- 1Cawthron Institute, Nelson, New Zealand
- 2Nelson Marlborough Institute of Technology, Nelson, New Zealand
- 3Ministry for Primary Industries, Wellington, New Zealand
- 4Marine Invasive Species Program, California State Lands Commission, Sacramento, CA, United States
Internal seawater systems (ISS) are critical to the proper functioning of maritime vessels. Sea water is pumped on board ships for a broad array of uses, primarily for temperature control (e.g., engine and electrical systems), cooling capacity (e.g., air conditioners and refrigeration), and water provision (e.g., drinking, firefighting, steam, and ballast). Although sea water may spend only a brief period within ISS of a vessel, it can carry microorganisms and larval stages of macroorganisms throughout the system leading to biofouling accumulation that can impair system function or integrity. ISS can also act as a sub-vector of species translocations, potentially facilitating biological invasions. This review describes ships’ ISS with a focus on operational impacts of biofouling and current drivers and barriers associated with ISS biofouling management. As ISS internal components are difficult to access, reports and studies of ISS biofouling are uncommon and much of the dedicated literature is decades old. The impact of biofouling on ISS and vessel operations is based on increased surface roughness of pipework and equipment, restricted water flow, corrosion and subsequent component impingement, reduced surface functional efficiency, and potential contamination by pathogens that can affect human and aquatic animal health. Biofouling management is primarily achieved using antifouling coatings and marine growth prevention systems, but independent and accessible data on their efficacy in ISS remain limited. Further research is required to resolve the extent to which biofouling occurs in ISS of the modern commercial fleet and the efficacy of preventive systems. Such information can ultimately inform decisions to improve operational efficiency for vessel operators and ensure any biosecurity risks are appropriately managed.
Introduction
Biofouling is a ubiquitous and enduring problem for the maritime shipping industry, requiring constant management to optimize operational performance (Townsin, 2003; Dafforn et al., 2011; Davidson et al., 2016). Coating roughness, biofilms, and algal and animal biofouling all contribute to a gradient of operational drag that can increase annual vessel running costs by several millions of dollars (Townsin, 2003; Schultz et al., 2011). The hydrodynamic deficiencies of increased surface roughness can drive up fuel costs to levels that greatly exceed biofouling management costs (Schultz et al., 2011). As such, the incentive for proactive, or preventive, biofouling management of external submerged surfaces is obvious for vessel operators. An equivalent understanding of biofouling occurrence and impairment of ships’ internal seawater systems (ISS)—drawing from various fields of engineering, ship operations, biology, and economics—has not been developed despite long-standing questions on the topic (Houghton and Gage, 1979; Carlton, 1985). The current lack of quantitative knowledge on the impacts of biofouling within ISS leads to an underappreciation of potential direct and indirect benefits of ISS biofouling management. Direct benefits are likely to include increased operational efficiency and reliability, while indirect benefits include reducing biosecurity risks associated with species translocations (Georgiades et al., 2020).
Ships’ ISS deliver ambient sea water to a range of on-board locations via a network of pipes and pumps. This seawater delivery system is used for a variety of purposes critical to the proper functioning of ships, including engine cooling, ballasting, firefighting, freshwater production, air conditioning, and other specialty functions dependent on the ship type (Coutts et al., 2003; Gust et al., 2018b). In extreme situations, impairment of these systems can threaten the seaworthiness of vessels, endanger crew and passengers, and damage cargo. For example, damaged and blocked ISS have resulted in complete power loss (blackouts) and engine room flooding of ships with subsequent running aground or sinking (UK Marine Accident Investigation Branch, 1999, 2010, 2013; Transportation Safety Board of Canada, 2014; United States Coast Guard [USCG], 2018). As such, ISS and associated equipment are considered critical safety elements (International Maritime Organization [IMO], 1993) because their impairment or abrupt failure creates hazardous conditions or can prevent the operation of other critical systems.
Impairment of ISS occurs when the system or its components are compromised due to blockages, equipment failure, or operational failure. Blockages can occur in the form of abrupt occlusion due to ice, marine life (e.g., jellyfish and krill), marine debris (e.g., plastic bags) or from the gradual accumulation of sediments within ISS components. Equipment failure typically occurs because of electrical or mechanical impairment, corrosion, or a combination of these (Edyvean, 2010). Because raw sea water enters ISS, the internal surfaces of ISS are also subjected to constant biofouling pressure which can itself lead to ISS impairment or exacerbate other sources of impairment (e.g., blockages and corrosion) (Jones and Little, 1990a; Grandison et al., 2011).
Even though ISS perform a range of critical functions on board commercial ships, difficulties in accessing these systems is a widely acknowledged limitation that contributes to the lack of studies of biofouling accumulation and associated impacts, costs, and inefficiencies (Scianni and Georgiades, 2019). The purpose of this review was to:
• Briefly describe ISS of ships;
• Synthesize reports of biofouling accumulation within these systems;
• Assess the relationship between biofouling and ISS structural and operational performance;
• Summarize maintenance procedures to mitigate and manage biofouling impairment of these systems and;
• Examine information or data on the benefits and costs of ISS maintenance on commercial ships.
The Purpose, Configuration, and Function of ISS
Sea water has been used in large quantities on board ships since the early 20th century when steel ships replaced wooden ones, combustion engines became the dominant propulsion for ocean-going vessels, and sea water became a dominant source of ballast (Carlton, 1985; Stopford, 2009). In addition to being plentiful, cheap, and easily accessible, sea water has a range of beneficial properties that can be exploited for shipping purposes, including thermal conductivity, density, fire-quenching, and a source for freshwater generation. A key ISS function requiring continual waterflow is the removal of heat from engine equipment or conversion of gases to liquids in condensers. Sea water absorbs heat from engine systems and its abundance means the heat can be diffused and discharged rather than recirculated or subjected to treatment. ISS also deliver sea water on board to provide ballast, in contrast to earlier times when the more laborious process of solid ballasting dominated (using sand, rocks, discarded port debris; Carlton, 1992). Sea water in ballast tanks provides trim and balance that ensures correct buoyancy, submerged running gears, and optimal ship maneuverability (David, 2015). The main firefighting capacity and sprinkler systems on board ships are also supplied with sea water, as are general service outlets that use sea water intermittently for cleaning and deck wash (Gust et al., 2018b). Ships extract their freshwater supplies using desalination plants, and air conditioning and refrigeration systems require sea water as a coolant in condensers. More recently, sea water is used as part of exhaust scrubber systems that remove greenhouse gases from ships’ emissions (Andreasen and Mayer, 2007). There are many other uses that pertain to various vessel types, often involving cooling for mechanical equipment (e.g., auxiliary engines, thrusters, and emergency generators), or seawater supply for distillers, deluge pumps, cleaning equipment, and toilet systems (Gust et al., 2018b).
The configuration, construction, and scale of ships’ ISS vary greatly among vessel types, with most ISS being tailored installations that broadly adhere to classification society requirements for material type, minimum critical dimensions, and labeling (e.g., DNV GL, 2018; Gust et al., 2018b; Cahill and Floerl, 2019). Accounting for the inherent diversity of ISS is essential to effectively understand and manage ISS biofouling, but common features of ISS can illustrate the nature and extent of systems and provide context for biofouling within them (Figure 1).
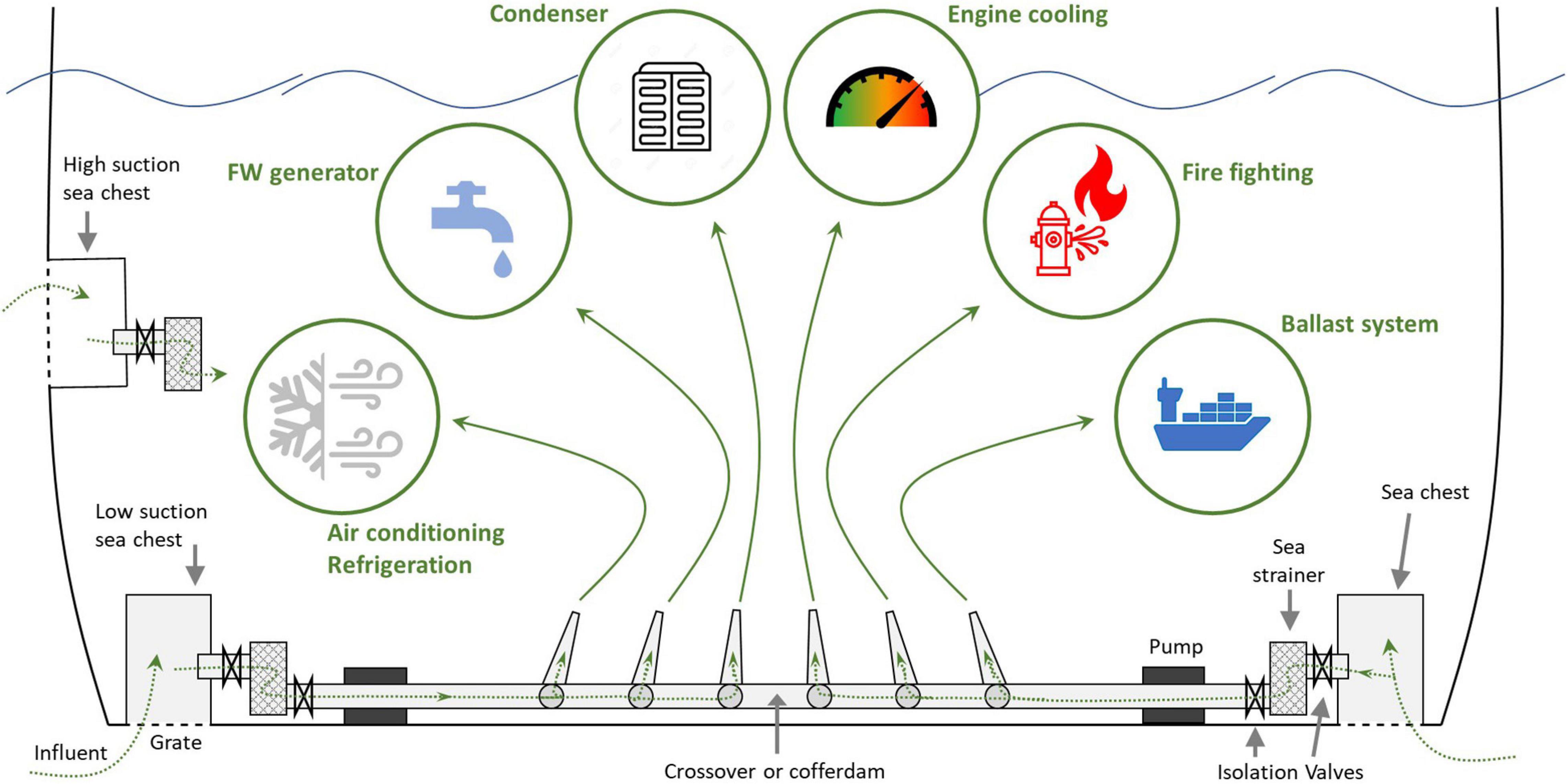
Figure 1. Schematic of initial sea water passage through an integrated ISS, depicting the range of uses for sea water on board a ship. Diagram based on Coutts and Dodgshun (2007); Kongsberg (2014); and Gust et al. (2018b). FW refers to fresh water.
The Flow of Sea Water Through ISS
A sea chest is a recessed compartment (≈ 1–3 m3 on large commercial ships) within the hull through which sea water is drawn and delivered to ISS pipework and equipment by pumps. Grates at sea chest entrances prevent coarse material from entering ISS (Dodgshun and Coutts, 2002). The sea chest acts as a reservoir or “halfway house” for sea water, straddling the space between the 12 to 20-knot laminar flow adjacent to a ship’s external hull and the suction side of intake pipes. The chest prevents countervailing forces that would cause water cavitation that reduces or disrupts pumping efficiency of incoming water while also increasing propulsion drag for the ship (Coutts et al., 2003). The size and number of sea chests varies among commercial ships, with larger ships typically having larger and more numerous sea chests (Frey et al., 2014; Davidson et al., 2018). Naval ships, cruise ships, and some other specialty vessels may not adhere to this generalization as they have configurations and operational tempos that differ substantially from cargo vessels (Moser et al., 2017; Polglaze, 2019). Sea chest redundancy is commonplace, with “high” and “low” sea chests referring to their position on the hull: low sea chests typically draw water at or near the flat bottom of the hull and high sea chests are usually located on the vertical side of a ship’s hull. Ship operators switch intake suction between sea chests based on water depths and available clearance to the seafloor, meaning high sea chests are often used in ports to reduce the likelihood of sediment entrainment while low sea chests are used when the ship is underway or in deeper anchorage (Coutts and Dodgshun, 2007).
From the sea chest, water moves into an intake pipe, bypassing an open valve before reaching an internal sea strainer. This is the second filter through which incoming water passes and is often a removable basket- or bucket-shaped metal filter that sits in a housing. After the strainer, sea water passes another open valve and through the main pump. Sea chest grates (≈ 15–35 mm gaps) and sea strainers (≈ 5–20 mm holes) prevent larger unwanted material from reaching, damaging, or clogging this pump (Coutts et al., 2003; Coutts and Dodgshun, 2007). Pumps are often centrifugal or single-entry impeller pumps (Gust et al., 2018b) that accelerate water through a chamber or impeller casing and onward from the pumps’ discharge piping. For ISS servicing multiple on-board functions (integrated ISS), sea water moves from the pump into a crossover pipe or cofferdam, both of which act as a common seawater reservoir for use in ISS throughout the ship. Crossover pipes or cofferdams often span the width of a ship, connecting the sea chests on both sides. For ISS servicing a single function (independent ISS), sea water travels directly from a sea chest to perform a function without a shared intake pipe or crossovers.
After passing through suction pumps, sea water is used to perform various functions, of which heat exchange for engine equipment is a primary use by overall volume. Engine cooling systems are found on almost all motorized vessels (the terms heat exchangers and coolers are used interchangeably in the maritime industry). In integrated ISS, sea water typically travels from the crossover pipes and often through secondary pumps and filters into an ever-decreasing range of pipe diameters. When it reaches plate or shell-and-tube heat exchangers, sea water is confined inside a chamber that is separate from the hot fresh water (or coolant) from which heat is being transferred. Sea water entering plate-type heat exchangers can occupy 1.5 mm-thin spaces within a chamber separated by titanium or stainless-steel plates through which heat is advected from fresh water and diffused by sea water departing the chamber (Gust et al., 2018b). Modern plate designs include varying degrees of herringbone patterns on plate surfaces (theta chevron angles) which can increase water velocity and turbulence in the narrow gaps between plates to increase heat exchange efficiency while reducing fouling risk (Ahn et al., 2019). Likewise, shell-and-tube coolers can have pipe diameters of <1 cm with heat from fresh water in the chamber (shell) being transferred and removed by sea water flowing through a bundle of pipes (tubes) (Gust et al., 2018b). The sea water traversing this equipment can experience dramatic shifts in temperature, up to 15°C, in a short time span (US Environmental Protection Agency, 1999). After passing through heat exchangers, sea water can move directly through overboard pipes that typically take the shortest distance for discharge at the exterior hull.
Sea water cooling systems are designed to be as short and direct as possible due to acknowledged issues of ISS biofouling and the expense of corrosion-resistant materials. Sea water is pumped by the main sea water pumps through the main heat exchanger(s) where low temperature (LT) fresh water is kept at a constant temperature (typically 32–34°C). LT fresh water is then pumped by LT pumps to equipment that needs cooling. This decoupling from sea water makes the temperature control of secondary systems easier as it avoids or reduces problems caused by (1) biofouling of internal surfaces; and (2) the influence of seawater temperature fluctuations.
Similar heat transfer principles are employed for sea water that traverses through refrigeration and air-conditioning systems. In these systems, sea water is used within condensers, typically turning a chemical refrigerant from a gaseous to a liquid state (Eames et al., 1992). Sea water also flows from crossovers or independent ISS to seawater supply systems, including ballast tanks, freshwater makers, and firefighting systems (Gust et al., 2018a). Unlike sea water that travels through heat exchangers, these systems can be used intermittently and result in sea water that is stationary for varying periods. Ballast tanks represent one of the few situations where sea water travels from relatively constricted spaces into large tanks. Sea water traveling through freshwater makers is subjected to evaporators and condensers, or passes through membranes in reverse osmosis systems, to convert sea water into a ship’s freshwater supply, with salty brine discharged overboard (Gust et al., 2018b). Ultimately, most sea water entrained in ISS travels through various routes and equipment until discharge to the sea from overboard pipes (US Environmental Protection Agency, 1999).
Overall, ISS are complex systems whereby sea water passes grates, chambers, pipes, fittings, valves, strainers, pumps, seals, gaskets, filters, plates, tubes, tanks, and/or membranes. These components can be made of (or contain) a huge diversity of material types, including, but not limited to carbon steel, stainless steel, brass, bronze, aluminum, copper-nickel, titanium, rubber, neoprene, epoxy, nylon, polypropylene, plastic, silicone, coatings, and anodes (Gust et al., 2018b; Cahill and Floerl, 2019). Sea water flowing through these systems can contain traces of copper, iron, aluminum, zinc, nickel, tin, titanium, arsenic, manganese, chromium, lead, oil, and grease (US Environmental Protection Agency, 2013). Sea water can undergo a range of temperature fluctuations, including discharges of 60°C (US Environmental Protection Agency, 1999) and conversion to steam. Up-taken sea water can traverse ISS spaces of varying sizes, ranging from millimeters wide (in heat exchangers) to large tanks several meters high, wide, or long (Krata et al., 2012; David, 2015). Water residence times vary from minutes for continuous flow-through cooling systems (US Environmental Protection Agency, 1999) to days or weeks for ballast systems (Verling et al., 2005).
Functional Components of ISS
Ship Engine Cooling
Internal combustion engines generate heat sufficient to melt engine materials or set fire to lubricants and equipment (Ezgi et al., 2014). Using sea water in engine cooling systems makes it possible to have practically sized heat transfer equipment on ships within a “single pass” open system that transfers the heat through seawater (typically via a heat exchanger rather than direct raw water cooling) that is released overboard or redirects this energy to other purposes (Balaji and Yaakob, 2012; Kongsberg, 2014; Garcia and Trueba, 2019). Efficient heat exchange prevents catastrophic engine failure and reduces the rate of fuel consumption that helps maintain power. This critical function explains the need for continuous seawater flow and the large volumes required to maintain these systems. For example, an aircraft carrier can process 170,000 gallons (644 m3) of sea water per minute when underway (US Environmental Protection Agency, 2013).
There are two main types of heat exchangers to service engine cooling: plate coolers and shell-and-tube (or pipe) coolers. Both types work to transfer heat from hot fresh water within a closed-loop engine cooling system to the colder sea water traversing the cooling chamber within an open seawater system. In addition to onboard heat exchangers, ships can have box (or keel) coolers which are essentially recessed compartments (similar to sea chests) on the external hull. Closed-loop freshwater piping is housed in the “box” and is cooled by ambient sea water before following the pipelines back inside the hull. These compartments are not accessible from inside the ship and while they are typically coated with antifouling or foul-release coatings to reduce biofouling accumulation, the pipework for heat exchange often remain uncoated. Box coolers are rare in cargo ships but relatively common in certain types of tugs, fishing vessels, and other small vessel types (Gust et al., 2018a).
Ballast
Floodable cargo holds are used as ballast tanks on some commercial ships (e.g., bulk carriers) but dedicated ballast tanks between the hull and internal superstructure (i.e., double bottoms, wing- and deep tanks) on all ships can be numerous, widely distributed, and contain complex internal configurations (Hewitt et al., 2009; Krata et al., 2012). Longitudinal and transversal structures, baffles, ledges, stairs, struts, and platforms contribute to convoluted flow patterns during flooding and emptying, including localized low-flow or dead zones (Hewitt et al., 2009; Guney et al., 2020). Tank sizes and ballast operations vary among ship types (Verling et al., 2005), but cumulative surface areas of internal tank walls and interconnecting pipes are typically thousands of square meters per ship. Ballast pumps are often some of the largest within ship ISS and must cater for potentially vast amounts of water movement over relatively short docking timeframes (David, 2015). For example, single deballasting events of 103,000 m3 have been reported (Minton et al., 2005). Pumps are also activated during open-ocean exchanges when ballast water from ports is removed and oceanic water is entrained in its place for biosecurity purposes (International Maritime Organization [IMO], 2004; Hewitt et al., 2009). More recently, ballast treatment systems have been installed on ships which are intended to control the numbers of organisms in ballast tank water (Davidson et al., 2017). The waste heat from engines can be redirected via ISS to treat ballast water (e.g., Bawat A/S, 2021).
Firefighting
Firefighting systems on ships are connected to crossover pipes or independent ISS (usually both) to supply sea water to main firefighting installations (emergency mains with hose connections), sprinkler systems, and water spray systems (Gust et al., 2018b). The capacity and configuration of the firefighting system is highly regulated, including placement and numbers of pumps, hydrants, and hoses. Hoses and couplings are internationally compatible systems, but regulations vary by ship type and potential fire hazards (i.e., cargo and operational considerations). Valves of these systems can only be closed for maintenance or icing risk, and corrosion of steel piping is typically monitored. Because water for these systems is not in continuous demand, standing sea water can lead to unacceptable corrosion problems. For this reason, sprinkler systems that are supplied by ISS typically sit filled with fresh water via a header tank (Murdoch, 2012). When in use, sea water is used after the fresh water is discharged and the sub-system is backfilled with fresh water after use. Ultimately, the firefighting system is reliant on functioning ISS pumps and pipes.
Freshwater Makers
Desalination plants on board ships provide on-demand fresh water. There are two main types of desalination plant supplied with raw sea water from ISS: distillation generators and reverse osmosis systems. For distillation generators, sea water passes through an evaporator and the subsequent steam through a condenser to produce fresh water. Distillation water makers operate in a vacuum to enable evaporation at 40–45°C and use waste heat energy from the engine’s cylinder cooling water as a heating source. Reverse osmosis systems apply pressure to the seawater side of a membrane-separated chamber to produce fresh water on the other side. The latter systems can have seawater heaters upstream of the unit to improve efficiency for ships operating in cold waters. Both systems can be affected by biofouling and entrained biota at filters just upstream of the systems.
Air Conditioning and Refrigeration
Air conditioning and refrigeration systems operate similarly to engine cooling systems. A chemical refrigerant (e.g., freon and ammonia) is converted to a gas in an evaporator and then compressed and returned to liquid form by a fresh water or an ISS-fed condenser (Eames et al., 1992; Gust et al., 2018b). The sea water running through the condenser cools the gas and is discharged overboard. These systems cater to crew needs on many ships (e.g., comfort and food storage) and are essential to maintain safe and comfortable working conditions in engine rooms (and living areas). Larger versions of air conditioning and refrigeration plants can be required on cruise ships and some fishing vessels to cater to larger numbers of people or maintain low temperature to prevent catch spoilage (Hafner et al., 2018).
Other Systems
Internal seawater systems are also used for steam condensers, general service (i.e., deck wash and toilets), lubrication oil and fuel oil cooling, cargo pump turbines, some specialty functions on certain ship types, and exhaust scrubber systems (Andreasen and Mayer, 2007; Kongsberg, 2014). Exhaust scrubber systems are a relatively recent innovation used to remove nitrogen oxides (NOx), sulfur oxides (SOx), and particulate matter from ships’ exhaust emissions to adhere with recent regulations from the International Maritime Organization (Ji, 2020; Teuchies et al., 2020). In these systems, the natural alkalinity of sea water is used to remove SO2, for example, from the exhaust plume, producing an increase in the amount of sulfate in discharged sea water (Andreasen and Mayer, 2007). Systems involving sea water generally push exhaust gas through a chamber in which sea water is sprayed to remove SO2. Cleaner exhaust gas is emitted, while the scrubbing effluent (liquid) is further treated or discharged overboard (Issa et al., 2019). Open-loop scrubber systems are considered effective and dominate the market but require large pumping capacities to maintain high amounts of water passing through the system (Teuchies et al., 2020). However, the amount and possible impacts of contaminants in discharged effluent are being further scrutinized (Teuchies et al., 2020) and a growing number of jurisdictions have restricted or banned scrubber effluent discharge in their waters (Britannia P&I, 2020).
Biofouling in ISS
Biofouling throughout ISS can impair the flow of water, the integrity of the system or its component equipment, and the function of those component systems (Houghton and Gage, 1979; Jones and Little, 1990a; Coutts and Dodgshun, 2007; Gust et al., 2018a).
Biofouling Accumulation in ISS
The internal surfaces of ISS are subjected to biofouling pressure because raw sea water, in particular sea water associated with coastal marine (and port) environments, carries microorganisms and larval stages of a broad range of marine macroorganisms from the surrounding environment (Carlton, 1985; Frey et al., 2014). While the range of conditions biofouling organisms could be exposed to within ISS is very broad, localized conditions at a particular point of settlement are likely to be comparatively stable. For example, a given settlement site in pipework upstream of an engine-cooling heat exchanger will be subject to a continuous flow of ambient sea water, even though seawater temperatures along the entire engine cooling ISS will range from ambient up to 60°C (US Environmental Protection Agency, 1999). As such, biofouling organisms may experience quite stable conditions, and there may be scenarios where conditions are more favorable and stable within ISS than on external hull surfaces and niche areas (e.g., because it is a “protected” space with a constant source of sea water for food and respiration, or if heat exchangers yield more suitable temperatures for given biofouling organisms).
In general, there is likely an inverse relationship between biofouling accumulation within ISS components and distance from suction sea chests. That is, biofouling accumulation probably decreases through the system from sea chests to overboard pipes because of water filtration and processing through various equipment, but this has yet to be explicitly tested. After passing through the sea chest, settlement sites within ISS tend not to have surface coatings to prevent colonization (Cahill and Floerl, 2019) but some pipework and other ISS components (e.g., impeller housings and valve bodies) are made of copper-nickel (cupronickel) alloys. Cupronickel alloys are increasingly widespread in critical ISS components, primarily due to their excellent resistance to seawater corrosion (Powell and Michels, 2000). Most cupronickel grades also confer antifouling activity against both macrofouling and microfouling akin to copper-based antifouling coatings or elemental copper (Schutz and Scaturro, 1991). Wet and dry periods within certain components likely reduce macrofouling amount and viability (e.g., ballast tank walls), and the absence of light in ISS, which does not penetrate these systems far beyond a sea chest grate, prevents the occurrence of marine algae (i.e., seaweeds).
The occurrence of biofouling within ships’ pipework was acknowledged some time ago (Newman, 1963; Carlton, 1985), however, it remains poorly understood relative to more easily accessible external biofouling (Visscher, 1928; Woods Hole Oceanographic Institution, 1952; Davidson et al., 2016). Despite this limitation, ISS biofouling and the ways in which microfouling and macrofouling can impinge on the functioning and integrity of ISS components has been a focus of some naval and applied research (Edyvean, 2010). Houghton and Gage (1979) characterized the role of biologists in applied shipping fleet research as resolving “problems which are peculiar to ships and their operation” which could be classified as “exterior to the vessel” and “internal to the structure.” The impacts were understood to be both critical to ship structure and functioning but also more subtle, cryptic, or gradual impacts that reduce overall efficiency. Hull biofouling and the impact of microorganisms (including biofilms) on fuel and hydraulic fluids and their tanks were serious concerns, but biofouling in ISS was also considered important. Houghton and Gage (1979) reported macrofouling—primarily mussels, barnacles, tubeworms, hydroids, and ascidians—variously occurring extensively in end-boxes of heat exchange elements, reducing the bore of pipes (internal space) within and adjacent to these systems, inducing “impingement attack” on heat exchanger tubes with subsequent leakage, and filter blockage leading to greatly reduced flow rates.
Likewise, Alberte et al. (1992) described the overriding approach for decades of biofouling research at the United States Office of Naval Research (ONR) covering bioadhesion, biofilms, biofouling succession, and antifouling coatings. Most of the attention was directed to external hull surfaces based on fleet readiness to reach design speeds, increases in acoustic noise, and estimates of biofouling management costs that increased from $200 million USD to $360 million USD per annum between 1974 and 1981. Their estimated cost-benefit of 10–60% fuel savings exceeded the remediation costs but was only based on external hull fouling. ISS were mentioned in terms of needing research and fouling control strategies that catered for sea chests and cooling water systems, but similar scoping of biofouling in these systems or its impact was not reported.
A study by Gust et al. (2018a) collated observations from 170 inspections of 126 vessels predominately engaged in the maritime extraction industry in Australia. While these vessels may not reflect broader trends for in-service commercial (cargo) ships, the report described seawater systems and nodes susceptible to biofouling accumulation, with significant (>5%) levels of biofouling associated with 80% of ISS inspected. The literature also provides some information, which is largely qualitative, about the occurrence of biofouling in ISS for individual ships.
Sea Chests
Sea chests and their grates are a relatively frequently reported contributor to ship biofouling communities (e.g., Coutts and Dodgshun, 2007; Inglis et al., 2010; Frey et al., 2014). Sea chests have been described as ideal environments for biofouling, biofilms, and mobile marine species because they are protected spaces with supplies of nutrients and clean sea water, but without strong current flows that could cause dislodgement (Jones and Little, 1990a). Antifouling and foul-release coating efficacy is also challenged by these conditions, notably via baffles, curves, and corners that can create low water flow “dead zones” on sea chest walls (Leary et al., 2016; Georgiades et al., 2018). Several studies that evaluated biodiversity or abundance of marine organisms in sea chests have found a broad array of taxa that are not often found elsewhere in ship biofouling assemblages (Coutts et al., 2003; Coutts and Dodgshun, 2007; Frey et al., 2014; Lewis, 2016). Naval vessels with long port residence times accumulated significant and problematic levels of biofouling in sea chests, primarily mussels, hydroids, and serpulids (Jones and Little, 1990a). Several studies have provided inventories of species from sampled ships’ sea chests, including 150 taxa from 53 sea chests examined in New Zealand, with a range of 1–33 species per sea chest (Coutts and Dodgshun, 2007). A single ship sampled in Sydney had 11 species with large clumps of biofouling at intake pipe entrances (Coutts et al., 2003). Frey et al. (2014) sampled 82 sea chests from 39 ships in Canada, finding 80% with biofouling encompassing 299 different species. Their analysis suggested in-service period since last cleaning and vessel voyage range (international versus domestic) influenced the abundance and richness of biofouling assemblages. More recently, Lewis (2016) reported images from dry dock surveys of sea chest grates that were entirely covered with hard-bodied macrofouling, indicating significant occlusion of the grate gaps. Gust et al. (2018a) recorded significant biofouling in over 60% of 738 sea chests surveyed in their collation of data from vessel inspections.
Sea Strainers
Sea strainers are one of the most accessible internal nodes of ISS and are situated within meters of the sea chest. Because they can be isolated, drained, and opened while the vessel is afloat, sea strainers can be routinely inspected and cleaned. This practice is not necessarily reflected in reports from the literature on biofouling occurrence in sea strainers and their housings. Anecdotal mentions of sea strainer biofouling occur in regulatory texts, but detailed records are few; for example, Jones and Little (1990a) reported heavy fouling of basket strainers of United States naval ships, with mussels a particular concern. One exception is the Gust et al. (2018a) study in which 34% of 773 sea strainer inspections had significant biofouling.
Pumps and Pipes
Crossovers and cofferdams are typically the largest pipes or seawater reservoirs (excepting ballast tanks) through which sea water continuously flows. Access to internal surfaces of pipes (for inspection or cleaning) is limited. Pipe sections adjacent to sea chests and sea strainers, but upstream of the primary suction pump, are slightly more accessible than pipework further downstream and may accumulate biofouling that reduces the bore of those pipes (Gust et al., 2018a). Jones and Little (1990a) reported biofouling near strainers at multiple nodes of an ISS of a single United States Navy ship. Gust et al. (2018a) ranked crossovers as the highest priority for treatment compared to box coolers, sea chests, downstream pipework, and sea strainers as they had 87% (n = 116) prevalence of significant biofouling. There were no reports of pump biofouling found in this review; copper-nickel impeller housings or pump casings may play a role in this.
Heat Exchangers and Cooling Systems
The impact of biofouling on cooling system efficiency drives cooling system monitoring, especially within heat exchanger components. As these systems are not easily accessible, there are relatively few studies relating to biofouling of ship cooling systems. Fouling from non-biological sources is also reported in these systems, including chemical reaction fouling, corrosion fouling, precipitate fouling, freezing fouling, and particulate fouling (Garcia and Trueba, 2019). While the source and mechanisms of fouling are important for diagnosing and resolving fouling problems, reports do not always distinguish among them.
Jones and Little (1990a) reported fouling and clogging of various oil coolers and condensate drain cooler tubes with mussels, barnacles, shells, and wood chips. These problems instigated their research on United States naval ships to better understand the locations and effects of biofouling and to determine best practices for resolving these problems fleet-wide. Likewise, biofouling by the serpulid polychaete Hydroides elegans and the hydroid Tubularia ralphi were responsible for problems in the seawater cooling systems of submarines in Sydney (Lewis and Smith, 1991). Gust et al. (2018a) recorded significant biofouling in 179 of 244 main engine cooling systems. Because this was a system-wide measurement, these results do not reflect biofouling in heat exchangers alone, but any point within a main-engine cooling system, including shared components. The broader literature provides generic reports on the issue of biofouling in cooling systems and the need to implement regular maintenance, but without reporting particular instances of biofouling accumulation. The US EPA regulations for incidental discharges from ships highlight the occurrence of biota (biofouling) and other debris that is discharged from a ship after “blowdown” (clearing) of cooling system strainer plates (US Environmental Protection Agency, 2013). This information implies that biofouling is commonplace in these systems, even though not reported in a systematic way.
Ballast Systems
Ballast tanks are large compartments in ships providing hundreds to thousands of square meters for potential biofouling colonization. Ballast tanks are typically associated with carrying organisms that live in the water column (i.e., plankton and larval stages of species) and sediments that can accumulate on ballast tank floors (Smith et al., 1999; Wonham et al., 2000). Ballast tank biota ranges in size from viruses to fish, including some specimens up to 25 cm long (Davidson pers. obs.) suggesting some uncertainty about longevity and growth for some species in ballast tanks and the size of organisms that can pass through ships’ pumps (Gollasch et al., 2002). Benthic species on floating debris have also been sampled from ballast tanks (Carlton and Geller, 1993). Ballast sediments can be 30 cm deep and account for hundreds of tons per ship (Hamer, 2002) with a distinct soft-sediment community, including cysts and spores, occupying this discrete niche (Bailey et al., 2005).
Biofouling conditions within ballast tanks have been described as “dark intertidal zone” environments with variable hydrological conditions that nonetheless support various taxa (Drake et al., 2005). Studies suggest this environment rarely supports macrofouling and only about 10% of available surfaces are colonized by biofilms (Drake et al., 2007). Nonetheless, biofilms sampled from in-service ships consist of bacteria, virus-like particles, cysts of microalgae, and have included human pathogens (Drake et al., 2005, 2007). Ballast treatment of influent water is likely to further reduce the risk of biofouling in these systems.
Firefighting Systems
Firefighting capacity on ships is highly regulated, requiring specific numbers and capacities of pumps and outlet stations to ensure redundancy and ability to quickly control fire outbreaks wherever they occur on a vessel (International Maritime Organization [IMO], 2002; Gust et al., 2018b). There are usually pumps connected to main firefighting lines (pipe systems) that can be single main systems or horizontal loop systems (US Maritime Training Advisory Board, 1994). The feed lines upstream of freshwater-filled branch lines (e.g., sprinkler systems) are constantly filled with sea water unless draining is required to prevent freezing. Branch lines connected to these main lines may only service fire hydrants, other fire systems, and deck wash facilities. Biofouling could potentially reduce the flow of water in these systems, which are subject to volume (i.e., pipe diameter) and testing pressure requirements (US Maritime Training Advisory Board, 1994). Palermo (1992) reported that firefighting systems of vessels operating in United States freshwater systems were susceptible to zebra mussel (Dreissena polymorpha) infestation, which affects compliance with safety regulations and can endanger life in emergencies. Significant biofouling was recorded in 64% of main firefighting systems and 47% of emergency firefighting systems (n = 98 and 122, respectively) inspected by Gust et al. (2018a).
Freshwater Makers
Freshwater makers that use reverse osmosis or distillation (evaporator/condenser) are unlikely to support biofouling organisms within the units themselves, but the piping and filters directly upstream of these units are likely to be sensitive to biofouling and biotic accumulation that restricts flow and affects water quality entering the units. In this review of literature, the only report of biofouling associated with freshwater makers was from Gust et al. (2018a), in which biofouling was recorded in 62% of 37 freshwater generating systems evaluated (systems included pipework and filters).
Overboard Piping
Biofouling in overboard pipes is largely unreported, except for points of discharge at the outer hull, which is a more heterogeneous surface than adjacent vertical hull surfaces. These point locations can be colonized by biofouling at the mouth of the pipe. This biofouling is part of the external biofouling community on a relatively minor external niche area rather than biofouling derived from water passing through ISS. Biofouling in this location can reduce the bore of these relatively small diameter pipes at their outlets (e.g., 5 cm), but ISS effluent blockage has not been reported in the literature.
Impact of Biofouling on ISS Components and Function
Biofouling can affect surfaces, disrupt water flow, and compromise the structural integrity of ships’ ISS. There are several mechanisms by which these issues can occur (Table 1), and these can work in concert to exacerbate the scale or speed of impacts.
Surface Roughness
It is widely accepted that all seawater-immersed ship surfaces will be affected by biofouling (e.g., for outer hull surfaces, Schultz et al., 2011), with a continuous impact of performance deterioration below design specifications (i.e., idealized conditions). For ISS, the simple presence of biofouling changes how water flows over surfaces. Failures in pipework and components can occur because of seawater turbulence, flow impingement, and cavitation at elbows, joints, valves, pumps, and other orifices (Schutz and Scaturro, 1991). Schutz and Scaturro (1991) described such impacts on newly commissioned military vessels as “blatant examples” of accelerated failure of cupronickel pipework with less than one year of service (in this instance, the cause of turbulence was not explicitly tied to biofouling). The remedy—to increase the diameter size of pipework—had the knock-on effect of significantly increasing system cost and weight while not eliminating the flow-erosion-corrosion problem. An intended solution to replace the inner surfaces of pipes with titanium was thought to reduce pipe sizes, improve flow rates, reduce costs, and minimize surface biofilms, biofouling, and sedimentation.
Flow
A key impact of biofouling in ships’ ISS is reduced water flow (Table 1). In general, smaller diameter pipework is more prone to clogging and will see proportionally greater restrictions in flow for a given thickness of biofouling compared to larger diameter pipework. In some cases, restricted flow resulting from biofouling is considered at the design stage of a seawater system component. For example, engineers of heat exchangers consider an allowable fouling resistance in their design calculations to ensure some leeway before maintenance is required (Ezgi and Ozbalta, 2012). Similarly, water flow within certain pipes should not exceed specified flow rates, based on pipe composition and diameter (Croatian Register of Shipping, 2013). This can happen if the bore of pipes is reduced by biofouling. Nonetheless, it appears most pipework and components within ISS are designed and manufactured to deliver a specified capacity, largely discounting biofouling impairment, as is the case with hull design and static water-use industries (Schultz et al., 2011; Polman et al., 2013).
Sea chests and their grates are prone to dense aggregations of biofouling that cover entire grates and the entry ways to pipes, significantly impeding flow (Lewis, 2016). However, reports of biofouling acting as a complete barrier to water movement within these components were not found. Severe occlusions in these systems almost certainly require much higher work rates for pumps, increasing the overall workload of an affected ship’s equipment and energy usage (Polman et al., 2013). Biofouling also increases the risk of full occlusion by other means (e.g., trash or marine life) because a partial blockage (by barnacles, mussels, hydroids, or other organism types) is already in place.
Restricted flow has been anecdotally reported by several authors (see Grandison et al., 2011). Houghton and Gage (1979) observed mussels, tubeworms, and barnacles fouling heat exchangers, reducing flow, and reducing the bore of pipes upstream from those units. They also reported ships’ strainer and filter occlusion by ascidians. Heat exchangers are sensitive to restricted flow and can cause serious problems for engine output. Ezgi et al. (2014) examined the performance of a ship’s shell-and-tube heat exchanger immediately after biofouling was cleaned from the unit and after 2500 subsequent working hours. They recorded significant negative effects on water pressure in the system, temperature of cooling fluids, and overall system effectiveness over time. These thermohydraulic effects can accumulate substantial costs over the service duration of a cooling system and should be considered when planning proactive and ongoing maintenance schedules.
While restricted flow is a key parameter that can affect seawater systems and components, biofouling is typically a chronic issue because accumulation and growth of larger organisms on surfaces occurs over time (i.e., days, weeks, and months; Greene and Schoener, 1982; Davidson et al., 2020). Acute occlusion of systems, including clogging of strainers, filters, and pumps occurs with sudden blockage. In the worst-case scenario, acute occlusion can result in total power loss and can have catastrophic impacts on ship and crew safety. Such scenarios are most often attributed to icy conditions, trash, and free-swimming marine life (e.g., fish, krill, and jellyfish; Transportation Safety Board of Canada, 2014; United States Coast Guard [USCG], 2018), but acute flow restriction can occur from biofouling when mass mortality causes the release of organisms from surfaces that subsequently clog and completely occlude strainers or filters. Such catastrophic blockages occur in other seawater-use industries (e.g., hydroids in Chesapeake Bay are known to clog power plant intakes; Tamburri, pers. comm.) and is possible on ships, particularly after reactive treatment of biofouling (Jones and Little, 1990a; Cahill et al., 2019). Transition from sea water to a freshwater area (e.g., Panama Canal or river passages) can kill marine life that then accumulates in strainers or can block narrow ISS passages (Hinz, pers. obs.).
Corrosion
Sea water is well known to accelerate corrosion, and biofouling (both micro- and macro- fouling) has been shown to initiate and enhance the aggressiveness of corrosive attack on pipework and within ISS components (Edyvean, 2010). The contribution of biofilms to this problem is well described in the literature (e.g., Jones and Little, 1990a,b; Machuca, 2019). Pipe erosion occurs when very localized changes in fluid velocity occur. This can be triggered by biofouling organisms when their mechanism of attachment or adhesion undermines the surface boundary layer (US Environmental Protection Agency, 1999). Sea strainers have been observed to be highly corroded under heavy macrofouling, requiring strainer replacement (Jones and Little, 1990a). Similarly, heat exchangers for refrigeration units, main engine cooling, and other system coolers and condensers have been undermined by combinations of macrofouling affecting flow distribution and aggressive microbiologically induced corrosion (Jones and Little, 1990b; Eames et al., 1992). These effects can be relatively rapid (within weeks) and serious corrosion problems require expensive unscheduled maintenance (Grandison et al., 2011) or cause flooding if left unattended (UK Marine Accident Investigation Branch, 2013).
Microbiologically influenced corrosion (MIC) is corrosion of metallic surfaces and structures that is initiated or enhanced by microorganisms (Machuca, 2019). MIC was responsible for a trans-Alaskan pipeline failure in 2006 that caused the leak of 200,000 gallons of crude oil, a major loss of production, and an impact on global oil prices (Machuca, 2019). MIC contributes to more than 20% of total corrosion loss worldwide, including 40% of internal corrosion of underground pipelines, and its impact is so great that it is measured at the scale of lost proportions of national and global Gross Domestic Product (Hashemi et al., 2018; Li and Ning, 2019).
It is long known that biofilms can accelerate corrosion by factors of 1,000 to 100,000 (Costello, 1969) and severely compromise pressurized water systems. Examples of heat exchanger and ISS failure due to MIC have been reported. Eames et al. (1992) described MIC-damaged seawater-cooled refrigerator condensers used for air conditioning, resulting in complete failure within months of installation. This case highlights the rapid effects of bacterial enhanced corrosion of tubes, resulting in end plates damaged by a mixture of refrigerant and leaked sea water. Considerable deposits of sediment and debris combined with biofilm activity to undermine the unit and cause it to fail. Jones and Little (1990a,b) reported multiple nodes of “under-deposit” corrosion and MIC. As such, the effects of MIC promote serious and sustained problems for ships, including damage to cargo, fuel systems, and ISS, including total failure of propulsion systems (Edyvean, 2010). Furthermore, inadequate maintenance or last-minute design alterations within ISS can exacerbate MIC attack, which can lead to stress corrosion cracking or contribute to corrosion fatigue. The outcome may be substantial repair work to a sub-section of a pipe or catastrophic failure along the entire pipe length (Edyvean, 2010).
Function
In addition to impaired water flow and structural integrity issues, biofouling can alter functional aspects of ISS surfaces (Table 1). Functional impacts mainly pertain to heat exchangers, where surface fouling or deposits on heat exchange plates or tubes can lower the thermal conductivity of surfaces and thus greatly reduce efficiency of heat passed from high to low temperature water (Faes et al., 2019; Kah Hou et al., 2019). Biofouling can provide substantial thermal resistance, with biofilms of 1-mm thickness reported to reduce heat transfer efficiency by 50%, which exceeds the impact of most types of mineral fouling (Muilenberg and Candir, 2013).
Contamination
Pathogens occurring in ISS can affect the health of vessel crews and passengers, and ISS can act as dispersal vectors for local and global spread of human and aquatic animal pathogens. While such concerns are usually associated with ballast water organisms (McCarthy and Khambaty, 1994; Pagenkopp Lohan et al., 2017) or external hull biofouling (Howard, 1994; Whittington et al., 2018; Pagenkopp Lohan et al., 2020), pathogens have been sampled from biofilms inside ships (Drake et al., 2007). Hosts of disease agents that are of concern for external biofouling could also occur in ISS biofouling (e.g., Mytilus spp., Crassostrea gigas, Carcinus maenas, Styela clava; Coutts et al., 2003; Coutts and Dodgshun, 2007; Georgiades et al., 2021). Outbreaks of human diseases linked to water systems on board cruise ships, such as Vibrio parahaemolyticus and Legionella pneumophila, have had serious implications for those affected (Lawrence et al., 1979; Pastoris et al., 1999). In one case, a direct link to seawater systems was made because of seafood preparation using sea water from ISS, a practice that was discontinued following this discovery (Lawrence et al., 1979).
Operations
The range of biofouling impact mechanisms and their outcomes for ISS are often overshadowed by concerns for external hull biofouling, but they are significant nonetheless (Table 2). Biofouling can exert a penalty on the efficient operation and structural integrity of ships’ seawater systems. These penalties range from chronic underperformance of systems or ISS components to critical losses of integrity that can threaten the safety of the entire vessel and its crew (Tables 1, 2). Considering the known impacts of biofouling on land-based industrial cooling systems (e.g., Rajagopal et al., 2012), it is likely that the relative lack of information for ISS reflects the difficulties accessing ISS to quantify biofouling and assign impacts, rather than a lack of impacts (Grandison et al., 2011; Growcott et al., 2017).
Biofouling incurs costs across the lifetime of equipment: capital expenditure, energy costs, maintenance costs, production loss, and environmental management costs (Steinhagen et al., 1993; Kah Hou et al., 2019; Table 2). Some direct costs are straightforward to quantify but indirect costs are difficult to estimate and there appears to be a lack of corresponding ship-scale models. For example, it is not clear how much initial capital outlays cater for expected biofouling pressure. For cooling systems at least, that cost is incurred in the form of larger heat-exchange units, increased space and weight in the engine room, and increased installation costs (Ezgi and Ozbalta, 2012). There are known costs to installation and upkeep of marine growth prevention systems (MGPS) (Grandison et al., 2011). Production losses may vary broadly across a range of impacts from reduced capacity or offline systems, including ship scheduling issues. Throughout the life cycle of a ship, ISS will incur energy losses, lost productivity, and maintenance and personnel expenses that each cause substantial cumulative costs (Stopford, 2009; Kah Hou et al., 2019). Losses occur as systems get older and no longer run as new, with biofouling and a range of other causes contributing to higher maintenance and running costs. Environmental management costs to operators are usually restricted to direct compliance costs, i.e., costs associated with meeting regulatory standards. Broader environmental costs are perhaps the most difficult to quantify as they are indirect and largely external to industry (vessel owners and operators). However, potential environmental costs could be large, possibly involving costly management interventions to respond to biosecurity incidents or environmental contamination breaches. There have been at least two biosecurity responses partially or fully funded by maritime liability insurance, albeit the circumstances involved lost or run-aground vessels (Wotton et al., 2004; Wanless et al., 2010).
While chronic impacts often have underlying costs that go unmeasured, there are also acute negative consequences if biofouling affects critical safety elements or otherwise violates safety standards. Worst-case scenarios result in the loss of ships or loss of life at sea. Annual reviews of maritime casualties and incidents may prove useful for identifying human health implications of equipment failure, but thus far (in the incident reports and summaries examined for this review) there is insufficient detail about the causes or circumstances of equipment failure to make a strong link to a role of biofouling or maintenance issues. For example, governmental organizations provide evidence that equipment failure and engine rooms are an important driver of marine casualties and incidents at sea (European Maritime Safety Agency [EMSA], 2016). However, they do not always provide levels of detail that pinpoint those incidents occurred because of ships’ ISS specifically, much less that biofouling may have been a contributing factor.
Biofouling Mitigation and Management Within ISS
Several reviews have been conducted on proactive and reactive management approaches for ISS biofouling (Lewis and Dimas, 2007; Grandison et al., 2011; Bracken et al., 2016; Growcott et al., 2016, 2017; Cahill and Floerl, 2019). These studies highlight the range of tools that can be installed prior to the development of ISS biofouling or as a remediation measure if problems associated with biofouling have occurred. In general, ships’ ISS are currently managed proactively using coatings (usually antifouling or foul-release paints) applied to the surfaces of sea chest grates and the sea chest itself, with questionable efficacy based on the range of hydrodynamic conditions at these locations (Lewis, 2016). Due to issues including access, compatibility, and utility, these coatings are not applied throughout the system and are usually limited to ISS components most exposed to ambient seawater conditions. Some critical ISS components are also constructed of (or lined with) fouling-resistant materials, mainly cupronickel. In addition, MGPS are installed within the sea chest or sea strainer as a means of chemically treating the influent of ISS to reduce biofouling accumulation throughout the system (Grandison et al., 2011; Lewis, 2016). The most common MGPS use sacrificial copper anodes or electro-chlorination dosing systems that release biocide into the influent stream to treat pipework and component systems (Grandison et al., 2011; Growcott et al., 2017). There are, however, very few independently verified, published, and accessible data on the efficacy of these systems (Lewis, 2016; Growcott et al., 2017). Reactive treatments involve a range of chemical additions (e.g., descalers, chlorine, bromine, acetic acid, and quaternary ammonium compounds) or physical treatments (such as manual cleaning, steam/hot water, fresh water, deoxygenation) to kill or remove biofouling in ISS (Growcott et al., 2017; Cahill and Floerl, 2019). While there are some promising approaches and existing commercially available reactive treatments, these can be prohibitively expensive, lack information on efficacy as well as safety or compatibility with ISS components, and/or may not be feasible at ship-scale (Bracken et al., 2016; Cahill and Floerl, 2019). Treatments may also inadvertently contribute to occlusion problems if reactive techniques remove biofouling from pipework surfaces and drive debris to block pumps, filters, or equipment (Grandison et al., 2011; Cahill et al., 2019).
There are few explicit accounts or data that show the cost-benefit of ISS biofouling management in the real world (Grandison et al., 2011). Ezgi et al. (2014) conducted a ship-scale measurement and modeling study and determined savings of USD $16,500 per 2,500 h of usage if their recommended cleaning schedule (every 600 h) was implemented. Similarly, Pamitran et al. (2016) modeled the effect of biofouling on a ships’ cooling system and determined a monthly cost of USD $464,000 in excessive fuel use is incurred from biofouling-caused performance loss of the main-engine heat exchanger. In terms of proactive treatments, Coutts and Dodgshun (2007) recorded a significant difference in species richness of sea chest biofouling when comparing MGPS presence versus absence (7 ± 1.1 species versus 11 ± 1.1 species). This comparison was not controlled for other factors, such as duration since last cleaning, vessel history (such as voyage routes, stationary periods, etc.), or MGPS operational factors. Best practice recommendations for preventing biofouling accumulation on intake grates and within sea chests suggest that foul-release coatings be applied to intake grates and soft biocidal antifouling coatings be applied to internal sea chest surfaces (Lewis, 2016; Georgiades et al., 2018). Lewis (2016) recorded no effect of copper anodic, chemical dosing, or sonic MGPS on sea chest biofouling. This finding aligned with a study in Canada that observed significant biofouling in sea chests with MGPS installed (Frey et al., 2014). In earlier studies, questions were raised regarding the efficacy of MGPS copper dosing in submarines (Lewis et al., 1988) and an experimental system was fitted on a submarine in Sydney that kept an ISS cooling system free of fouling over two summers (Lewis and Smith, 1991).
Management of ships’ ISS is governed directly and indirectly by various regulatory systems at international, national, and regional levels. Seawater systems fall under management requirements for operational safety reasons, for technical operation standards, or for environmental reasons related to discharges. The International Maritime Organization introduced the International Safety Management (ISM) code in 1993 to “provide an international standard for the safe management and operation of ships” (International Maritime Organization [IMO], 1993) as part of the Safety of Life at Sea (SOLAS) Convention. The code includes a requirement for companies to “identify equipment and technical systems the sudden operational failure of which may result in hazardous situations. The SMS [safety management system] should provide for specific measures aimed at promoting the reliability of such equipment or systems.” By any standard, ISS components would fit this description. Classification societies are a key oversight body within this system and provide very detailed specifications and auditing requirements on ships’ ISS (Murdoch, 2012; DNV GL, 2018). Examination and recording of biofouling do not play an explicit role within classification society procedures, however, which aligns with hull requirements and specifications. Dry docking schedules that occur based on classification society rules are an opportunity to manage ISS biofouling rather than a specific requirement, meaning that out-of-water biofouling maintenance is an added value of dry-docking schedules rather than an explicit reason for dry docking. International Standards Organization (ISO) standards exist to identify piping systems by their purpose and contents (International Standards Organisation [ISO], 2008), although these are not required across all jurisdictions and different systems can exist from place to place (Gust et al., 2018b). ISS are also regulated in national and international waters with respect to discharges from ships, which include permitting requirements to minimize incidental effluents or compliance with biosecurity regulations in various countries (International Maritime Organization [IMO], 2011; US Environmental Protection Agency, 2013; New Zealand Ministry for Primary Industries, 2014). The overarching effect of these regulations is that ISS biofouling may carry a compliance burden for ship operators whereby a benefit of ISS management is likely compliance with port state controllers and international standards.
Ship owners acknowledge the importance and potential value of maintaining low levels of biofouling on ships’ ISS. In a survey of ship owners, Strietman and Leemans (2019) reported fuel costs, regulatory compliance, and protection of seawater cooling systems as the main (self-reported) benefits of biofouling control on ships. Auditors also recognize the importance of ships’ ISS for safe and efficient vessel performance and the “hidden danger” of pipework management that is often neglected or weakly developed (Murdoch, 2012; International Association of Classification Societies [IACS], 2018). There is clearly a demand for products and techniques that reduce biofouling impairment of ISS and manage biosecurity risks associated with these systems.
Discussion and Conclusion
The occurrence and impact of biofouling in ships’ ISS is a curious case whereby the significance of the issue is widely acknowledged but is not supported by broadly available literature or supporting data from appropriate sample sizes of ships. This contradiction may be because data on ISS operations and functioning are scattered among private industry and navy accounts, industry bulletins and paywalled sources, and standards and code texts (Kah Hou et al., 2019). Most of the examples in the literature describe a phenomenon of ISS problems linked to biofouling (without biofouling data) or individual case-study examples to highlight the issue. Some exceptions are driven by accessibility of ISS components and interests in biofouling that are more environmental than operational in nature (e.g., sea chest studies; Coutts et al., 2003; Coutts and Dodgshun, 2007; Frey et al., 2014; Lewis, 2016). Further insights are also driven by an understanding of strong impacts of fouling on heat-exchangers (Ezgi et al., 2014; Pamitran et al., 2016) and a renewed consideration of the importance of MIC as a source of corrosion problems more generally (Machuca, 2019).
Limited accessibility to the internal surfaces of most ISS while vessels are afloat is undoubtedly a factor in a lack of ISS biofouling sampling, which contributes to a poor understanding of biofouling impacts on these systems. This poor understanding was noted decades ago in relation to transport of non-indigenous species with a presumption that such instances were rare because ISS were not permitted to become heavily fouled (Newman, 1963; Carlton, 1985). Since then, although few studies have been conducted, the role of certain components of ISS has been highlighted as biofouling hotspots and thus sub-vectors for species translocations, notably sea chests and easily accessible pipework (Lewis and Smith, 1991; Coutts and Dodgshun, 2007; Frey et al., 2014). Hence niche area management, including ISS, is heavily emphasized in regulations and guidance materials (International Maritime Organization [IMO], 2011; California State Lands Commission, 2017; Georgiades et al., 2018; Georgiades and Kluza, 2020).
It is not clear how much of a role biofouling plays in the design stage of many ISS components, apart from heat exchangers. Notably, hull surfaces and propeller systems are also currently designed to consider fluid dynamics and power (Carlton, 2018), but not biofouling, and thus rely on post-construction remedies to manage biofouling which reduces efficiency to below design specifications (Owen et al., 2018). Unlike external biofouling, however, quantitative ship-scale models of the impacts of biofouling on ISS do not appear to exist. Some measurement and modeling have occurred for certain components (e.g., heat exchangers), providing a possible template for much broader evaluations across modern commercial ships under a variety of environmental and operational conditions. There are also anecdotal reports of individual issues or incidents, broader understanding of drivers of problems (e.g., MIC), and larger macro-scale estimates of impact of corrosion and biofouling on other water use industries (e.g., energy production industries).
Ship-scale assessments of biofouling impacts across ISS that include costs and benefits of management approaches at relevant maritime time scales (voyage itineraries, inter-dry-docking periods, or the lifetime of a ship) are absent from the accessible literature. Possibilities for this absence include:
• The complexity of the issue;
• The issue cannot be readily observed or measured and is therefore “out of sight, out of mind;”
• The issue is overshadowed by external hull biofouling and other engineering concerns for a ship’s operations;
• A lack of effect or impact on modern ships (possibly linked to antifouling technology and a cost-benefit ratio that leans heavily toward continuing with operations until planned maintenance events);
• Third-party contractors that carry out maintenance tasks occasionally when vessels are in port and that may clean or otherwise treat ISS without leaving detailed data or records with the ship (i.e., the client understands an issue has been resolved but the information may only be retained by maritime service contractors and is not accessible);
• A shifting baseline acceptance of reduced efficiency because of inevitable biofouling effects (as occurs to varying degrees for external hull biofouling); and/or
• A lack of options for effective mitigation and management (Grandison et al., 2011; Growcott et al., 2016).
Developing knowledge at the ship scale is essential to promote understanding of biofouling within ISS, the operational impact of its occurrence, and the benefits of ISS biofouling management. Reductions in biofouling for operational purposes would contribute to reductions in international and domestic biofouling transfers with ships and associated biosecurity risks. Incorporating direct and indirect economic components to ship-scale ISS models is likely to provide compelling evidence to improve alignment between industry and environmental priorities.
Author Contributions
ID, PC, and EG conceived the idea for the manuscript which was drafted by ID. PC, AH, DK, CS, and EG contributed ideas and edits to the draft based on their areas of expertise. All authors contributed to manuscript revisions and finalized and approved the submitted version.
Funding
This study was funded by the Ministry of Primary Industries (MPI), New Zealand under MPI’s Operational Research Programme (Project 405759).
Conflict of Interest
The authors declare that the research was conducted in the absence of any commercial or financial relationships that could be construed as a potential conflict of interest.
Publisher’s Note
All claims expressed in this article are solely those of the authors and do not necessarily represent those of their affiliated organizations, or those of the publisher, the editors and the reviewers. Any product that may be evaluated in this article, or claim that may be made by its manufacturer, is not guaranteed or endorsed by the publisher.
Acknowledgments
We acknowledge earlier reviews of the manuscript and technical input of Emma Hill, Enrico Perotti, Michael Ormsby, Trecia Smith, Tracey Bates, Lizzy Green, and Oliver Floerl. We also thank two reviewers for their insights and suggestions that improved the manuscript.
References
Ahn, H. S., Kim, K. M., Lim, S. T., Lee, C. H., Han, W., Choi, H., et al. (2019). Anti-fouling performance of chevron plate heat exchanger by the surface modification. Int. J. Heat Mass Transf. 144, 118634. doi: 10.1016/j.ijheatmasstransfer.2019.118634
Alberte, R. S., Snyder, S., Zahuranec, B. J., and Whetstone, M. (1992). Biofouling research needs for the United States Navy: program history and goals. Biofouling 6, 91–95. doi: 10.1080/08927019209386214
Andreasen, A., and Mayer, S. (2007). Use of seawater scrubbing for SO2 removal from marine engine exhaust gas. Energy Fuels 21, 3274–3279. doi: 10.1021/ef700359w
Bailey, S. A., Duggan, I. C., Jenkins, P. T., and MacIsaac, H. J. (2005). Invertebrate resting stages in residual ballast sediment of transoceanic ships. Can. J. Fish. Aquat. Sci. 62, 1090–1103. doi: 10.1139/f05-024
Balaji, R., and Yaakob, O. (2012). An analysis of shipboard waste heat availability for ballast water treatment. J. Mar. Eng. Technol. 11, 15–29.
Bawat A/S. (2021). Ballast Water Management: In-Voyage Treatment Exploiting on-Board Waste Energy. Available online at: https://www.bawat.com/ship-bwms/ship-bwms/ (accessed May, 2021).
Bracken, J., Gust, N., Ross, J., and Coutts, A. (2016). An assessment of the efficacy of chemical descalers for managing non-indigenous marine species within vessel internal seawater systems and niche areas. Manage. Biol. Invasions. 7, 241–256. doi: 10.3391/mbi.2016.7.3.04
Britannia P&I. (2020). List of Jurisdictions Restricting or Banning Scrubber Wash Water Discharges. London: Tindall Riley Britannia Limited.
Cahill, P., and Floerl, O. (2019). Reactive Treatment Approaches for Biofouling in Internal Seawater Systems of Large Commercial Vessels. (Canberra: Australian Government Department of Agriculture and Water Resources), 71.
Cahill, P., Hickey, C. W., Lewis, P., Tait, L., and Floerl, O. (2019). Treatment Agents for Biofouling in Internal Pipework of Recreational Vessels. Available online at: https://www.mpi.govt.nz/dmsdocument/33606-treatment-agents-forbiofouling-in-internal-pipework-of-recreational-vessels-a-review-ofpipework-configurations-biofouling-risk-and-operational-considerations (accessed March, 2019).
California State Lands Commission (2017). Guidance Document for: Biofouling Management Regulations to Minimize the Transfer of Nonindigenous Species from Vessels Arriving at California Ports. California Code of Regulations, title 2, section 2298.1 et seq. Available online at: https://www.slc.ca.gov/wp-content/uploads/2018/10/4_8_GuidanceDoc.pdf (accessed May, 2021).
Carlton, J. (2018). Marine Propellers and Propulsion. (Oxford: Butterworth-Heinemann), 609. doi: 10.1016/B978-0-08-100366-4.00012-2
Carlton, J. T. (1985). Transoceanic and interoceanic dispersal of coastal marine organisms: the biology of ballast water. Oceanogr. Mar. Biol. Annu. Rev. 23, 313–371.
Carlton, J. T. (1992). Introduced marine and estuarine mollusks of North America: An end-of-the-20th-century perspective. J. Shellfish Res. 11, 489–505.
Carlton, J. T., and Geller, J. B. (1993). Ecological roulette: the global transport of non-indigenous marine organisms. Science 261, 78–82. doi: 10.1126/science.261.5117.78
Costello, J. A. (1969). The corrosion of metals by microorganisms: a literature survey. Int. Biodeterior. 5, 101–118.
Coutts, A. D. M., and Dodgshun, T. (2007). The nature and extent of organisms in vessel sea-chests: a protected mechanism for marine bioinvasions. Mar. Pollut. Bull. 54, 875–886. doi: 10.1016/j.marpolbul.2007.03.011
Coutts, A. D. M., Moore, K. M., and Hewitt, C. L. (2003). Ships’ sea-chests: An overlooked transfer mechanism for non-indigenous marine species? Mar. Pollut. Bull. 46, 1504–1515. doi: 10.1016/S0025-326X(03)00292-3
Croatian Register of Shipping (2013). Rules for the Classification Of ships. Part 8 – Piping. Available online at: http://www.crs.hr/Portals/0/HRB%20CRS%20pravila/2021%20pravila/Rules%20for%20the%20classification%20of%20ships,%20Pt.%208,%2001_2021.pdf?ver=2021-01-18-163336-150 (accessed May, 2020).
Dafforn, K. A., Lewis, J. A., and Johnston, E. L. (2011). Antifouling strategies: history and regulation, ecological impacts and mitigation. Mar. Pollut. Bull. 62, 453–465. doi: 10.1016/j.marpolbul.2011.01.012
David, M. (2015). “Vessels and ballast water,” in Global Maritime Transport and Ballast Water Management, eds M. David and S. Gollasch (Berlin: Springer), 13–34. doi: 10.1007/978-94-017-9367-4_2
Davidson, I., Scianni, C., Hewitt, C., Everett, R., Holm, E., Tamburri, M., et al. (2016). Mini-review: assessing the drivers of ship biofouling management – aligning industry and biosecurity goals. Biofouling 32, 411–428. doi: 10.1080/08927014.2016.1149572
Davidson, I., Scianni, C., Minton, M., and Ruiz, G. (2018). A history of ship specialization and consequences for marine invasions, management, and policy. J. Appl. Ecol. 55, 1799–1811. doi: 10.1111/1365-2664.13114
Davidson, I. C., Minton, M. S., Carney, K. J., Miller, A. W., and Ruiz, G. M. (2017). Pioneering patterns of ballast treatment in the emerging era of marine vector management. Mar. Policy 78, 158–162. doi: 10.1016/j.marpol.2017.01.021
Davidson, I. C., Smith, G., Ashton, G. V., Ruiz, G. M., and Scianni, C. (2020). An experimental test of stationary lay-up periods and simulated transit on biofouling accumulation and transfer on ships. Biofouling 36, 455–466. doi: 10.1080/08927014.2020.1769612
DNV GL (2018). Rules for Classification, Ships. Part 4 Systems and Components, Chapter 6: Piping Systems. Available online at: https://rules.dnv.com/docs/pdf/DNV/ru-ship/2017-01/DNVGL-RU-SHIP-Pt4Ch6.pdf (accessed May, 2020).
Dodgshun, T., and Coutts, A. (2002). Ships’ Sea Chests: A “Side Door” for Marine Pests? (Nelson: Cawthron Institute), 9.
Drake, L. A., Doblin, M. A., and Dobbs, F. C. (2007). Potential microbial bioinvasions via ships’ ballast water, sediment, and biofilm. Mar. Pollut. Bull. 55, 333–341. doi: 10.1016/j.marpolbul.2006.11.007
Drake, L. A., Meyer, A. E., Forsberg, R. L., Baier, R. E., Doblin, M. A., Heinemann, S., et al. (2005). Potential invasion of microorganisms and pathogens via ‘interior hull fouling’: biofilms inside ballast-water tanks. Biol. Invasions 7, 969–982. doi: 10.1007/s10530-004-3001-8
Eames, I. W., Edyvean, R., and Brook, R. (1992). Corrosion of refrigerator condensers cooled with estuarine water. Build. Serv. Eng. Res. Technol. 13, 101–105. doi: 10.1177/014362449201300207
Edyvean, R. (2010). “Consequences of fouling on shipping,” in Biofouling, eds S. Durr and J. C. Thomason (Oxford: Wiley-Blackwell), 217–225. doi: 10.1002/9781444315462.ch15
European Maritime Safety Agency [EMSA] (2016). Annual Overview of Marine Casualties and Incidents 2016. (Lisbon: European Maritime Safety Agency), 119.
Ezgi, C., and Ozbalta, N. (2012). Optimization of heat exchanger cleaning cycle on a ship. J. Naval Sci. Eng. 8, 33–46.
Ezgi, C., Ozbalta, N., and Girgin, I. (2014). Thermohydraulic and thermoeconomic performance of a marine heat exchanger on a naval surface ship. Appl. Therm. Eng. 64, 413–421. doi: 10.1016/j.applthermaleng.2013.12.061
Faes, W., Lecompte, S., Ahmed, Z. Y., Bael, J. V., Salenbien, R., Verbeken, K., et al. (2019). Corrosion and corrosion prevention in heat exchangers. Corrosion Rev. 37, 131–155. doi: 10.1515/corrrev-2018-0054
Frey, M. A., Simard, N., Robichaud, D. D., Martin, J. L., and Therriault, T. W. (2014). Fouling around: vessel sea-chests as a vector for the introduction and spread of aquatic invasive species. Manage. Biol. Invasions 5, 21–30. doi: 10.3391/mbi.2014.5.1.02
Garcia, S., and Trueba, A. (2019). “Fouling in heat exchangers,” in Inverse Heat Conduction and Heat Exchangers, eds S. Bhattacharya, M. M. Ardekani, R. Biswas, and R. C. Mehta (London: Intech Open), 1–26. doi: 10.5772/intechopen.88079
Georgiades, E., Growcott, A., and Kluza, D. (2018). Technical Guidance on Biofouling Management for Vessels Arriving to New Zealand. Ministry for Primary Industries Technical Paper No: 2018/07. (Wellington: Ministry for Primary Industries), 20.
Georgiades, E., and Kluza, D. (2020). Conduct of in-Water Biofouling Surveys for Domestic Vessels. Biosecurity New Zealand Technical Paper No: 2020/04. (Wellington: Ministry for Primary Industries), 42.
Georgiades, E., Kluza, D., Bates, T., Lubarsky, K., Brunton, J., Growcott, A., et al. (2020). Regulating vessel biofouling to support New Zealand’s marine biosecurity system – A blueprint for evidence-based decision making. Front. Mar. Sci. 7:390. doi: 10.3389/fmars.2020.00390
Georgiades, E., Scianni, C., Davidson, I., Tamburri, M. N., First, M. R., Ruiz, G., et al. (2021). The role of vessel biofouling in the translocation of marine pathogens: management considerations and challenges. Front. Mar. Sci. 8:660125. doi: 10.3389/fmars.2021.660125
Gollasch, S., MacDonald, E., Belson, S., Botnen, H., Christensen, J. T., Hamer, J. P., et al. (2002). “Life in ballast tanks,” in Invasive Aquatic Species of Europe: Distribution, Impacts and Management, eds E. Leppakoski, S. Gollasch, and S. Olenin (Dordrecht: Kluwer Academic Publishers), 217–231. doi: 10.1007/978-94-015-9956-6_23
Grandison, C., Piola, R., and Fletcher, L. (2011). A Review of Marine Growth Protection System (MGPS) Options for the Royal Australian Navy. Defence Science and Technology Organisation (Australia), Maritime Platforms Division, DSTO-TR-2631. (Canberra: Defence Science and Technology Organisation), 38.
Greene, C. H., and Schoener, A. (1982). Succession on marine hard substrata: a fixed lottery. Oecologia 55, 289–297. doi: 10.1007/BF00376914
Growcott, A., Kluza, D., and Georgiades, E. (2016). Literature Review: In-Water Systems to Remove or Treat Biofouling in Vessel Sea Chests and Internal Pipework. MPI Technical Paper No: 2016-16. (Wellington: New Zealand Ministry for Primary Industries), 66.
Growcott, A., Kluza, D., and Georgiades, E. (2017). Review: in-water systems to reactively manage biofouling in sea chests and internal pipework. Mar. Technol. Soc. J. 51, 89–104. doi: 10.4031/MTSJ.51.2.3
Guney, C. B., Danisman, D. B., and Bozkurtoglu, S. N. E. (2020). Reduction of ballast tank sediment: evaluating the effect of minor structural changes and developing a pneumatic cleaning system. Ocean Eng. 203:107204. doi: 10.1016/j.oceaneng.2020.107204
Gust, N., Coutts, A., Reinfrank, R., and Lewis, P. (2018b). Engineering and Material Considerations for Potential Treatment of Internal Seawater Systems on Large Vessels. Report for the Australian Department of Agriculture and Water Resources. (Canberra: Australian Department of Agriculture and Water Resources), 78.
Gust, N., Coutts, A., Reinfrank, R., and Lewis, P. (2018a). Biofouling Characteristics of ISS on Large Vessels. Report for the Australian Department of Agriculture and Water Resources. (Canberra: Australian Department of Agriculture and Water Resources), 65.
Hafner, I. A., Gabrielli, C. H., and Widell, K. (2018). Refrigeration Units in Marine Vessels: Alternatives to HCFCs and High GWP HFCs. (Copenhagen: Nordic Council of Ministers), 80. doi: 10.6027/TN2019-527
Hamer, J. P. (2002). “Ballast tank sediments,” in Invasive Aquatic Species of Europe: Distribution, Impacts and Management, eds E. Leppäkoski, S. Gollasch, and S. Olenin (Dordrecht: Springer), 232–234. doi: 10.1007/978-94-015-9956-6_24
Hashemi, S. J., Bak, N., Khan, F., Hawboldt, K., Lefsrud, L., and Wolodko, J. (2018). Bibliometric analysis of microbiologically influenced corrosion (MIC) of oil and gas engineering systems. Corrosion 74, 468–486. doi: 10.5006/2620
Hewitt, C. L., Gollasch, S., and Minchin, D. (2009). “The vessel as a vector – Biofouling, ballast water and sediment,” in Biological Invasions in Marine Ecosystems Ecological Studies (Analysis and Synthesis), Vol. 204, eds G. Rilov and J. A. Crooks (Berlin: Springer), 117–131. doi: 10.1007/978-3-540-79236-9_6
Howard, A. E. (1994). The possibility of long-distance transmission of Bonamia by fouling on boat hulls. Bull. Eur. Assoc. Fish Pathol. 14, 211–212.
Inglis, G. J., Floerl, O., Ahyong, S., Cox, S., Unwin, M., Ponder-Sutton, A., et al. (2010). The Biosecurity Risks Associated with Biofouling on International Vessels Arriving in New Zealand: Summary of the Patterns and Predictors of Fouling. Report to the Ministry of Agriculture and Forestry. (Wellington: Ministry of Agriculture and Forestry), 182.
International Association of Classification Societies [IACS] (2018). A Guide to Managing Maintenance in Accordance with the Requirements of the ISM Code. IACS Rec 2001/Rev2 2018. (London: IACS), 9.
International Maritime Organization [IMO] (1993). International Management Code for the Safe Operation of Ships and for Pollution Prevention (International Safety Management (ISM) Code). London: International Maritime Organization.
International Maritime Organization [IMO] (2002). Construction – fire protection, fire detection, and fire extinction. Summary of International Convention of Safety of Life at Sea (SOLAS) chapter II – 2. Available online at: https://www.imo.org/en/OurWork/Safety/Pages/summaryofsolaschapterii-2-default.aspx (accessed May, 2021).
International Maritime Organization [IMO] (2004). International Convention for the Control and Management of Ships’ Ballast Water and Sediments. London: International Maritime Organization.
International Maritime Organization [IMO] (2011). Guidelines for the Control and Management of Ships’ Biofouling to Minimize the Transfer of Invasive Aquatic Species. (London: Committee MEP), 62.
International Standards Organisation [ISO] (2008). ISO 14726:2008. Ships and Marine Technology – Identification Colours for the Content of Piping Systems. (Geneva: ISO), 13.
Issa, M., Ibrahim, H., Ilinca, A., and Hayyani, M. (2019). A review and economic analysis of different emission reduction techniques for marine diesel engines. Open J. Mar. Sci. 9, 148–171. doi: 10.4236/ojms.2019.93012
Ji, J. S. (2020). The IMO 2020 sulphur cap: A step forward for planetary health? Lancet Planet. Health 4, e46–e47. doi: 10.1016/S2542-5196(20)30002-4
Jones, J. M., and Little, B. (1990a). USS Princeton (CG 59): Impact of Marine Macrofouling (Mussels and Hydroids) ON Failure/Corrosion Problems in Seawater Piping Systems. (Washington, DC: Naval Oceanographic and Atmospheric Research Laboratory), 19.
Jones, J. M., and Little, B. (1990b). USS Princeton (CG 59): Microbiologically Influenced Corrosion (MIC) and Macrofouling Status of Seawater Piping Systems. (Dahlgren, VA: Naval Surface Warfare Center), 60. doi: 10.21236/ADA476675
Kah Hou, T., Kazi, S. N., Mahat, A. B., Teng, C. B., Al-Shamma’a, A., and Shaw, A. (2019). “Industrial heat exchanger: operation and maintenance to minimize fouling and corrosion,” in Heat exchangers – Advanced features and applications, eds S. M. Sohel Murshed and M. M. Lopes (London: Intech Open), 193–207.
Kongsberg (2014). Kongsberg 2014 ERS MAN B&W 5L90MC-L11 Machinery & Operation MC90-V. Operators Manual Part 3. Doc.no.: SO-1342-G/26-Mar-14. (Kongsberg: Kongsberg Maritime), 210.
Krata, P., Wawrzynski, W., and Wieckiewicz, and Jachowski, J. (2012). Ships’ ballast tanks size and dimensions review for the purpose of model research into the liquid sloshing phenomenon. Sci. J. 29, 88–94.
Lawrence, D., Blake, P. A., Yashuk, J. C., Wells, J. G., Creech, W. B., and Hughes, J. H. (1979). Vibrio parahaemolyticus gastroenteritis outbreaks aboard two cruise ships. Am. J. Epidemiol. 190, 71–80. doi: 10.1093/oxfordjournals.aje.a112661
Leary, M., Piola, R., Shimeta, J., Toppi, S., Mayson, S., McMillan, M., et al. (2016). Additive manufacture of anti-biofouling inserts for marine applications. Rapid Prototyp. J. 22, 416–434. doi: 10.1108/RPJ-02-2014-0022
Lewis, J. A. (2016). Assessment of Preventative Biofouling Management Measures. Report Prepared for Ministry for Primary Industries. (Wellington: Ministry for Primary Industries), 125.
Lewis, J. A., and Dimas, J. (2007). Treatment of Biofouling in Internal Seawater Systems – phase 2. Defence Science and Technology Organisation (Australia), Marine Platforms Division. DSTO-TR-2081. (Canberra: Defence Science and Technology Organisation), 31.
Lewis, J. A., and Smith, B. S. (1991). “Hydroides settlement in Sydney Harbour (Australia) and its control in sea-water cooling systems,” in Biodeterioration and Biodegradation 8. Elsevier Applied Science, ed. H. W. Rossmoore (London: Elsevier), 464–466.
Lewis, J. A., Smith, B. S., Taylor, R. J., and Batten, J. J. (1988). “Fouling of RAN submarine seawater systems and a comparison of electro-chemical control methods,” in Proceedings of the Eighth Inter-Naval Corrosion Control Conference, Plymouth, 20.
Li, Y., and Ning, C. (2019). Latest research progress of marine microbiological corrosion and biofouling, and new approaches of marine anti-corrosion and anti-fouling. Bioact. Mater. 4, 189–195. doi: 10.1016/j.bioactmat.2019.04.003
Machuca, L. L. (2019). Understanding and addressing microbiologically influenced corrosion (MIC). Corros. Mater. 44, 88–96.
McCarthy, S. A., and Khambaty, F. M. (1994). International dissemination of epidemic Vibrio cholerae by cargo ship ballast and other nonpotable waters. Appl. Environ. Microbiol. 60, 2597–2601. doi: 10.1128/aem.60.7.2597-2601.1994
Minton, M. S., Verling, E., Miller, A. W., and Ruiz, G. M. (2005). Reducing propagule supply and coastal invasions via ships: effects of emerging strategies. Front. Ecol. Environ. 3, 304–308. doi: 10.2307/3868563
Moser, C. S., Wier, T. P., First, M. R., Grant, J. F., Riley, S. C., Robbins-Wamsley, S. H., et al. (2017). Quantifying the extent of niche areas in the global fleet of commercial ships: the potential for “super-hot spots” of biofouling. Biol. Invasions 19, 1745–1759. doi: 10.1007/s10530-017-1386-4
Muilenberg, T., and Candir, C. (2013). How Stripping Biofilm from the Cooling Water Loop Impacts Power Plant Production Output. Cooling Technology Institute Paper No TP13-09. (Houston, TX: Cooling Technology Institute), 9.
New Zealand Ministry for Primary Industries (2014). Craft Risk Management Standard: Biofouling on Vessels Arriving to New Zealand. (Wellington: Ministry for Primary Industries), 8.
Newman, W. A. (1963). On the introduction of an edible oriental shrimp (Caridea, Palaemonidae) to San Francisco Bay. Crustaceana 5, 199–132. doi: 10.1163/156854063X00390
Owen, D., Demirel, Y. K., Oguz, E., Tezdogan, T., and Incecik, A. (2018). Investigating the effect of biofouling on propeller characteristics using CFD. Ocean Eng. 159, 505–516. doi: 10.1016/j.oceaneng.2018.01.087
Pagenkopp Lohan, K. M., Fleischer, R. C., Carney, K. J., Holzer, K. K., and Ruiz, G. M. (2017). Molecular characterisation of protistan species and communities in ships’ ballast water across three US coasts. Diversity and Distributions. 23, 680–691. doi: 10.1111/ddi.12550
Pagenkopp Lohan, K. M., Ruiz, G. M., and Torchin, M. E. (2020). “Invasions can drive marine disease dynamics,” in Marine Disease Ecology, eds D. C. Behringer, K. D. Lafferty, and B. D. Silliman (Oxford: Oxford University Press), 115–138. doi: 10.1093/oso/9780198821632.003.0007
Palermo, M. (1992). Components of Vessels and Dredges Susceptible to Zebra Mussel Infestations. Technical Note ZMR-3-07 US Army Engineer Waterways Experiment Station. (Vicksburg: US Army Engineer Waterways Experiment Station), 3.
Pamitran, A. S., Adam, S. A., and Alhamid, M. I. (2016). Cost estimation study for the effect of biofouling on engine cooling system performance with an 8000 BHP vessel. Int. J. Appl. Mech. 819, 427–431. doi: 10.4028/www.scientific.net/AMM.819.427
Pastoris, M. C., Lo Monaca, R., Goldoni, P., Mentore, B., Balestra, G., Ciceroni, L., et al. (1999). Legionnaires’ disease on a cruise ship linked to the water supply systems: clinical and public health implications. Clin. Infect. Dis. 28, 33–38. doi: 10.1086/515083
Polglaze, J. (2019). Warships and biofouling: a comparison with commercial ships. Paper Presented at the 4th ANZPAC Workshop on Biofouling Management for Sustainable Shipping, Melbourne.
Polman, H., Verhaart, F., and Bruijs, M. (2013). Impact of biofouling in intake pipes on the hydraulics and efficiency of pumping capacity. Desalination Water Treat. 51, 997–1003. doi: 10.1080/19443994.2012.707371
Powell, C. A., and Michels, H. T. (2000). Copper-Nickel Alloys for Seawater Corrosion Resistance and Anti-Fouling -A State of the Art Review. In: CORROSION 2000 NACE International. Available online at: https://www.copper.org/applications/marine/cuni/properties/corrosion/corrosion_resistance_and_antifouling.html (accessed May, 2020).
Rajagopal, S., Jenner, H. A., and Venugopalan, V. P. (2012). Operational and Environmental Consequences of Large Industrial Cooling Water Systems. (New York, NY: Springer), 477. doi: 10.1007/978-1-4614-1698-2
Schultz, M. P., Bendick, J. A., Holm, E. R., and Hertel, W. M. (2011). Economic impact of biofouling on a naval surface ship. Biofouling 27, 87–98. doi: 10.1080/08927014.2010.542809
Schutz, R. W., and Scaturro, M. R. (1991). An overview of current and candidate titanium alloy applications on US Navy surface ships. Naval Eng. J. 103, 175–191. doi: 10.1111/j.1559-3584.1991.tb00948.x
Scianni, C., and Georgiades, E. (2019). Vessel in-water cleaning or treatment: identification of environmental risks and science needs for evidence-based decision making. Front. Mar. Sci. 6:647. doi: 10.3389/fmars.2019.00467
Smith, L. D., Wonham, M. J., McCann, L. D., and Ruiz, G. M. (1999). Invasion pressure to a ballast-flooded estuary and an assessment of inoculant survival. Biol. Invasions 1, 67–87. doi: 10.1023/A:1010094527218
Steinhagen, R., Müller-Steinhagen, H., and Maani, K. (1993). Problems and costs due to heat exchanger fouling in New Zealand industries. Heat Transfer Eng. 14, 19–30. doi: 10.1080/01457639308939791
Strietman, W. J., and Leemans, E. (2019). Review of the Implementation of the IMO’s 2011 Biofouling Guidelines in the Netherlands. Wageningen Economic Research, Report 2019-013. (Wageningen: Wageningen Economic Research), 30.
Teuchies, J., Cox, T. J., Van Itterbeeck, K., Meysman, F. J., and Blust, R. (2020). The impact of scrubber discharge on the water quality in estuaries and ports. Environ. Sci. Europe 32:103. doi: 10.1186/s12302-020-00380-z
Townsin, R. L. (2003). The ship hull fouling penalty. Biofouling 19, 9–15. doi: 10.1080/0892701031000088535
Transportation Safety Board of Canada (2014). Marine Investigation Report M14A0051. Flooding and Subsequent Grounding of Bulk Carrier John I off the Southwest Coast of Newfoundland and Labrador 14 March 2014. (Quebec: Government of Canada), 26.
UK Marine Accident Investigation Branch (1999). Marine Accident Report 5/99. Report of the Inspector’s Inquiry into the Loss of MV Green Lily on the 19 November 1997 off the east coast of Bressay, Shetland Islands. (London: Department of the Environment), 79.
UK Marine Accident Investigation Branch (2010). Short Summary of the Accident and Action Taken: Thames Fisher. (Southampton: Marine Accident Investigation Branch), 2.
UK Marine Accident Investigation Branch (2013). Report on the Investigations of the Flooding and Foundering of the Fishing Vessels Audacious and Chloe T. (Southampton: Marine Accident Investigation Branch), 65.
United States Coast Guard [USCG] (2018). Marine Safety Information Bulletin 10-18: Loss of Vessel Propulsion, Maneuverability and Safety Systems Due to Clogged Sea Strainers. (Houston, TX: Coast Guard Sector Houston-Galveston), 7.
US Environmental Protection Agency (1999). Phase I Final Rule and Technical Development Document of Uniform National Discharge Standards (UNDS): Seawater Cooling Overboard Discharge, Nature of Discharge. (Washington, DC: US Environmental Protection Agency), 24.
US Environmental Protection Agency (2013). Vessel General Permit for Discharges the Normal Operation of Vessels (VGP). (Washington, DC: US Environmental Protection Agency), 194.
US Maritime Training Advisory Board (1994). Marine Fire Prevention, Firefighting and Fire Safety. (Darby, PA: Diane Publications), 388.
Verling, E., Ruiz, G. M., Smith, L. D., Galil, B., Miller, A. W., and Murphy, K. R. (2005). Supply-side invasion ecology: characterizing propagule pressure in coastal ecosystems. Proc. R. Soc. Lond. Ser. B 272, 1249–1257. doi: 10.1098/rspb.2005.3090
Visscher, J. P. (1928). Nature and extent of fouling of ships’ bottoms. Bull. Bureau Fish. 43, 193–252. doi: 10.5962/bhl.title.39203
Wanless, R. M., Scott, S., Sauer, W. H., Andrew, T. G., Glass, J. P., Godfrey, B., et al. (2010). Semi-submersible rigs: a vector transporting entire marine communities around the world. Biol. Invasions 12, 2573–2583. doi: 10.1007/s10530-009-9666-2
Whittington, R. J., Paul-Pont, I., Evans, O., Hick, P., and Dhand, N. K. (2018). Counting the dead to determine the source and transmission of the marine herpesvirus OsHV-1 in Crassostrea gigas. Vet. Res. 49:34. doi: 10.1186/s13567-018-0529-7
Wonham, M., Carlton, J., Ruiz, G., and Smith, L. D. (2000). Fish and ships: relating dispersal frequency to success in biological invasions. Mar. Biol. 136, 1111–1121. doi: 10.1007/s002270000303
Woods Hole Oceanographic Institution (1952). Marine Biofouling and its Prevention. (Annapolis, MD: United States Naval Institute Press), 388.
Keywords: internal seawater systems, biofouling, ship pipework, engine cooling, operational impacts, biosecurity
Citation: Davidson I, Cahill P, Hinz A, Kluza D, Scianni C and Georgiades E (2021) A Review of Biofouling of Ships’ Internal Seawater Systems. Front. Mar. Sci. 8:761531. doi: 10.3389/fmars.2021.761531
Received: 20 August 2021; Accepted: 07 October 2021;
Published: 28 October 2021.
Edited by:
Satya Panigrahi, Indira Gandhi Centre for Atomic Research (IGCAR), IndiaReviewed by:
Sriyutha Murthy, Bhabha Atomic Research Centre, IndiaMarie Nydam, Soka University of America, United States
Copyright © 2021 Davidson, Cahill, Hinz, Kluza, Scianni and Georgiades. This is an open-access article distributed under the terms of the Creative Commons Attribution License (CC BY). The use, distribution or reproduction in other forums is permitted, provided the original author(s) and the copyright owner(s) are credited and that the original publication in this journal is cited, in accordance with accepted academic practice. No use, distribution or reproduction is permitted which does not comply with these terms.
*Correspondence: Ian Davidson, SWFuLkRhdmlkc29uQGNhd3Rocm9uLm9yZy5ueg==