evidence from the Holocene evolution of the central mid-Atlantic coast, USA. Quat. Sci. Rev. 267:107096.
Shepard, C. C., Crain, C. M., and Beck, M. W. (2011). The protective role of coastal marshes: a systematic review and meta-analysis. PloS One 6:e27374. doi: 10.1371/journal.pone.0027374
Tabar, J. R. (2018). “Adaptation to a changing climate in the coastal zone–a case study of Prime Hook National Wildlife Refuge,” in Proceedings of the 36th Conference on Coastal Engineering, ed. P. Lynett (Baltimore, MD: International Conference on Coastal Engineering), 36. doi: 10.9753/icce.v36.risk.2
Thomsen, M. S., McGlathery, K. J., Schwarzschild, A., and Silliman, B. R. (2009). Distribution and ecological role of the non-native macroalga Gracilaria vermiculophylla in Virginia salt marshes. Biol. Invasions 11, 2303–2316. doi: 10.1007/s10530-008-9417-9
Tolley, P. M., and Christian, R. R. (1999). Effects of increased inundation and wrack deposition on a high salt marsh plant community. Estuaries 224, 944–954. doi: 10.2307/1353074
Tweel, A. W., and Turner, R. E. (2014). Contribution of tropical cyclones to the sediment budget for coastal wetlands in Louisiana. USA. Landsc. Ecol. 29, 1083–1094.
Tyler, A. C., and Zieman, J. C. (1999). Patterns of development in the creekbank region of a barrier island Spartina alterniflora marsh. Mar. Ecol. Prog. Ser. 180, 161–177. doi: 10.3354/meps180161
Ulibarri, N., Goodrich, K. A., Wagle, P., Brand, M., Matthew, R., Stein, E. D., et al. (2020). Barriers and opportunities for beneficial reuse of sediment to support coastal resilience. Ocean Coast. Manag. 195:105287. doi: 10.1016/j.ocecoaman.2020.105287
USACE (US Army Corps of Engineers) (2021). Status Update for 21 January 2021 on the Waterway on the Coast of Virginia Federal Navigation Project to the Shore Regional Navigable Waterways Committee, Corps of Engineers Norfolk District, unpublished report. Washington, DC: USACE.
Walsh, J. P. (1998). Low Marsh Succession Along an Over-Wash Salt Marsh Chronosequence. Ph. D thesis. Charlottesville, VA: University of Virginia.
Walters, D., Moore, L. J., Duran Vinent, O., Fagherazzi, S., and Mariotti, G. (2014). Interactions between barrier islands and backbarrier marshes affect island system response to sea level rise: insights from a coupled model. J. Geophys. Res. Earth Surf. 119, 2013–2031. doi: 10.1002/2014JF003091
Walters, D. C., and Kirwan, M. L. (2016). Optimal hurricane overwash thickness for maximizing marsh resilience to sea level rise. Ecol. Evol. 6, 2948–2956. doi: 10.1002/ece3.2024
Warren, R. S., Fell, P. E., Rozsa, R., Brawley, A. H., Orsted, A. C., Olson, E. T., et al. (2002). Salt marsh restoration in Connecticut: 20 years of science and management. Restor. Ecol. 10, 497–513. doi: 10.1046/j.1526-100X.2002.01031.x
Keywords: saltmarsh restoration, barrier islands, ecogeomorphology, coastal resilience, tidal inlet, overwash
Citation: Hein CJ, Fenster MS, Gedan KB, Tabar JR, Hein EA and DeMunda T (2021) Leveraging the Interdependencies Between Barrier Islands and Backbarrier Saltmarshes to Enhance Resilience to Sea-Level Rise. Front. Mar. Sci. 8:721904. doi: 10.3389/fmars.2021.721904
Received: 07 June 2021; Accepted: 04 August 2021;
Published: 07 September 2021.
Edited by:
Nicoletta Leonardi, University of Liverpool, United KingdomReviewed by:
Jorge Guillen, Consejo Superior de Investigaciones Científicas (CSIC), SpainChristian Schwarz, University of Delaware, United States
Copyright © 2021 Hein, Fenster, Gedan, Tabar, Hein and DeMunda. This is an open-access article distributed under the terms of the Creative Commons Attribution License (CC BY). The use, distribution or reproduction in other forums is permitted, provided the original author(s) and the copyright owner(s) are credited and that the original publication in this journal is cited, in accordance with accepted academic practice. No use, distribution or reproduction is permitted which does not comply with these terms.
*Correspondence: Christopher J. Hein, aGVpbkB2aW1zLmVkdQ==