Homeostasis Regulation by Potassium Channel Subfamily K Member 3 (KCNK3) in Various Fishes
- 1Key Laboratory of Sichuan Province for Fishes Conservation and Utilization in the Upper Reaches of the Yangtze River, Neijiang Normal University, Neijiang, China
- 2College of Life Science, Neijiang Normal University, Neijiang, China
- 3Key Laboratory of Bio-Resources and Eco-Environment of Ministry of Education, College of Life Sciences, Sichuan University, Chengdu, China
- 4Shenzhen Key Lab of Marine Genomics, Guangdong Provincial Key Lab of Molecular Breeding in Marine Economic Animals, BGI Academy of Marine Sciences, BGI Marine, BGI, Shenzhen, China
Potassium channels are important for K+ transport and cell volume regulation, which play important roles in many biological processes such as hormone secretion, ion homeostasis, excitability, and cell development. In mammals, a total of 15 potassium channels were identified and they were divided into six subfamilies, including TALK (TALK1, TALK2, TASK2), TASK (TASK1, TASK3, TASK5), TREK (TREK1, TREK2, TRAAK), TWIK (TWIK1, TWIK2, KCNK7), THIK (THIK1, THIK2) and TRESK. TASK1, also known as potassium channel subfamily k member 3 (KCNK3), is the first member identified in the TASK subfamily. This K2P channel has potential applications in fish breeding and aquaculture industry due to its important roles in various physiological processes. Despite its functional role has been well studied in mammals; however, it is less known in fishes. In this review, we systematically summarize recent research advances of this critical potassium channel in representative fishes, such as gene number variation, tissue distribution, phylogeny, and potential homeostasis regulation role. This paper provides novel insights into the functional properties of these fish kcnk3 genes (including osmoregulation, energy homeostasis maintenance and fatty acids metabolism regulation), and also expands our knowledge about their variations among diverse fishes.
Introduction
Potassium channels are the largest ion channel family with more than 78 genes encoding pore-forming subunits in the human genome (Feliciangeli et al., 2015). This family consists of three structure-differential subclasses including inward rectifiers (Kir), voltage-gated K+ (Kv) channels and two-pore domain K+ (K2P) channels (Goldstein et al., 2001; Gutman et al., 2005; Gonzalez et al., 2012). Different from the other two subclasses, K2P channels were originally identified from DNA database mining without any clues about their electrophysiological and functional properties (Lesage et al., 1996). Thus far, a total of 15 genes have been identified to encode K2P channels that are involved in the development and excitability of many cell types (Mathie, 2007; Feliciangeli et al., 2015). These channels are modulated by a wide range of chemical and physical stimuli, and they have been widely used as the targets of various drugs such as neuroprotective, anti-depressive and anesthetics agents (Talley and Bayliss, 2002; Olschewski et al., 2017). These K2P channels can be divided into six subfamilies comprising Two P-domain in a weakly inward rectifying K+ channel (TWIK1, TWIK2, KCNK7), TWIK-related acid-sensitive K+ channel (TASK1, TASK3, TASK5), TWIK-related K+ channel (TREK1, TREK2, TRAAK), TWIK-related alkaline-sensitive K+ channel (TALK1, TALK2, TASK2), Tandem pore domain halotane-inhibited K+ channel (THIK1, THIK2) and TWIK-related spinal cord K+ channel (TRESK), according to their structural and functional properties (Feliciangeli et al., 2015; Wen et al., 2019).
Thus far, various K2P channels have been well studied in mammals. However, only a few counterparts were preliminarily characterized and investigated in zebrafish, an well-established model for human diseases (Shin and Fishman, 2002). The TREK2 is the first K2P channel identified in zebrafish, and this channel exhibits similar structural and functional properties as human TREK2 (Gierten et al., 2012). Meanwhile, TRESK was characterized in zebrafish, and functional experiments revealed that this channel shares similar functions as its counterpart in human, such as open rectification properties, inhibition by barium, and regulation by signaling molecules including protein kinases PKC & PKA and phospholipase C (Rahm et al., 2014). Additionally, zebrafish TASK2 channel was determined to be inhibited by increased CO2 and intracellular acidification (Pena-Munzenmayer et al., 2014). Interestingly, two isoforms of TASK1 channels were identified in zebrafish, and they were proposed to contribute to an atrial substrate for arrhythmogenesis (Liang et al., 2014). Similarly, two paralogs of kcnk10 were identified in zebrafish, which were predicted to play important roles in regulation of reproduction (Loganathan et al., 2017). Recently, another two paralogs of TWIK related potassium channels, Kcnk2a and Kcnk2b, were reported and they were proved to exhibit similar functional traits as their counterpart in human (Nasr et al., 2018). Furthermore, two THIK1 isoforms are newly identified in zebrafish, and they were considered to exhibit similar structural and functional properties as human THIK1, due to their activation by arachidonic acid and reduction by barium, mexiletine, lidocaine, and inhibited phospholipase C (Staudacher et al., 2019).
TASK1, also known as potassium channel subfamily k member 3 (KCNK3) or K2P3.1, is the first member identified in the TASK subfamily to participate in regulation of resting membrane potential in several cell types (Olschewski et al., 2017; Lambert et al., 2018, 2019). This channel is characterized by four transmembrane domains and two pore domains per subunit (Ma et al., 2013; Feliciangeli et al., 2015). KCNK3 channels are primarily expressed in the central nervous system (CNS) but also in periphery tissues including heart, adrenal gland, and pulmonary arterial smooth muscle (PASM) (Lesage and Barhanin, 2011; Lambert et al., 2018). KCNK3 is modulated by a variety of biochemical agents, such as unsaturated fatty acids (UFAs), extracellular pH, hypoxia, and anesthetics (Olschewski et al., 2017; Wen et al., 2020b). Due to these physiological traits, KCNK3 has been proposed to mediate central as well as peripheral chemoreception, such as sensing O2 and CO2 in glomus cells (Mulkey et al., 2007) and smooth muscle cells of pulmonary arteries (Olschewski et al., 2006). Meanwhile, KCNK3 is also considered as a nutrient sensor for sensing glucose in hypothalamic orexin neurons (Burdakov et al., 2006). Simultaneously, KCNK3 plays important roles in adrenal gland development and aldosterone secretion (Czirjak et al., 2000; Czirjak and Enyedi, 2002), and mineralocorticoid hormone stimulates K+ excretion and Na+ reabsorption in the kidney, contributing to fluid volume and blood pressure control (Lesage and Barhanin, 2011). Additionally, one of the most important functions of KCNK3 is involved in regulation of neuronal excitability with pH variations by affecting ion channel activity (Linden et al., 2006; Lesage and Barhanin, 2011). Moreover, KCNK3 also plays critical roles in other physiological process, such as protecting brain against ischemia (Meuth et al., 2009), regulating thermogenesis through the mineralocorticoid receptor pathway (Pisani et al., 2016) and cAMP-PKA signaling (Chen et al., 2017).
Different with mammals, studies focusing on kcnk3 are rarely reported in fishes. The fish KCNK3 channels were first identified and investigated in zebrafish (Danio rerio) in 2014 (Liang et al., 2014), but no further related results were reported in other fishes. Until recently, we systematically examined the functional properties of this channel in three representative fish species including freshwater carnivorous Northern snakehead (Channa argus) (Wen et al., 2019), euryhaline omnivorous Nile tilapia (Oreochromis niloticus) (Wen et al., 2020b) and marine herbivorous rabbitfish (Siganus canaliculatus) (Wen et al., 2020a). In this review, we are planning to summarize the gene copy number variation, tissue distribution pattern, phylogeny, and potential homeostasis regulation role of kcnk3 genes in various fishes. Our works will not only provide novel insights into the functional properties of these fish KCNK3 channels, but also establish guidelines for related studies and applications in diverse fishes in the coming future.
Gene Copy Variations in Vertebrates
Thus far, only a single copy gene of KCNK3 was identified in mammals. However, two kcnk3 paralogs were discovered in zebrafish, the first teleost that was reported to possess kcnk3 gene (Liang et al., 2014). Consistently, our further studies showed that two kcnk3 genes (kcnk3a and kcnk3b) were also existed in many other fishes such as Northern snakehead (Wen et al., 2019), Nile tilapia (Wen et al., 2020b) and rabbitfish (Wen et al., 2020a) by a comparative genomics strategy (Figure 1). These findings suggest that two copies of kcnk3 genes might be widely existed in teleost, and this phenomenon may be caused by the specific whole genome duplication (WGD) event in teleost (Hughes et al., 2018; Xu et al., 2019; Wen et al., 2020c). Additionally, a more complex situation of the gene copy numbers may have occurred in cypriniformes and salmoniformes fishes (Figure 1), because of an additional WGD event in these two linages (Lien et al., 2016; Xu et al., 2019). These gene copy variations of the kcnk3s implies that the functional properties of this channel could be also variable in various vertebrates, especially in the numerous fishes.
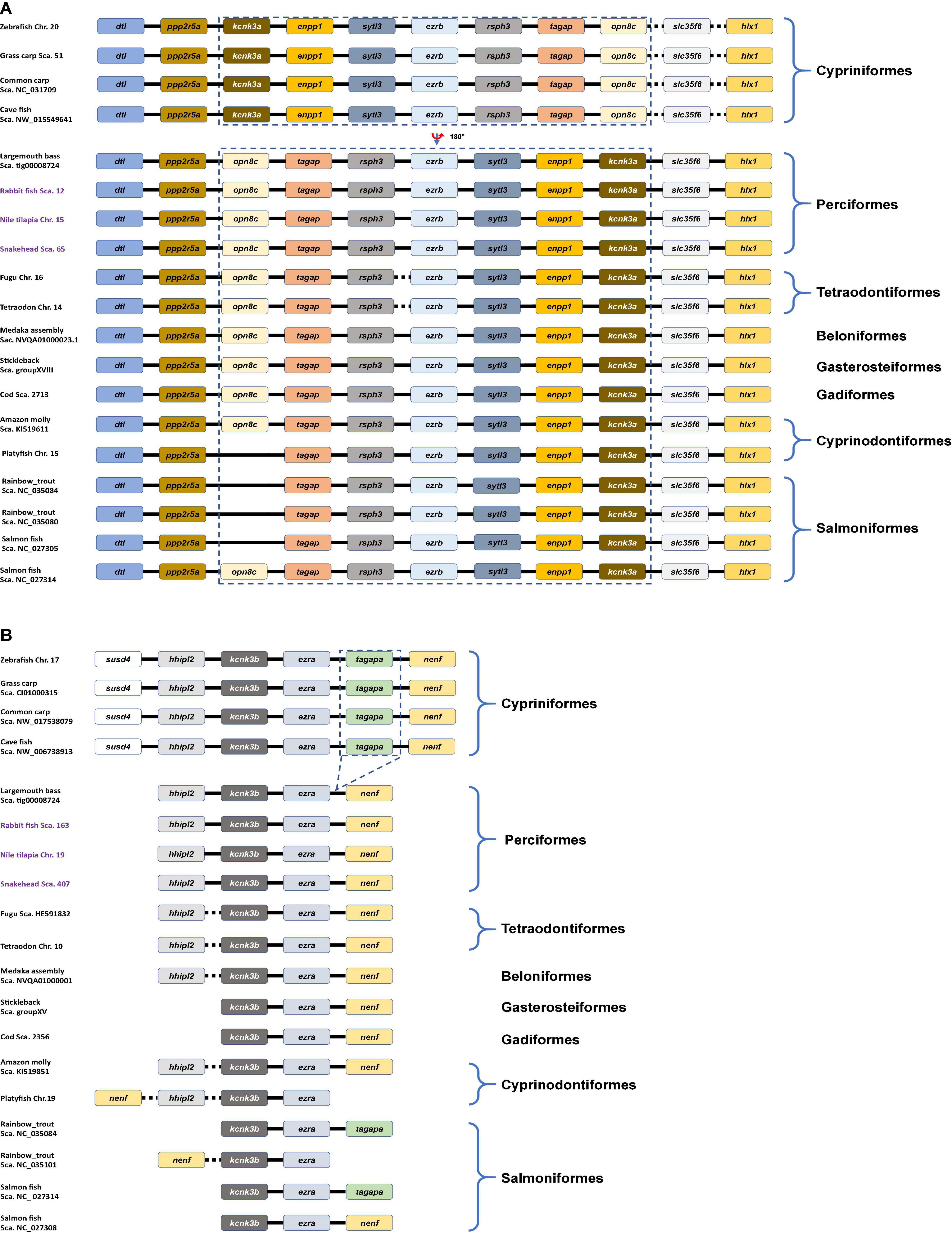
Figure 1. Genetic synteny comparisons of the kcnk3a (A) and kcnk3b (B) genes in representative fish genomes. The colorful blocks represent different genes, and the solid and dotted lines represent intergenic regions without genes and with genes, respectively. As shown in the figure, both kcnk3 genes are identified from these fish genomes, and a reversal region (A) and an insertion (B) are marked within dotted boxes. This figure was adopted from our previous report Wen et al. (2020b) with permission.
Tissue Distribution Traits in Vertebrates
In mammals, KCNK3 is primarily distributed in the CNS, but also expressed in certain periphery tissues and cells, such as heart, adrenal gland, glomus cells and smooth muscle cells of pulmonary arteries (Olschewski et al., 2006; Mulkey et al., 2007; Lesage and Barhanin, 2011). Similarly, both kcnk3 paralogs were proved to be highly transcribed in the CNS and heart in zebrafish by combining whole-mount in situ hybridization and real-time quantitative PCR (Liang et al., 2014). Meanwhile, we observed that kcnk3a and kcnk3b showed a similar distributed pattern, and both genes were widely transcribed in Nile tilapia and rabbitfish with the highest transcription levels in heart (Wen et al., 2020a,b). However, kcnk3a was mainly transcribed in heart, while kcnk3b was highly expressed in gill, brain and kidney in Northern snakehead (Wen et al., 2019). These findings suggest that the distribution patterns of kcnk3 genes are variable in various fishes, although we found a common trait that these channels are primarily expressed in the excitable tissues including CNS and heart. Meanwhile, the extensive distribution of kcnk3 genes implies diverse functions of these channels in various fishes, which needs to be further explored with in-depth investigations.
Heart Rhythm Regulation Role in Fishes
Previous studies have shown that a variety of atrium-specific K+ and Ca2+ channels are crucial in shaping the atrial action potential in the heart, including Kv1.5, K2P3.1 (KCNK3)/K2P9.1 (KCNK9), Kir3.1/Kir3.4, and KCa2.x channels (Bertaso et al., 2002; Wettwer et al., 2004; Limberg et al., 2011; Schmitt et al., 2014; Skarsfeldt et al., 2016). In human, KCNK3 contributes to action potential repolarization and the resting membrane potential of atrial cardiomyocytes (Le Ribeuz et al., 2020). Knockdown of KCNK3 in human-induced pluripotent stem cell (iPSC)-derived cardiomyocytes results in a significant prolongation of action potential duration, suggesting that KCNK3 also contributes to action potential repolarization in human ventricular cardiomyocytes (Chai et al., 2017). Meanwhile, KCNK3-knockout mice showed a ventricular phenotype with prolonged QT interval, wide QRS, reduced heart rate variability (HRV) and increased ventricular action potential duration (Donner et al., 2011). Similarly, knockdown both kcnk3a and kcnk3b genes resulted in lower heart rate and higher atrial and ventricular diameters in zebrafish (Liang et al., 2014), indicating that inactivation of kcnk3 may have diverse effects on atrial size and electrophysiological properties that can contribute to an arrhythmogenic substrate.
However, a recent study revealed that KCNK3/KCNK9 and KCa2.x channels do not appear to be involved in regulation of the action potential in the zebrafish heart (Skarsfeldt et al., 2016). Despite the controversial results were obtained in zebrafish, the important roles of the KCNK3 channels in heart rhythm regulation should be noticed and more investigations are required to illustrate the detailed functions in both mammals and fishes.
Osmoregulation Roles in Fishes
Different from mammals, most fishes reside in various water environments and they must address the problem of ion-homeostasis between the body and the waters, especially for euryhaline fishes. As an important ion channel family, potassium channels may highly play potential roles in osmoregulation in fishes. Indeed, a previous transcriptomic study in Mozambique tilapia (Oreochromis mossambicus) revealed that kcnk3s could be involved in osmoregulation (Lam et al., 2014). In order to validate this speculation, we explored the potential osmoregulation roles of both kcnk3a and kcnk3b genes in euryhaline Nile tilapia and marine rabbitfish (Wen et al., 2020a,b). In tilapia, both kcnk3 genes in the gill were proved to have a similar transcriptional change pattern in response to two-week acclimation in various salinity of seawater (0, 5, 10, and 20 psu) with the lowest transcription levels in 10 psu (Wen et al., 2020b), implying that these two channels might be involved in osmoregulation. Meanwhile, three putative transcription factors (TF) including arid3a, arid3b, and arid5a exhibited a similar transcription pattern with the two kcnk3 genes, implying their positive regulatory roles in osmoregulation by targeting these two kcnk3 genes (Wen et al., 2020b). Consistently, our further study in rabbitfish showed that both kcnk3 genes had higher transcription levels in sweater in comparison with that in brackish water (Figure 2), which further confirmed that these two channels are involved in osmoregulation in fishes (Wen et al., 2020a). However, the exactly regulated mechanisms are still unknown, which are required for further investigation.
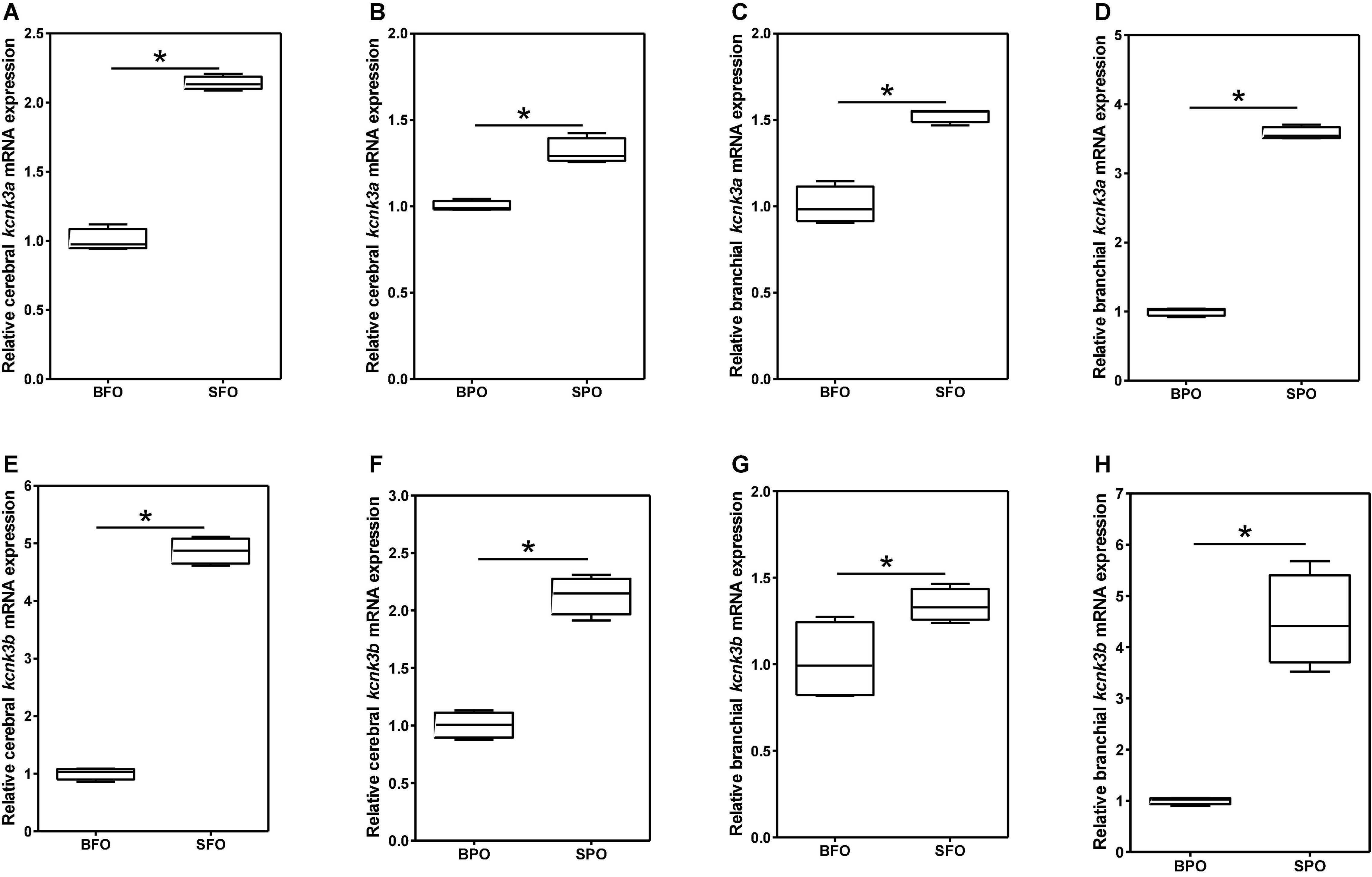
Figure 2. Effects of different water salinities on the mRNA levels of kcnk3a (A–D) and kcnk3b (E–H) in rabbitfish. Two tissues including brain and gill were examined. 18S RNA was selected and used as the reference gene. Experimental fishes were fed with two different diets, fish oil (FO) or plant oil (PO) as the dietary lipid source. Asterisk (*) represents a significant difference between the two groups. This figure was adopted from our previous work Wen et al. (2020a) with permission.
Energy Homeostasis Maintenance Role in Fishes
In mammals, hypothalamic orexin neurons are able to sense extracellular glucose concentration to trigger adaptive responses in response to low body energy level, including wakefulness and food-seeking behavior, by inhibiting a background K+ current that can promote depolarization and enhanced excitability (Burdakov et al., 2006; Burdakov and Lesage, 2010). This phenomenon suggests that kcnk3 may play important roles in nutrients and energy homeostasis regulation in vertebrates. However, no related reports were provided in fishes. In order to explore the potential regulatory role of kcnk3 in energy homeostasis maintenance, we examined the transcription patterns of both kcnk3a and kcnk3b genes in response to different feeding status in Northern snakehead (Wen et al., 2020a). Our results showed that two kcnk3 genes in the brain presented totally opposite change patterns in response to both short- (24 h) and long-term (2 weeks) food deprivations, implying that both snakehead kcnk3a and kcnk3b might form a negative feedback control during the physiological process of appetite regulation, and the former potentially serves as an orexigenic factor while the latter may play as an anorexigenic factor. Anyway, our findings indicate that the two kcnk3 genes in the snakehead fish might function differentially, while with cooperation, in appetite regulation and energy homeostasis maintenance.
Long Chain Polyunsaturated Fatty Acids Metabolism Regulation in Fishes
K2P channels have been regarded as important biochemical and medical sensors since they are sensitive to natural and chemical effectors, such as extracellular acidification (Duprat et al., 1997), volatile anesthetics (Patel et al., 1999; Talley and Bayliss, 2002), hypoxia (Kemp et al., 2004), and long chain polyunsaturated fatty acids (LC-PUFA) (Feliciangeli et al., 2015). Several previous studies have shown that LC-PUFA can induce activation of the K2P channels, such as TREK1 (Fink et al., 1998), TREK2 (Bang et al., 2000), and TRAAK1 (Lesage et al., 2000). However, whether kcnk3 is sensitive to LC-PUFA has not yet well studied. Our research in rabbitfish showed that fishes fed diets with fish oil as dietary lipid (rich in LC-PUFA) induced higher mRNA levels of the two kcnk3 genes in comparison with those in fishes fed with plant oil diet (lack of LC-PUFA) at two different salinity environments (32 and 15‰), suggesting that LC-PUFA also can stimulate the activation of the KCNK3 channels, and thus these channels may play important roles in LC-PUFA metabolism regulation in various vertebrates, including fishes. However, more studies are required to explore the exact mechanisms of this phenomenon.
Conclusion
The KCNK3 potassium channels are crucial for many biological processes. Although the structural and functional properties of KCNK3s have been well investigated in mammals, the genetic variations and functional traits of the counterparts are still rarely known in fishes. A previous study revealed that two kcnk3 genes are existed in zebrafish and they may play important roles in heart beating. Our further works on three representative fishes with different environments and feeding habits showed that two kcnk3 paralogs (kcnk3a and kcnk3b) are widely existed in diverse fish species, and these two channels are involved in heart rhythm regulation, osmoregulation, energy homeostasis maintenance, and LC-PUFA metabolism regulation. However, extensive distribution of the two channels in various fishes implies that they may play important roles in different tissues. Therefore, more in-depth studies are required to further investigate the genetic and functional traits of these channels, which can be selected as potential targets for molecular breeding in practical fish industry, such as developing new variants that can survive healthily in broader salinity environments.
Author Contributions
Z-YW and QS conceived the project. Z-YW wrote the manuscript. C-JQ, Y-YL, Y-PL, Y-CZ, and S-TG provided assistance in the manuscript preparation. QS revised the manuscript. All authors contributed to the article and approved the submitted version.
Funding
This manuscript was funded by the Scientific Program of Sichuan Department of Science and Technology (nos. 2021YFYZ0015 and 21ZDYF2526), the Special Research Program of Neijiang Normal University (no. 17ZL03), and Shenzhen Science and Technology Program for International Cooperation (no. GJHZ20190819152407214).
Conflict of Interest
The authors declare that the research was conducted in the absence of any commercial or financial relationships that could be construed as a potential conflict of interest.
Publisher’s Note
All claims expressed in this article are solely those of the authors and do not necessarily represent those of their affiliated organizations, or those of the publisher, the editors and the reviewers. Any product that may be evaluated in this article, or claim that may be made by its manufacturer, is not guaranteed or endorsed by the publisher.
References
Bang, H., Kim, Y., and Kim, D. (2000). TREK-2, a new member of the mechanosensitive tandem-pore K+ channel family. J. Biol. Chem. 275, 17412–17419. doi: 10.1074/jbc.m000445200
Bertaso, F., Sharpe, C. C., Hendry, B. M., and James, A. F. (2002). Expression of voltage-gated K+ channels in human atrium. Basic Res. Cardiol. 97, 424–433. doi: 10.1007/s00395-002-0377-4
Burdakov, D., Jensen, L. T., Alexopoulos, H., Williams, R. H., Fearon, I. M., O’Kelly, I., et al. (2006). Tandem-pore K+ channels mediate inhibition of orexin neurons by glucose. Neuron 50, 711–722. doi: 10.1016/j.neuron.2006.04.032
Burdakov, D., and Lesage, F. (2010). Glucose-induced inhibition: how many ionic mechanisms? Acta Physiol. 198, 295–301. doi: 10.1111/j.1748-1716.2009.02005.x
Chai, S., Wan, X., Nassal, D. M., Liu, H., Moravec, C. S., Ramirez-Navarro, A., et al. (2017). Contribution of two-pore K(+) channels to cardiac ventricular action potential revealed using human iPSC-derived cardiomyocytes. Am. J. Physiol. Heart. Circ. Physiol. 312, H1144–H1153. doi: 10.1152/ajpheart.00107.2017
Chen, Y., Zeng, X., Huang, X., Serag, S., Woolf, C. J., and Spiegelman, B. M. (2017). Crosstalk between KCNK3-mediated ion current and adrenergic signaling regulates adipose thermogenesis and obesity. Cell 171, 836–848 e813. doi: 10.1016/j.cell.2017.09.015
Czirjak, G., and Enyedi, P. (2002). TASK-3 dominates the background potassium conductance in rat adrenal glomerulosa cells. Mol. Endocrinol. 16, 621–629. doi: 10.1210/mend.16.3.0788
Czirjak, G., Fischer, T., Spat, A., Lesage, F., and Enyedi, P. (2000). TASK (TWIK-related acid-sensitive K+ channel) is expressed in glomerulosa cells of rat adrenal cortex and inhibited by angiotensin II. Mol. Endocrinol. 14, 863–874. doi: 10.1210/mend.14.6.0466
Donner, B. C., Schullenberg, M., Geduldig, N., Huning, A., Mersmann, J., Zacharowski, K., et al. (2011). Functional role of TASK-1 in the heart: studies in TASK-1-deficient mice show prolonged cardiac repolarization and reduced heart rate variability. Basic Res. Cardiol. 106, 75–87. doi: 10.1007/s00395-010-0128-x
Duprat, F., Lesage, F., Fink, M., Reyes, R., Heurteaux, C., and Lazdunski, M. (1997). TASK, a human background K+ channel to sense external pH variations near physiological pH. EMBO J. 16, 5464–5471. doi: 10.1093/emboj/16.17.5464
Feliciangeli, S., Chatelain, F. C., Bichet, D., and Lesage, F. (2015). The family of K2P channels: salient structural and functional properties. J. Physiol. 593, 2587–2603. doi: 10.1113/jphysiol.2014.287268
Fink, M., Lesage, F., Duprat, F., Heurteaux, C., Reyes, R., Fosset, M., et al. (1998). A neuronal two P domain K+ channel stimulated by arachidonic acid and polyunsaturated fatty acids. EMBO J. 17, 3297–3308. doi: 10.1093/emboj/17.12.3297
Gierten, J., Hassel, D., Schweizer, P. A., Becker, R., Katus, H. A., and Thomas, D. (2012). Identification and functional characterization of zebrafish K(2P)10.1 (TREK2) two-pore-domain K(+) channels. Biochim. Biophys. Acta 1818, 33–41. doi: 10.1016/j.bbamem.2011.09.015
Goldstein, S. A., Bockenhauer, D., O’Kelly, I., and Zilberberg, N. (2001). Potassium leak channels and the KCNK family of two-P-domain subunits. Nat. Rev. Neurosci. 2, 175–184. doi: 10.1038/35058574
Gonzalez, C., Baez-Nieto, D., Valencia, I., Oyarzun, I., Rojas, P., Naranjo, D., et al. (2012). K(+) channels: function-structural overview. Compr. Physiol. 2, 2087–2149. doi: 10.1002/cphy.c110047
Gutman, G. A., Chandy, K. G., Grissmer, S., Lazdunski, M., McKinnon, D., Pardo, L. A., et al. (2005). International union of pharmacology. LIII. nomenclature and molecular relationships of voltage-gated potassium channels. Pharmacol. Rev. 57, 473–508. doi: 10.1124/pr.57.4.10
Hughes, L. C., Orti, G., Huang, Y., Sun, Y., Baldwin, C. C., Thompson, A. W., et al. (2018). Comprehensive phylogeny of ray-finned fishes (Actinopterygii) based on transcriptomic and genomic data. Proc. Natl. Acad. Sci. U.S.A. 115, 6249–6254. doi: 10.1073/pnas.1719358115
Kemp, P. J., Peers, C., Lewis, A., and Miller, P. (2004). Regulation of recombinant human brain tandem P domain K+ channels by hypoxia: a role for O2 in the control of neuronal excitability? J. Cell. Mol. Med. 8, 38–44. doi: 10.1111/j.1582-4934.2004.tb00258.x
Lam, S. H., Lui, E. Y., Li, Z., Cai, S., Sung, W. K., Mathavan, S., et al. (2014). Differential transcriptomic analyses revealed genes and signaling pathways involved in iono-osmoregulation and cellular remodeling in the gills of euryhaline Mozambique tilapia, Oreochromis mossambicus. BMC Genomics 15:921. doi: 10.1186/1471-2164-15-921
Lambert, M., Capuano, V., Boet, A., Tesson, L., Bertero, T., Nakhleh, M. K., et al. (2019). Characterization of Kcnk3-mutated rat, a novel model of pulmonary hypertension. Circ. Res. 125, 678–695. doi: 10.1161/CIRCRESAHA.119.314793
Lambert, M., Capuano, V., Olschewski, A., Sabourin, J., Nagaraj, C., Girerd, B., et al. (2018). Ion channels in pulmonary hypertension: a therapeutic interest? Int. J. Mol. Sci. 19:3162. doi: 10.3390/ijms19103162
Le Ribeuz, H., Capuano, V., Girerd, B., Humbert, M., Montani, D., and Antigny, F. (2020). Implication of potassium channels in the pathophysiology of pulmonary arterial hypertension. Biomolecules 10:1261. doi: 10.3390/biom10091261
Lesage, F., and Barhanin, J. (2011). Molecular physiology of pH-sensitive background K2P channels. Physiology 26, 424–437.
Lesage, F., Guillemare, E., Fink, M., Duprat, F., Lazdunski, M., Romey, G., et al. (1996). TWIK-1, a ubiquitous human weakly inward rectifying K+ channel with a novel structure. EMBO J. 15, 1004–1011. doi: 10.1002/j.1460-2075.1996.tb00437.x
Lesage, F., Maingret, F., and Lazdunski, M. (2000). Cloning and expression of human TRAAK, a polyunsaturated fatty acids-activated and mechano-sensitive K(+) channel. FEBS Lett. 471, 137–140. doi: 10.1016/s0014-5793(00)01388-0
Liang, B., Soka, M., Christensen, A. H., Olesen, M. S., Larsen, A. P., Knop, F. K., et al. (2014). Genetic variation in the two-pore domain potassium channel, TASK-1, may contribute to an atrial substrate for arrhythmogenesis. J. Mol. Cell. Cardiol. 67, 69–76. doi: 10.1016/j.yjmcc.2013.12.014
Lien, S., Koop, B. F., Sandve, S. R., Miller, J. R., Kent, M. P., Nome, T., et al. (2016). The Atlantic salmon genome provides insights into rediploidization. Nature 533, 200–205. doi: 10.1038/nature17164
Limberg, S. H., Netter, M. F., Rolfes, C., Rinne, S., Schlichthorl, G., Zuzarte, M., et al. (2011). TASK-1 channels may modulate action potential duration of human atrial cardiomyocytes. Cell Physiol. Biochem. 28, 613–624. doi: 10.1159/000335757
Linden, A. M., Aller, M. I., Leppa, E., Vekovischeva, O., Aitta-Aho, T., Veale, E. L., et al. (2006). The in vivo contributions of TASK-1-containing channels to the actions of inhalation anesthetics, the a2 adrenergic sedative dexmedetomidine, and cannabinoid agonists. J. Pharmacol. Exp. Ther. 317, 615–626. doi: 10.1124/jpet.105.098525
Loganathan, K., Moriya, S., Sivalingam, M., Ng, K. W., and Parhar, I. S. (2017). Sequence and localization of kcnk10a in the brain of adult zebrafish (Danio rerio). J. Chem. Neuroanat. 86, 92–99. doi: 10.1016/j.jchemneu.2017.10.004
Ma, L., Roman-Campos, D., Austin, E. D., Eyries, M., Sampson, K. S., Soubrier, F., et al. (2013). A novel channelopathy in pulmonary arterial hypertension. N. Engl. J. Med. 369, 351–361.
Mathie, A. (2007). Neuronal two-pore-domain potassium channels and their regulation by G protein-coupled receptors. J. Physiol. 578, 377–385. doi: 10.1113/jphysiol.2006.121582
Meuth, S. G., Kleinschnitz, C., Broicher, T., Austinat, M., Braeuninger, S., Bittner, S., et al. (2009). The neuroprotective impact of the leak potassium channel TASK1 on stroke development in mice. Neurobiol. Dis. 33, 1–11. doi: 10.1016/j.nbd.2008.09.006
Mulkey, D. K., Talley, E. M., Stornetta, R. L., Siegel, A. R., West, G. H., Chen, X., et al. (2007). TASK channels determine pH sensitivity in select respiratory neurons but do not contribute to central respiratory chemosensitivity. J. Neurosci. 27, 14049–14058. doi: 10.1523/JNEUROSCI.4254-07.2007
Nasr, N., Faucherre, A., Borsotto, M., Heurteaux, C., Mazella, J., Jopling, C., et al. (2018). Identification and characterization of two zebrafish Twik related potassium channels, Kcnk2a and Kcnk2b. Sci. Rep. 8:15311. doi: 10.1038/s41598-018-33664-9
Olschewski, A., Li, Y., Tang, B., Hanze, J., Eul, B., Bohle, R. M., et al. (2006). Impact of TASK-1 in human pulmonary artery smooth muscle cells. Circ. Res. 98, 1072–1080. doi: 10.1161/01.RES.0000219677.12988.e9
Olschewski, A., Veale, E. L., Nagy, B. M., Nagaraj, C., Kwapiszewska, G., Antigny, F., et al. (2017). TASK-1 (KCNK3) channels in the lung: from cell biology to clinical implications. Eur. Respir. J. 50, 1700754. doi: 10.1183/13993003.00754-2017
Patel, A. J., Honore, E., Lesage, F., Fink, M., Romey, G., and Lazdunski, M. (1999). Inhalational anesthetics activate two-pore-domain background K+ channels. Nat. Neurosci. 2, 422–426. doi: 10.1038/8084
Pena-Munzenmayer, G., Niemeyer, M. I., Sepulveda, F. V., and Cid, L. P. (2014). Zebrafish and mouse TASK-2 K(+) channels are inhibited by increased CO2 and intracellular acidification. Pflugers Arch. 466, 1317–1327. doi: 10.1007/s00424-013-1365-2
Pisani, D. F., Beranger, G. E., Corinus, A., Giroud, M., Ghandour, R. A., Altirriba, J., et al. (2016). The K+ channel TASK1 modulates beta-adrenergic response in brown adipose tissue through the mineralocorticoid receptor pathway. FASEB J. 30, 909–922. doi: 10.1096/fj.15-277475
Rahm, A. K., Wiedmann, F., Gierten, J., Schmidt, C., Schweizer, P. A., Becker, R., et al. (2014). Functional characterization of zebrafish K2P18.1 (TRESK) two-pore-domain K+ channels. Naunyn Schmiedebergs Arch. Pharmacol. 387, 291–300. doi: 10.1007/s00210-013-0945-1
Schmitt, N., Grunnet, M., and Olesen, S. P. (2014). Cardiac potassium channel subtypes: new roles in repolarization and arrhythmia. Physiol. Rev. 94, 609–653. doi: 10.1152/physrev.00022.2013
Shin, J. T., and Fishman, M. C. (2002). From zebrafish to human: modular medical models. Annu. Rev. Genomics Hum. Genet. 3, 311–340. doi: 10.1146/annurev.genom.3.031402.131506
Skarsfeldt, M. A., Jepps, T. A., Bomholtz, S. H., Abildgaard, L., Sorensen, U. S., Gregers, E., et al. (2016). pH-dependent inhibition of K(2)P3.1 prolongs atrial refractoriness in whole hearts. Pflugers Arch. 468, 643–654. doi: 10.1007/s00424-015-1779-0
Staudacher, I., Seehausen, S., Gierten, J., Illg, C., Schweizer, P. A., Katus, H. A., et al. (2019). Cloning and characterization of zebrafish K2P13.1 (THIK-1) two-pore-domain K(+) channels. J. Mol. Cell. Cardiol. 126, 96–104. doi: 10.1016/j.yjmcc.2018.11.013
Talley, E. M., and Bayliss, D. A. (2002). Modulation of TASK-1 (Kcnk3) and TASK-3 (Kcnk9) potassium channels: volatile anesthetics and neurotransmitters share a molecular site of action. J. Biol. Chem. 277, 17733–17742. doi: 10.1074/jbc.M200502200
Wen, Z. Y., Bian, C., You, X., Zhang, X., Li, J., Zhan, Q., et al. (2020b). Characterization of two kcnk3 genes in Nile tilapia (Oreochromis niloticus): molecular cloning, tissue distribution, and transcriptional changes in various salinity of seawater. Genomics 112, 2213–2222. doi: 10.1016/j.ygeno.2019.12.017
Wen, Z., Li, Y., Bian, C., Shi, Q., and Li, Y. (2020a). Characterization of two kcnk3 genes in rabbitfish (Siganus canaliculatus): molecular cloning, distribution patterns and their potential roles in fatty acids metabolism and osmoregulation. Gen. Comp. Endocrinol. 296:113546. doi: 10.1016/j.ygcen.2020.113546
Wen, Z. Y., Qin, C. J., Wang, J., He, Y., Li, H. T., Li, R., et al. (2020c). Molecular characterization of two leptin genes and their transcriptional changes in response to fasting and refeeding in Northern snakehead (Channa argus). Gene 736:144420. doi: 10.1016/j.gene.2020.144420
Wen, Z. Y., Wang, J., Bian, C., Zhang, X., Li, J., Peng, Y., et al. (2019). Molecular cloning of two kcnk3 genes from the Northern snakehead (Channa argus) for quantification of their transcriptions in response to fasting and refeeding. Gen. Comp. Endocrinol. 281, 49–57. doi: 10.1016/j.ygcen.2019.05.016
Wettwer, E., Hala, O., Christ, T., Heubach, J. F., Dobrev, D., Knaut, M., et al. (2004). Role of IKur in controlling action potential shape and contractility in the human atrium: influence of chronic atrial fibrillation. Circulation 110, 2299–2306. doi: 10.1161/01.CIR.0000145155.60288.71
Keywords: potassium channel, kcnk3, osmoregulation, energy maintenance, fish
Citation: Wen Z-Y, Qin C-J, Lv Y-Y, Li Y-P, Zou Y-C, Guo S-T and Shi Q (2021) Homeostasis Regulation by Potassium Channel Subfamily K Member 3 (KCNK3) in Various Fishes. Front. Mar. Sci. 8:816861. doi: 10.3389/fmars.2021.816861
Received: 17 November 2021; Accepted: 29 November 2021;
Published: 14 December 2021.
Edited by:
Shengming Sun, Shanghai Ocean University, ChinaReviewed by:
Tiziano Verri, University of Salento, ItalyCopyright © 2021 Wen, Qin, Lv, Li, Zou, Guo and Shi. This is an open-access article distributed under the terms of the Creative Commons Attribution License (CC BY). The use, distribution or reproduction in other forums is permitted, provided the original author(s) and the copyright owner(s) are credited and that the original publication in this journal is cited, in accordance with accepted academic practice. No use, distribution or reproduction is permitted which does not comply with these terms.
*Correspondence: Zheng-Yong Wen, zhengyong_wen@126.com; Qiong Shi, shiqiong@genomics.cn