- 1Research and Development Center for Efficient Utilization of Coastal Bioresources, Yantai Institute of Coastal Zone Research, Chinese Academy of Sciences, Yantai, China
- 2University of Chinese Academy of Sciences, Beijing, China
- 3College of Marine Science and Engineering, Qingdao Agricultural University, Qingdao, China
- 4Zhejiang Mariculture Research Institute, Wenzhou, China
Ark shells (Scapharca subcrenata) grown on the tidal flats are often exposed to high temperature stresses in summer. In order to better understand their adaption to extreme or natural high temperature, we first determined the 96-h upper lethal temperature of ark shell and then investigated their physiological and transcriptional responses to acute or chronic thermal stress at the 96-h upper median lethal temperature (32°C). A significantly higher cumulative mortality (52% in 96 h) was observed in the acute heating treatment (AHT) group than that (22% in 7 days) in the chronic heating treatment (CHT) group. The apoptosis and necrosis rates of hemocytes were increased significantly in a time-dependent manner under both thermal stress strategies. Activities of antioxidant enzymes [superoxide dismutase (SOD) and catalase (CAT)] increased dramatically in a short time followed by a quick decline and reached to a lower level within 12 h in the AHT group, but maintain relatively high levels over a long period in the CHT group. The contents of malondialdehyde (MDA) were increased significantly firstly and restored to the original later in both acute and chronic thermal stress. Moreover, expression of the genes related to heat shock proteins (HSPs; HSP90, HSP70, HSP20, and sHSP), apoptosis [TNF receptor-associated factor 6 (TRAF6), glucose regulated protein 78 kD (GRP78), and caspase-3 (Casp-3)] and antioxidant responses [glutathione S-transferase (GST) and multidrug resistance protein (MRP)] could be induced and up-regulated significantly by thermal stress, however, expression of regucalcin (RGN), metallothionein (MT), and peroxiredoxin (PRX) was down-regulated dramatically under the two heating treatments. These results suggested that anti-apoptotic system, antioxidant defense system and HSPs could play important roles in thermal tolerance of ark shells, and the heat-resistant ark shell strains could be selected continuously by properly chronic thermal stress.
Introduction
In recent decades, the seawater temperature keeps rising with the acceleration of global warming (Hoegh-Guldberg et al., 2007), and in the worst cases, seawater temperature could increase by 4.5°C by the end of this century (IPCC, 2013). As a consequent, many intertidal marine mollusks are living at temperatures close to or just below their upper thermal limits (Stillman, 2003; Tomanek and Zuzow, 2010; Clark et al., 2021; Ning et al., 2021). Unlike terrestrial animals, marine organisms inhabiting nearshore are usually unable to shelter from high temperature environment (Burrows et al., 2019). Therefore, investigation of their biological responses to thermal stresses may provide insights into underlying the adaptive mechanisms of these intertidal mollusk to elevated temperatures.
Habitat temperature is a prominent stressor that can affect the survival, growth, development, reproduction, metabolism, immunity and geographical distribution of marine organisms (Chen et al., 2007; Anestis et al., 2010; Jiang et al., 2016; Yang et al., 2017; Monaco and McQuaid, 2019). For instance, thermal stress can induce overproduction of reactive oxygen species (ROS), which damages biological macromolecules (proteins, lipids, and DNA) and initiates a cascade of cellular defense events (Meng et al., 2014; Luo et al., 2014). Moreover, the physiological performance and gene expression of marine organisms can be disorganized by thermal stress (Clark et al., 2018, 2021; Li et al., 2020; Shi et al., 2020). It has been reported that enzymatic defense system, antioxidant system and apoptosis pathway of marine mollusk can be activated to counteract the adverse effect of oxidative stress induced by temperature changes (Zhang et al., 2012; Zhou et al., 2019; Ning et al., 2021). However, intertidal organisms have poor capacities in acclimation to rapid climate warming (Stillman, 2003). For instance, the growth and gonad development of Crassostrea gigas can be inhibited by thermal discharge from a nuclear power plant (Dong et al., 2018). It is thus necessary to investigate the thermo-resistant mechanisms of intertidal organisms in response to elevating temperatures.
The ark shells, Scapharca subcrenata, are economically important species and widely distributed in the coastal waters of China, Japan, and Korea (Chen et al., 2009). In the past decades, the wild resources of ark shells in China have suffered serious damage owing to overfishing and ecological changes, resulting in the decline of genetic diversity and stress resistance. Moreover, cultured ark shells, especially those cultured in outdoor ponds in China, are vulnerable to high temperature and their growth and survival are often threatened severely by continuous or extreme high temperature in summers (Lin J. L. et al., 2020; Ning et al., 2021). The seawater temperature of outdoor shallow ponds in Northern China can reach as high as 33.5°C in summer (Ning et al., 2015), thus pond cultured ark shells are often exposed to acute or chronic high temperature stress during this period. Therefore, in this study, we investigated the effects of acute or chronic thermal stress on apoptosis, physiological performance, and gene expression of ark shells, which would help to understand the thermal tolerance and contribute to selection of heat-resistant strains in ark shells.
Materials and Methods
Experimental Animals and Determination of the Upper Median Lethal Temperature
Healthy ark shells (23.81 ± 2.05 mm in shell-length, 19.42 ± 1.63 mm in shell-height, 15.68 ± 1.05 mm in shell-width, and 5.10 ± 1.26 g in whole weight; n = 30) were collected from the intertidal flats of Dongying, China (38°08′ N, 118°17′ E). The ark shells were reared in 100-l tanks containing filtered seawater (temperature 18 ± 0.5°C, salinity 28 ± 1 and pH 8.13 ± 0.02), fed with a concentrated microalgae diet and the seawater was changed twice a day in the lab. After acclimation for 1 week, 420 healthy ark shells were randomly divided into six groups, which were heated to 22, 24, 26, 28, 30, 32, and 34°C, respectively, at a rate of 1°C/h and maintained at that temperature for 96 h. The animals were checked once every 6 h and those failed to react to mechanical touch and cannot recover within 1 h in the optimum water temperature were considered as dead and picked out. The water in the tanks were replaced twice every day with clean seawater preheated to the designated temperatures. The 96-h upper LT50 was determined according to the two-point method as described in Liu et al. (2007) (Figure 1).
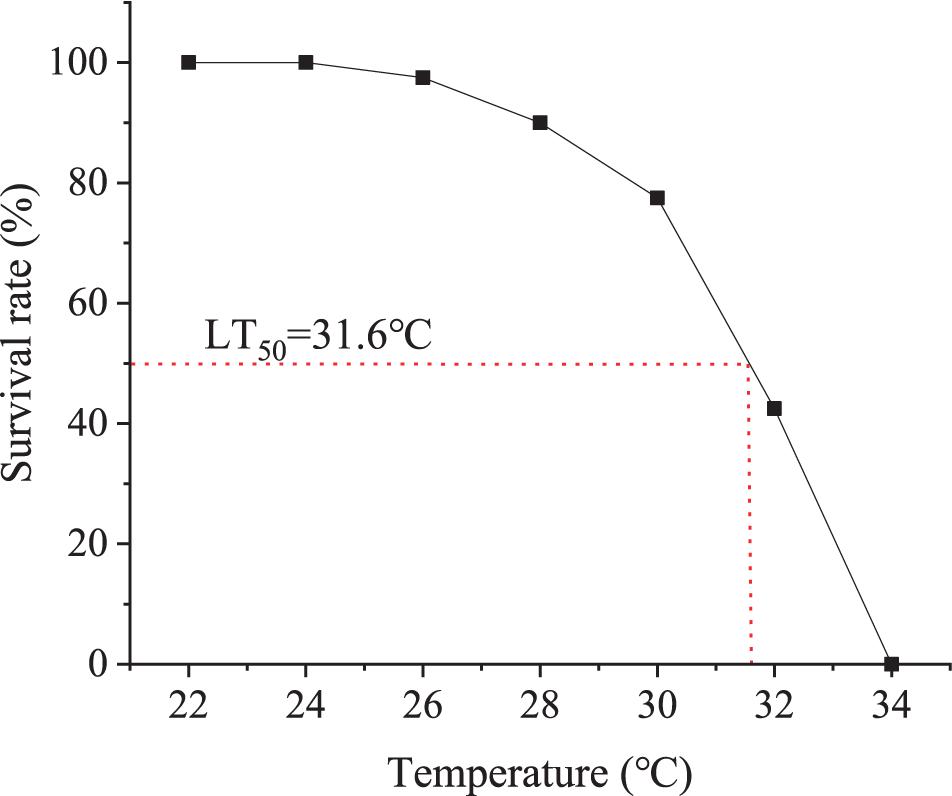
Figure 1. Determination of 96-h LT50. Sixty animals were exposed to 22, 24, 26, 28, 30, 32, and 34°C for 96 h. The survival rate of each group was normalized to percentage of that of the control group at 22°C.
Setup of Acute Heating Treatment and Chronic Heating Treatment
Prior to the experiment, 480 healthy ark shells were randomly selected and divided equally into two experimental groups, one for acute heating treatment (AHT) and another for chronic heating treatment (CHT).
Acute Heating Treatment
The seawater in the tank holding the ark shells was heated rapidly from 18 to 32°C at a rate of 1°C/h. Six animals were randomly sampled at 1, 3, 6, 12, and 24 h after the water reached 32°C (Figure 2A). Six animals maintained at 18°C were sampled at the beginning of the experiment and used as a control. The animals were not fed during the experiment.
Chronic Heating Treatment
The seawater in the tank holding the ark shells was heated slowly from 18 to 32°C at a rate of 1°C/day, and maintained one day every 3°C. Six animals were randomly sampled at Day 1, 2, 3, 4, and 7 after the water reached 32°C (Figure 2B). Six animals maintained at 18°C were sampled at the beginning of the experiment and used as a control. During the experiments, the animals were fed with concentrated microalgae diet twice a day, and the seawater was changed 2 h after each feeding.
The sampled ark shells were dissected immediately to collect their hemocytes. In brief, 0.5 ml of hemolymph was harvested with a sterile syringe pre-filled with an equal volume of precooled anticoagulant buffer (27 mM sodium citrate, 336 mM NaCl, 115 mM glucose, 9 mM EDTA-Na2, pH 7.0), and filtered with a 300 mesh sieve. The hemocytes were saved in a mixture of ice water at 0°C and collected after centrifugation at 1,000 × g at 4°C for 5 min and eventually stored at (80°C until further analyses. Meanwhile, the gill tissues from the same animal were collected and cleaned thoroughly with phosphate buffered saline (PBS, PH 7.4) and stored at −80°C until further analyses. Hemocytes were selected as they are the main components for cellular immune response and non-specific defense mechanism, as well as play a key role in the heat stress response of mollusks (Anestis et al., 2010). Gills are often chosen in analysis of thermal stress response mechanism in aquatic animals as gills, with large surface area, are directly exposed to a variety of environmental factors (Lim et al., 2016).
Determination of Apoptosis and Necrosis Rate of Hemocytes
The hemocytes were resuspended in 195 μl 1 × binding buffer solution at a final concentration of 2.5–5.0 × 105 cells/ml, and stained with 5 μl Annexin V-Fluorescein Isothiocyanate (FITC) and 10 μl Propidium Iodide (PI) dyes at 20°C in dark for 20 min. The cell suspensions were analyzed by the flow cytometry (CytoFLEX, United States). The negative control group without dye addition and the single dye control group with only FITC or PI added were set to determine the position of the cross gate in the scatter diagram (Figure 3). The apoptosis rate of ark shell hemocytes in each sample was represented as the percentage of the number of FITC positive and PI negative cells in the total number of cells. The necrosis rate of the hemocytes in each sample was expressed as the percentage of the number of FITC positive and PI positive cells in the total number of cells (Schutte et al., 1998).
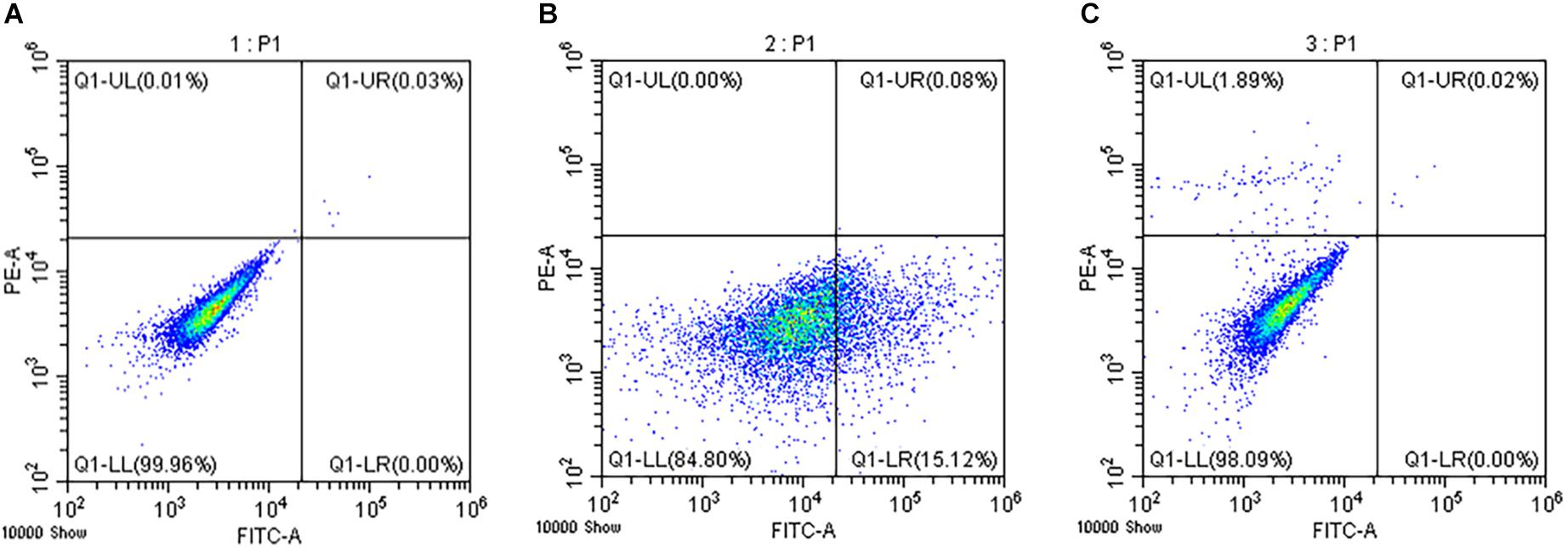
Figure 3. Determination of the apoptosis and necrosis rates. The negative control group without dye addition (A) and the single dye group with FITC (B) or PI (C) only were set to determine the position of the cross gate in the scatter diagram.
Enzyme Activity Assays
Prior to analyses, the frozen gill samples were thawed on ice. About 100 mg gill tissues were homogenized in nine volume of ice-cold PBS buffer (0.01M, pH 7.4) using a homogenizer (T10 Ultra Turrax basic, Germany). After homogenization, the extract was centrifuged at 10,000 × g for 30 min at 4°C, and stored at 4°C until analyzed.
The activities of superoxide dismutase (SOD) and catalase (CAT) and the content of malondialdehyde (MDA) were selected to evaluate the physiological responses to thermal stress. The activities of SOD and CAT were measured using a commercial kit (Nanjing Jiancheng Chemical Industries, China). The activity was expressed as units per mg protein (U mg prot–1). The content of MDA was measured spectrophotometrically with the thiobarbituric acid method according to Esterbauer and Cheeseman (1990) with a kit from the Nanjing Jiancheng Chemical Industries (China). Briefly, gill extract was added to an equal volume of 1% thiobarbituric acid in a 95°C water bath for 40 min. After cooling down on ice, thiobarbituric acid reactive substance was centrifuged at 2,000 × g for 10 min and the supernatant was measured at 532 nm against a blank control consisting of anhydrous ethanol mixed with 1% thiobarbituric acid. Tetraethoxypropane was used as a standard. MDA content was expressed as nmol mg prot–1.
RNA Extraction and cDNA Synthesis
Total RNA was extracted from the frozen gill samples using TRIzol reagent (Invitrogen, United States), and genomic DNA was removed using RNase-free DNase I (TaKaRa, China). The quantity and purity of the RNA samples were determined by the Nanodrop 2000 device (Thermo, United States), and RNA quality was analyzed using 1% agarose gel electrophoresis. The first-strand cDNA was synthesized for the quantitative real-time PCR (qRT-PCR) analyses using a PrimeScriptTM first Strand cDNA Synthesis Kit (TaKaRa, China) with an oligo dT primer.
Gene Expression Analyses
The expression levels of twelve selected genes involved in heat stress responses (Ning et al., 2021) were examined using qRT-PCR. The primers used for the qRT-PCR analyses were listed in Table 1. 18S rRNA was employed as the reference gene. Reactions were carried out on the QuantStudioTM 5 Real-Time PCR Instrument (Applied Biosystems) using SYBR green II as fluorescent dye. PCR amplifications were conducted in 20-μl reaction mixtures containing 10 μl of 2 SYBR Premix Ex Taq (TaKaRa), 1.2 μl 50 × ROX Reference Dye, 2 μl of the diluted cDNA, 0.4 μl of each primer (10 μM), and 6 μl of sterile distilled H2O. The PCR programs were 95°C for 60 s, followed by 40 cycles of 95°C for 5 s and 60°C for 15 s. Melting curve analysis was performed following each PCR reaction to confirm the single amplification product. All reactions were performed in triplicates. The obtained results were analyzed by the 2–ΔΔCt methods (Livak and Schmittgen, 2001).
Statistical Analyses
Statistical analyses were processed using SPSS 17.0 software. Significant differences were determined via one-way analysis of variance and Duncan test. Differences were considered statistically significant at P < 0.05 (∗).
Results
The Cumulative Mortality of Ark Shells Under Acute and Chronic Thermal Stress
In the AHT, the cumulative mortality of ark shells showed a linear increase with time, and reached 52% at 96 h (Figure 4A). Moreover, no feeding and thus no feces were observed during the AHT experiment. The seawater in the tank often turned turbid as the animals died.
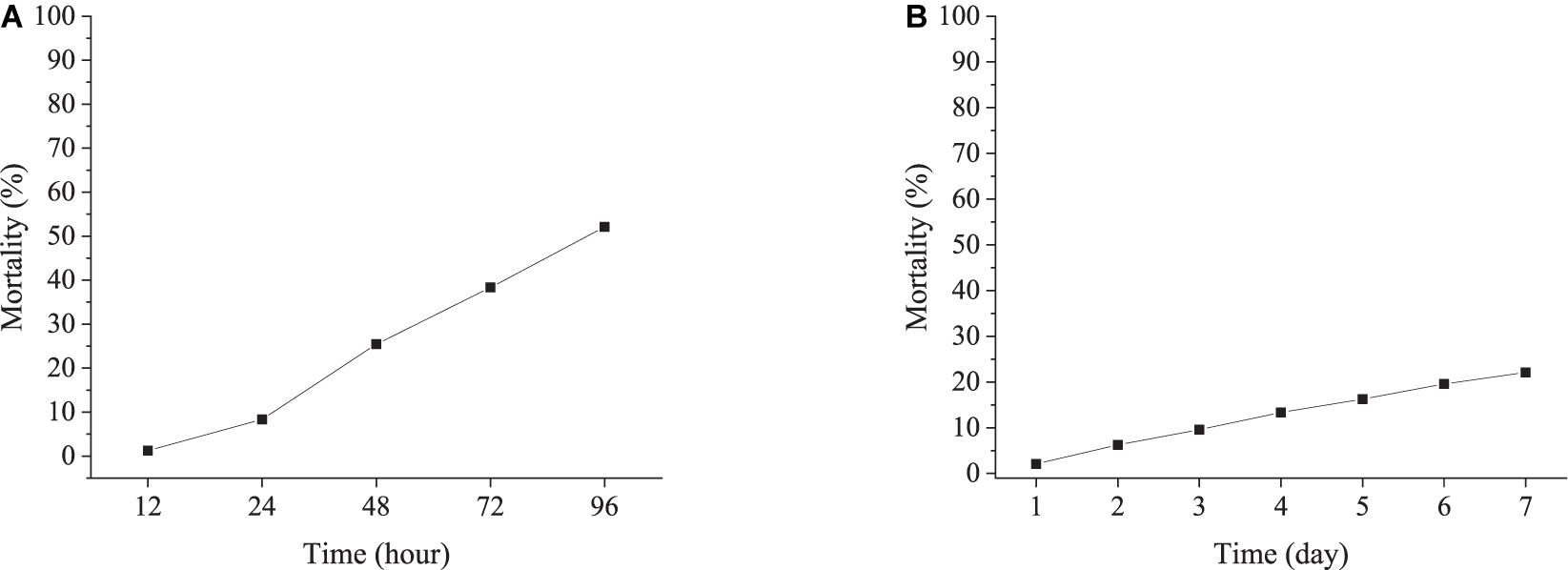
Figure 4. Cumulative mortality of ark shells during the acute (A) and chronic heating experiment (B).
In the CHT group, the cumulative mortality was much lower than that in the AHT, with a cumulative mortality rate of only 22% by 7 days (Figure 4B). The ark shells fed normally and lots of feces were observed during the experiment.
The Apoptosis and Necrosis of Hemocytes Under Acute and Chronic Thermal Stress
The apoptosis and necrosis of ark shell hemocytes were assessed by Annexin V-FITC and PI staining followed by analyses with flow cytometry. In the AHT, the apoptosis rate of hemocytes increased significantly at a short time and showed a linear increase with the prolonging of thermal stress, with the highest value (17.38%) at 12 h. Similar trend was also observed for the necrosis rate of hemocytes during the AHT (Figure 5A).
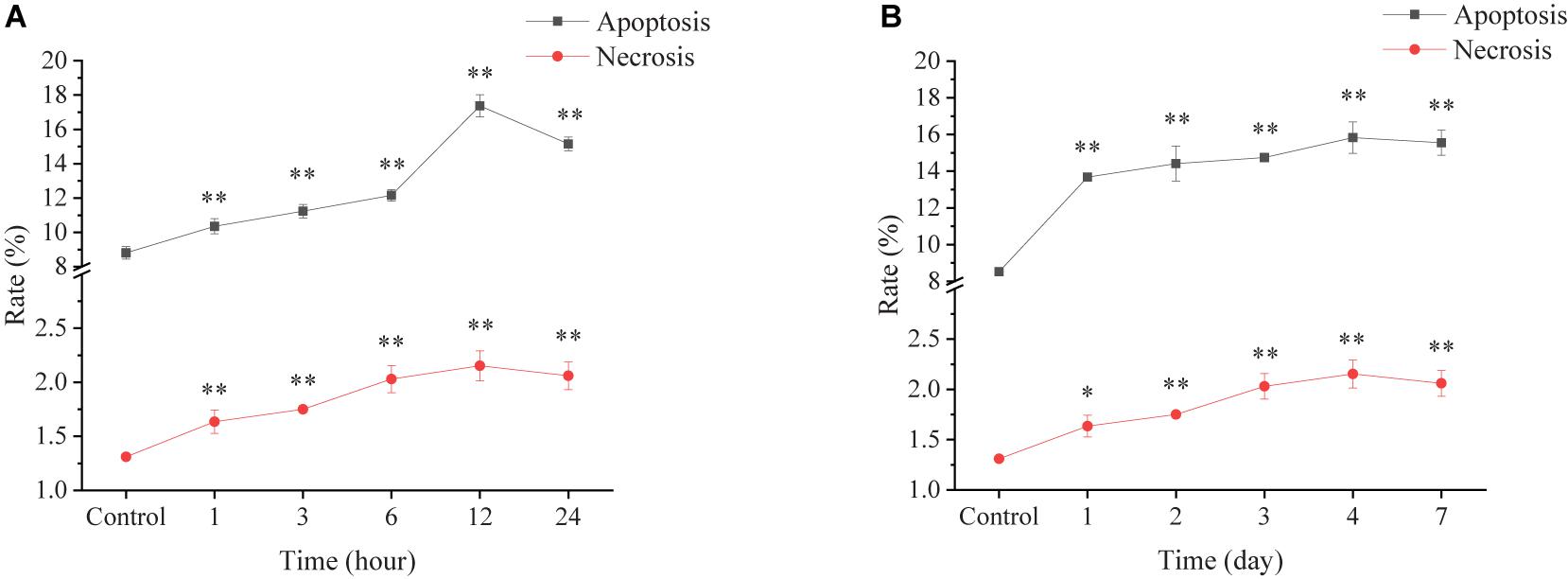
Figure 5. Apoptosis and necrosis rates of ark shell hemocytes under acute (A) and chronic thermal stress (B).
In the CHT group, the apoptosis rate increased significantly at day 1 and sustained steadily high over the whole period of the experiment. The necrosis rate showed a linear increase within first 4 days and decreased a bit at the end of the experiment (Figure 5B).
Changes in Enzyme Activity in the Ark Shell Gill Under Acute Thermal Stress
In the AHT, SOD activity increased significantly at 1 h (24.53 U mg prot–1) followed by a continuous decline over the experimental period. Moreover, the SOD activity became significantly lower than the control group after 6 h (Figure 6A). The CAT activity increased significantly after exposure to high temperature stress, reaching a peak value of 16.40 U mg prot–1 at 3 h, followed by a sharp decrease and became significantly lower than the control group at 12 h (Figure 6B). The content of MDA also increased significantly with time and reached the highest value of 31.94 U mg prot–1 at 6 h, followed by a continuous decline (Figure 6C).
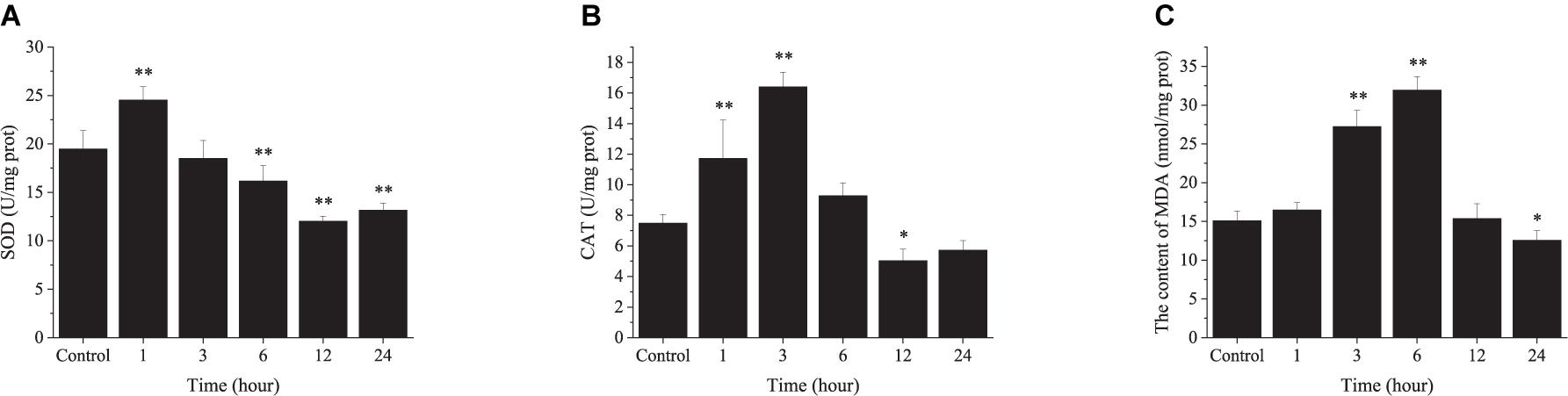
Figure 6. The changes in enzyme activity in the ark shell gill under acute thermal stress. SOD, superoxide dismutase (A); CAT, catalase (B); MDA, malondialdehyde (C).
The Changes in Enzyme Activity in the Ark Shell Gill Under Chronic Thermal Stress
In the CHT, SOD activity was increased significantly at day 1 and day 2, and restored to the initial level after day 3 (Figure 7A). CAT activity was increased significantly at day 2 and remained elevated until the end of the experiment (Figure 7B). The content of MDA was increased significantly at day 1 and day 3, and returned to the initial level at day 4 (Figure 7C).
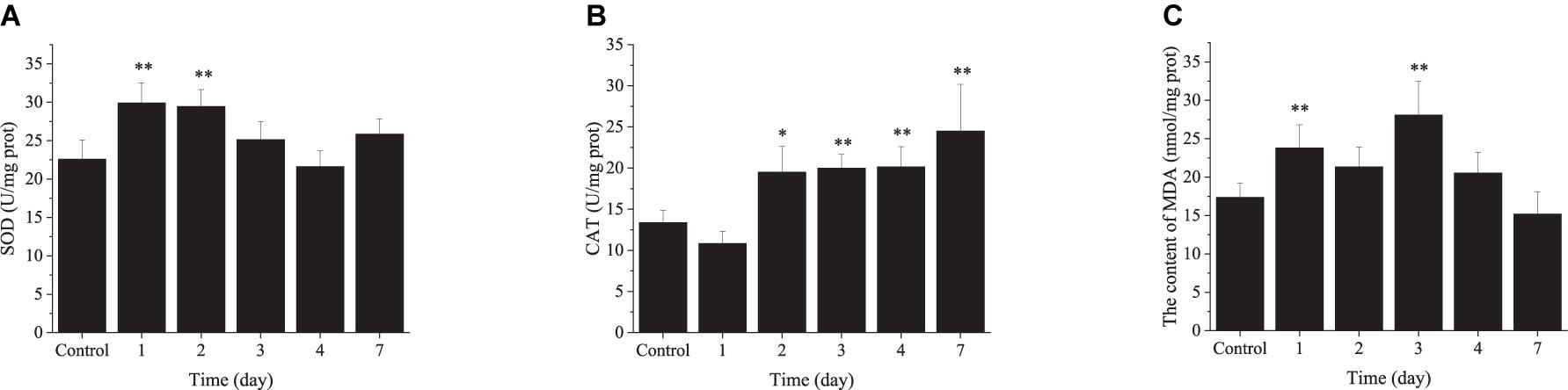
Figure 7. The changes in enzyme activity in the ark shell gill under chronic thermal stress. SOD, superoxide dismutase (A); CAT, catalase (B); MDA, malondialdehyde (C).
Transcriptional Response in the Ark Shell Gills to Acute Thermal Stress
As shown in Figure 8, the expression of selected genes related to stress proteins (HSP90, HSP70, HSP20, and sHSP), apoptosis [TNF receptor-associated factor (TRAF), glucose regulated protein 78 kD (GRP78), and caspase-3 (Casp-3)] and antioxidative [glutathione S-transferase (GST) and multidrug resistance protein (MRP)] were up-regulated significantly under acute thermal stress, with peak values observed at 3 or 6 h. However, compared with the control groups, the expression of peroxiredoxin (PRX), regucalcin (RGN), and metallothionein (MT) was down-regulated significantly in the AHT.
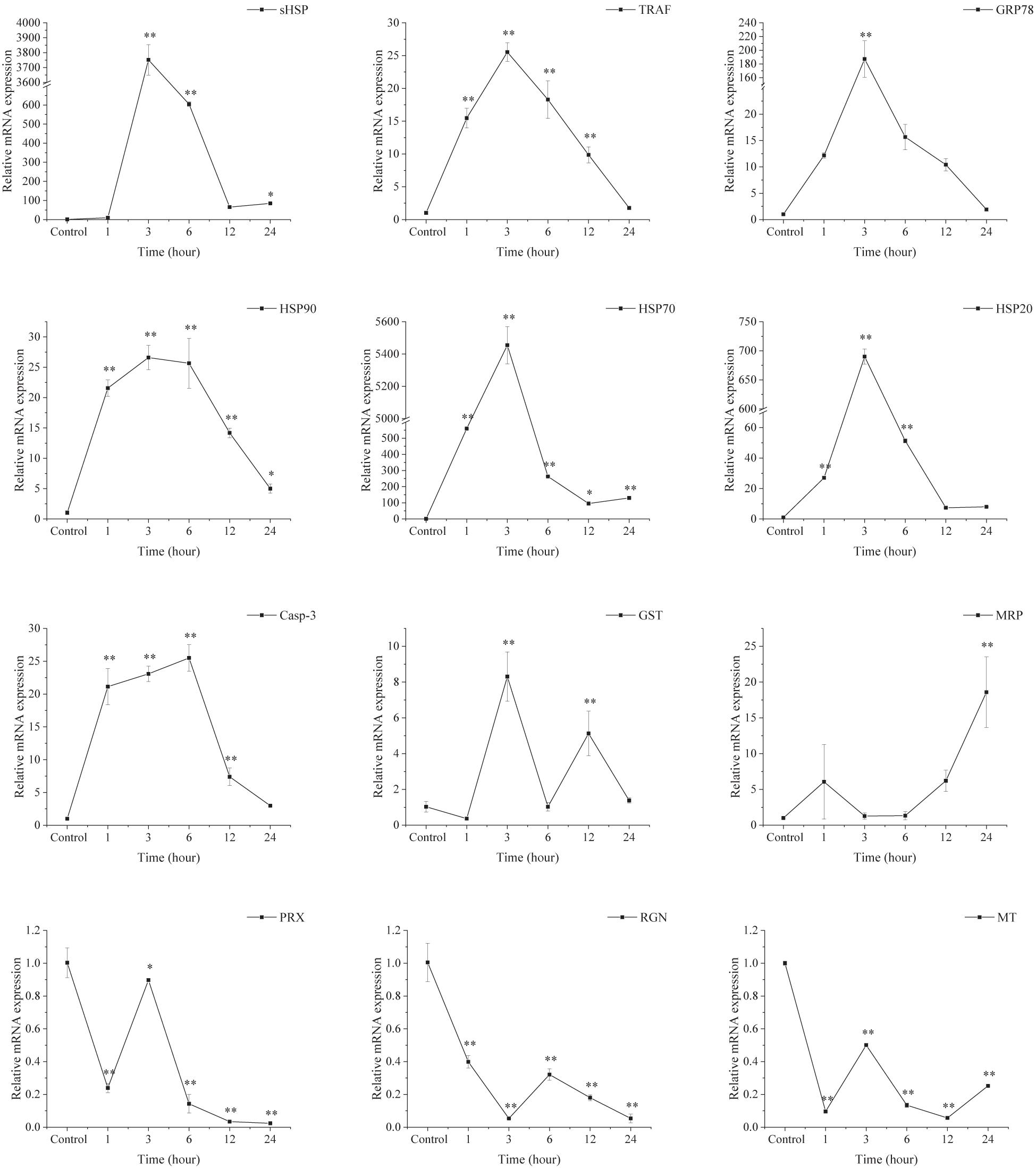
Figure 8. Transcriptional response of ark shell gills to acute thermal stress. GST, glutathione S-transferase; GRP78, glucose regulated protein 78 kD; TRAF-6, TNF receptor-associated factor 6; Casp-3, caspase-3; MRP, multidrug resistance protein; HSP, heat shock protein; RGN, regucalcin; PRX, peroxiredoxin; MT, metallothionein.
Transcriptional Response of Ark Shell Gills to Chronic Thermal Stress
As depicted in Figure 9, the expression of the selected genes involved in stress proteins (HSP90, HSP70, HSP20, and sHSP), apoptosis (TRAF, GRP78, and Casp-3) and antioxidative (GST and MRP) were up-regulated significantly during the CHT experiment, with peak values mainly at day 2. However, the expression of PRX and RGN was down-regulated significantly in the CHT. Noticeably, the expression of MT was increased dramatically at day 1 and day 2, but decreased sharply after day 3 and remained down-regulated until the end of the experiment.
Discussion
Temperature, as an important abiotic factor, can substantially affect the physiological responses in marine organisms (Windisch et al., 2011; Dong et al., 2019). In this study, the 96-h upper LT50 of ark shells was determined to be 31.6°C, based upon acute temperature tolerance experiments. However, the highest temperature in outdoor ponds or tidal flats in summer is far beyond this temperature, indicating that the cultured ark shells might be subjected to severe thermal stress. Moreover, the high temperature stress could last for up to over 1 week, suggesting that the ark shells could suffer from a long-term thermal stress. Therefore, it is important to examine the responses of the ark shells to both acute and chronic thermal stress.
Apoptosis, also called programmed cell death, is a vital physiological protective mechanism of organisms in adaptive responses to environmental stress, which can be induced significantly by high temperature stress (Zhang et al., 2012; Cheng et al., 2018; Zhou et al., 2019). However, severe stress may also cause necrosis when the stress is beyond the tolerance of the animal. In consistent with previously published results in oyster (Yang et al., 2017), we also observed that the apoptosis and necrosis rates of hemocytes were increased significantly in a time-dependent manner under both acute and chronic thermal stress, suggesting the apoptosis process of hemocytes has been activated and the stress injury gradually became irreparable with the prolonging of stress time and eventually caused necrosis in the ark shells. This is supported by the significant upregulation of Casp-3, a member of cysteine-dependent protease families that plays important roles in the activation of apoptotic pathway (Qin et al., 2020) in the two thermal stress treatments. It is notable that higher apoptosis level was observed under the acute thermal stress than under the chronic stress, suggesting the ark shells suffered more damage when exposed to acute temperature changes. The higher cumulative mortality rates of ark shells in the AHT also support this point.
In order to counteract the irreparable injuries caused by apoptosis, anti-apoptotic pathways could be activated immediately when organisms suffer from abiotic stresses. The expansion of inhibitors of apoptosis proteins in the oyster genome and their response to various stresses are crucial for oysters in adaption to sessile life in the highly stressful intertidal zone (Zhang et al., 2012). Glucose regulated protein 78 kD (GRP78) is an essential molecular chaperone located in the endoplasmic reticulum (Melnick et al., 1994; Ni et al., 2011). It has been reported that GRP78 could reduce apoptosis induced injuries by suppression of Caspase 7 activation (Reddy et al., 2003). Tumor necrosis factor receptor-associated factors (TRAFs) TRAFs plays crucial roles in stress resistance. Among which, TRAF6 is an important signaling molecule involving in cell proliferation and metabolism through a series of signal transduction (Rothe et al., 1995). As reported in recent study, TRAF6 could suppress the apoptosis of oyster hemocytes through activation of Pellino (Lin Y. et al., 2020). In the present study, the expression of anti-apoptosis related genes (GRP78 and TRAF6) was up-regulated significantly, indicating anti-apoptotic system also exists in ark shells in response to thermal stresses. This has been confirmed by our previous study according to the transcriptomic analyses (Ning et al., 2021).
Marine invertebrates are equipped with antioxidant defense system such as SOD and CAT to maintain the balance between normal physiological function and ROS accumulation (Lesser, 2006; Soldatov et al., 2007). SOD can catalyze the conversion of intracellular superoxide radicals into hydrogen peroxide (H2O2) and molecule oxygen (O2), whereas CAT can convert the H2O2 into O2 and H2O. Therefore, the elevated activities of antioxidant enzymes are essential for animal to counteract oxidative damage induced by thermal stress (Cheng et al., 2018). In this study, the activities of SOD and CAT can be induced and increased significantly in response to both acute and chronic thermal stress, which was consistent with previous studies in Yesso scallop (Jiang et al., 2016) and Pacific oyster (Ding et al., 2020). However, the activities of SOD and CAT were decreased and lower than the initial level after 12 h under the acute thermal stress, suggesting the antioxidant ability could be repressed with the extension of acute thermal stress. This is consistent with the conclusion observed in giant clam, Tridacna crocea (Zhou et al., 2019), indicating a transition occurs from aerobic to anaerobic metabolism. Just as shown in our previous study, ark shells may suffer irreparable injuries due to oxygen limitation and metabolic dysregulation if the stress sustains (Ning et al., 2021).
Malondialdehyde content has been widely used as a marker for the extent of oxidative damage in marine organisms (Talmage and Gobler, 2011; Ferreira et al., 2019). In this study, the content of MDA was increased significantly firstly under the two thermal stress modes, suggesting the elevated seawater temperature induced oxidative damage in ark shells. However, the content of MDA was decreased and restored to the initial level subsequently, probably because the increased activity of antioxidant enzymes in a short time removed harmful substances induced by thermal stress (Wang et al., 2020). The enhanced activities of SOD and CAT were also observed under the two thermal stress modes in this study. The restored expression levels of most stress-related genes (GST, GRP78, TRAF-6, Casp-3, MRP, sHSP, HSP70, and HSP90) at day 4 also support the above viewpoint. Moreover, the higher MDA content under the acute thermal stress than under the chronic stress is consistent with the results found in Yesso scallop (Jiang et al., 2016), suggests that ark shells suffered more damage during the acute temperature changes.
Except for antioxidant enzymes, non-enzymatic antioxidant defense mechanisms also existed in marine invertebrates to prevent oxidative damage (Jiang et al., 2016). Peroxiredoxin is widely existed in prokaryotes and eukaryotes, which plays a vital role in the elimination of metabolically produced peroxides (Rothe et al., 1995; Fujii and Ikeda, 2002; Papadia et al., 2008). MT is responsible for removing metal ion toxicity through combination with them, which is involved in scavenging free radicals and the process of stress resistance (Masters et al., 1994; Carginale et al., 2000). RGN has the function of maintaining the dynamic balance of Ca2+ in cells and overexpression of RGN could inhibit cell death and apoptosis caused by a variety of signaling factors (Laurentino et al., 2011). In marine mollusks, the anti-oxidative responses to thermal stress are complicated and often affected by the differences in temperature gradient and exposure duration (Ning et al., 2021). Similar results were also observed in this study, with expression of GST and MRP up-regulated significantly and PRX, MT, and RGN down-regulated in both thermal stress modes. In consistence with previous results observed in oyster (Lim et al., 2016) and ark shells (Ning et al., 2021), our results suggest that marine mollusks may consume more energy for their redox responses.
Heat shock proteins (HSPs) are a group of highly conserved proteins which play important roles in cellular homeostasis after exposure to drastic environmental changes (Kayhan and Duman, 2010). HSP70s and HSP90s are well-known stress proteins in response to thermal stress in many marine mollusks (Farcy et al., 2007; Ivanina et al., 2009; Kim et al., 2009; Lim et al., 2016). HSP20s (stress induced protein 1) could promote the regeneration of denatured proteins and prevent stress induced protein aggregation when suffered elevated temperature stress (Nover and Scharf, 1997; Ning et al., 2021). In this study, the expression of HSPs (sHSP, HSP20, HSP70 and HSP90) were up-regulated significantly under both thermal stress modes. These results are consistent with our previous study (Ning et al., 2021), suggesting that the significant up-regulation of HSPs may help ark shells become more resistant to elevated seawater temperature. Moreover, the fact that the expression levels of HSPs (sHSP, HSP20, and HSP70) in the AHT were much higher than that in the CHT mode may imply that the ark shells were subjected to more severe cellular damage induced by acute thermal stress. Similar results were also observed in Yesso scallop (Jiang et al., 2016) and pufferfish, Takifugu obscurus (Cheng et al., 2018).
Conclusion
In summary, the physiological performance and gene expression were affected strongly by both acute and chronic thermal stress in ark shells. The apoptosis and necrosis rates of hemocytes were stimulated significantly in a time-dependent manner, and the content of MDA was increased in gills, indicating elevated seawater temperature has caused oxidative stress to ark shells. Thermal stress also induced actions in the anti-apoptotic system, antioxidant defense system and HSPs to protect ark shells from oxidative damage. The activities of antioxidant enzyme and expression of most genes in the CHT could be restored to initial level, which did not occur in the AHT, suggesting that the damage caused by rapid ambient temperature increase is irreversible, and thus more harmful to marine mollusks. Our results may provide more clues for management strategy of ark shell husbandry and further understanding of the mechanisms underlying the tolerance of ark shells to high temperature stress.
Data Availability Statement
The raw data supporting the conclusions of this article will be made available by the authors, without undue reservation.
Author Contributions
DZ: investigation and roles/writing – original draft. JN: methodology, validation, software, and writing – review and editing. XL: supervision, formal analysis, and visualization. XW: validation and data curation. MC: software and data curation. BL: conceptualization and resources. JF: conceptualization and resources. CW: funding acquisition, writing – review and editing, and project administration. All authors contributed to manuscript revision, read, and approved the submitted version.
Funding
This research was supported by the National Key R&D Program of China (2019YFD0900704), the National Natural Science Foundation of China (3197210450), the Scientific and Technological Project of Yantai, Shandong Province (2019LJRC141), and the Key Deployment Projects of Center for Ocean Mega-Science, Chinese Academy of Sciences (ZR2017QD001).
Conflict of Interest
The authors declare that the research was conducted in the absence of any commercial or financial relationships that could be construed as a potential conflict of interest.
Publisher’s Note
All claims expressed in this article are solely those of the authors and do not necessarily represent those of their affiliated organizations, or those of the publisher, the editors and the reviewers. Any product that may be evaluated in this article, or claim that may be made by its manufacturer, is not guaranteed or endorsed by the publisher.
Acknowledgments
We are grateful to all the laboratory members and reviewers for their technical advice and helpful discussions.
References
Anestis, A., Portner, H. O., Karagiannis, D., Angelidis, P., Staikou, A., and Michaelidis, B. (2010). Response of Mytilus galloprovincialis (L.) to increasing seawater temperature and to marteliosis: metabolic and physiological parameters. Comp. Biochem. Physiol. A Mol. Integr. Physiol. 156, 57–66. doi: 10.1016/j.cbpa.2009.12.018
Burrows, M. T., Bates, A. E., Costello, M. J., Edwards, M., Edgar, G. J., Fox, C. J., et al. (2019). Ocean community warming responses explained by thermal affinities and temperature gradients. Nat. Clim. Chang. 9, 959–963. doi: 10.1038/s41558-019-0631-5
Carginale, V., Scudiero, R., Capasso, C., and Parisi, E. (2000). Metallothionein in Antarctic notothenioids: genetic polymorphism and differential gene expression. Ital. J. Zool. 67, 13–20. doi: 10.1080/11250000009356350
Chen, M. Y., Yang, H. S., Delaporte, M., and Zhao, S. J. (2007). Immune condition of Chlamys farreri in response to acute temperature challenge. Aquaculture 271, 479–487. doi: 10.1016/j.aquaculture.2007.04.051
Chen, S. Y., Ji, H. J., Xu, S. X., Zhang, Z. W., and Xu, X. F. (2009). Polymorphic microsatellite DNA markers for the ark shell Scapharca subcrenata (bivalve: Arcidae). Conserv. Genet. 10, 1409–1412. doi: 10.1007/s10592-008-9742-7
Cheng, C. H., Guo, Z. X., Luo, S. W., and Wang, A. L. (2018). Effects of high temperature on biochemical parameters, oxidative stress, DNA damage and apoptosis of pufferfish (Takifugu obscurus). Ecotox. Environ. Safe 150, 190–198. doi: 10.1016/j.ecoenv.2017.12.045
Clark, M. S., Peck, L. S., and Thyrring, J. (2021). Resilience in Greenland intertidal Mytilus: the hidden stress defense. Sci. Total Environ. 767:144366. doi: 10.1016/j.scitotenv.2020.144366
Clark, M. S., Thorne, M. A. S., King, M., Hipperson, H., Hoffman, J. I., and Peck, L. S. (2018). Life in the intertidal: cellular responses, methylation and epigenetics. Funct. Ecol. 32, 1982–1994. doi: 10.1111/1365-2435.13077
Ding, F. F., Li, A., Cong, R. H., Wang, X. X., Wang, W., Que, H. Y., et al. (2020). The phenotypic and the genetic response to the extreme high temperature provides new insight into thermal tolerance for the pacific oyster Crassostrea gigas. Front. Mar. Sci. 7:399. doi: 10.3389/fmars.2020.00399
Dong, Z. G., Chen, Y. H., Ge, H. X., Li, X. Y., Wu, H. L., Wang, C. H., et al. (2018). Response of growth and development of the Pacific oyster (Crassostrea gigas) to thermal discharge from a nuclear power plant. BMC Ecol. 18:31. doi: 10.1186/s12898-018-0191-y
Dong, Z. G., Mao, S., Chen, Y. H., Ge, H. X., Li, X. Y., Wu, X. G., et al. (2019). Effects of air-exposure stress on the survival rate and physiology of the swimming crab Portunus trituberculatus. Aquaculture 500, 429–434. doi: 10.1016/j.aquaculture.2018.10.049
Esterbauer, H., and Cheeseman, K. H. (1990). Determination of aldehydic lipid peroxidation products: malonaldehyde and 4-hydroxynonenal. Methods Enzymol. 186, 407–421. doi: 10.1016/0076-6879(90)86134-h
Farcy, E., Voiseux, C., Lebel, J. M., and Fievet, B. (2007). Seasonal changes in mRNA encoding for cell stress markers in the oyster Crassostrea gigas exposed to radioactive discharges in their natural environment. Sci. Total Environ. 374, 328–341. doi: 10.1016/j.scitotenv.2006.11.014
Ferreira, C. M., Nagelkerken, I., Goldenberg, S. U., Walden, G., Leung, J. Y. S., and Connell, S. D. (2019). Functional loss in herbivores drives runaway expansion of weedy algae in a near-future ocean. Sci. Total Environ. 695:133829. doi: 10.1016/j.scitotenv.2019.133829
Fujii, J., and Ikeda, Y. (2002). Advances in our understanding of peroxiredoxin, a multifunctional, mammalian redox protein. Redox Rep. 7, 123–130. doi: 10.1179/135100002125000352
Hoegh-Guldberg, O., Mumby, P. J., Hooten, A. J., Steneck, R. S., Greenfield, P., Gomez, E., et al. (2007). Coral reefs under rapid climate change and ocean acidification. Science 318, 1737–1742. doi: 10.1126/science.1152509
Ivanina, A. V., Taylor, C., and Sokolova, I. M. (2009). Effects of elevated temperature and cadmium exposure on stress protein response in eastern oysters Crassostrea virginica (Gmelin). Aquat. Toxicol. 91, 245–254. doi: 10.1016/j.aquatox.2008.11.016
Jiang, W. W., Li, J. Q., Gao, Y. P., Mao, Y. Z., Jiang, Z. J., Du, M. R., et al. (2016). Effects of temperature change on physiological and biochemical responses of Yesso scallop, Patinopecten yessoensis. Aquaculture 451, 463–472. doi: 10.1016/j.aquaculture.2015.10.012
Kayhan, F. E., and Duman, B. S. (2010). Heat shock protein genes in fish. Turk. J. Fish. Quat. Sci. 10, 287–293. doi: 10.4194/trjfas.2010.0218
Kim, M., Ahn, I. Y., Kim, H., Cheon, J., and Park, H. (2009). Molecular characterization and induction of heat shock protein 90 in the Antarctic bivalve Laternula elliptica. Cell Stress Chaperones 14, 363–370. doi: 10.1007/s12192-008-0090-9
Laurentino, S. S., Correia, S., Cavaco, J. E., Oliveira, P. F., Rato, L., Sousa, M., et al. (2011). Regucalcin is broadly expressed in male reproductive tissues and is a new androgen-target gene in mammalian testis. Reproduction 142, 447–456. doi: 10.1530/rep-11-0085
Lesser, M. P. (2006). Oxidative stress in marine environments: biochemistry and physiological ecology. Annu. Rev. Physiol. 68, 253–278. doi: 10.1146/annurev.physiol.68.040104.110001
Li, Y. F., Yang, X. Y., Cheng, Z. Y., Wang, L. Y., Wang, W. X., Liang, X., et al. (2020). Near-future levels of ocean temperature weaken the byssus production and performance of the mussel Mytilus coruscus. Sci. Total Environ. 733:139347. doi: 10.1016/j.scitotenv.2020.139347
Lim, H. J., Kim, B. M., Hwang, I. J., Lee, J. S., Choi, I. Y., Kim, Y. J., et al. (2016). Thermal stress induces a distinct transcriptome profile in the Pacific oyster Crassostrea gigas. Comp. Biochem. Physiol. Part D Genomics Proteomics 19, 62–70. doi: 10.1016/j.cbd.2016.06.006
Lin, J. L., Ning, J. H., Lu, X., Chen, M., Cao, W. A., and Wang, C. D. (2020). Transcriptomic analysis and expression of C-type lectins in response to Vibrio parahaemolyticus challenge in Scapharca subcrenata. Fish Shellfish Immunol. 106, 365–373. doi: 10.1016/j.fsi.2020.08.016
Lin, Y., Mao, F., Zhang, X. Y., Xu, D., He, Z. Y., Li, J., et al. (2020). TRAF6 suppresses the apoptosis of hemocytes by activating pellino in Crassostrea hongkongensis. Dev. Comp. Immunol. 103:103501. doi: 10.1016/j.dci.2019.103501
Liu, Z. G., Wang, H., Li, Z. M., and Zhang, Y. L. (2007). Upper incipient lethal temperature of Argopecten irradians concentricus Say. J. Fish. Sci. China 14, 778–785.
Livak, K. J., and Schmittgen, T. D. (2001). Analysis of relative gene expression data using real-time quantitative PCR and the 2(T)(-Delta Delta C) method. Methods 25, 402–408. doi: 10.1006/meth.2001.1262
Luo, S. W., Cai, L., Liu, Y., and Wang, W. N. (2014). Functional analysis of a dietary recombinant Fatty acid binding protein 10 (FASP10) on the Epinephelus coioides in response to acute low temperature challenge. Fish Shellfish Immunol. 36, 475–484. doi: 10.1016/j.fsi.2013.12.028
Masters, B. A., Kelly, E. J., Quaife, C. J., Brinster, R. L., and Palmiter, R. D. (1994). Targeted disruption of metallothionein I and II genes increases sensitivity to cadmium. Proc. Natl. Acad. Sci. U.S.A. 91, 584–588. doi: 10.1073/pnas.91.2.584
Melnick, J., Dul, J. L., and Argon, Y. (1994). Sequential interaction of the chaperones BiP and GRP94 with immunoglobulin chains in the endoplasmic reticulum. Nature 370, 373–375. doi: 10.1038/370373a0
Meng, X. L., Liu, P., Li, J., Gao, B. Q., and Chen, P. (2014). Physiological responses of swimming crab Portunus trituberculatus under cold acclimation: antioxidant defense and heat shock proteins. Aquaculture 434, 11–17. doi: 10.1016/j.aquaculture.2014.07.021
Monaco, C. J., and McQuaid, C. D. (2019). Climate warming reduces the reproductive advantage of a globally invasive intertidal mussel. Biol. Invasions 21, 2503–2516. doi: 10.1007/s10530-019-01990-2
Ni, M., Zhang, Y., and Lee, A. S. (2011). Beyond the endoplasmic reticulum: atypical GRP78 in cell viability, signalling and therapeutic targeting. Biochem. J. 434, 181–188. doi: 10.1042/bj20101569
Ning, J., Zou, D., Lu, X., Cao, W., Chen, M., Liu, B., et al. (2021). Transcriptomic analyses provide insights into the adaptive responses to heat stress in the ark shells, Scapharca subcrenata. Comp. Biochem. Physiol. Part D Genomics Proteomics 38:100813. doi: 10.1016/j.cbd.2021.100813
Ning, J. H., Chang, Y. Q., Liu, W., Song, J., Zhang, W. J., and Ding, J. (2015). Stress responses to mild and acute temperature decrease for two strains of sea cucumber Apostichopus japonicus. Aquaculture 448, 552–563. doi: 10.1016/j.aquaculture.2015.06.035
Nover, L., and Scharf, K. D. (1997). Heat stress proteins and transcription factors. Cell. Mol. Life Sci. 53, 80–103. doi: 10.1007/pl00000583
Papadia, S., Soriano, F. X., Leveille, F., Martel, M. A., Dakin, K. A., Hansen, H. H., et al. (2008). Synaptic NMDA receptor activity boosts intrinsic antioxidant defenses. Nat. Neurosci. 11, 476–487. doi: 10.1038/nn2071
Qin, Y. P., Zhang, Y. H., Li, X. Y., Noor, Z., Li, J., Zhou, Z. H., et al. (2020). Characterization and functional analysis of a caspase 3 gene: evidence that ChCas 3 participates in the regulation of apoptosis in Crassostrea hongkongensis. Fish Shellfish Immunol. 98, 122–129. doi: 10.1016/j.fsi.2020.01.007
Reddy, R. K., Mao, C. H., Baumeister, P., Austin, R. C., Kaufman, R. J., and Lee, A. S. (2003). Endoplasmic reticulum chaperone protein GRP78 protects cells from apoptosis induced by topoisomerase inhibitors–role of ATP binding site in suppression of caspase-7 activation. J. Biol. Chem. 278, 20915–20924. doi: 10.1074/jbc.M212328200
Rothe, M., Sarma, V., Dixit, V. W., and Goeddel, D. V. (1995). TRAF2-mediated activation of NF-kappa B by TNF receptor 2 and CD40. Science 269, 1424–1427. doi: 10.1126/science.7544915
Schutte, B., Nuydens, R., Geerts, H., and Ramaekers, F. (1998). Annexin V binding assay as a tool to measure apoptosis in differentiated neuronal cells. J. Neurosci. Methods 86, 63–69. doi: 10.1016/s0165-0270(98)00147-2
Shi, K. P., Li, J. T., Lv, J. J., Liu, P., Li, J., and Li, S. D. (2020). Full-length transcriptome sequences of ridgetail white prawn Exopalaemon carinicauda provide insight into gene expression dynamics during thermal stress. Sci. Total Environ. 747:141238. doi: 10.1016/j.scitotenv.2020.141238
Soldatov, A. A., Gostyukhina, O. L., and Golovina, I. V. (2007). Antioxidant enzyme complex of tissues of the bivalve Mytilus galloprovincialis Lam. under normal and oxidative-stress conditions: a review. Appl. Biochem. Microbiol. 43, 556–562. doi: 10.1134/s0003683807050092
Stillman, J. H. (2003). Acclimation capacity underlies susceptibility to climate change. Science 301:65. doi: 10.1126/science.1083073
Talmage, S. C., and Gobler, C. J. (2011). Effects of elevated temperature and carbon dioxide on the growth and survival of larvae and juveniles of three species of northwest Atlantic bivalves. PloS One 6:e26941. doi: 10.1371/journal.pone.0026941
Tomanek, L., and Zuzow, M. J. (2010). The proteomic response of the mussel congeners Mytilus galloprovincialis and M-trossulus to acute heat stress: implications for thermal tolerance limits and metabolic costs of thermal stress. J. Exp. Biol. 213, 3559–3574. doi: 10.1242/jeb.041228
Wang, B. Y., Gao, C., Huang, P. Y., and Chai, L. H. (2020). Effects of acute raising temperature stress and recovery on antioxidant enzyme activities of Amur sleeper Perccottus glenii. Fish. Sci. 39, 394–399.
Windisch, H. S., Kathover, R., Portner, H. O., Frickenhaus, S., and Lucassen, M. (2011). Thermal acclimation in Antarctic fish: transcriptomic profiling of metabolic pathways. Am. J. Physiol. Regul. Integr. Comp. Physiol. 301, R1453–R1466. doi: 10.1152/ajpregu.00158.2011
Yang, C. Y., Gao, Q., Liu, C., Wang, L. L., Zhou, Z., Gong, C. H., et al. (2017). The transcriptional response of the Pacific oyster Crassostrea gigas against acute heat stress. Fish Shellfish Immunol. 68, 132–143. doi: 10.1016/j.fsi.2017.07.016
Zhang, G. F., Fang, X. D., Guo, X. M., Li, L., Luo, R. B., Xu, F., et al. (2012). The oyster genome reveals stress adaptation and complexity of shell formation. Nature 490, 49–54. doi: 10.1038/nature11413
Keywords: Scapharca subcrenata, thermal stress, apoptosis, antioxidant enzymes, gene expression response
Citation: Zou D, Ning J, Lu X, Wang X, Chen M, Liu B, Fang J and Wang C (2021) Physiological and Transcriptional Responses to Acute and Chronic Thermal Stress in the Ark Shell Scapharca subcrenata. Front. Mar. Sci. 8:739662. doi: 10.3389/fmars.2021.739662
Received: 11 July 2021; Accepted: 31 July 2021;
Published: 03 September 2021.
Edited by:
Guangxu Liu, Zhejiang University, ChinaReviewed by:
Zhiguo Dong, Jiangsu Ocean University, ChinaDonghong Niu, Shanghai Ocean University, China
Copyright © 2021 Zou, Ning, Lu, Wang, Chen, Liu, Fang and Wang. This is an open-access article distributed under the terms of the Creative Commons Attribution License (CC BY). The use, distribution or reproduction in other forums is permitted, provided the original author(s) and the copyright owner(s) are credited and that the original publication in this journal is cited, in accordance with accepted academic practice. No use, distribution or reproduction is permitted which does not comply with these terms.
*Correspondence: Junhao Ning, amhuaW5nQHlpYy5hYy5jbg==; Chunde Wang, Y2h1bmRld2FuZzIwMDdAMTYzLmNvbQ==