- 1Postgraduate and Research Department of Zoology, Pachaiyappa’s College for Men, Chennai, India
- 2Department of Biotechnology, Dwaraka Doss Goverdhan Doss Vaishnav College, Chennai, India
Introduction
Global warming and ocean acidification (OA) are being brought on by an increase in atmospheric carbon dioxide (CO2) concentration (Feely et al., 2004; Orr et al., 2005), and both are now widely acknowledged as significant factors in modulating physiological systems in the marine organism (Srinivasan et al., 2022). Increased CO2 levels in the surface waters result in a drop in seawater pH, and changes in the chemistry of carbonates, and can have an impact on the bioavailability of contaminants (Bijma et al., 2013). Although OA has a significant impact on the acid-base balance, energy metabolism, and biomineralization of marine organisms (Kroeker et al., 2010), its effects on immune functions are still poorly understood (Calder-Potts et al., 2008), which makes it difficult to predict how susceptible marine fish to overcome the parasites and diseases attack as the ocean physicochemical concentration changes.
The survival of marine organisms are significantly influenced by good seawater quality (Jha et al., 2013), diseases and parasites free suitable locations, which are regarded as the main risk factor in both fish farms and the open ocean (Kumar et al., 2009; Jha et al., 2017). The resistance of aquatic organisms to parasites and diseases can be modulated by environmental stresses, such as fluctuations in temperature, salinity, and pollution (Galloway and Depledge, 2001; Marcogliese, 2008). Fish that live in water are constantly exposed to a variety of pathogenic and non-pathogenic microorganisms; as a result, they may evolve powerful defence systems that aid in their survival. Aquatic organism immune systems, like that of fish, are constantly impacted by periodic or unanticipated changes in their surroundings. Fish health status may be negatively impacted by adverse environmental circumstances either acutely or chronically, either by changing certain biochemical parameters or by regulating innate and adaptive immune responses (Domingueza et al., 2004). Fish may therefore serve as significant indicator in the biomonitoring of water quality, particularly immunotoxic environmental contaminants, depending on their biological factors related to immunity.
The available literature regarding the alteration of immunity in shellfish because of ocean acidification shows that earlier studies were focused on marine invertebrates since the shellfish are more vulnerable to acidified environment since most of the higher invertebrates rely more on these environment for calcification (Ivanina et al., 2014). Our previous study documented that acidified environment impairs haematological and serum biochemical parameters in Lates calcarifer (Srinivasan et al., 2022). In the present study, it was further observed that long-term chronic exposure to acidified medium modulated the immunocompetence of fish in terms of the change in immune-associated parameters tested. To our knowledge, negligible work has been carried out to observe the impact of seawater acidification on immunomodulation in fish with different size groups. Therefore the present study investigated the effect of acidification on the nonspecific immune-associated parameters, including respiratory burst, myeloperoxidase (MPO) activity, bactericidal activity, circulating total immunoglobulin, and on cellular components (leukocytes) in two different size groups (fish fry and fingerlings) of Lates calcarifer.
Methods
The collection of fish and experimental design were explained in detail as described in Srinivasan et al., 2022. Briefly, a total of 360 fish were used for this study (180 fry and 180 fingerlings). The control, pH 7.7, and pH 7.4 were three groups and for time point, we used 18 fish per experimental group. Sampling was done at 20, 40, and 60 days (d) of exposure. Fish were anesthetized for 30 seconds in ice-cold aquarium water before collecting the blood. Blood samples were split into two aliquots; one was heparinized (at a concentration of 50 IU/ml blood), and the other was left to coagulate at room temperature for 30 minutes before being stored at 4°C for 24 hours. The serum was extracted from the clotted sample by centrifuging it at 3,500 rpm for 5 minutes at 4°C, and it was then kept at -20°C until it was used. Heparinized blood was utilised for Nitroblue tetrazolium assay and differential leukocyte counts. The University of Madras’ Animal Ethics Committee authorized the aforementioned animal procedures.
Differential leukocyte count was done according to the method described by Horton and Okamura (2003). Briefly, by preparing a blood smear on a slide by using 20 µL of heparinized blood, the slide was then fixed in 95% methanol before staining with Giemsa for 5 min. The slides were washed gently in tap water and allowed to dry before microscopic examination. The percentage of monocytes, lymphocytes, neutrophils, and thrombocytes in the blood was determined by counting leukocytes under a microscope randomly until the count reached 100 cells per slide.
Respiratory burst activity was assessed by following the modified method described by Thilagam et al. (2009) and Havixbeck et al. (2016). About 50 µL of heparinized blood was incubated at 37°C for 1 h to facilitate adhesion of cells in the Eppendorf tube followed by washing with phosphate buffer. About 50 µL of nitroblue tetrazolium was added to the tube and incubated for a further 1 h. The tubes were shaken well and centrifuged at 1000 rpm for 15 minutes, after which the supernatant was removed. Absolute methanol was used to fix the cells followed by washing in 30% methanol. The tubes were air dried and extraction fluid of 2 mL (potassium hydroxide and dimethyl sulfoxide) was added to each tube to dissolve the precipitate. Tubes were then centrifuged at 6,000 rpm for 10 minutes to collect the supernatant and read at 630 nm optical density.
Myeloperoxidase (MPO) was measured in the serum following the method described by Quade and Roth (1997). A serum sample (10 µL) and 140 µL of Hank’s balanced salt solution (Ca2+ and Mg2+ free) were added to a 96- well plate. The 50 µL of 20 mM 3,3’,5,5’ tetra methyl benzidine, and 5 mM H2O2 were added to initiate the reaction. After 2 minutes the reaction was stopped by adding 50 µL of 4 M sulphuric acid and the absorbance was measured at 450 nm in a microplate reader.
The procedure described by Siwicki and Anderson (1993) was adapted to test the total immunoglobulin level. Briefly, polyethylene glycol was used to precipitate immunoglobulin. Blood serum was incubated with equal volume polyethylene glycol solution for two hours at room temperature followed by spinning the sample tube at 3,000 rpm for 15 minutes, the supernatant was used for measuring the protein content using Bradford method (1976). The quantity of measured protein was subtracted from the serum protein content to determine the total immunoglobulin level.
Serum bactericidal activity was measured following a modified method described by Rainger and Rowley (1993). A pellet of Aeromonas hydrophila was washed and suspended in phosphate buffer. The absorbance of the suspension was adjusted to 0.500 at 600 nm and the suspension was serially diluted (1:10) with phosphate buffer. The bactericidal activity was determined by incubating 2 µL of the A. hydrophila suspension with 20 µL of serum in a microvial for 1 h at 37°C. In control group, phosphate buffer was used in place of serum sample and nutrient broth was used in place of the serum for positive control. By counting the colonies that appeared on a nutrient agar plate after 24 hours at 37°C following incubation, the number of live bacteria was ascertained.
SPSS software was used to perform the statistical analysis (version 20). Graph pad prism (version 8) was used to plot the graph. Prior to using a two-way analysis of variance (ANOVA) to assess differences between the groups, the data were normalized, and homogeneity test also conducted. The statistical difference between various treatment groups was assessed using Tukey’s multiple comparison post hoc tests, and a p-value of less than 0.05 was regarded as significant.
Results and discussion
Leukocytes count in fish fry and fingerlings are given in Figures 1A–D. Monocyte counts in the fish fry and fingerlings increased significantly (p<0.05) when the fish were exposed to pH 7.4. All the exposure periods showed a significant rise in monocyte counts (Figure 1A). There were no discernible changes between the respective control and fish fry or fingerling exposed to pH 7.7. In contrast, the neutrophil counts decreased significantly in fry exposed to pH 7.7 and pH 7.4 after 20 d (Fig. 1B). Both fry and fingerlings exposed to pH 7.7 reduced neutrophil counts after 40 d of exposure. However, fish exposed to both pH (7.7 and 7.4) for 60 d showed no significant difference with respective control groups (Figure 1B)
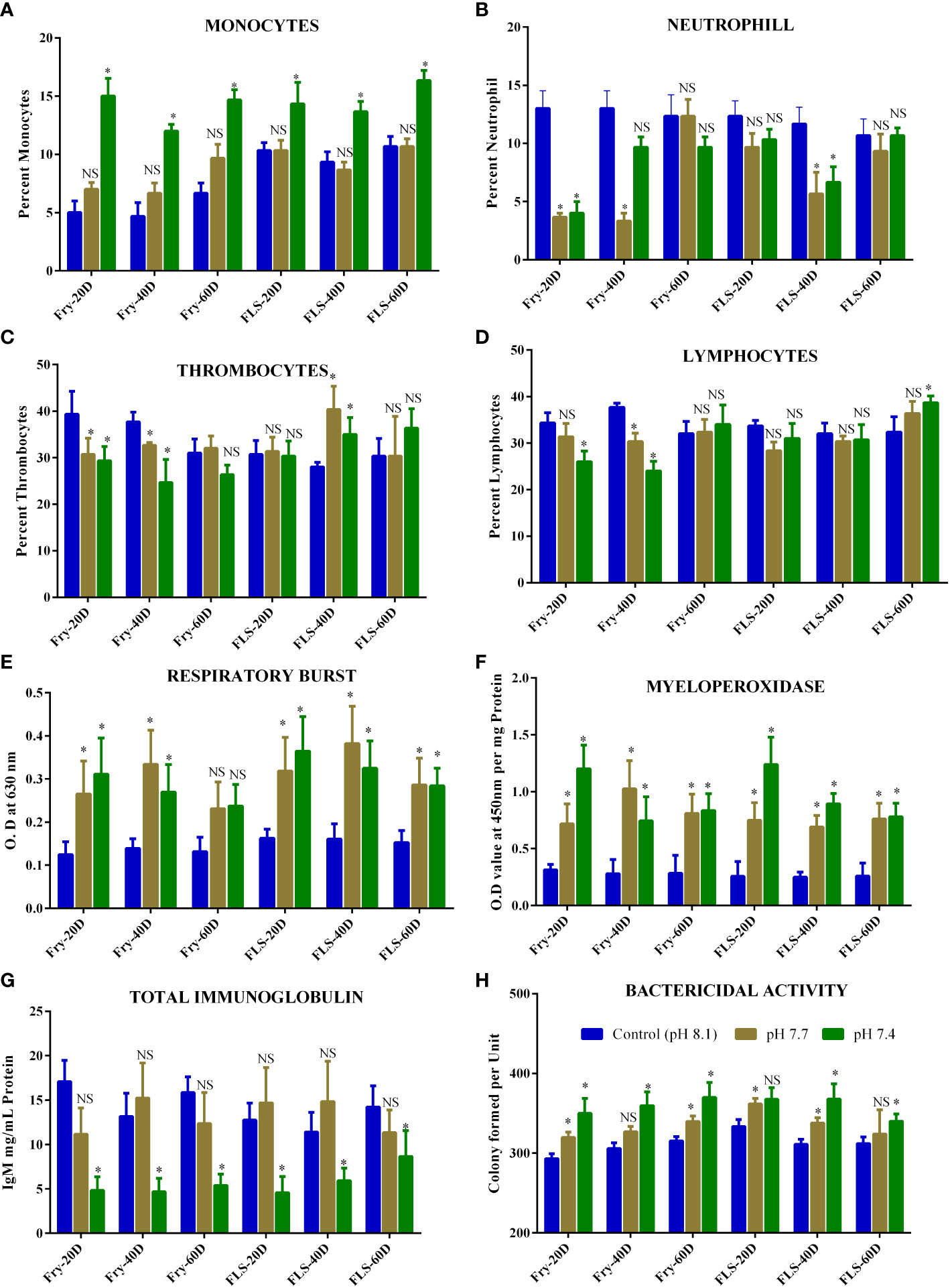
Figure 1 (A-D) is the data representing the biochemical components such as (A) Monocytes (B) Neutrophil (C) Thrombocytes (D) Lymphocytes (E) Respiratory burst (F) Myeloperoxidase (G) Immunoglobulin (H) Bactericidal activity in fish fry and fingerling of Asian seabass exposed for 60 d under different acidified conditions. Each bar represents mean ± standard deviation of six samples. Two-way analysis of variance followed by Tukey’s post hoc test was used. The asterisk indicates significant difference between the exposure and control groups whereas NS letters No significant difference (p< 0.05) between different groups.
After 20 d and 40 d of exposure, fish fry exposed to pH 7.7 and pH 7.4 had considerably less thrombocytes than the corresponding control group. However, fingerlings exposed to pH 7.7 and pH 7.4 exhibits a rise in thrombocytes that was statistically significant over 40 d (Figure 1C) of exposure. With respect to the control groups, fingerling exposed to pH 7.4 for 60 d exhibits an increase in thrombocytes but no discernible change. After 20 d and 40 d of exposure to pH 7.7 and pH 7.4, the lymphocyte counts in fry dramatically dropped, but after 60 d of exposure, there was no discernible change observed (Figure 1D). Fish fingerlings subjected to pH 7.7 and pH 7.4 did not significantly differ from the corresponding control in terms of lymphocyte count, except for fish fingerlings exposed to pH 7.4 for 60 d (Figure 1D). Reduced circulating lymphocyte count were a sign that immunosuppression had taken place in fish exposed to the lower pH, as documented by Machado et al. (2020). Additionally, after 20 d of exposure, neutrophils in fish fry drastically reduced, but not in fingerling for the same exposure period. On the contrary monocytes, the count was increased when the fish were exposed to pH 7.4. Increase in circulating lymphocyte and thrombocyte count were a sign of nonspecific immunological activation (Machado et al., 2020) and the drastic change in these leukocyte counts in fish may lead to immunomodulation (Thilagam et al., 2009).
The increase in respiratory burst activity in fish is a measure of the amount of oxidative radicals produced as a result of acidified exposure (1 E). When the fish fingerlings and fry were exposed to pH 7.7 and pH 7.4 respiratory burst activity increased significantly after 20 d and 40 d respectively compared to the respective control group of L. calcarifer (Figure 1E). Interestingly fish fry exposed to both pHs (7.7 and 7.4) showed no significant change after 60 d of exposure when compared to the respective groups showing that the fish might adapt to the acidified environment.
In recent times, respiratory burst activity has been utilised to examine how fish’s immune systems respond to exposure to environmental factors (Barbosa et al., 2020). An indicator of the modulation of nonspecific immunity is the increase in respiratory burst activity in fish, which is a measure of oxidative radical production due to environmental stress (Thilagam et al., 2009). Furthermore, the ROS produced during the respiratory burst activity are thought to be potent microbicidal agents. The current investigation found that exposure of fish to acidified environment increased respiratory burst activity in both groups and that the increase depended on the amount of acidity and the length of exposure. It’s interesting to see that the prolonged acidification exposure (60 d) to the fish fry did not significantly increase the ROS. Though both fry and fingerlings were shown to be more vulnerable to acidified environment, according to the findings of the current study and these modulations in ROS generation after prolonged exposure could be either fish adaptability to the environmental condition or due to enhance the antioxidant defence of the fish and needs to be elucidated.
The MPO activity increased when fish fry and fingerlings were exposed to acidified environment throughout the exposure period as compared to the corresponding control group (Figure 1F). At 20 d, both groups exposed to pH 7.4 showed greater MPO activity. MPO activity had a similar pattern to that of the respiratory burst. In many situations where neutrophils play a role in pathological diseases, MPO levels, and neutrophil activation can be connected. A significant association between the respiratory burst and MPO was found (Figures 2A, B), indicating that most of the produced during this respiratory burst was transformed to the bactericidal oxidant hypochlorous acid via a sequence of events involving superoxide dismutase and MPO (Thilagam et al., 2009).
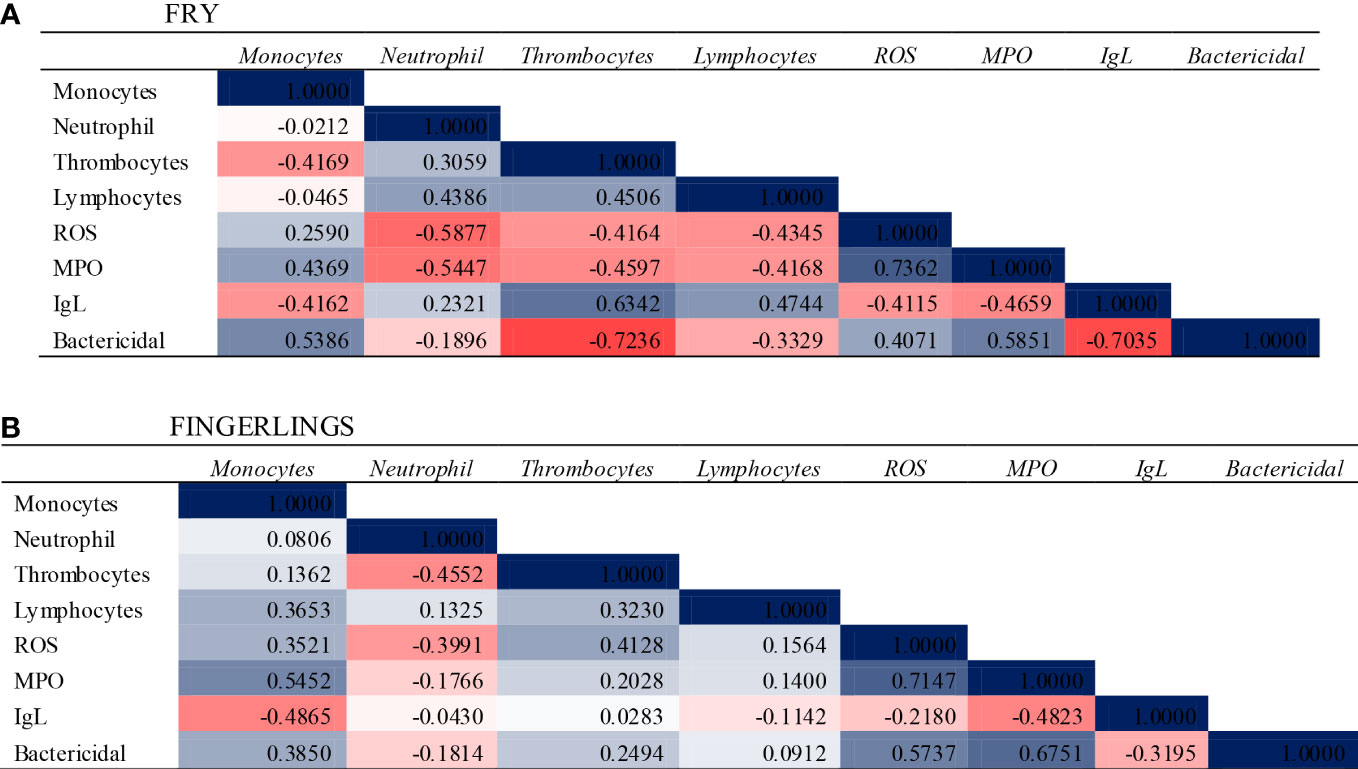
Figure 2 Correlation Analysis and heat map showing the relationship between the immune associated parameters when the Asian seabass L. calcarifer exposed to OA for 60 d. (A) Fry (B) Fingerlings. The values and colour depict the relationship between the parameters.
Total immunoglobulin levels in fish exposed to acidified environment is given in Figure 1G. Both the fry and fingerling groups showed a drop in total immunoglobulin levels after exposure to acidified medium, however, only fish exposed to pH 7.4 experienced a significant drop in total immunoglobulin during the exposure period. Fingerling exposed to pH 7.7 showed a noticeable rise in total immunoglobulin levels, but such increase was not statistically significant. Environmental influences are thought to interact with cell nucleoproteins and nucleic acids, changing protein synthesis and cell integrity. Changes in blood protein levels as a result of environmental stress have been documented (Dominguez et al., 2004; Srinivasan et al., 2022). Except for fish fingerlings exposed to pH 7.7 in the current investigation, the total immunoglobulin level was significantly lower in fish exposed to experimentally acidified environment compared to the respective control group. When Nile tilapia were exposed to acidified environment it was observed that there was no appreciable change in immunoglobulin levels (Dominguez et al., 2004), but the fish did react to changes in salinity and temperature. Machado et al. (2020) recorded a decrease in total immunoglobulin when Senegalese sole exposed to acidified seawater and in the present study decrease in total immunoglobulin was statistically significant (p< 0.05) in the fish fry which supports the previous study by Machado et al. (2020) and revealed that decrease in total immunoglobulin could be attributed to increasing acidification in the oceanic environment. The discrepancy in the results for fish fry and fingerlings could be attributed to the adaptability of different size groups to the acidified environment and subsequent stress.
Figure 1H illustrates how exposure to acidified environment affected the serum bactericidal activity in both fish fry and fingerlings. When the fry was exposed to pH 7.7 and pH 7.4 for 20 d, 40 d, and 60 d, the serum bactericidal activity drastically reduced. Similarly declines in bactericidal activity were observed in fingerling serum at both pH (7.7 and 7.4) after 20 and 40 d. However, fingerling exposed to pH 7.7 after 60 d did not exhibit any significant differences from the respective control group. The decrease in serum bactericidal activity at all exposure periods in both groups as compared to the respective control group can be used to explain why fish exposed to acidified environment developed less resistance to A. hydrophila.
Statistically significant (p< 0.05) associations were observed between parameters of the immune system in the blood components of fish (Figure 2). There were statistically significant correlations between leukocyte counts and other parameters studied in both fish fry and fingerlings. A significant positive correlation was found between the leukocytes (Monocytes and Lymphocytes) with most of the immune associated parameters in fish fingerlings and the same was negative correlated in fish fry. This discrepancy might due to the size variation or adaptation of the fingerling to the acidified environment needs to elucidate further.
This is the first comparison study of how the immune system in L. calcarifer modulated when two different size groups of fish are exposed to an acidified environment. In light of the findings of current study, we hypothesised that seawater acidification might have an impact on the structure and immune function of L. calcarifer leukocytes. Acidification exposure led to an excess of ROS, which was responsible for causing oxidative stress in the leukocytes, and at the same time, the acidified environment induced further accumulation of ROS by inhibiting the activity of the antioxidant system and these changes might result in immune system dysfunction, which could have negative effects including decreased disease resistance, growth impairment, and poorer survival rates. Furthermore it has been demonstrated that the increasing H+ level is just one of several stressors brought on by the CO2-induced acidification of seawater. In order to clarify the underlying processes, further study should concentrate on analysing the energy metabolism, oxidative stress and antioxidant defense of L. calcarifer subjected to acidified environment.
Data availability statement
The original contributions presented in the study are included in the article/supplementary material. Further inquiries can be directed to the corresponding authors.
Ethics statement
Ethical review and approval was not required for the animal study because only commercial fish were used with no ethical issues.
Author contributions
PM, PS, GS, RS: Sample collection, laboratory analysis, data processing. TH: Data validation and manuscript preparation. SB, GS: Review the manuscript, suggestion, and project administration. All authors contributed to the article and approved the submitted version.
Acknowledgments
The authors thank the Head, Research Department of Zoology and also the Principal Pachaiyappa’s College for Men, Chennai- 600 030 and Department of Biotechnology and the Principal Dwaraka Doss Goverdhan Doss Vaishnav College, Chennai, 600106, Tamil Nadu, India for extending their support through the experiment.
Conflict of interest
The authors declare that the research was conducted in the absence of any commercial or financial relationships that could be construed as a potential conflict of interest.
Publisher’s note
All claims expressed in this article are solely those of the authors and do not necessarily represent those of their affiliated organizations, or those of the publisher, the editors and the reviewers. Any product that may be evaluated in this article, or claim that may be made by its manufacturer, is not guaranteed or endorsed by the publisher.
References
Barbosa L. M. G., Moraes G., Anibal F. F., Machado C. M. M. (2020). Effect of environmental thermal fluctuations on innate immune responses in pacu Piaractus mesopotamicus juveniles. Aquac Rep. 17, 100303. doi: 10.1016/j.aqrep.2020.100303
Bijma J., Pörtner H. O., Yesson C., Rogers A. D. (2013). Climate change and the oceans–what does the future hold? Mar. Pollut. Bull. 74 (2), 495–505. doi: 10.1016/j.marpolbul.2013.07.022
Bradford M. M. (1976). A rapid and sensitive method for the quantitation of microgram quantities of protein utilizing the principle of protein-dye binding. Anal Biochem. 72, 248–254. doi: 10.1006/abio.1976.9999
Calder-Potts R., Widdicombe S., Parry H., Spicer J., Pipe R. (2008). Effects of ocean acidification on the immune response of the blue mussel Mytilus edulis. Aquat. Biol. - AQUAT Biol. 2, 67–74. doi: 10.3354/ab00037
Dominguez M., Takemurab A., Tsuchiyaa M., Nakamura S. (2004). Impact of different environmental factors on the circulating immunoglobulin levels in the Nile tilapia, Oreochromis niloticus. Aquaculture 241, 491–500. doi: 10.1016/j.aquaculture.2004.06.027
Feely R. A., Sabine C. L., Lee K., Berelson W., Kleypas J., Fabry V. J., et al. (2004). Impact of anthropogenic CO2 on the CaCO3 system in the oceans. Science 305, 362–366. doi: 10.1126/science.1097329
Galloway T. S., Depledge M. H. (2001). Immunotoxicity in invertebrates: measurement and ecotoxicological relevance. Ecotoxicol (London England) 10 (1), 5–23. doi: 10.1023/a:1008939520263
Havixbeck J. J., Rieger A. M., Wong M. E., Hodgkinson J. W., Barreda D. R. (2016). Neutrophil contributions to the induction and regulation of the acute inflammatory response in teleost fish. J. Leukoc Biol. 99 (2), 241–252. doi: 10.1189/jlb.3HI0215-064R
Horton T., Okamura B. (2003). Post-haemorrhagic anaemia in sea bass, dicentrarchus labrax (L.), caused by blood feeding of ceratothoa oestroides (Isopoda: Cymothoidae). J. Fish Dis. 26 (7), 401–406. doi: 10.1046/j.1365-2761.2003.00476.x
Ivanina A. V., Hawkins C., Sokolova I. M. (2014). Immunomodulation by the interactive effects of cadmium and hypercapnia in marine bivalves crassostrea virginica and mercenaria. Fish Shellfish Immunol. 37 (2), 299–312. doi: 10.1016/j.fsi.2014.02.016
Jha D. K., Devi M. P., Vinithkumar N. V., Das A. K., Dheenan P. S., Venkateshwaran P., et al. (2013). Comparative investigation of water quality parameters of aerial & rangat bay, Andaman islands using in-situ measurements and spatial modelling techniques. Water Qual. Expo. Health 5, 57–67. doi: 10.1007/s12403-013-0086-2
Jha D. K., Rajaprabhu G., Kirubagaran R., Sendhil Kumar R., Dharani G., Das A. K., et al. (2017). Estimation of potential zones for offshore mariculture in the Indian Sea using geographical information system as a management tool. J. Coast. Conserv. 21, 893–902. doi: 10.1007/s11852-017-0556-y
Kroeker K. J., Kordas R. L., Crim R. N., Singh G. G. (2010). Meta-analysis reveals negative yet variable effects of ocean acidification on marine organisms. Ecol. Lett. 13 (11), 1419–1434. doi: 10.1111/j.1461-0248.2010.01518.x
Kumar T. S., Vijayakumaran M., Murugan T. S., Jha D. K., Sreeraj G., Muthukumar S. (2009). Captive breeding and larval development of the scyllarine lobster Petrarctus rugosus. n. Z. J. Mar. Freshw. Res. 43, 101–112. doi: 10.1080/00288330909509985
Machado M., Arenas F., Svendsen J. C., Azeredo R., Pfeifer L. J., Wilson J. M., et al. (2020). Effects of water acidification on Senegalese sole solea senegalensis health status and metabolic rate: Implications for immune responses and energy use. Front. Physiol. 11. doi: 10.3389/fphys.2020.00026
Marcogliese D. J. (2008). The impact of climate change on the parasites and infectious diseases of aquatic animals. Rev. sci tech (Int Office Epizootics) 27 (2), 467–484. doi: 10.20506/rst.27.2.1820
Orr J., Fabry V., Aumont O., Bopp L., Doney S. C., Feely R. A., et al. (2005). Anthropogenic ocean acidification over the twenty-first century and its impact on calcifying organisms. Nature 437, 681–686. doi: 10.1038/nature04095
Quade M. J., Roth J. A. (1997). A rapid, direct assay to measure degranulation of bovine neutrophil primary granules. Vet Immunol. immunopathol 58 (3-4), 239–248. doi: 10.1016/s0165-2427(97)00048-2
Rainger G. E., Rowley A. F. (1993). Antibacterial activity in the serum and mucus of rainbow trout, oncorhynchus mykiss, following immunization with Aeromonas salmonicida. Fish Shellfish Immunol. 3, 475–482. doi: 10.1006/fsim.1993.1046
Siwicki A. K., Anderson D. P. (1993). Nonspecific defence mechanisms assay in fish II; potential killing activity of neutrophils and manocytes, lysozyme activity in serum and organs and total immunoglobulin (Ig) level in serum (Olsztyn, Poland: Olsztyn: Wydawnictwo Instytutu Rybactwa Strodladowego).
Srinivasan G., Marcus P. N., Sivakumar P., Paramasivam P., Sivakumar R., Harikrishnan T., et al. (2022). Ocean acidification impact on haematological and serum biochemical parameters in lates calcarifer. Front. Mar. Sci. 9. doi: 10.3389/fmars.2022.940573
Keywords: Asian seabass, ocean acidification, marine environment, immunomodulation, carbon dioxide, leukocytes, respiratory burst
Citation: Marcus PN, Sivakumar P, Srinivasan G, Sivakumar R, Harikrishnan T, Balasubramaniam S and Singaram G (2022) Immunomodulatory response of Asian seabass Lates calcarifer in an ex situ environment: Implications for future ocean acidification scenario. Front. Mar. Sci. 9:1011149. doi: 10.3389/fmars.2022.1011149
Received: 03 August 2022; Accepted: 05 September 2022;
Published: 20 September 2022.
Edited by:
Prashanthi Devi Marimuthu, Bharathidasan University, IndiaReviewed by:
Obulisamy Parthiba Karthikeyan, South Dakota School of Mines and Technology, United StatesRajakumar S, Bharathidasan University, India
Copyright © 2022 Marcus, Sivakumar, Srinivasan, Sivakumar, Harikrishnan, Balasubramaniam and Singaram. This is an open-access article distributed under the terms of the Creative Commons Attribution License (CC BY). The use, distribution or reproduction in other forums is permitted, provided the original author(s) and the copyright owner(s) are credited and that the original publication in this journal is cited, in accordance with accepted academic practice. No use, distribution or reproduction is permitted which does not comply with these terms.
*Correspondence: Sujatha Balasubramaniam, c3VqYXRoYXBhY2hhaXlhcHBhc0BnbWFpbC5jb20=; Gopalakrishnan Singaram, Z29wYWx0aGlsYWdhbUBnbWFpbC5jb20=; Thilagam Harikrishnan, dGhpbGFnYW1wYWNoYWl5YXBwYXNAZ21haWwuY29t
†These authors have contributed equally to this work