Effect of long-term manganese exposure on oxidative stress, liver damage and apoptosis in grouper Epinephelus moara ♀ × Epinephelus lanceolatus ♂
- 1College of Fisheries and Life Science, Shanghai Ocean University, Shanghai, China
- 2Key Laboratory for Sustainable Development of Marine Fisheries, Ministry of Agriculture, Qingdao Key Laboratory for Marine Fish Breeding and Biotechnology, Yellow Sea Fisheries Research Institute, Chinese Academy of Fishery Sciences, Qingdao, China
- 3Department of Marine Fish Culture, Yuhai Hongqi Ocean Engineering Co. LTD, Rizhao, China
Manganese is an indispensable trace element, however, it may be present at high concentrations in water and sediments of aquatic ecosystems due to natural and anthropogenic activities, and can interfere with physiological and biochemical mechanisms in fish. This study was conducted to determine the toxic effects associated with exposure to Mn2+ (0, 0.5, 1, 2, and 4 mg/L) for 30 d, regarding liver damage and apoptosis in Yunlong grouper (Epinephelus moara♀×E. lanceolatus♂). Expression of superoxide dismutase (sod) and catalase (cat) genes in the liver was significantly increased on days 10 and 20 following Mn2+ exposure (4 mg/L), but was reduced on day 30. Similarly, expression of glutathione peroxidase (gpx) and glutathione reductase (gr) genes was elevated after 10 d of exposure to 2 and 4 mg/L Mn2+, but decreased after 20 and 30 d. After 30 d of exposure to high concentrations (2 and 4 mg/L) of Mn2+, liver tissue showed hepatic sinusoidal gap congestion, dilatation, cell vacuolation, and necrosis. In addition, the activities of alanine aminotransferase (ALT) and aspartate transaminase (AST) as well as 8-hydroxydeoxyguanosine (8-OHdG) levels were significantly increased after Mn2+ exposure. Moreover, Mn2+ exposure altered the expression pattern of some pivotal genes associated to apoptosis (p53, bax, bcl-2, apaf-1, caspase-9, and caspase-3), which suggested that Mn2+ exposure induces apoptosis through the mitochondrial pathway. The above results showed that excessive Mn2+ induced apoptosis and liver damage in grouper through elicitation of oxidative stress. These insights help elucidate the mechanism by which Mn2+ induces toxicity in marine fish, and provide a new perspective regarding the detrimental effects of heavy metals in fish.
Introduction
Manganese, a crucial trace element for aquatic animals, plays an important role in maintaining normal physiological activities. However, it may cause abnormal changes in the physiology and behavior of aquatic animals (Howe et al., 2004) and even cause fatal and sublethal effects at excessive concentrations (Deng et al., 2019). An investigation on Mn2+ pollution found that the highest concentration of Mn2+ exceeding the standard could reach up to 1.74 mg/L in drinking water sources (Chen et al., 2011). In addition, in soft bottom sediments of the ocean, manganese may reach 80 mg/g on a dry weight basis, and eutrophication leads to hypoxia in many coastal areas where manganese is reduced and released as Mn2+ (Diaz and Rosenberg, 1995). Continuous exposure to Mn2+ results in its accumulation, causing undesirable physiological effects, such as oxidative stress and alterations in physiological parameters.
Manganese serves a crucial function with respect to antioxidant defense and activation of various enzymes (Hernroth et al., 2004). However, excess manganese is harmful to health and exerts toxic effects on the nervous system at high dosages. Liver cells contain numerous mitochondria where Mn2+ can accumulate in large quantities, disrupt oxidative phosphorylation, and increase the amount of reactive oxygen species (ROS) (Liu et al., 2013). Excessive accumulation of ROS can lead to an imbalance of redox state, thereby causing oxidative stress. Currently, oxidative stress is one of the recognized mechanisms of manganese toxicity, which can directly damage proteins, amino acids, and nucleic acids, and may result in cell structure damage or cell death (Nagalakshmi and Prasad, 1998; Atli and Canli, 2010; Chtourou et al., 2012). Zhang et al. (2003) found that exposure to Mn2+ caused mild liver stasis, vacuolar degeneration of hepatocytes, increased lipid peroxidation, and decreased antioxidant capacity in rats. Chronic exposure to Mn2+ may lead to its bioaccumulation in the liver which can cause liver damage (Awasthi et al., 2018a).
Heavy metal exposure leads to increased ROS levels, causing extensive DNA damage and apoptosis. Oxidative stress can induce apoptosis through mitochondria-dependent and death receptor-dependent pathways (Sinha et al., 2013). Apoptosis is a highly ordered physiological process, which is characterized by cell shrinkage, chromatin condensation, internucleosomal DNA fragmentation, and apoptotic body formation accompanied by the activation of a variety of intracellular proteases and endonucleases (Chandra et al., 2000; Yahiro et al., 2010; Dolka et al., 2016). It is controlled by several factors. For instance, BCL2-Associated X (bax) is one of the most important pro-apoptotic genes whereas B-cell lymphoma-2 (bcl-2) can inhibit apoptosis induced by various toxic factors (Gomez-Lazaro et al., 2007). Heavy metals cause apoptosis by decreasing bcl-2 expression and increasing bax, caspases, and p53 mRNA expression (Zhu et al., 2016). Excess cadmium upregulates p53, caspase-3, and caspase-9 expression, and causes apoptosis in the head kidney of Channa punctatus (Chen et al., 2021). Excessive lead inhibits bcl-2 but promotes bax and p53 expression, causing bax/bcl-2 imbalance and leading to liver apoptosis in mice (Xu et al., 2008).
Yunlong grouper (Epinephelus moara ♀ × E. lanceolatus ♂) is a new breed of grouper with good meat quality, fast growth, and high adaptability, making it suitable for large-scale intensive farming. However, due to the water pollution caused by improper discharge of industrial effluent and household sewage, Yunlong grouper is likely to be exposed to high concentrations of Mn2+ during aquaculture, which is detrimental to its growth. In contrast to other heavy metals, such as cadmium and lead, the toxic effects of Mn2+ are less studied, especially with respect to aquatic ecosystems. Therefore, it is important to examine the responses of fish to Mn2+ exposure. The liver is a crucial detoxification organ in fish; thus, it has been chosen to evaluate the potential pathways of Mn2+-induced oxidative stress, which causes tissue damage and apoptosis. To the best of our knowledge, this is the first study to evaluate the potential response mechanism to long-term exposure of Mn2+ based on oxidative stress, liver damage, and apoptosis responses after long-term exposure. This study may provide valuable insights to improve the understanding of mechanisms of liver injury and apoptosis due to Mn2+ exposure.
Materials and methods
Experimental animals
Yunlong grouper were purchased from the Yuhai Hongqi Ocean Engineering Co. LTD (Shandong, China) and were acclimated to experimental conditions in fish tanks (45 × 48 × 48 cm) for at least one week. The experimental environment was illuminated for 12 h. The seawater used for the experiments had a pH of 7.5–7.8, temperature of 25–27°C, salinity of 28–29 ppt, total ammonia of 6 ± 1.0 µg/L, and total nitrite of 0.05 ± 0.02 mg/L. Ammonia nitrogen and nitrite were determined using spectrophotometry. The fish tanks were oxygenated with air stones attached to an air compressor to maintain dissolved oxygen at 7–8 mg O2/L. Commercial fish diet (QiHao Biotechnology Co., Qingdao, China) was supplied four times per day at 2% body weight per day, and uneaten pellets were retrieved. All animal experiments were reviewed and approved by the Committee on the Ethics of Animal Experiments of Chinese Academy of Fishery Sciences.
Experimental design
The 96 h-LC50 of Mn2+ to groupers was determined according to our previous study (Wang et al., 2022). According to these results, we selected five concentrations of Mn2+ (0, 0.5, 1, 2, and 4 mg/L), with three replicate fish tanks per treatment, referring to the setting method of Sayadi et al. (2020). Fifty fish (initial body weight 0.44 ± 0.08 g) were placed in each tank containing 50 L exposure solution or seawater. The treatments were conducted for 30 d. The exposure solution was prepared using MnCl2-4H2O (AR, 99%) purchased from Yuanye Biotechnology Co. (Shanghai, China). The solutions in the fish tanks were exchanged daily. The concentrations of Mn2+ were assessed daily using the potassium periodate spectrophotometric method (Li and Xue, 2012).
Sample collection
Seven fish were collected from each tank at 0, 10, 20, and 30 d of exposure, and the samples were collected after anesthesia in tricaine methanesulfonate (MS-222, 45 mg/L, Sigma Diagnostics INS, St. Louis, MO, USA).
Three whole fish and the livers of three other fish were rinsed with saline to remove surface impurities, after which they were immediately frozen in liquid nitrogen and stored at −80°C for biochemical and qRT-PCR analyses. The liver of the remaining fish was fixed in Buin’s solution at room temperature for 24 h, as described previously (Gao et al., 2019), and was then rinsed with water and placed in 70% ethanol for histopathology.
Histopathology
The fixed tissues were dehydrated in 80%, 90%, 95%, and 100% alcohol and embedded in paraffin wax. A rotary microtome (Leica, Wetzlar, Germany) was used to produce 4-μm sections. Hematoxylin and eosin staining was performed before examination using an optical microscope (Olympus BX51, Olympus, Tokyo, Japan).
Biochemical analyses
Activities of alanine aminotransferase (ALT) and aspartate transaminase (AST) activities in the whole fish were determined using a commercial kit (Nanjing Jiancheng Bioengineering Institute, Nanjing, China) according to Salamat et al. (2017). 8-Hydroxydeoxyguanosine (8-OHdG) levels in the whole fish were measured using an ELISA kit (Nanjing Jiancheng Bioengineering Institute, Nanjing, China), according to the antibody-antigen-enzyme-antibody complex method. Absorbance was measured at 450 nm using a microplate reader (Rayto RT-6100), 8-OHdG levels were calculated from a standard curve and are expressed as ng/mgprot. Protein concentrations were measured at 595 nm using Coomassie blue staining.
RNA extraction, cDNA synthesis, and quantitative reverse-transcription PCR (qRT-PCR)
Expression of genes related to antioxidants and apoptosis in liver tissue was assessed using qRT-PCR. Total RNA was extracted from liver tissue using an Animal Total RNA Isolation Kit (Foregene, Chengdu, China), according to the manufacturer’s instructions. RNA concentration was determined using a Nanodrop 2000 device (Thermo Fisher Scientific, Shanghai), and RNA quality was assessed using 1% agarose gel electrophoresis.
Reverse transcription was performed according to the manufacturer of the Evo M-MLV kit from Accurate Biotechnology (Hunan) Co., Ltd. Genomic DNA was removed in a reaction containing 1 μg total RNA, 2 µL of 5 × gDNA Clean Reaction Mix, and RNase-free dH2O to a final volume of 10 μL, which was incubated at 42°C. Reverse transcription was performed in a total volume of 20 μL, including 10 µL of reaction solution from the first step, 4 µL of 5 × Evo M-MLV RT Reaction Mix, and 6 µL of RNase-free water, which was incubated at 37°C for 15 min and at 85°C for 5 sec.
Primers for amplifying cDNA of the genes β-actin, superoxide dismutase (sod), catalase(cat), glutathion peroxidase (gpx), glutathione (gr), p53, BCL-2 associated X(bax), B-Cell Lymphoma-2 (bcl-2), apoptotic peptidase activating factor-1 (apaf-1), caspase-9, and caspase-3 were designed according to gene sequences published on NCBI (Table 1; synthesized by Shanghai Sangon Biotech, Shanghai, China). Expression levels were assessed through qRT-PCR using TB Green Premix Ex Taq (Dalian Takara, China), and the reaction was performed on an Eppendorf Mastercycler ep realplex (Applied Biosystems, Foster City, CA, USA). The reaction comprised 2 µL of cDNA, 10 µL of TB Green Premix Ex Taq, 0.8 µL each of the forward and reverse primers (10 mM), and 7.2 µL of ddH2O. The reaction program was 95°C for 30 s, followed by 40 cycles at 95°C for 5 s and 60°C for 20 s. Relative gene expression levels were evaluated using the 2−ΔΔCT method (Livak and Schmittgen, 2001). For each tested gene, qRT-PCR was performed using three replicates.
Statistical analyses
Data were analyzed with SPSS software for Windows (Version 25.0, IBM, Chicago, IL, USA). The Kolmogorov-Smirnov test was used to test normal distribution, and Levine’s test to access variance homogeneity. Two-way ANOVA was used to test interaction effects of exposure time and Mn2+ concentrations. In case of significant differences, Tukey’s test was used post-hoc. Statistical significance is reported at P < 0.05.
Results
Expression of genes related to the antioxidant defense system
The responses of grouper to Mn2+ exposure with respect to sod, cat, gpx and gr mRNA levels are shown in Figure 1. There were significant effects of Mn2+ concentrations and exposure time on expression levels of antioxidant enzyme genes and significant interactions between Mn2+ concentrations and exposure time on expression of all antioxidant enzyme genes (P < 0.05). The expression levels of sod and cat were significantly increased on day 10 in the 2, and 4 mg/L treatment groups and on day 20 in the 1, 2, and 4 mg/L Mn2+ treatment groups, compared to the controls (P < 0.05). Expression of sod and cat was significantly higher in the 1 and 2 mg/L Mn2+ treatment groups than that in the controls after 30 d, however, it was significantly lower in the 4 mg/L treatment group.
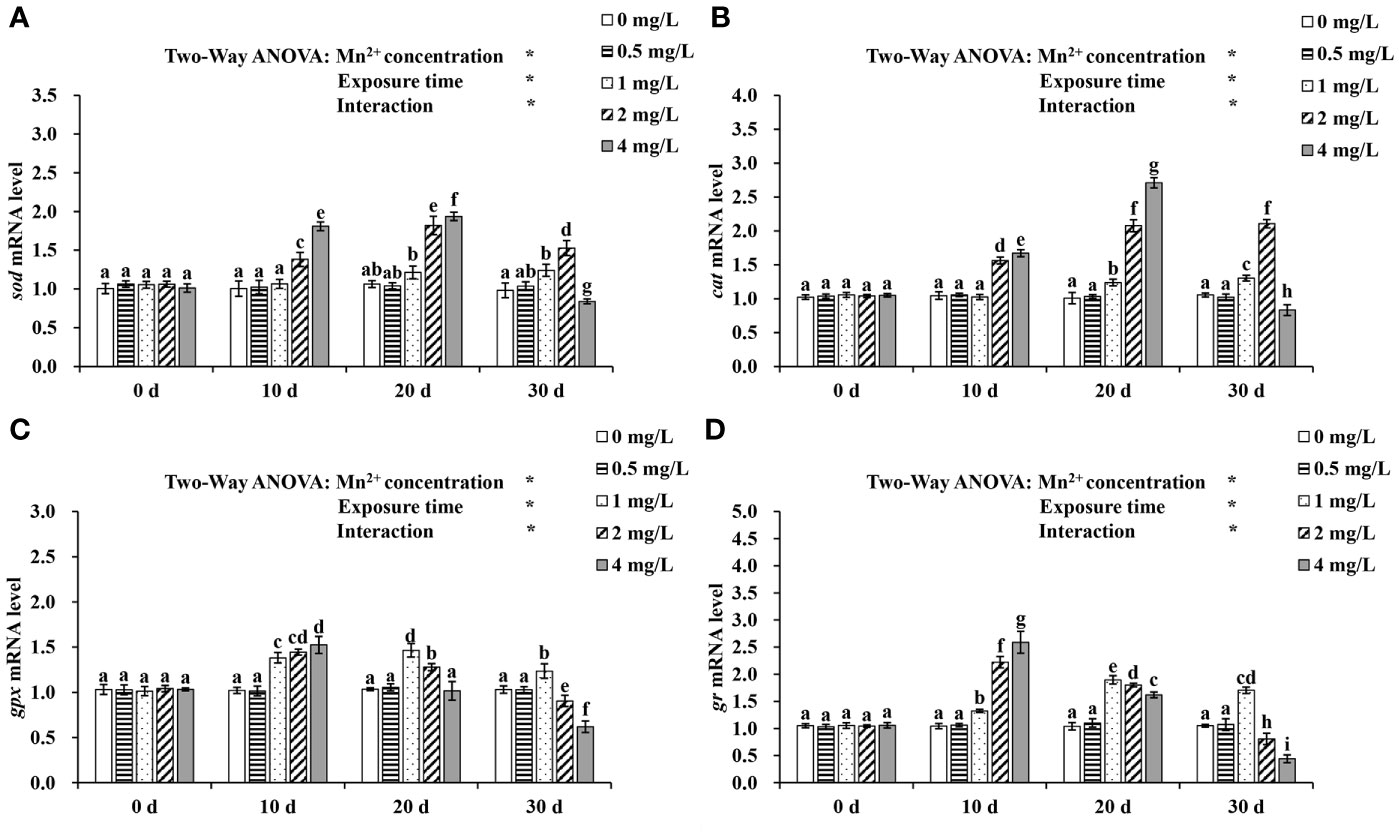
Figure 1 The mRNA expressions of (A) sod, (B) cat, (C) gpx and (D) gr at different concentrations of Mn2+ treatment for 0, 10, 20, and 30 days. Values are expressed as mean ± SD (n = 9), Significant differences (P < 0.05) between groups are indicated by different lowercase letters. *p < 0.05. sod, superoxide dismutase; cat, catalase; gpx, glutathione peroxidase; gr: glutathione reductase.
On days 10 and 20, a pronounced increase in gpx and gr expression was observed in the 1, 2, and 4 mg/L Mn2+ treatment groups. Moreover, fish exposed to 1 mg/L Mn2+ for 30 d showed significantly increased gpx and gr expression, which was, however, significantly decreased in the 2 and 4 mg/L Mn2+ treatment groups.
Liver histopathology
Differences in liver tissue structure between treatments are shown in Figure 2. The livers of the controls and the 0.5 mg/L Mn2+ group showed normal tissue structure, dense cytoplasm, clear hepatic platelet structure, and rounded central vein cross-sections (Figure 2A, B). However, exposure to higher Mn2+ concentrations for 30 d resulted in dilated hepatic sinusoidal spaces and scattered cell arrangement in some areas in the 1 mg/L group (Figure 2C); irregularly shaped central venous cross-sections and congested hepatic sinusoidal spaces in the 2 mg/L group (Figure 2D); and vacuole-like appearance, blurred cell gaps, and cell necrosis, in addition to the aforementioned anomalies, in the 4 mg/L group (Figure 2E).
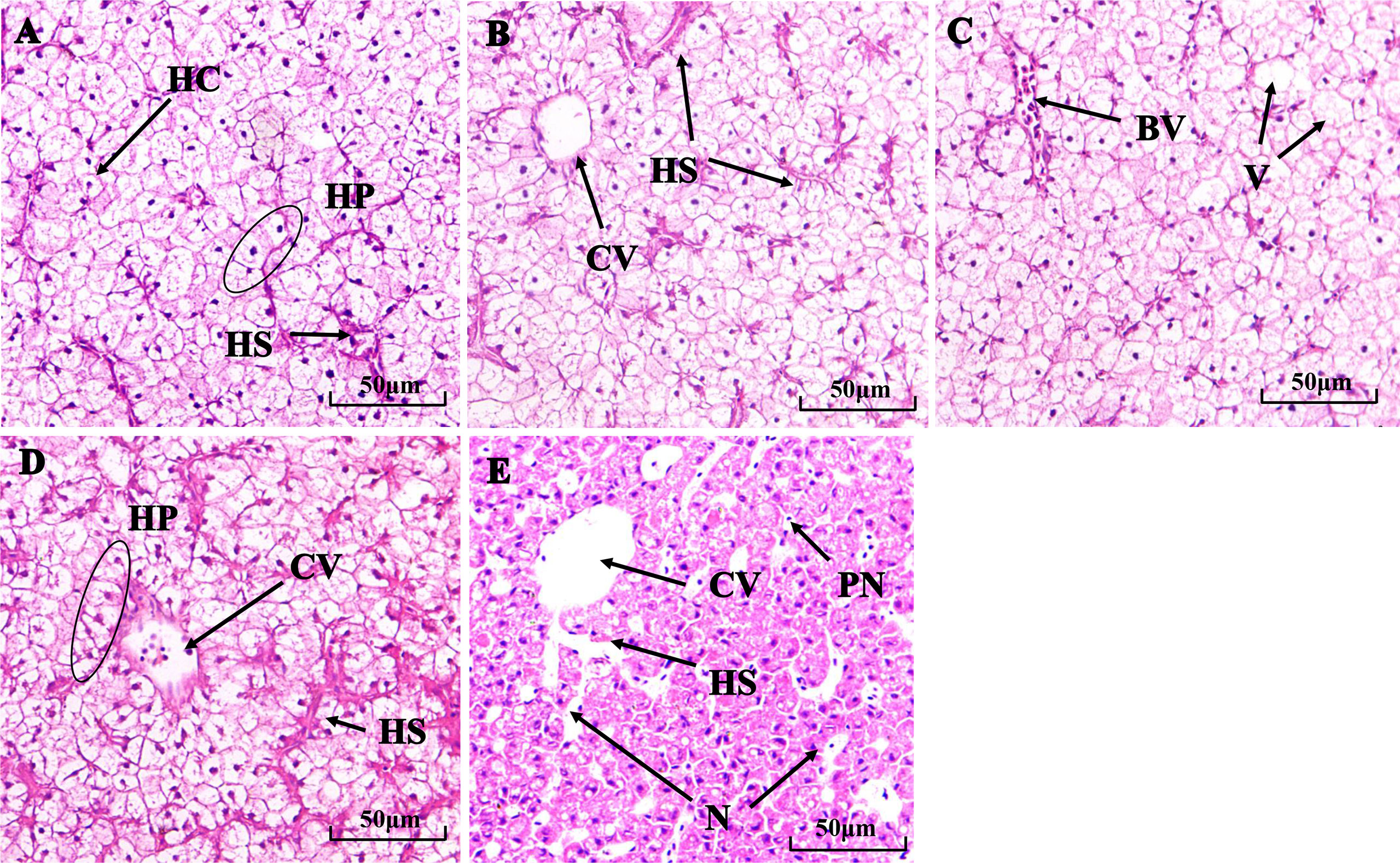
Figure 2 Histological structure of fish livers after 30 d in the (A) control group, (B) 0.5 mg/L Mn2+, (C) 1 mg/L Mn2+, (D) 2 mg/L Mn2+, (E) 4 mg/L Mn2+ treatments. HC:, Hepatic cell; HS, Hepatic sinusoid; HP, Hepatic plate; HM, Hepatic macrophage; CV, Central vena; PN, Pycnose; V, Vacuolization; N, necrocytosis. (H & E, ×200).
ALT and AST activity
Changes in ALT and AST activity at various stages during 30 d of Mn2+ exposure are shown in Figure 3. Mn2+ concentrations and exposure time have significant effects on ALT and AST activities (P < 0.05). From day 10 to 30, the activities of ALT and AST in the 1, 2, and 4 mg/L treatment groups were increasing continuously and were significantly higher than those in the control group (P < 0.05).
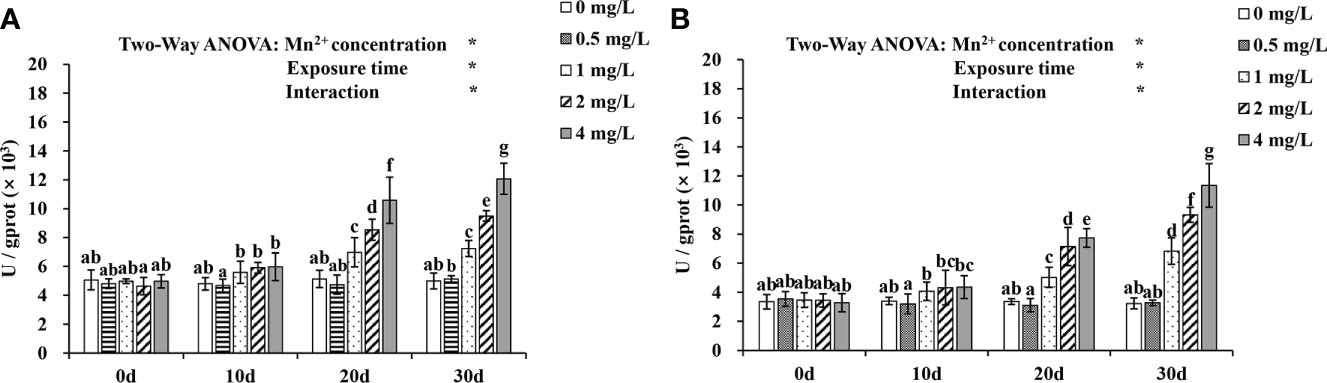
Figure 3 The (A) ALT and (B) AST variations during 30 d when exposed to various Mn2+ concentrations. Values are expressed as mean ± SD (n = 9), Significant differences (P < 0.05) between groups are indicated by different lowercase letters.*p < 0.05. ALT, alanine transaminase; AST, aspartate transaminase.
8-OHdG levels
Changes in 8-OhdG levels are shown in Figure 4. There were significant effects of Mn2+ concentrations and exposure time on 8-OHdG levels and significant interactions between Mn2+ concentrations and exposure time (P < 0.05). Over time, 8-OhdG levels increased significantly in the 1, 2, and 4 mg/L Mn2+ treatment groups (P < 0.05), and the increase was highest in the 4 mg/L Mn2+ treatment on day 20.
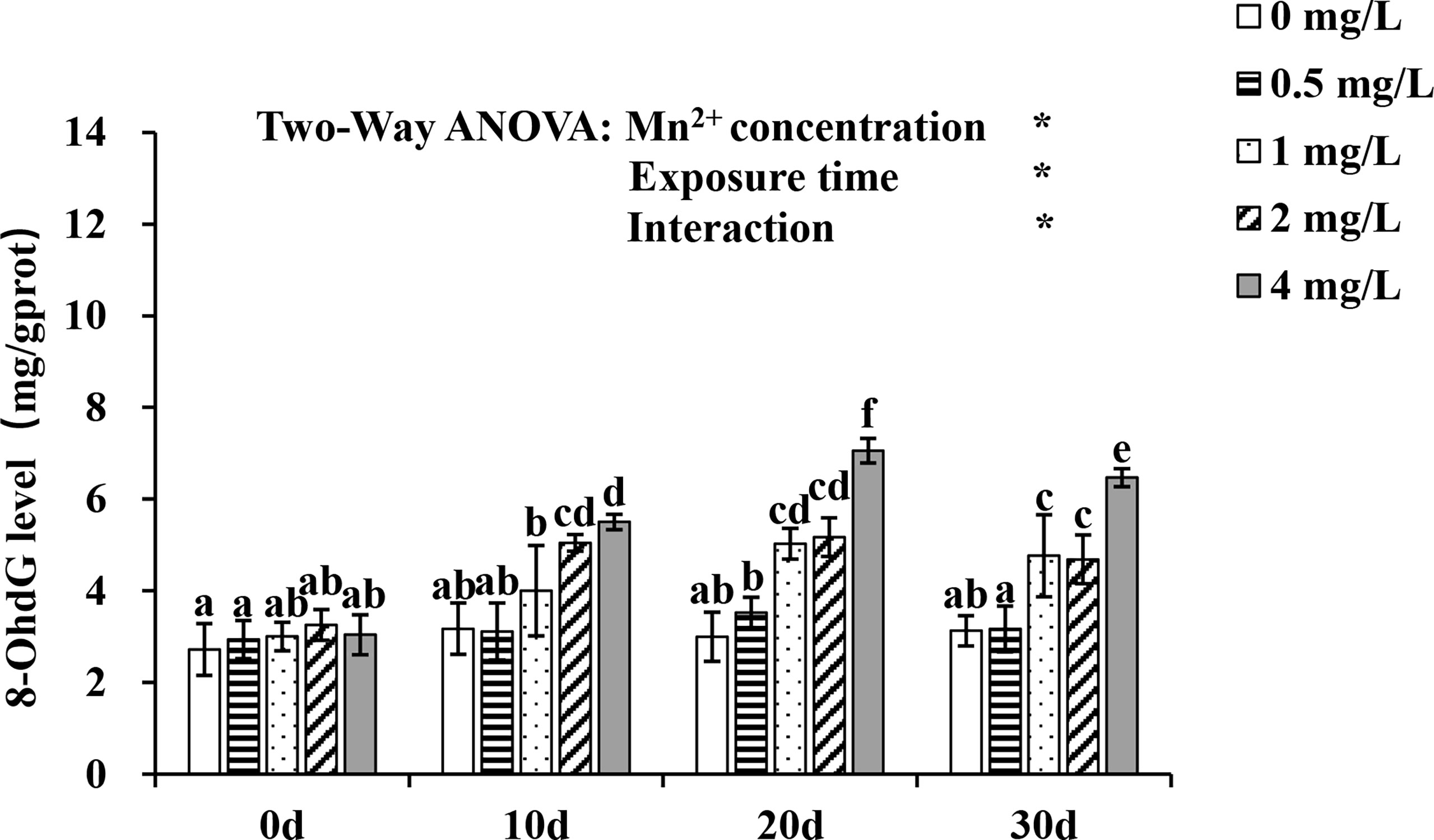
Figure 4 Change of 8-OHdG level exposed to different concentrations of Mn2+ over 30 d. Values are expressed as mean ± SD (n = 9), Significant differences (P < 0.05) between groups are indicated by different lowercase letters.*p < 0.05. 8-OHdG: 8-hydroxy-2 deoxyguanosine.
Expression of apoptosis-associated genes
Effects of Mn2+ exposure on mRNA levels of apoptosis-related genes are shown in Figure 5. Mn2+ concentration and exposure time have a significant interaction effect on the expression of apoptosis-associated genes (P < 0.05). On day 10 of Mn2+ exposure, p53 expression was elevated in the 2 mg/L group, and p53, bax, and caspase-9 expression was significantly higher in the liver of 4 mg/L treatment than in the controls (P < 0.05). On day 20, p53, bax, and caspase-3 expression was increased but bcl-2 expression was decreased in the 1, 2 and 4 mg/L groups, compared to the control group. In addition, apaf-1 and caspase-9 expression was significantly higher in the 2 and 4 mg/L groups. On day 30, the expression of p53, bax, caspase-3, and caspase-9 was upregulated in the 1, 2, and 4 mg/L treatment groups compared to the control group, whereas bcl-2 expression was downregulated. Moreover, apaf-1 expression was significantly higher in the 2 and 4 mg/L groups.
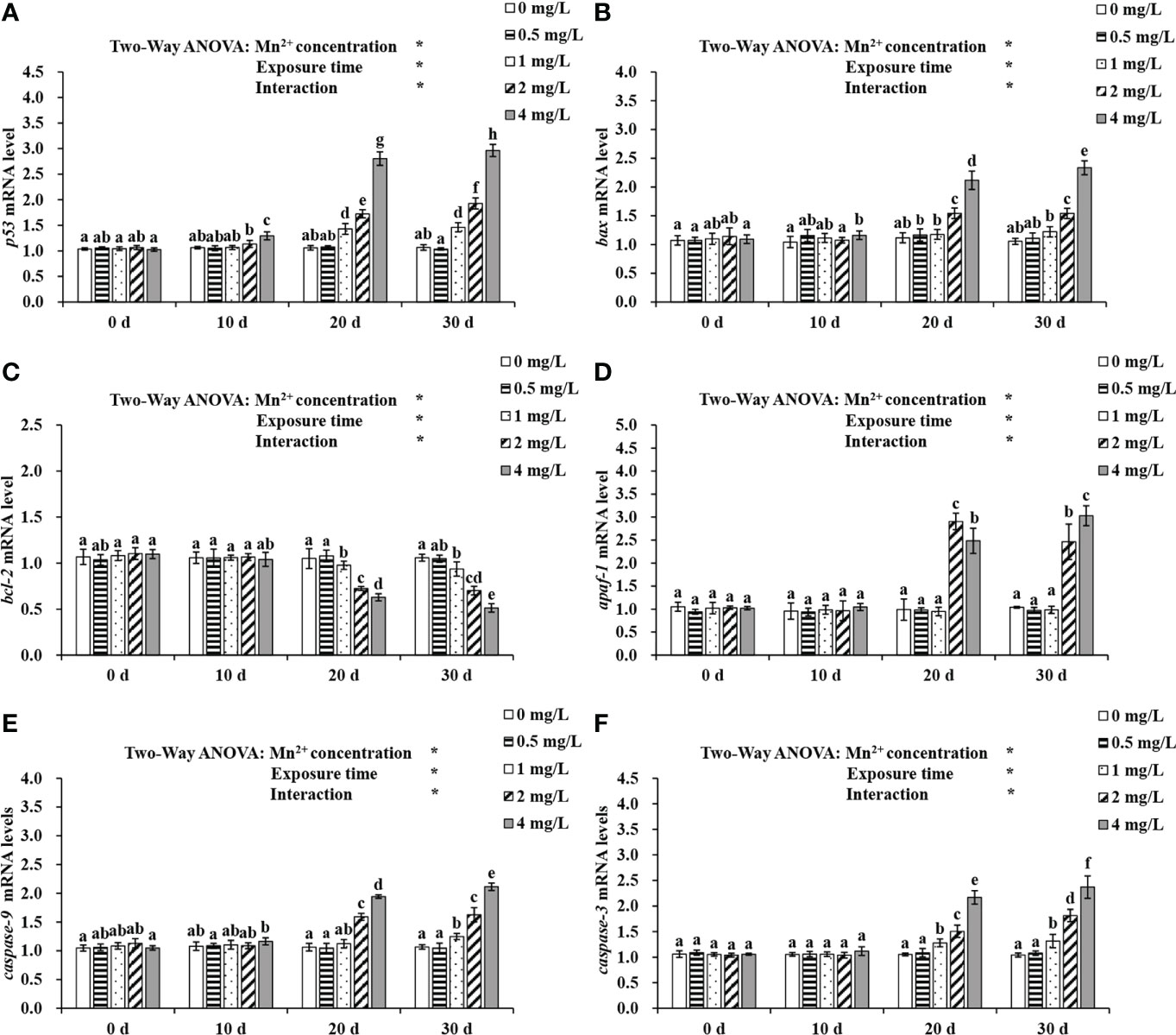
Figure 5 Expression of (A) p53, (B) bax, (C) bcl-2, (D) apaf-1, (E) caspase-9 and (F) caspase-3 exposed to different concentrations of Mn2+ over 30 d. Values are expressed as mean ± SD (n = 9), Significant differences (P < 0.05) between groups are indicated by different lowercase letters.*p < 0.05. p53, tumor suppressor protein p53; bax, bc12-associated X; bcl-2: B-cell lymphoma-2; apaf-1: apoptotic protease activating factor-1.
Discussion
Effects of Mn2+ exposure on oxidative stress
With accelerating industrial progress, increasing amounts of Mn2+ are discharged into rivers, lakes, and oceans. Thus Mn2+ is enriched at considerable quantities in aquatic animals, which can exert toxic effects. Antioxidant defense systems help prevent excessive ROS levels by maintaining a dynamic balance between free radical production and scavenging (Bhansali et al., 2017). Antioxidant enzymes including SOD, CAT, GPx, and GR can help defend against oxidative damage and play a crucial role in maintaining ROS homeostasis (Jia et al., 2019). SOD removes ROS from the organism and converts O2- to hydrogen peroxide (H2O2). Subsequently, CAT converts H2O2 to H2O and O2 (Mai et al., 2010; Liu et al. 2013). Glutathione (GSH) is an antioxidant that protects the -SH protein group from oxidation and the activity of sulfhydryl proteins and enzymes. GSH can also reduce H2O2 to H2O with the participation of GPx, thus eliminating H2O2 and reducing its toxic effects. GSH is oxidized to oxidized glutathione (GSSG), which is catalyzed by GR to generate GSH to maintain cellular redox homeostasis (Storey, 1996; Jin et al., 2008; Wei et al., 2018). Previous studies have shown that heavy metal exposure induces ROS formation and alters the expression of antioxidant enzymes (Awasthi et al., 2018b; Zhao L. et al., 2020). Liu et al. (2013) reported that the activities of SOD and GPx in cocks decreased along with increasing long-term exposure to dietary Mn2+. Awasthi and Ratn et al. (2018) showed that, chronic exposure to Cr6+ at 5% and 10% of the 96 h-LC50 resulted in the upregulation of sod and cat genes, increasing SOD and CAT activities and eliminating high levels of ROS. Ren et al. (2019) found that the transcription of cat, gpx, and gr was upregulated in flounder larvae at 10.0 μg/L MeHg and confirmed that such gene transcriptional up-regulation usually occurred in parallel with the enhancement of the corresponding antioxidants. Similarly, in the current study, we observed that the expression levels of antioxidant-related genes in grouper increased on days 10 and 20 of Mn2+ exposure at 1, 2, and 4 mg/L, but decreased at 30 d with respect to sod and cat in the 4 mg/L group and gpx and gr in the 2 and 4 mg/L groups. Increasing the expression of genes related to antioxidants can lead to a decrease in ROS levels in response to oxidative stress, thereby protecting cells from oxidative damage. In addition, according to Zhao L. L. et al. (2020), largemouth bass (Micropterus salmoides) antioxidant enzyme-related genes were elevated after a first exposure to hypoxia and ammonia, and then gradually decreased with increasing exposure time. It was also observed in the present study that fish subjected to high Mn2+ concentrations for long periods of time showed lower expression of genes producing antioxidants, probably because the antioxidant system may not be able to sufficiently eliminate or neutralize excess ROS, and severe oxidative damage may occur.
Effects of Mn2+ exposure on liver damage
Heavy metals may enter the digestive tract and respiratory organs of exposed fish, be distributed throughout the entire body via the blood circulatory system, and eventually deposited in the liver, which is an important detoxification organ (Jang et al., 2014; Vali et al., 2020). We examined the histopathological status of fish livers to further assess the toxic potential of Mn2+. Histopathological results showed that low concentration of Mn2+ (1 mg/L) induced hyperemia in hepatic sinusoidal spaces and some cytoplasmic vacuolation. Moreover, nuclear pyknosis, blurred cell boundaries, enlarged hepatic sinusoids and cell necrosis were observed in the livers of fish treated with higher concentrations of Mn2+ (2 and 4 mg/L). Exposure to toxins increases blood flow to the liver, a process that elicits sinus congestion and hepatic sinus dilation to ensure the detoxification activity of liver cells (Hued et al., 2012). Ratn et al. (2018) reported histological changes in the liver after zinc exposure and observed vacuolization and pyknosis at low concentrations and cell necrosis, inflammation and hypertrophic at high concentrations. Similar histopathological changes, such as hemosiderosis, hemorrhage, hydropic swelling, and pyknotic nuclei, occurred in livers of goldfish exposed to silver nanoparticles and silver salt in a study by Abarghouei et al. (2016). ALT and AST, which are are widely used as clinical indicators for hepatotoxicity assessment, accumulated in the liver (Ross, 2010; Yan et al., 2021). In the study of Zhao et al. (2019), both ALT and AST levels in supernatant of zebrafish larvae homogenate were significantly up-regulated (P<0.05) compared with the control, which indicated that the liver was one of the major toxic target organs of Euphorbia kansui. Similarly, the results of a study by Abedi et al. (2013) showed that exposure to different metals significantly (P < 0.05) increased AST levels and ALT activity. Also, the results of the current investigation showed that 1, 2, and 4 mg/L Mn2+ groups had consistently greater ALT and AST activities than the control group. These results were in line with previous studies that reported lead exposure significantly elevated ALT and AST activities causing tissue damage and impaired metabolism (Atli et al., 2015). Pyrazinamide treatment doses of 2.5 mM and 5 mM at 72 hpe progressively decreased liver size and increased larvae transaminases, suggestive of hepatocyte death and consistent with the histological analysis (Zhang et al., 2016). Therefore, enzymatic alterations found in the present study may reflect the imbalance of fish physiology which was also induced liver damage of grouper.
Effects of Mn2+ exposure on DNA damage
Once oxidative stress exceeds the homeostatic potential of the antioxidant defense system, oxidative damage is initiated. Approximately 2 × 104 DNA damage events have been estimated to occur in every cell daily, and a significant proportion of the damage is caused by ROS (Barzilai and Yamamoto, 2004). Excess ROS causes damage to cellular macromolecules, including DNA (Ari et al., 2002). The oxidative modification of deoxyguanosine to 8-OHdG in mtDNA is the major DNA lesion induced by oxidative stress and is considered as an index of DNA damage (Lu et al., 2001). 8-OHdG, the main product of ROS-induced DNA damage, is extensively used as an indicator of DNA damage as it increases during oxidative stress. Alak et al. (2019) found that copper exposure caused DNA damage in liver and gill tissues of rainbow trout, as evidenced by significantly increased levels of 8-OHdG. Meanwhile, in a study about H2O2, 8-OHdG levels increased in H2O2 (500 μM)-treated cells, indicating DNA damage. Similarly, in the current study, 8-OhdG levels in grouper livers were significantly increased in the 1, 2, and 4 mg/L Mn2+ treatments, indicating DNA damage. These data demonstrate that Mn2+-induced oxidative stress evoked DNA damage, as previously observed in a study on mice (Seiler et al., 2001) showing that 8-OHdG levels in the lungs were significantly increased after quartz exposure above 0.3 mg/day, and this trend was dose- and time-dependent.
Effects of Mn2+ exposure on apoptosis
Apoptosis plays a vital role in the morphogenesis of homeostasis within tissues and organs during development (Capriello et al., 2021). However, exposure to other metals, including cadmium (Chen et al., 2021) and copper (Nagalakshmi and Prasad, 1998), may change this biological mechanism, thereby reducing the organism’s fitness. Generally, p53 plays an important role in apoptosis, and stress signals resulting from the accumulation of ROS, especially H2O2, affect multiple cellular pathways. In addition, oxidative stress-induced DNA damage increases the mRNA levels of p53, and prolonged and sustained activation of p53 can upregulate the expression level of bax and downregulate the expression of bcl-2 together with the promotion of apoptosis (Whiteman et al., 2007; Deng et al., 2009). Choudhury et al. (2020) found that activated p53 in cadmium-exposed fish stimulated bax, leading to a bax/bcl-2 imbalance and ultimately to apoptosis. In the present study, p53 expression in the liver was upregulated following exposure to 1, 2, and 4 mg/L Mn2+ for 20 and 30 d. In addition, the gene expression levels of bax were significantly upregulated with increasing exposure concentration and time, whereas the gene expression levels of bcl-2 were significantly decreased. This suggests that high concentrations of Mn2+ can trigger apoptosis through the P53-Bax-Bcl2 pathway, which is also reflected in the gene expression levels of caspases. Apaf-1, caspase-3, and caspase-9 are key genes in the mitochondrial pathway: apaf-1 activates caspase-9, which in turn activates caspase-3, and changes in their expression levels lead to apoptosis (Fulda and Debatin, 2006; Sakr et al., 2015). The observed upregulation of apaf-1, caspase-9, and caspase-3 in fish exposed to Mn2+ for 30 d in this study confirmed apoptosis of hepatocytes. This phenomenon may be due to excessive ROS production induced by high doses of Mn2+. Awasthi and Ratn et al. (2018) also demonstrated that Cr6+-induced oxidative stress leads to apoptosis in hepatocytes by transcriptional upregulation of bax, apaf-1, and casp3a. Thus, the differential transcriptional expression of these genes suggests that Mn2+-induced apoptosis occurs through activation of the mitochondria-dependent apoptotic pathway.
Conclusion
We investigated the molecular biomarkers related to oxidative stress, liver damage, and apoptosis in grouper after Mn2+ exposure. The increased expression of four major antioxidant-related genes (sod, cat, gpx, and gr) clearly indicated the induction of oxidative stress due to Mn2+ accumulation in grouper livers. However, this increased antioxidant capacity was not sufficient to rescue cells from oxidative stress, and these genes were downregulated on day 30.Thus, long-term exposure to higher Mn2+ concentrations caused oxidative damage and subsequently liver damage, such as hyperemia, cytoplasmic vacuolation, nuclear pyknosis, blurred cell boundaries, hepatic sinusoids, and cell necrosis. The elevated levels of ALT, AST, and 8-OHdG in the whole fish further demonstrated the damage caused by Mn2+ exposure. In addition, we confirmed the transcriptional regulation of apoptosis-related genes p53, bax, bcl-2, apaf-1, caspase-9, and caspase-3 by Mn2+, which indicated apoptosis in grouper liver cells through the mitochondrial pathway. Importantly, the oxidative stress, liver damage, DNA damage, and apoptosis caused by Mn2+ exposure exhibited a dose and time-dependence difference. However, little is known about the mechanisms elicited by this metal, and more research is required to fully understand its possible effects on grouper.
Data availability statement
The datasets presented in this study can be found in online repositories. The datasets generated for this study are freely available in Figshare, DOI: https://doi.org/10.6084/m9.figshare.20300997.v1.
Ethics statement
The animal study was reviewed and approved by the Ethics of Animal Experiments of Chinese Academy of Fishery Sciences.
Author contributions
BL, XG: designed study and manuscript revision. XW, YF, KZ, and FW: experimental operation and sampling. XZ, SC: analyzed data analysis. XW: drafted manuscript. All authors contributed to the article and approved the submitted version.
Funding
This study was supported by the National Key R&D Program of China (No. 2019YFD0900503), Central Public-interest Scientific Institution Basal Research Fund, YSFRI, CAFS (No. 20603022021007), Central Public-interest Scientific Institution Basal Research Fund, CAFS (No. 2020TD49), and China Agriculture Research System for Marine Fish Culture Industry (CARS-47).
Acknowledgments
We thank the Yuhai Hongqi Ocean Engineering Co. LTD for providing the samples used in this study and the Yellow Sea Fisheries Research Institute for their excellent technical assistance.
Conflict of interest
Author KZ and FW are employed by Yuhai Hongqi Ocean Engineering Co. LTD. The remaining authors declare that the research was conducted in the absence of any commercial or financial relationships that could be construed as a potential conflict of interest.
Publisher’s note
All claims expressed in this article are solely those of the authors and do not necessarily represent those of their affiliated organizations, or those of the publisher, the editors and the reviewers. Any product that may be evaluated in this article, or claim that may be made by its manufacturer, is not guaranteed or endorsed by the publisher.
References
Abarghoei S., Hedayati A., Ghorbani R., Miandareh H. K., Bagheri T. (2016). Histopathological effects of waterborne silver nanoparticles and silver salt on the gills and liver of goldfish carassius auratus. Int. J. Environ. Sci. Technol. 13 (7), 1753–1760. doi: 10.1007/s13762-016-0972-9
Abedi Z., Hasantabar F., Mohammad A., Khalesi K., Babaei S. (2013). Effect of sublethal concentrations of cadmium, lead and chromium on some enzymatic activities of common carp; cyprinus carpio. World J. Zool. 8 (1), 98–105. doi: 10.5829/idosi.wjz.2013.8.1.7197
Alak G., Parlak V., Yeltekin A.Ç., Ucar A., Çomaklı S., Topal A., et al. (2019). The protective effect exerted by dietary borax on toxicity metabolism in rainbow trout (Oncorhynchus mykiss) tissues. Comp. Biochem. Physiol. C Toxicol. Pharmacol. 216, 82–92. doi: 10.1016/j.cbpc.2018.10.005
Ari B., Galit R., Yosef S. (2002). ATM Deficiency and oxidative stress: a new dimension of defective response to DNA damage. DNA Repair (Amst.) 1 (1), 3–25. doi: 10.1016/S1568-7864(01)00007-6
Atli G., Ariyurek S. Y., Kanak E. G., Canli M. (2015). Alterations in the serum biomarkers belonging to different metabolic systems of fish (Oreochromis niloticus) after cd and Pb exposures.”. Environ. Toxicol. Pharmacol. 40 (2), 508–515. doi: 10.1016/j.etap.2015.08.001
Atli G., Canli M. (2010). Response of antioxidant system of freshwater fish oreochromis niloticus to acute and chronic metal (Cd, Cu, cr, zn, fe) exposures. Ecotoxicol. Environ. Saf. 73 (8), 1884–1889. doi: 10.1016/j.ecoenv.2010.09.005
Awasthi Y., Ratn A., Prasad R., Kumar M., Trivedi S. P. (2018a). An in vivo analysis of cr 6+ induced biochemical, genotoxicological and transcriptional profiling of genes related to oxidative stress, DNA damage and apoptosis in liver of fish, channa punctatus (Bloc). Aquat. Toxicol. 200, 158–167. doi: 10.1016/j.aquatox.2018.05.001
Awasthi Y., Ratn A., Prasad R., Kumar M., Trivedi S. P. (2018b). An in vivo analysis of cr 6+ induced biochemical, genotoxicological and transcriptional profiling of genes related to oxidative stress, DNA damage and apoptosis in liver of fish, channa punctatus (Bloc). Aquat. Toxicol. 200, 158–167. doi: 10.1016/j.aquatox.2018.05.001
Barzilai A., Yamamoto K.-I. (2004). DNA Damage responses to oxidative stress. DNA Repair 3, 1109–1115. doi: 10.1016/j.dnarep.2004.03.002
Bhansali S., Bhansali A., Dhawan V. (2017). Favourable metabolic profile sustains mitophagy and prevents metabolic abnormalities in metabolically healthy obese individuals. Diabetol. Metab. Syndr. 9 (1), 1–10. doi: 10.1186/s13098-017-0298-x
Capriello T., Monteiro S. M., Félix L. M., Donizetti A., Aliperti V., Ferrandino I. (2021). Apoptosis, oxidative stress and genotoxicity in developing zebrafish after aluminium exposure. Aquat. Toxicol. 236, 105872. doi: 10.1016/j.aquatox.2021.105872
Chandra J., Samali A., Orrenius S. (2000). Triggering and modulation of apoptosis by oxidative stress. Free Radic. Biol. Med. 29 (3-4), 323–333. doi: 10.1016/S0891-5849(00)00302-6
Chen J., Chen D., Li J., Liu Y., Gu X., Teng X. (2021). Cadmium-induced oxidative stress and immunosuppression mediated mitochondrial apoptosis via JNK-FoxO3a-PUMA pathway in common carp (Cyprinus carpio l.) gills. Aquat. Toxicol. 233, 105775. doi: 10.1016/j.aquatox.2021.105775
Chen X., Shao W., Song R., Wen X.. (2011). Discussion on the characteristics and causes of iron and manganese pollution in drinking water sources in zhoushan, zhejiang province. Earth Environ. 39 (2), 7. doi: 10.14050/j.cnki.1672-9250.2011.02.007
Choudhury C., Mazumder R., Kumar R., Dhar B., Sengupta M. (2020). Cadmium induced oxystress alters Nrf2-Keap1 signaling and triggers apoptosis in piscine head kidney macrophages. Aquat. Toxicol. 231, 105739. doi: 10.1016/j.aquatox.2020.105739
Chtourou Y., Fetoui H., Garoui E. M., Boudawara T., Zeghal N. (2012). Improvement of cerebellum redox states and cholinergic functions contribute to the beneficial effects of silymarin against manganese-induced neurotoxicity. Neurochem. Res. 37 (3), 469–479. doi: 10.1007/s11064-011-0632-x
Deng L. F., Huang T. L., Li N., Li K., Lü X. L., Mao X. J. (2019). Migration characteristics of manganese during Rainfall events and its impacts on water quality in a drinking water source reservoir. Huan Jing Ke Xue. 40 (6), 2722–2729. doi: 10.13227/j.hjkx.201810199
Deng J., Yu L., Liu C., Yu K., Shi X., Yeung L. W., et al. (2009). Hexbromocyclododecane-induced developmental toxicity and apoptosis in zebra fish embryos. Aquat. Toxicol. 93 (1), 29–36. doi: 10.1016/j.aquatox.2009.03.001
Diaz R. J., Rosenberg R. (1995). Marine benthic hypoxia: A review of its ecological effects and the behavioural response of benthic macrofauna. Oceanogr. Mar. Biol. 33, 245–303.
Dolka I., Król M., Sapierzyński R. (2016). Evaluation of apoptosis-associated protein (Bcl-2, bax, cleaved caspase-3 and p53) expression in canine mammary tumors: An immunohistochemical and prognostic study. Res. Vet. Sci. 105, 124–133. doi: 10.1016/j.rvsc.2016.02.004
Fetoui H., Gdoura R. (2012). Synthetic pyrethroid increases lipid and protein oxidation and induces glutathione depletion in the cerebellum of adult rats: Ameliorative effect of vitamin C. Hum. Exp. Toxicol. 31 (11), 1151–1160. doi: 10.1177/0960327112444478
Fulda S., Debatin K. M. (2006). Extrinsic versus intrinsic apoptosis pathways in anticancer chemotherapy. Oncogene. 25 (34), 4798–4811. doi: 10.1038/sj.onc.1209608
Gao X. Q., Fei F., Huo H. H., Huang B., Meng X. S., Zhang T., et al. (2019). Exposure to nitrite alters thyroid hormone levels and morphology in takifugu rubripes. Comp. Biochem. Physiol. C Toxicol. Pharmacol. 225, 108578. doi: 10.1016/j.cbpc.2019.108578
Gomez-Lazaro M., Galindo M. F., Melero-Fernandez de Mera R. M., Fernandez-Go’mez F. J., Concannon C. G., Segura M. F., et al. (2007). Reactive Oxygen Species and p38 Mitogen-Activated Protein Kinase Activate Bax to Induce Mitochondrial Cytochrome c Release and Apoptosis in Response to Malonate. Mol. Pharmacol. 71 (3), 736. doi: 10.1124/mol.106.030718
Hernroth B., Baden S. P., Holm K., André T., Söderhäll I. (2004). Manganese induced immune suppression of the lobster, nephrops norvegicus. Aquat. Toxicol. 70:3, 223–231. doi: 10.1016/j.aquatox.2004.09.004
Howe P., Malcolm H., Dobson S. (2004). Manganese and its compounds: environmental aspects (Geneva: World Health Organization), Vol. 63 (6), 737–745.
Hued A. C., Oberhofer S., de los Ángeles Bistoni M. (2012). Exposure to a commercial glyphosate formulation (Roundup) alters normal gill and liver histology and affects male sexual activity of jenynsia multidentata (Anablepidae, cyprinodontiformes). Arch. Environ. Contam. Toxicol. 62 (1), 107–117. doi: 10.1007/s00244-011-9686-7
Jang M. H., Kim W. K., Lee S. K., Henry T. B., Park J. W. (2014). Uptake, tissue distribution, and depuration of total silver in common carp (Cyprinus carpio) after aqueous exposure to silver nanoparticles. Environ. Sci. Technol. 48 (19), 11568–11574. doi: 10.1021/es5022813
Jia Y., Jing Q., Zhai J., Guan C., Huang B. (2019). Alternations in oxidative stress, apoptosis, and innate-immune gene expression at mRNA levels in subadult tiger puffer (Takifugu rubripes) under two different rearing systems. Fish Shellfish Immunol. 92, 756–764. doi: 10.1016/j.fsi.2019.07.016Shirley Landicho Ocampo
Jin X., Lian L. J., Wu C, Wang X-f, Fu W-y, Xu L-h. (2008). Lead induces oxidative stress, DNA damage and alteration of p53, Bax and Bcl-2 expressions in mice. Chem. Toxicol. 46 (5), 1488–94. doi: 10.1016/j.fct.2007.12.016
Liu X. F., Zhang L. M., Guan H. N., Zhang Z. W., Xu S. W. (2013). Effects of oxidative stress on apoptosis in manganese-induced testicular toxicity in cocks. Food Chem. Toxicol. 60, 168–176. doi: 10.1016/j.fct.2013.07.058
Livak K., Schmittgen T. (2001). Analysis of relative gene expression data using real-time quantitative PCR and the 2 (–delta delta C(T)) method. Methods-A Companion To Methods Enzymology. 25, 402–408. doi: 10.1006/meth.2001.1262
Li M. D., Xue X. L. (2012). Determination of manganese (II) in water with an improved potassium periodate spectrophotometric method. J. Huaqiao Univ. (Natural Science) 33 (2), 176–178.
Liu X. F., Zhang L. M., Guan H. N., Zhang Z. W., Xu S. W. (2013). “Effects of oxidative stress on apoptosis in manganese-induced testicular toxicity in cocks.” Food & Chemical Toxicology 60.Complete (2013), 168–176. doi: 10.1016/j.fct.2013.07.058
Lu A. L., Li X., Gu Y., Wright P. M., Chang D. Y.. (2001). "Repair of oxidative DNA damage: mechanisms and functions.". Cell Biochem. Biophysics 35 (2), 141–170. doi: 10.1385/CBB:35:2:141
Mai W. J., Yan J. L., Wang L., Zheng Y., Xin Y., Wang W. N. (2010). Acute acidic exposure induces p53-mediated oxidative stress and DNA damage in tilapia (Oreochromis niloticus) blood cells. Aquat. Toxicol. 100 (3), 271–281. doi: 10.1016/j.aquatox.2010.07.025
Nagalakshmi N., Prasad M. N. V. (1998). Copper-induced oxidative stress in scenedesmus bijugatus: protective role of free radical scavengers. Bull. Environ. Contam. Toxicol. 61 (5), 623–628. doi: 10.1007/s001289900806
Ratn A., Prasad R., Awasthi Y., Kumar M., Misra A., Trivedi S. P. (2018). Zn2+ induced molecular responses associated with oxidative stress, DNA damage and histopathological lesions in liver and kidney of the fish, channa punctatus (Bloc). Ecotoxicol. Environ. Saf. 151, 10–20. doi: 10.1016/j.ecoenv.2017.12.058
Ren Z., Liu J., Huang W., Cao L., Cui W., Dou S. (2019). Antioxidant defenses and immune responses of flounder paralichthys olivaceus larvae under methylmercury exposure. Comp. Biochem. Physiol. C Toxicol. Pharmacol. 225, 108589. doi: 10.1016/j.cbpc.2019.108589
Ross T. (2010). Fundamentals of toxicologic pathology. Aust. Vet. J. 88:6, 235–235. doi: 10.1111/j.1751-0813.2010.00594.x
Salamat N., Ardeshir R. A., Movahedinia A., Rastgar S. (2017). Liver Histophysiological Alterations in Pelagic and Benthic Fish as Biomarkers for Marine Environmental Assessment. Int. J. Environ. Res. 11, 251–262. doi: 10.1007/s41742-017-0023-5
Sakr S. A., Mahran H. A., Fahmy A. M., El-Kholy M. A., Meawad M. (2015). Expression of c-erb-B2 gene in bladder cancer of Egyptian patients and its correlation with p53 and bcl-2. Biomed. Pharmacother. 76, 73–81. doi: 10.1016/j.biopha.2015.10.021
Sayadi M. H., Mansouri B., Shahri E., Tyler C. R., Shekari H., Kharkan J. (2020). Exposure effects of iron oxide nanoparticles and iron salts in blackfish ( capoeta fusca ): Acute toxicity, bioaccumulation, depuration, and tissue histopathology. Chemosphere 247, 125900. doi: 10.1016/j.chemosphere.2020.125900
Seiler F., Rehn B., Rehn S., Hermann M., Bruch J. (2001). Quartz exposure of the rat lung leads to a linear dose response in inflammation but not in oxidative DNA damage and mutagenicity. Am. J. Respir. 24:4, 492–498. doi: 10.1165/ajrcmb.24.4.4181
Sinha K., Das J., Pal P. B., Sil P. C. (2013). Oxidative stress: the mitochondria-dependent and mitochondria-independent pathways of apoptosis. Arch. Toxicol. 87:7, 1157–1180. doi: 10.1007/s00204-013-1034-4
Storey K. B. (1996). Oxidative stress: animal adaptations in nature. Braz. J. Med. Biol. Res. 29:12, 1715–1733.
Vali S., Mohammadi G., Tavabe K. R., Moghadas F., Naserabad S. S. (2020). The effects of silver nanoparticles (Ag-NPs) sublethal concentrations on common carp (Cyprinus carpio): Bioaccumulation, hematology, serum biochemistry and immunology, antioxidant enzymes, and skin mucosal responses. Ecotoxicol. Environ. Saf. 194, 110353. doi: 10.1016/j.ecoenv.2020.110353
Wang X., Gao X. Q., Wang X. Y., Fang Y. Y., Xu L., Zhao K. F., et al. (2022). Bioaccumulation of manganese and its effects on oxidative stress and immune response in juvenile groupers (Epinephelus moara ♀ × e. lanceolatus ♂). Chemosphere 297, 134235. doi: 10.1016/j.chemosphere.2022.134235
Wei J., Zhou T., Hu Z., Li Y., Yuan H., Zhao K., et al. (2018). Effects of triclocarban on oxidative stress and innate immune response in zebrafish embryos. Chemosphere. 210, 93–101. doi: 10.1016/j.chemosphere.2018.06.163
Whiteman M., Chu S. H., Siau J. L., Rose P., Sabapathy K., Schantz J. T., et al. (2007). The pro-inflammatory oxidant hypochlorous acid induces bax-dependent mitochondrial permeabilisation and cell death through AIF-/EndoG-dependent pathways. Cell. Signal 19 (4), 705–714. doi: 10.1016/j.cellsig.2006.08.019
Xu J., Lian L. J., Wu C., Wang X. F., Fu W. Y., Xu L. H. (2008). Lead induces oxidative stress, DNA damage and alteration of p53, bax and bcl-2 expressions in mice. Food Chem. Toxicol. 46 (5), 1488–1494. doi: 10.1016/j.fct.2007.12.016
Yahiro K., Morinaga N., Moss J., Noda M. (2010). Subtilase cytotoxin induces apoptosis in HeLa cells by mitochondrial permeabilization via activation of Bax/Bak, independent of C/EBF-homologue protein (CHOP), Ire1α or JNK signaling. Microb. Pathog. 49 (4), 153–163. doi: 10.1016/j.micpath.2010.05.007
Yan X., Chen Y., Dong X., Tan B., Liu H., Zhang S., et al. (2021). Ammonia toxicity induces oxidative stress, inflammatory response and apoptosis in hybrid grouper (♀ epinephelus fuscoguttatus × ♂ e. lanceolatu). Front. Mar. Sci. 8. doi: 10.3389/fmars.2021.667432
Zhang Y., Liu K., Hassan H. M., Guo H., Ding P., Han L., et al. (2016). L-FABP-deficiency provoked oxidative stress, inflammation and apoptosis-mediated hepatotoxicity induced by pyrazinamide on zebrafish larvae. Antimicrob. Agents Chemother. 60, 12. doi: 10.1128/AAC.01693-16
Zhang S., Zhou Z., Fu J. (2003). Effect of manganese chloride exposure on liver and brain mitochondria function in rats. Environ. 93 (2), 149–157. doi: 10.1016/S0013-9351(03)00109-9
Zhao L. L., Cui C., Liu Q., Sun J., He K., Adam A. A., et al. (2020). Combined exposure to hypoxia and ammonia aggravated biological effects on glucose metabolism, oxidative stress, inflammation and apoptosis in largemouth bass (Micropterus salmoides). Aquat. Toxicol. 224, 105514. doi: 10.1016/j.aquatox.2020.105514
Zhao C., Jia Z., Li E., Zhao X., et al. (2019). "Hepatotoxicity evaluation of euphorbia kansui on zebrafish larvae in vivo. Phytomedicine 62, 152959. doi: 10.1016/j.phymed.2019.152959
Zhao L., Zheng Y. G., Feng Y. H., Wang G. Q., Li M. Y. (2020). Toxic effects of waterborne lead (Pb) on bioaccumulation, serum biochemistry, oxidative stress and heat shock protein-related genes expression in channa argus. Chemosphere 261, 127714. doi: 10.1016/j.chemosphere.2020.127714
Keywords: Manganese, liver damage, apoptosis, oxidative stress, 8-OHdG, yunlong grouper, epinephelus moara, epinephelus lanceolatus
Citation: Wang X, Liu B-L, Gao X-Q, Fang Y-Y, Zhang X-H, Cao S-Q, Zhao K-F and Wang F (2022) Effect of long-term manganese exposure on oxidative stress, liver damage and apoptosis in grouper Epinephelus moara ♀ × Epinephelus lanceolatus ♂. Front. Mar. Sci. 9:1000282. doi: 10.3389/fmars.2022.1000282
Received: 22 July 2022; Accepted: 05 September 2022;
Published: 21 September 2022.
Edited by:
Rui Jia, Chinese Academy of Fishery Sciences, ChinaReviewed by:
Changhong Cheng, South China Sea Fisheries Research Institute (CAFS), ChinaYanting Cui, Qingdao Agricultural University, China
Zhen Lu, Shenzhen University, China
Copyright © 2022 Wang, Liu, Gao, Fang, Zhang, Cao, Zhao and Wang. This is an open-access article distributed under the terms of the Creative Commons Attribution License (CC BY). The use, distribution or reproduction in other forums is permitted, provided the original author(s) and the copyright owner(s) are credited and that the original publication in this journal is cited, in accordance with accepted academic practice. No use, distribution or reproduction is permitted which does not comply with these terms.
*Correspondence: Bao-Liang Liu, liubl@ysfri.ac.cn