- 1Biogeochemistry Research Center, Japan Agency for Marine-Earth Science and Technology (JAMSTEC), Yokosuka, Kanagawa, Japan
- 2Kochi Institute for Core Sample Research, Japan Agency for Marine-Earth Science and Technology (JAMSTEC), Nankoku, Kochi, Japan
- 3Faculty of Geo-Environmental Science, Rissho University, Kumagaya, Saitama, Japan
Stable isotope ratios of Sr (88Sr/86Sr) are a novel indicator for identifying terrestrial and oceanic Sr cycling and biological processes. Here we evaluate the temperature dependence of stable Sr isotope ratios (δ88Sr) in the calcite skeletons of precious corals, known as octocorals. High-precision Sr isotope measurements by double spike thermal ionization mass spectrometry were applied to different precious corals (Corallium japonicum, Pleurocorallium elatius, P. konojoi, Corallium sp., Keratoisis sp., and Coralliidae sp.) collected from water depths of 30–1500 m in the Pacific Ocean, corresponding to annual mean water temperatures of 2.5 to 19.5°C. The precious corals showed mean δ88Sr values of 0.101 ± 0.023‰. The magnitude of Sr isotope fractionation between the skeleton and ambient seawater (Δ88Sr) is −0.291 and is about −0.1‰ lower than the value predicted from the precipitation of inorganic calcite. This fractionation is not affected by changes in skeletal Sr/Ca ratio and associated biological effects on δ88Sr are negligible. δ88Sr of precious coral skeleton is an excellent recorder of ambient seawater.
Introduction
Trace elements in coral skeletons provide information about geographical, biological, and environmental conditions over a wide range of water depths, from the surface reef to deep water (Adkins, 1998; Eltgroth et al., 2006; Sherwood and Risk, 2007). The reconstruction of past temperature and seawater chemistry based on coral skeletal proxies is important to understand the mechanisms of environmental changes over various geological timescales of recent tens of years to more deep geologic time. The use of the stable strontium isotope ratio (δ88Sr) in combination with the radiogenic isotope ratio (87Sr/86Sr) is for investigating Sr sources (riverine, groundwater, hydrothermal, and porewater discharges) and sinks (dominantly by carbonate deposition) in the ocean (e.g., Krabbenhöft et al., 2010; Yoshimura et al., 2020; Paytan et al., 2021; Yoshimura et al., 2021).
Since coral skeletons grow from an aqueous solution termed extracellular calcifying fluid (ECF), it has been suggested that metal incorporation into coral skeletons may not directly reflect ambient environmental conditions. Coral ECF is partly open to seawater, but is under strict biological controls of fluid chemistry, especially on [DIC], [Ca2+], and pH, then aragonite supersaturation than ambient seawater is achieved (e.g., McCulloch et al., 2012). Controls on DIC and Ca have also been reported for precious corals (Allemand and Grillo, 1992; McCulloch et al., 2012), but are weaker than for aragonite corals (McCulloch et al., 2012), or ECF saturation may not be the case for octocorals (Le Goff et al., 2017). Paracellular material transport, including passive diffusion of ions and molecules via junctions between epithelial cells, water passage through aquaporins, and CO2 diffusion through membranes, is considered to accompany negligible element partitioning. Another pathway is active transport from seawater to coral ECF by protein chelation and ion channels. These processes preferentially incorporate certain elements or isotopes to facilitate optimum skeletal growth. These biochemical biases are species-specific, and therefore significant efforts have been made to identify robust geochemical calibration between skeletal element/isotopic compositions and environmental parameters for each biological carbonate.
A geochemical record of well-preserved fossil corals is an excellent constraint on past seawater chemistry. For example, recent studies of Mg isotope ratios (δ26Mg) using well-calibrated aragonitic corals enables the reconstruction of seawater δ26Mg during the Cenozoic era, tracking changes in Mg-bearing carbonate weathering versus dolomite formation through time (Gothmann et al., 2017). Strontium in biogenic calcium carbonate of fossil brachiopods (Vollstaedt et al., 2014) and marine authigenic barite (Paytan et al., 2021) are used to reconstruct past ocean temperature and seawater element cycles. Of these, biogenic carbonates are excellent samples for reconstructing the continuous geologic record, but the biological effects of elemental partitioning and isotopic fractionation need to be assessed for each species and genus. Given that there is no physiological role of Sr, less magnitudes of biological effect during Sr cellular pathways on δ88Sr can be expected. It is established that biogenic carbonates preferentially incorporate 86Sr isotopes relative to seawater (Fietzke and Eisenhauer, 2006; Rüggeberg et al., 2008; Raddatz et al., 2013; Stevenson et al., 2014; Vollstaedt et al., 2014). The magnitude of stable Sr isotope fractionation in planktonic foraminifera is similar to inorganic calcite (Böhm et al., 2012). However, some genera, especially low-Mg calcite of coccolithophores, show a marked biologically induced fractionation (Stevenson et al., 2014). Octocorallia (Anthozoa) corals dwell at water depths of several dozen to thousands of meters, and their skeletons are composed of high-Mg calcite. Some octocorals are reef-dwelling and have photosynthetic symbiotic algae for this shallow-water species (van Oppen et al., 2005). As for calcite skeletons of precious corals, their Mg partitioning and isotopic compositions are generally similar to inorganic calcite and are expected to record ambient environmental conditions faithfully (Yoshimura et al., 2011). If calcitic corals exhibit a relatively constant Sr isotope fractionation factor regardless of species and environmental factors, it will become a good tool in paleoceanography to reconstruct past ocean chemistry. Among the most basic environmental parameters, temperature influence on skeletal geochemistry has been widely addressed in Ca, Mg, K, and Sr isotope fractionations of coral and other biogenic carbonates (Böhm et al., 2006; Fietzke and Eisenhauer, 2006; Inoue et al., 2015; Li et al., 2022).
To establish reliable proxy development and better understand the details of biomineralization mechanisms, this study aims to evaluate the stable Sr isotope ratio of precious corals as a new environmental proxy. We used precious corals (Corallium japonicum, Pleucorallium elatius, P. konojoi, Corallium sp., and Keratoisis sp.), known as octocorals or deep-sea corals, from sampling depths of the shallowest sample collected from a depth of 100 m and of the deepest sample from a range of 1,420-1620 m in the western, northwestern, and northern Pacific oceans (Table 1). Mean annual water temperatures at the sampling localities range from 2.5 to 19.5°C (Yoshimura et al., 2011). This genus is not suitable for laboratory culture experiments in a controlled tank due to its slow calcification rate, and thus, a calibration using natural species is necessary. We present the relationships among isotope values and water temperature and trace element concentrations in coral skeletons.
Sample and method
Coral materials
Precious coral (Corallium japonicum, Pleurocorallium elatius, P. konojoi, Corallium sp., Keratoisis sp., and Coralliidae sp.) specimens were collected from water depths of 30–1500 m in the western, northwestern, and northern Pacific (Table 1). Water temperatures are from [(Levitus and Boyer, 1994); http://ingrid.ldeo.columbia.edu/SOURCES/.LEVITUS94/]. The Sr/Ca ratios of these specimens have already been reported by Yoshimura et al. (2011). We used the value of Sr/Ca = 8.67 mmol/mol calculated from the following database: https://www.mbari.org/science/upper-ocean-systems/chemical-sensor-group/periodic-table-of-elements-in-the-ocean/. A recent literature has reported on the homogeneity and heterogeneity of the Sr/Ca in seawater, classified by sea area and depths (Lebrato et al., 2020). The average Sr/Ca values corresponding to our sampling area are around 8.6 mmol/mol, which is consistent with the value we used for calculation.
Coral skeletons were cut into slabs with a diamond saw and ultrasonically cleaned with ultrapure H2O2 and distilled water to remove organic compounds. After chemical treatment, the skeletons were crushed to the size of sand and ground to a homogeneous powder in an agate mortar before being subjected to trace metal and isotope analysis; Sr/Ca, Mg/Ca, and Mg isotope ratios have already been reported for the same homogeneous powder skeletons as for the Sr isotope ratio by Yoshimura et al. (2011). The Mg isotopic ratios show no difference between the sites of the skeletal axis, where the color and skeletal structure differences, whitish versus reddish, are more pronounced, and bulk samples were used for all Sr isotope measurements in this study.
Isotope analysis
The stable and radiogenic Sr isotopic compositions of the samples were analyzed simultaneously by double-spike thermal ionization mass spectrometry (DS-TIMS, Wakaki et al., 2017). An aliquot of the sample solution was mixed with 84Sr-86Sr spike. After Sr separation chemistry using Sr-spec resin (Eichrom), Sr isotope ratios of both unspiked and spiked samples were measured with a TRITON thermal ionization mass spectrometer (Thermo Scientific) at Kochi Core Center, Japan. The 87Sr/86Sr ratios of the unspiked sample were measured by conventional internal normalization technique using 86Sr/88Sr = 0.1194 and the exponential law. The stable Sr isotope composition was analyzed by the double spike technique using 84Sr/86Sr, 87Sr/86Sr and 88Sr/86Sr ratios of the unspiked and spiked samples, and was expressed as permil (‰) deviations relative to the NIST SRM-987 reference material:
The 2σ analytical uncertainty of the δ88Sr value was estimated as the root-sum square of the 2SE internal error and the 2SD external error. The internal error was calculated by error propagation of the 2 SE internal errors of the two isotope ratio measurements. The external error was estimated as 2SD repeatability of multiple SRM-987 analyses during an analytical session. The typical SE internal error and the 2SD external error of δ88Sr value were 0.006‰ and 0.020‰, respectively. Repeated analysis of an in-house Sr stable isotopic reference reagent Wako-9999 (Wakaki et al., 2017) gave a reproducibility of ± 0.025‰ (2SD, n = 45), showing the long-term reproducibility of the analysis. To check the accuracy of the analysis, we analyzed seawater reference material IAPSO. The δ88Sr value of seawater was 0.392 ± 0.015‰ (2SE, n = 3), which is consistent with published values for seawater samples from the Atlantic, Pacific, Indian, and Southern Oceans (e.g., Krabbenhöft et al., 2009; Neymark et al., 2014; Stevenson et al., 2014; Vollstaedt et al., 2014; Pearce et al., 2015; Wakaki et al., 2017). The δ88Sr value for JCp-1 coral standard was 0.189 ± 0.024‰ (2SD, n = 12).
Results
Stable and radiogenic Sr isotope values obtained from the precious corals are presented in Table 1. All 87Sr/86Sr are indistinguishable from the modern seawater value. The δ88Sr values of precious corals ranged from 0.077 to 0.120‰ (Table 1).
* The degree of stable Sr isotope fractionation between the coral skeletons and seawater (Δ88Sr) ranged from -0.315 to -0.272‰. The δ88Sr and Sr/Ca values for the samples are plotted against the ambient seawater temperature in Figure 1. The δ88Sr value and the ambient seawater temperature show a weak positive correlation. On the other hand, the Sr/Ca ratios lack temperature dependence, as reported by Yoshimura et al. (2011) and Vielzeuf et al. (2013). The relationship between Δ88Sr and ambient temperature is described as follows:
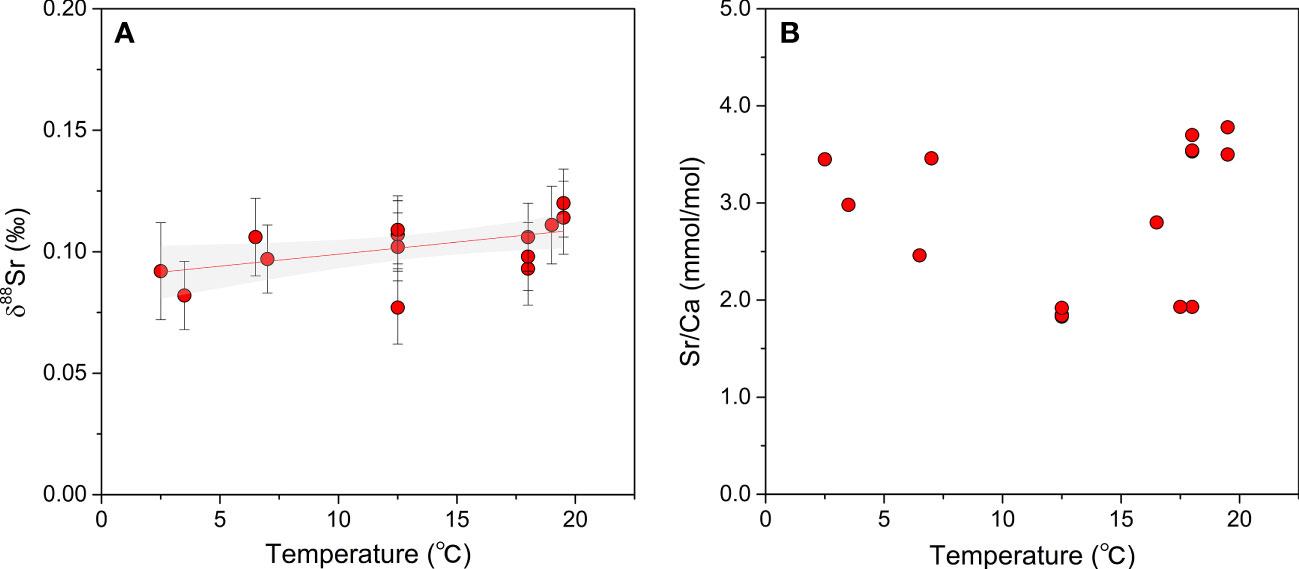
Figure 1 (A) Temperature dependence of Sr isotope fractionation and (B) Sr/Ca in precious corals. The solid line denotes the regression equation: δ88Sr = −0.0867 (± 0.0072) + 0.0011 (± 0.0005) *T (R= 0.51, p< 0.01). The gray shade is 95% confidence intervals. Sr/Ca ratios have previously been reported in Yoshimura et al. (2011).
The correlation between δ88Sr values and temperature was statistically significant (R = 0.51, p< 0.01).
Discussion
Sr partitioning
Coral skeletons grow in a tissue-bounded ECF. As for reef-building corals, some corals can elevate [Ca2+], pH, and therefore [CO32-], of ECF relative to ambient seawater, resulting in greater oversaturated ECFs and rapid skeletal growth (Venn et al., 2011; Sevilgen et al., 2019). McCulloch et al. (2012) reported boron isotope ratios (δ11B), a pH proxy, in scleractinian corals with aragonite skeletons collected from a wide range of water depths and regions and reported that the pH of the internal calcified solution increased by 0.6-0.8 units relative to ambient seawater. The calcification rates of precious corals are much lower by one or two orders of magnitude than those of reef-building corals (Marschal et al., 2004; Luan et al., 2013; Nguyen et al., 2014; Vielfeuf et al., 2018; Iwasaki et al., 2022), since their deep-benthic environment also results in fewer energy resources, leading to less biological modification on ECF chemistry. McCulloch et al. (2012) also measured δ11B of Corallium sp. from one sample depth of 951 m and reported a pH increase estimate of +0.31 units relative to seawater, showing that the pH modification is smaller than for scleractinian corals. In addition, recent in-vivo pH measurement at the site of calcification of octocorals suggests that pH is not up-regulated than the surrounding seawater (Le Goff et al., 2017).
As for cation uptake into ECFs, dissolved Ca of seawater (10.27 mmol/kg) is sufficient for carbonate precipitation. Following cation transports, regardless of passive or active, into ECF, the formation of solid-phase carbonates takes place in supersaturated (Ω > 1) solutions (Gilbert et al., 2022). Subsequently, coral skeletons grow both by particle attachment of precursor phases and ion attachment of ECF solutes. The particles and ions attach to the biomineral growth front, elongating the skeleton, and in the last step, the particles and ions crystallize into crystalline calcium carbonate.
Neither temperature nor other water depth-relevant parameters, such as dissolved inorganic carbon chemistry, exert a predominant control on the Sr content of the precious coral specimens (Yoshimura et al., 2011). The Sr, Mg, Na, Sr, Li, and U concentrations of Corallium skeletons are high in the fast-growing meddular zones and decrease towards slow-growing concentric layers, synchronized with continuous growth decline with age (Vielzeuf et al., 2018). This positive influence of calcite growth and precipitation rates on Sr concentrations is consistent with the experimental calcite precipitation studies (Lorens, 1981; Gabitov and Watson, 2006; Gabitov et al., 2014). In addition, Mg uptake into inorganic calcite has been reported to affect the behavior of trace element incorporation, including Sr: a positive Sr-Mg correlation caused by the Mg-induced lattice distortion effect (Mucci and Morse, 1983). The strong Mg enrichment in the central meddular zones of the skeleton has been reported not only in the Mediterranean (Vielzeuf et al., 2018) but also in precious corals in the Pacific pink coral Pleurocorallium elatius (Tamenori et al., 2014; Yoshimura et al., 2017). Sr enrichment associated with Mg enrichment is not found in the skeletal central axis of P. elatius, and both the center and the outer parts of the bone axis show a very homogeneous Sr distribution (Yoshimura et al., 2017). It has been reported that the growth rates at the center and the outer rim differ by about one order of magnitude (∼2 mm/year for central meddular zones and ∼0.2 mm/year for outer annular zones, see references in Vielzeuf et al., 2018), and the kinetic partitioning effect is characterized as strong for the Mg uptake. However, the distribution of Sr does not necessarily correspond to the growth rate or the growth mode of the skeleton. Furthermore, lattice distortion due to Mg substitution is probably not the primary factor controlling Sr incorporation into precious coral skeletons.
The Sr/Ca values of precious corals correspond to partition coefficient, Kd = (Sr/Ca)CaCO3/(Sr/Ca)Fluid, from 0.21 to 0.43 (Figure 2). The Kd value of inorganic calcite approaches 0.045 for calcite with slow growth rates near equilibrium (Gabitov et al., 2014). AlKhatib and Eisenhauer reported equilibrium Kd of 0.06 at 25°C and 0.09 at 37.5°C, and Lorens (1981) reported 0.021 at 25°C (Lorens, 1981), which is comparable to each other. Inorganic calcite has near-surface layers that are chemically different from the bulk crystal lattice (Gabitov and Watson, 2006). The near-surface layer is enriched in Sr, since they have a large difference in element diffusivity. The distinct surface chemical composition is fully entrapped into the bulk composition when the crystal grows fast. Since Sr is enriched in the near-surface layer, high crystal growth rates lead to a higher Kd value of ~0.3 (Gabitov and Watson, 2006; Gabitov et al., 2014). The Kd value at a high precipitation rate normalized by surface area (R*, μmol/m2/h) recently reported by AlKhatib and Eisenhauer is 0.19 at 37.5°C and 0.24 at 25.0°C (inorganic calcite of Figure 2). The model calculation by DePaolo (2011) estimates 0.24, which is in good agreement with laboratory calcite precipitation experiments. Sr partitioning of geological carbonates has been reported by Cicero and Lohmann (2001). They reported an average Kd of 0.147 for inorganic Holocene calcite, which appears to be in the range of laboratory calcite with high precipitation rates (e.g., Gabitov et al., 2014). In summary, the maximum Kd value for inorganic calcite was around 0.15-0.3, regardless of Mg content or precipitation environments (laboratory/natural).
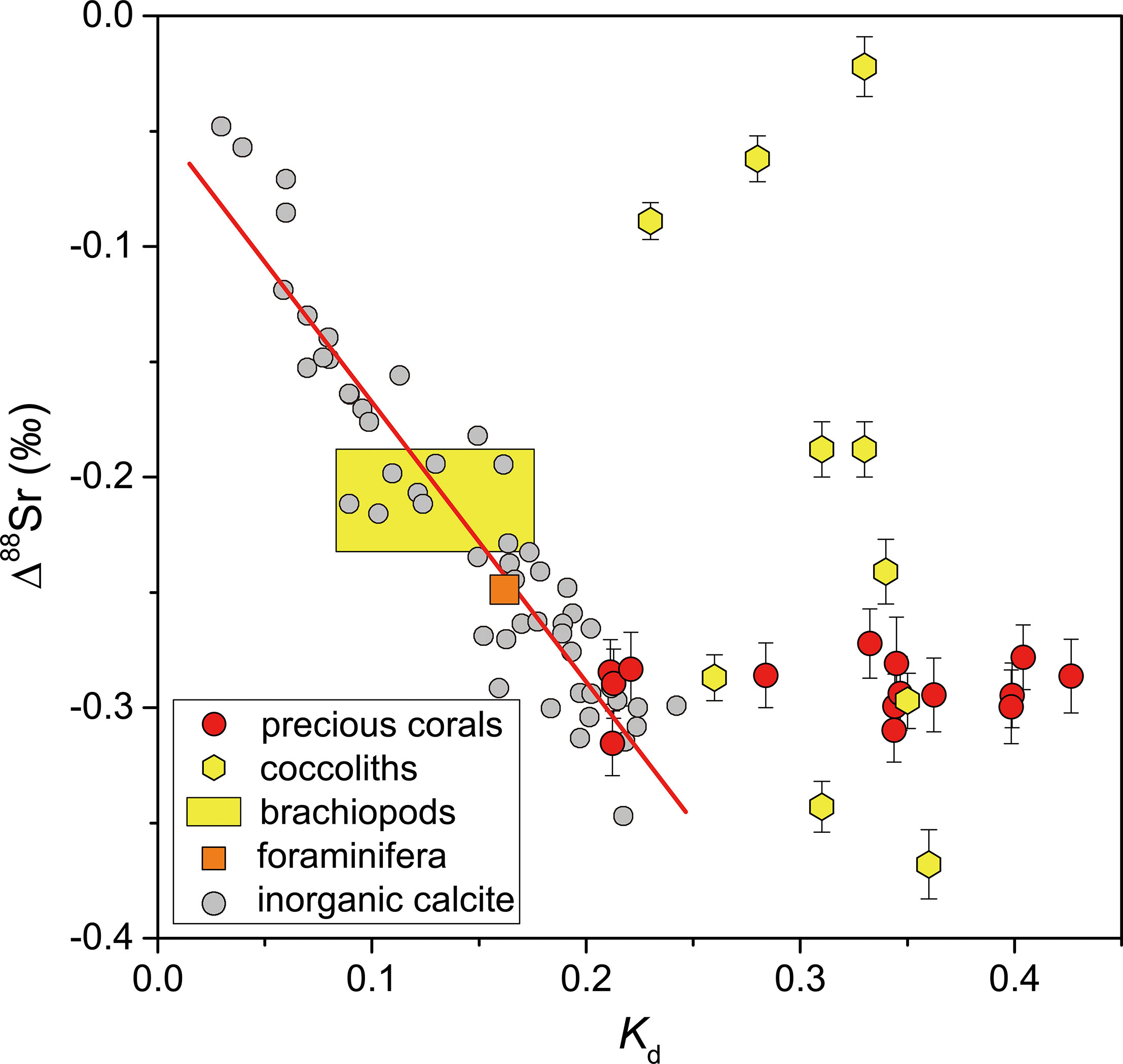
Figure 2 Partition coefficients of Sr (Kd) versus magnitude Sr isotope fractionation from seawater/fluids (Δ88Sr) for biogenic calcite of modern precious corals (this study), coccoliths (Stevenson et al., 2014), brachiopods (Vollstaedt et al., 2014), Holocene foraminifera (Böhm et al., 2012), and inorganic calcite (Böhm et al., 2012; Alkhatib and Eisenhauer, 2017). For brachiopods, only ppm concentration of Sr is available, so the approximate range of Kd is shown in the yellow area with their Δ88Sr values.
The “apparent” Kd observed for precious corals is very high, exceeding inorganic calcite maximum, and likely to be due to the effect of an element-selective active transcellular pathway, rather than the effect of a passive paracellular pathway, in which the Sr/Ca ratio is the same as that of seawater. Ohde and Kitano (1984) reported Sr concentrations and Kd for magnesian calcite in sea urchins, starfish, calcareous algae, and foraminifera, and found that Kd averaged around 0.3 for species with Mg/Ca as high as 100-200 mmol/mol. Their highest Mg/Ca (197 mmol/mol) calcareous algae had a Sr concentration of 2,200 μg/g, corresponding to a Kd of just under 0.4, which is close to our octocoral’s maximum. Assuming a higher Sr/Ca in precious coral’s ECF than ambient seawater is steady-state, the lack of correlation between Kd of the coral skeleton and δ88Sr values indicates that the coral Sr/Ca partitioning has little effect on strontium isotope fractionation.
Stable Sr isotope fractionation
The Δ88Sr of inorganic calcite decreases with increasing at 25°C (Böhm et al., 2012). This trend is further confirmed by experiments varying R* at 12.5°C, 25°C, and 37°C, respectively (Alkhatib and Eisenhauer, 2017). The latter study reported that changes in Δ88Sr may have plateaus when precipitation is low or high. No data on growth rates for individual samples exist. However, the growth rate of precious coral skeletons is often reported to be about 0.3 mm per year, even under different habitat conditions and using other measurement methods. Annual growth rates of 0.20 mm/yr to 0.44 mm/yr were reported by microscopic observation and 210Pb methods for C. japanicum, C. rubrum, and P. elatius (Marschal et al., 2004; Luan et al., 2013; Nguyen et al., 2014; Iwasaki et al., 2022). Although we do not take into account the seasonality of growth, the diurnal cycles, or the presence of growth cessation periods, the log R* of precious corals calculated from the skeletal growth rates can be estimated to be between 2.8 and 3.1 μmol/m2/h. We assumed a skeletal organic matter content of 2.8 wt% (Vielzeuf et al., 2018, n = 12). The relationship between isotopic fractionation and log R* at 12.5°C (Alkhatib and Eisenhauer, 2017), the closest temperature to the temperature of our coral samples, is expressed by the following equation:
The values of Δ88Sr corresponding to log R* of precious corals range from -0.172 to -0.202‰, which is about 0.1‰ higher than the average Δ88Sr of -0.29‰ observed in this study, giving a log R of 4.1 predicted from Eq. 3.
Δ88Sr of inorganic calcite is affected primarily by precipitation rate but also varies with temperature. For the same R*, δ88Sr values increase with increasing temperature. The difference of Δ88Sr between 12.5°C and 37.5°C is about 0.1‰. Although the relationship between Δ88Sr and temperature change was not always linear, it was thought to average about 0.004‰/°C as a function of temperature increase (Alkhatib and Eisenhauer, 2017). This predicted slope of temperature dependence is four times larger than that observed for precious corals (Figure 1). The difference in temperature sensitivity is related to the nonlinearity between Δ88Sr and temperature and between Δ88Sr and precipitation rate R*. The positive correlation between temperature dependence and Δ88Sr for inorganic precipitation and precious corals is a common feature, but the slope of the temperature dependence is not a constant rate with respect to changes in temperature because it is also affected by the precipitation rate. Consequently, the conditions for skeletal growth in precious corals may correspond to conditions for crystal growth where isotope ratio changes are less occur.
Element partitioning of Sr/Ca, and isotopic fractionation of 88Sr/86Sr in calcite are linked to each other, with a strong linear inverse correlation between Kd and Δ88Sr (Alkhatib and Eisenhauer, 2017):
This equation depends primarily on the precipitation rate and is thought to be a constant relationship regardless of the biogenic or inorganic origin of the calcite (Figure 2). Böhm et al. (2012) reported Δ88Sr for authigenic calcite in basalt crust. The Mg/Ca values of these basalt crust calcite samples are 53.6 and 57.8 mmol/mol (Böhm et al., 2012; Rausch et al., 2013), the lower range of high Mg calcite (defined as Mg/Ca > 40 mmol/mol). The precipitation rate of these samples is very slow, but the correlations between Δ88Sr and Kd as well as the precipitation rate are represented by the same regression equation as for laboratory low Mg calcite with a high precipitation rate (Böhm et al., 2012; Alkhatib and Eisenhauer, 2017).
Elemental partitioning of biogenic carbonates is generally known to be under the influence of biological selectivity, e.g., the changes in growth rate, but a characteristic feature of precious coral δ88Sr is a constant independent of Kd (Figure 2). The elemental partition coefficients for corals are determined using values for seawater and skeletons, but Sr/Ca partitioning may also occur during transport to seawater and ECFs. The timing of evolutional divergence of the major taxa of the phylum Cnidaria (Hexacorallia, Octocorallia, and Medusozoa) has been examined by Park et al. (2012) based on mitochondrial DNA sequences. Octocorallia and scleractinia may have differentiated from the survivors of the Permian-Triassic mass extinction, which is in agreement with the fossil record (Park et al., 2012). There have been reports on reef-building corals and jellyfish, although to what extent there are common processes in the selectivity of Sr and Ca in cnidarian calcification. Studies using inhibitors of cellular ion transport have demonstrated that the Ca-ATPase and Ca channels of reef-building corals transport a constant ratio of Sr2+ to Ca2+ present in seawater (Allison et al., 2011). Thus, element partitioning in the reef-building coral’s transcellular pathway is not selective for either Sr or Ca. Calcium channels in jellyfish, another group of cnidarians, have a marked selectivity between Ca and Sr ions (Jeziorski et al., 1998), indicating that Ca2+ channels are more permeable to Sr than to Ca. There are two kinds of Sr transport for the ECF: active transcellular and passive paracellular pathways. The δ88Sr composition of the ECF may be modified from the seawater value of 0.392‰ by the former active transport by protein chelation and ion channels. The latter passive pathway yields no isotope fractionation from ambient seawater. Therefore, the Sr isotopic fractionation in octocoral is expected to be offset from inorganic precipitation by active transport. δ88Sr is homogeneous regardless of the sample, but Kd is expected to vary. Since Sr/Ca is independent of δ88Sr, the strength of elemental selectivity does not seem to affect the magnitude of isotopic fractionation.
After transport to the ECF, element partitioning of calcite occurs. The Rayleigh fractionation affects skeletal chemical composition resulting from the effect on the discrimination or preferential uptake of trace element ions relative to Ca in carbonate and the rate at which the ECF element reservoir is replaced. The Rayleigh fractionation behavior differs depending on whether the partition coefficient is greater or less than 1, and for the Sr/Ca ratio of calcite, it is less than 0.3, as explained in 4.1. Therefore, as calcite precipitation proceeds in the solution reservoir, Ca is preferentially consumed and trace elements are relatively enriched. A proportional relationship between the fraction of element reservoir used for calcification and the Sr/Ca ratio of the precious coral skeleton is expected. The influence of this type of element partitioning is proposed by the synchrony of growth rates and multi-element partitioning behavior in precious corals (Vielzeuf et al., 2018). Skeletal sites with high Sr/Ca ratios correspond to periods of high growth rates, but for the range of Sr/Ca ratios we measured here, δ88Sr is independent of growth rates and associated biological or mineralogical element uptake processes. Precious coral will allow us to reconstruct δ88Sr in seawater with a high degree of accuracy.
It is widely known that most foraminifera takes up Mg at concentrations that are one to two orders of magnitude lower than those of inorganic calcite, which is also about 40% lower with respect to Sr (de Nooijer et al., 2014). Along with this biological element discrimination effect, the presence or absence of isotopic fractionation of Sr has already been reported by Böhm et al. (2012). Foraminifera in biogenic calcite are also plotted in the same precipitation rate versus isotope fractionation equation (Böhm et al., 2012; Alkhatib and Eisenhauer, 2017, Figures 2 and 3), and they propose that element partitioning by foraminifera does not affect Δ88Sr. In addition, brachiopods are also generally in the range of inorganic fractionation (Figures 2 and 3). This is either because the contribution of passive transport is relatively large in these two groups of organisms. On the contrary, the shift of δ88Sr of three coccolith species (Emiliania huxleyi, Coccolithus pelagicus spp. braarudii, Gephyrocapsa oceanica) to lighter values is explained by an increase in growth rate with temperature (Stevenson et al., 2014, Figure 3). Since the calcification of coccoliths takes place intracellularly, Sr/Ca ratios of coccolithophores are related to differences in the relative pumping rates of Sr and Ca ions across the cell membrane. As temperature increases, the selectivity for Sr transport is expected to decrease, increasing the Sr incorporation into the shells (Rickaby et al., 2002). At the same time, the higher reactivity of the lighter isotope of Sr (86Sr) is explained by its preferential incorporation into the calcite (Stevenson et al., 2014). The formation of coral skeletons takes place extracellularly, and consequently, the magnitude of Δ88Sr is the very narrow range of −0.291 ± 0.023‰ compared to a large isotopic variation (~0.4‰) of intracellular coccolith calcification.
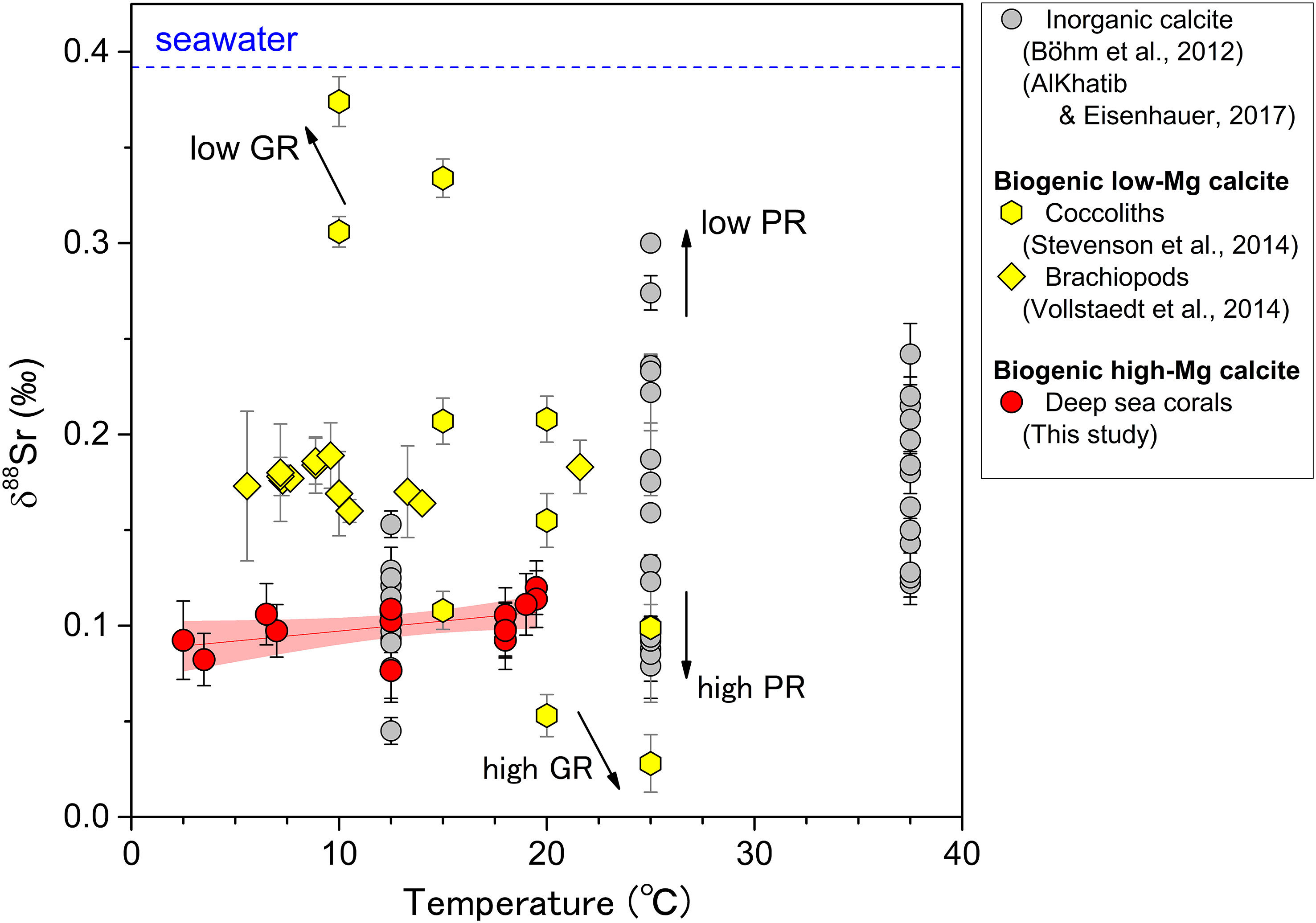
Figure 3 Compilation of δ88Sr versus temperature data for inorganic and biogenic calcite. Yellow and red symbols represent low-Mg calcite and high-Mg biogenic calcite, respectively. The variation of inorganic carbonates within each temperature is due to the large influence of the precipitation rate (PR, Böhm et al., 2012; Alkhatib and Eisenhauer, 2017). GR is abbreviations for growth rate for coccoliths (Stevenson et al., 2014).
Fruchter et al. (2016) conducted experiments on the precipitation of inorganic aragonite from natural seawater and reported that their δ88Sr averaged 0.215 ± 0.046‰, -0.18‰ lower than seawater. The cold-water coral skeleton of aragonite by Raddatz et al. (2013) averaged 0.189 ± 0.032 ‰, almost the same as inorganic precipitated aragonite. In addition, the aragonite skeleton of reef-building corals is also consistent with inorganic precipitation at ~0.20‰ (Fruchter et al., 2016). The octocorals showed 0.101‰, which is 0.088‰ lower than cold-water coral, and has an offset of -0.1‰ compared to inorganic calcite. This difference between aragonite and calcite corals with inorganic carbonate may be due to the different elemental partitioning of ion channels between coral types.
Conclusions
The stable isotope ratio of Sr in precious coral skeletons composed of high-Mg calcite is reported for the first time and is found to be uniform regardless of the locality and water depth and is independent of the Sr/Ca ratio. There appears to be an offset of -0.1‰ compared to inorganic calcite with the same precipitation rate, and cell-mediated Sr transport appears to have a constant contribution rate. Because the uniform δ88Sr values indicate that contributions of transcellular and paracellular ion pathways to ECF remain constant, no significant interspecific differences exist, and the magnitude of isotope fractionation between skeleton and seawater is unique to precious corals (Δ88Sr = −0.291‰). By selecting well-preserved samples, the Sr isotope ratios of seawater during geologic time can be precisely reconstructed. In addition, regarding the modern use of these characteristics of skeletal chemical composition, the minimal range of Sr isotopic ratios could be used to determine the authenticity of precious coral skeletons.
Data availability statement
The original contributions presented in the study are included in the article/supplementary material. Further inquiries can be directed to the corresponding author.
Author contributions
TY and SW contributed to conception of the study. NI managed all sampling surveys, and research cruises, and collected the coral samples. SW and TI conducted isotopic analysis. TY, SW, NI, TI and NO contributed to discussion, manuscript writing, and approved the submitted version.
Funding
This work was performed with the support of Japan Society for the Promotion of Science (JSPS) to TY (no. 21H01204) and SW (no. 18K03814).
Acknowledgments
The constructive and insightful suggestions of the reviewers greatly improved the manuscript. We express appreciation to the crew of the R/V Hakurei-maru No. 2, Fukada Salvage & Marine Works, Mr. Kawaguchi, Mr. Osato, Kochi Prefectural Deep Seawater Laboratory, and Geological Survey of Japan for providing the precious coral specimens.
Conflict of interest
The authors declare that the research was conducted in the absence of any commercial or financial relationships that could be construed as a potential conflict of interest.
Publisher’s note
All claims expressed in this article are solely those of the authors and do not necessarily represent those of their affiliated organizations, or those of the publisher, the editors and the reviewers. Any product that may be evaluated in this article, or claim that may be made by its manufacturer, is not guaranteed or endorsed by the publisher.
References
Adkins J. F. (1998). Deep-sea coral evidence for rapid change in ventilation of the deep north Atlantic 15,400 years ago. Science 280, 725–728. doi: 10.1126/science.280.5364.725
Alkhatib M., Eisenhauer A. (2017). Calcium and strontium isotope fractionation in aqueous solutions as a function of temperature and reaction rate; i. calcite. Geochimica Cosmochimica Acta 209, 296–319. doi: 10.1016/j.gca.2016.09.035
Allemand D., Grillo M. C. (1992). Biocalcification mechanism in gorgonians: 45Ca uptake and deposition by the Mediterranean red coral Corallium rubrum. J. Exp. Zoology 262, 237–246. doi: 10.1002/jez.1402620302
Allison N., Cohen I., Finch A. A., Erez J. (2011). Controls on Sr/Ca and Mg/Ca in scleractinian corals: The effects of Ca-ATPase and transcellular Ca channels on skeletal chemistry. Geochimica Cosmochimica Acta 75, 6350–6360. doi: 10.1016/j.gca.2011.08.012
Böhm F., Eisenhauer A., Tang J., Dietzel M., Krabbenhöft A., Kisakürek B., et al. (2012). Strontium isotope fractionation of planktic foraminifera and inorganic calcite. Geochimica Cosmochimica Acta 93, 300–314. doi: 10.1016/j.gca.2012.04.038
Böhm F., Gussone N., Eisenhauer A., Dullo W.-C., Reynaud S., Paytan A. (2006). Calcium isotope fractionation in modern scleractinian corals. Geochimica Cosmochimica Acta 70, 4452–4462. doi: 10.1016/j.gca.2006.06.1546
Cicero A. D., Lohmann K. C. (2001). Sr/Mg variation during rock-water interaction: Implications for secular changes in the elemental chemistry of ancient seawater. Geochimica Cosmochimica Acta 65, 741–761. doi: 10.1016/S0016-7037(00)00594-9
de Nooijer L. J., Spero H. J., Erez J., Bijma J., Reichart G. J. (2014). Biomineralization in perforate foraminifera. Earth-Science Rev. 135, 48–58. doi: 10.1016/j.earscirev.2014.03.013
DePaolo D. J. (2011). Surface kinetic model for isotopic and trace element fractionation during precipitation of calcite from aqueous solutions. Geochimica Cosmochimica Acta 75, 1039–1056. doi: 10.1016/j.gca.2010.11.020
Eltgroth S. F., Adkins J. F., Robinson L. F., Southon J., Kashgarian M. (2006). A deep-sea coral record of north Atlantic radiocarbon through the younger dryas: Evidence for intermediate water/deepwater reorganization. Paleoceanography 21, PA4207. doi: 10.1029/2005PA001192
Fietzke J., Eisenhauer A. (2006). Determination of temperature-dependent stable strontium isotope (88Sr/86Sr) fractionation via bracketing standard MC-ICP-MS. Geochemistry Geophysics Geosystems 7, Q08009. doi: 10.1029/2006GC001243
Fruchter N., Eisenhauer A., Dietzel M., Fietzke J., Böhm F., Montagna P., et al. (2016). 88Sr/86Sr fractionation in inorganic aragonite and in corals. Geochimica Cosmochimica Acta 178, 268–280. doi: 10.1016/j.gca.2016.01.039
Gabitov R., Sadekov A., Leinweber A. (2014). Crystal growth rate effect on Mg/Ca and Sr/Ca partitioning between calcite and fluid: An in situ approach. Chem. Geology 367, 70–82. doi: 10.1016/j.chemgeo.2013.12.019
Gabitov R. I., Watson E. B. (2006). Partitioning of strontium between calcite and fluid. Geochemistry Geophysics Geosystems 7, Q11004. doi: 10.1029/2005GC001216
Gilbert P. U., Bergmann K. D., Boekelheide N., Tambutté S., Mass T., Marin F., et al. (2022). Biomineralization: Integrating mechanism and evolutionary history. Sci. Adv. 8, eabl9653. doi: 10.1126/sciadv.abl9653
Gothmann A. M., Stolarski J., Adkins J. F., Higgins J. A. (2017). A Cenozoic record of seawater mg isotopes in well-preserved fossil corals. Geology 45, 1039–1042. doi: 10.1130/G39418.1
Inoue M., Gussone N., Koga Y., Iwase A., Suzuki A., Sakai K., et al. (2015). Controlling factors of Ca isotope fractionation in scleractinian corals evaluated by temperature, pH and light controlled culture experiments. Geochimica Cosmochimica Acta 167, 80–92. doi: 10.1016/j.gca.2015.06.009
Iwasaki N., Hasegawa H., Tamenori Y., Kikunaga M., Yoshimura T., Sawai H. (2022). Synchrotron μ-XRF mapping analysis of trace elements in in-situ cultured Japanese red coral, Corallium japonicum. PeerJ 10, e13931. doi: 10.7717/peerj.13931
Jeziorski M. C., Greenberg R. M., Clark K. S., Anderson P. A. (1998). Cloning and functional expression of a voltage-gated calcium channel α1 subunit from jellyfish. J. Biol. Chem. 273, 22792–22799. doi: 10.1074/jbc.273.35.22792
Krabbenhöft A., Eisenhauer A., Böhm F., Vollstaedt H., Fietzke J., Liebetrau V., et al. (2010). Constraining the marine strontium budget with natural strontium isotope fractionations (87Sr/86Sr∗, δ88/86Sr) of carbonates, hydrothermal solutions and river waters. Geochimica Cosmochimica Acta 74, 4097–4109. doi: 10.1016/j.gca.2010.04.009
Krabbenhöft A., Fietzke J., Eisenhauer A., Liebetrau V., Böhm F., Vollstaedt H. (2009). Determination of radiogenic and stable strontium isotope ratios (87Sr/86Sr; δ88/86Sr) by thermal ionization mass spectrometry applying an 87Sr/84Sr double spike. J. Analytical Atomic Spectrometry 24, 1967–1971. doi: 10.1039/b906292k
Lebrato M., Garbe-Schönberg D., Müller M. N., Blanco-Ameijeiras S., Feely R. A., Lorenzoni L., et al. (2020). Global variability in seawater Mg:Ca and Sr:Ca ratios in the modern ocean. Proc. Natl. Acad. Sci. 117, 22281–22292. doi: 10.1073/pnas.1918943117
Le Goff C., Tambutté E., Venn A., Techer N., Allemand D., Tambutté S. (2017). In vivo pH measurement at the site of calcification in an octocoral. Sci. Rep. 7, 1–14. doi: 10.1038/s41598-017-10348-4
Levitus S., Boyer T. P. (1994). World ocean atlas 1994, vol 4: temperature number 4 (Washington: US Government Printing Office).
Li W., Liu X. M., Wang K., Hu Y., Suzuki A., Yoshimura T. (2022). Potassium incorporation and isotope fractionation in cultured scleractinian corals. Earth Planetary Sci. Lett. 581, 117393. doi: 10.1016/j.epsl.2022.117393
Lorens R. B. (1981). S.R.X.X.X, cd, Mn and Co distribution coefficients in calcite as a function of calcite precipitation rate. Geochimica Cosmochimica Acta 45, 553–561. doi: 10.1016/0016-7037(81)90188-5
Luan N. T., Rahman M. A., Maki T., Iwasaki N., Hasegawa H. (2013). Growth characteristics and growth rate estimation of Japanese precious corals. J. Exp. Mar. Biol. Ecol. 441, 117–125. doi: 10.1016/j.jembe.2013.01.012
Marschal C., Garrabou J., Harmelin J. G., Pichon M. (2004). A new method for measuring growth and age in the precious red coral Corallium rubrum (L.). Coral Reefs 23, 423–432. doi: 10.1007/s00338-004-0398-6
McCulloch M., Trotter J., Montagna P., Falter J., Dunbar R., Freiwald A., et al. (2012). Resilience of cold-water scleractinian corals to ocean acidification: Boron isotopic systematics of pH and saturation state up-regulation. Geochimica Cosmochimica Acta 87, 21–34. doi: 10.1016/j.gca.2012.03.027
Mucci A., Morse J. W. (1983). The incorporation of Mg2+ and Sr2+ into calcite overgrowths: Influences of growth rate and solution composition. Geochimica Cosmochimica Acta 47, 217–233. doi: 10.1016/0016-7037(83)90135-7
Neymark L. A., Premo W. R., Mel'nikov N. N., Emsbo P. (2014). Precise determination of δ88Sr in rocks, minerals, and waters by double-spike TIMS: A powerful tool in the study of geological, hydrological and biological processes. J. Analytiacal Atomic Spectrometry 29, 65–75. doi: 10.1039/C3JA50310K
Nguyen L. T., Rahman M. A., Maki T., Tamenori Y., Yoshimura T., Suzuki A., et al. (2014). Distribution of trace element in Japanese red coral Paracorallium japonicum by μ-XRF and sulfur speciation by XANES: Linkage between trace element distribution and growth ring formation. Geochimica Cosmochimica Acta 127, 1–9. doi: 10.1016/j.gca.2013.11.023
Ohde S., Kitano Y. (1984). Coprecipitation of strontium with marine Ca-mg carbonates. Geochemical J. 18, 143–146. doi: 10.2343/geochemj.18.143
Park E., Hwang D. S., Lee J. S., Song J. I., Seo T. K., Won Y. J. (2012). Estimation of divergence times in cnidarian evolution based on mitochondrial protein-coding genes and the fossil record. Mol. Phylogenet. Evol. 62, 329–345. doi: 10.1016/j.ympev.2011.10.008
Paytan A., Griffith E. M., Eisenhauer A., Hain M. P., Wallmann K., Ridgwell A. (2021). A 35-million-year record of seawater stable Sr isotopes reveals a fluctuating global carbon cycle. Science 371, 1346–1350. doi: 10.1126/science.aaz9266
Pearce C. R., Parkinson I. J., Gaillardet J., Charlier B. L. A., Mokadem F., Burton K. W. (2015). Reassessing the stable (δ88/86Sr) and radiogenic (87Sr/86Sr) strontium isotopic composition of marine inputs. Geochimica Cosmochimica Acta 157, 125–146. doi: 10.1016/j.gca.2015.02.029
Raddatz J., Liebetrau V., Rüggeberg A., Hathorne E., Krabbenhöft A., Eisenhauer A., et al. (2013). Stable Sr-isotope, Sr/Ca, Mg/Ca, Li/Ca and Mg/Li ratios in the scleractinian cold-water coral lophelia pertusa. Chem. Geology 352, 143–152. doi: 10.1016/j.chemgeo.2013.06.013
Rausch S., Böhm F., Bach W., Klügel A., Eisenhauer A. (2013). Calcium carbonate veins in ocean crust record a threefold increase of seawater Mg/Ca in the past 30 million years. Earth Planetary Sci. Lett. 362, 215–224. doi: 10.1016/j.epsl.2012.12.005
Rickaby R., Schrag D., Zondervan I., Riebesell U. (2002). Growth rate dependence of Sr incorporation during calcification of emiliania huxleyi. Global Biogeochemical Cycles 16, 6–1-6-8. doi: 10.1029/2001GB001408
Rüggeberg A., Fietzke J., Liebetrau V., Eisenhauer A., Dullo W.-C., Freiwald A. (2008). Stable strontium isotopes (δ88/86Sr) in cold-water corals — a new proxy for reconstruction of intermediate ocean water temperatures. Earth Planetary Sci. Lett. 269, 570–575. doi: 10.1016/j.epsl.2008.03.002
Sevilgen D. S., Venn A. A., Hu M. Y., Tambutté E., De Beer D., Planas-Bielsa V., et al. (2019). Full in vivo characterization of carbonate chemistry at the site of calcification in corals. Sci. Adv. 5, eaau7447. doi: 10.1126/sciadv.aau7447
Sherwood O. A., Risk M. J. (2007). “Deep-Sea Corals: New insights to paleoceanography,” in Proxies in Late Cenozoic Paleoceanography, ed. C. Hillaire–Marcel, and A. D. Vernal (Amsterdam: Elsevier Inc.) 491–522.
Stevenson E. I., Hermoso M., Rickaby R. E. M., Tyler J. J., Minoletti F., Parkinson I. J., et al. (2014). Controls on stable strontium isotope fractionation in coccolithophores with implications for the marine Sr cycle. Geochimica Cosmochimica Acta 128, 225–235. doi: 10.1016/j.gca.2013.11.043
Tamenori Y., Yoshimura T., Nguyen T. L., Hasegawa H., Suzuki A., Kawahata H., et al. (2014). Identification of the chemical form of sulfur compounds in the Japanese pink coral (Corallium elatius) skeleton using μ-XRF/XAS speciation mapping. J. Struct. Biol. 186, 214–223. doi: 10.1016/j.jsb.2014.04.001
van Oppen M. J. H., Mieog J. C., Sanchez C. A., Fabricius K. E. (2005). Diversity of algal endosymbionts (zooxanthellae) in octocorals: The roles of geography and host relationships. Mol. Ecol. 14, 2403–2417. doi: 10.1111/j.1365-294X.2005.02545.x
Venn A., Tambutté E., Holcomb M., Allemand D., Tambutté S. (2011). Live tissue imaging shows reef corals elevate pH under their calcifying tissue relative to seawater. PloS One 6, e20013. doi: 10.1371/journal.pone.0020013
Vielzeuf D., Gagnon A. C., Ricolleau A., Devidal J.-L., Balme-Heuze C., Yahiaoui N., et al. (2018). Growth kinetics and distribution of trace elements in precious corals. Front. Earth Sci. 6, 167. doi: 10.3389/feart.2018.00167
Vielzeuf D., Garrabou J., Gagnon A., Ricolleau A., Adkins J., Günther D., et al. (2013). Distribution of sulphur and magnesium in the red coral. Chem. Geology 355, 13–27. doi: 10.1016/j.chemgeo.2013.07.008
Vollstaedt H., Eisenhauer A., Wallmann K., Böhm F., Fietzke J., Liebetrau V., et al. (2014). The phanerozoic δ88/86Sr record of seawater: New constraints on past changes in oceanic carbonate fluxes. Geochimica Cosmochimica Acta 128, 249–265. doi: 10.1016/j.gca.2013.10.006
Wakaki S., Obata H., Tazoe H., Ishikawa T. (2017). Precise and accurate analysis of deep and surface seawater Sr stable isotopic composition by double-spike thermal ionization mass spectrometry. Geochemical J. 51, 227–239. doi: 10.2343/geochemj.2.0461
Yoshimura T., Tamenori Y., Suzuki A., Kawahata H., Iwasaki N., Hasegawa H., et al. (2017). Altervalent substitution of sodium for calcium in biogenic calcite and aragonite. Geochimica Cosmochimica Acta 202, 21–38. doi: 10.1016/j.gca.2016.12.003
Yoshimura T., Tanimizu M., Inoue M., Suzuki A., Iwasaki N., Kawahata H. (2011). Mg isotope fractionation in biogenic carbonates of deep-sea coral, benthic foraminifera, and hermatypic coral. Analytical Bioanalycal Chem. 401, 2755–2769. doi: 10.1007/s00216-011-5264-0
Yoshimura T., Wakaki S., Ishikawa T., Gamo T., Araoka D., Ohkouchi N., et al. (2020). A systematic assessment of stable Sr isotopic compositions of vent fluids in arc/back-arc hydrothermal systems: Effects of host rock type, phase separation, and overlying sediment. Front. Earth Sci. 8, 591711. doi: 10.3389/feart.2020.591711
Keywords: strontium, stable isotope ratio, coral, TIMS, biomineralization
Citation: Yoshimura T, Wakaki S, Iwasaki N, Ishikawa T and Ohkouchi N (2022) Stable Sr isotope (88Sr/86Sr) fractionation in calcite precious corals. Front. Mar. Sci. 9:1045909. doi: 10.3389/fmars.2022.1045909
Received: 16 September 2022; Accepted: 18 November 2022;
Published: 01 December 2022.
Edited by:
Ed Hathorne, Helmholtz Association of German Research Centres (HZ), GermanyReviewed by:
Jacek Raddatz, Goethe University Frankfurt, GermanyFlorian Böhm, Helmholtz Association of German Research Centres (HZ), Germany
Copyright © 2022 Yoshimura, Wakaki, Iwasaki, Ishikawa and Ohkouchi. This is an open-access article distributed under the terms of the Creative Commons Attribution License (CC BY). The use, distribution or reproduction in other forums is permitted, provided the original author(s) and the copyright owner(s) are credited and that the original publication in this journal is cited, in accordance with accepted academic practice. No use, distribution or reproduction is permitted which does not comply with these terms.
*Correspondence: Toshihiro Yoshimura, eW9zaGltdXJhdEBqYW1zdGVjLmdvLmpw