- 1Key Laboratory of Tropical Marine Bio-resources and Ecology, South China Sea Institute of Oceanology, Chinese Academy of Sciences, Guangzhou, China
- 2University of Chinese Academy of Sciences, Beijing, China
- 3Guangdong Provincial Key Laboratory of Applied Marine Biology, South China Sea Institute of Oceanology, Chinese Academy of Sciences, Guangzhou, China
- 4Southern Marine Science and Engineering Guangdong Laboratory, Guangzhou, China
- 5Innovation Academy of South China Sea Ecology and Environmental Engineering, Chinese Academy of Sciences, Guangzhou, China
Copper (Cu) uptake and trophic transfer in marine plankton at the environmentally relevant levels have rarely been investigated. In this study, we investigated the Cu uptake and subcellular distribution in five phytoplankton (Isochrysis zhanjiangensis, Phaeocystis sp., Isochrysis galbana, Chaetoceros sp. and Phaeodactylum tricornutum) belonging to two taxa Chrysophyta and Bacillariophyta, and the following trophic transfer to rotifer Brachionus plicatilis by using stable isotope tracing method. In 2-hour exposure, Cu uptake rates were 20.8–60.3 amol h-1 cell-1 (amol = 10-18 mol) in the phytoplankton exposure to 4.82 × 10-13 mol L-1 free Cu activity ([Cu2+]). Cu uptake rates were increased with the increase of [Cu2+] in all the phytoplankton species. Generally, Cu uptake rate constants (kus) were lower in Bacillariophyta (Chaetoceros sp. and P. tricornutum) than Chrysophyta (I. zhanjiangensis, Phaeocystis sp. and I. galbana). While in long-term (6 day) exposure, more Cu was accumulated in the Bacillariophyta than in Chrysophyta, suggesting Bacillariophyta might have more Cu capacity than Chrysophyta. Size effects of cells on Cu uptake were not observed among these five phytoplankton. Cu was mainly distributed in the heat-denatured protein plus organelle fraction and head-stable protein fraction in all the five phytoplankton. The Cu assimilation efficiencies (AEs) in rotifer feeding I. zhanjiangensis, Phaeocystis sp., I. galbana, Chaetoceros sp. and P. tricornutum were 63.4%, 57.6%, 60.2%, 77.4% and 14.6%, respectively. These Cu AEs were positively correlated to the Cu distribution in the trophically available metal fraction (TAM) in different phytoplankton. These results herein demonstrated that different marine phytoplankton had different strategies to accumulate Cu that influence Cu transfer to the predators. Therefore, the variation of the phytoplankton community will change the Cu biogeochemistry in marine environment.
Introduction
Copper (Cu) is an essential but highly toxic trace metal to marine phytoplankton (Andresen et al., 2018; Tsvetkov et al., 2022). Due to the development of human society, a lot of Cu is discharged into marine environment through industrial emission, mining industry, and agricultural waste. It is considered that marine phytoplankton need to cope with the Cu in seawater (total dissolved Cu concentrations of 10-9–10-8 mol L-1) for their biological requirements (10-21 to 10-18 mol Cu cell-1), and their ability to do so was taxa specific (Quigg et al., 2006). However, there have been rare studies working on Cu uptake in marine phytoplankton at environmentally relevant levels comparing to other trace metals, since it is difficult to exclude the high background Cu levels in cells by normal Cu exposure method, and it is also difficult to trace Cu uptake by the very short half-life radio-isotopes (12.70 h for 64Cu and 61.83 h for 67Cu). Recently, a new tracing method was applied to study Cu bioaccumulation in marine organisms by using stable Cu isotopes (63Cu or 65Cu), allowing us to further investigate Cu bioaccumulation in phytoplankton (Guo et al., 2016; Chen et al., 2022).
It has been generally accepted that phytoplankton absorb Cu from surrounding water following the free ion activity model (FLAM), the same pattern as the other trace metals (Sunda and Guillard, 1976). Therefore, Cu uptake in phytoplankton relies on many environmental conditions, including pH (Zeng et al., 2010), salinity (Gonzalez-Davila et al., 1995), nutrients (Miao and Wang, 2007) and so on. Previous studies usually focused on the toxic effects of Cu, in which the free Cu activities [Cu2+] usually ranged from 10-12–10-8 mol L-1. However, [Cu2+] was usually lower than 10-12, even to 10-15 mol L-1 in offshore seawater, due to the physical and chemical properties of seawater (Li et al., 2011; Kong, 2022). Previous Cu uptake kinetic studies demonstrated that phytoplankton had a low-affinity and a high-affinity Cu uptake systems, depended on surrounding Cu concentration (Kong and Price, 2020). In some species like Synechococcus sp., Scenedesmus subspicatus and Thalassiosira weissflogii, the half-saturation constants (Km) were 10-11–10-12 mol L-1 [Cu2+] in the low-affinity Cu system and 10-14–10-15 mol L-1 [Cu2+] in the high-affinity Cu system (Knauer et al., 1997; Croot et al., 2003; Guo et al., 2010). It should be noticed that those previous studies were mainly working on the model phytoplankton species. What happening in the common species in the natural marine environment was still limited to understand.
It is also important to compare Cu uptake and bioaccumulation among different phytoplankton species. Due to the global climate change and anthropogenic effects (e.g. eutrophication), the alternation of phytoplankton community occurred commonly in many coastal areas. For example, although Bacillariophyta are the most abundant taxa, the proportion of Chrysophyta is rising in the coastal seawater of South China Sea over the past decades (Wang et al., 2021). Therefore, the alteration of phytoplankton community may change the Cu biogeochemistry in marine environment if Cu bioaccumulation varies intensively among different taxa/species. Although Cu uptake has been investigated in some model species belonging to marine Cyanophyceae, Bacillariophyceae, Coccolithophyceae, and Dinophyceae in different references, the results among them are hardly to compare since the big differences in the culture condition, Cu exposure concentrations, uptake duration, analytical methods, and so on (Croot et al., 1999; Quigg et al., 2006; Guo et al., 2012; Walsh and Ahner, 2014). One of the promising studies was Quigg et al. (2006), in which, Cu uptake was compared in two phytoplankton with huge difference in size, Cyanophyceae Synechococcus sp. and Bacillariophyceae Thalassiosira weissflflogii. In that study, size was considered as the determined factor to explain the different Cu uptake rates among phytoplankton species. While, whether size effects work between eukaryotic taxa such as Bacillariophyta and Chrysophyta has not been known.
After absorbing Cu from seawater, phytoplankton also has several strategies to preserve intracellular concentrations of metals. It has been proposed that Cu can be chelated to the intracellular biomolecules (such as metallothionine and GSH), sequestrated as insoluble metal complexes (e.g., metal sulfides), trapped in cell surfaces, eliminated from cells, and so on (Quigg et al., 2006). Recently, a subcellular fractionation method was applied to investigate the comprehensive strategies of metal bioaccumulation in microalgae (Wang and Wang, 2008). By this method, cells can be divided into the head-stable protein (HSP), heat-denatured protein (HDP), organelle, metal-rich granules fraction (MRG), and cellular debris (CD) fraction. Among them, metals distributed in the HSP and MRG were considered as the storage and detoxification pool, metals distributed in the HDP and organelle fractions were considered as the function or toxicity pool. Moreover, metals in HSP, HDP and organelle were considered to be trophically available metal pool (TAM) (Ng and Wang, 2005; Zhang and Wang, 2006). Again, because of the difficulties of Cu tracing technique, the subcellular distribution of Cu has not been clear yet.
Therefore, the objectives of this study were to investigate Cu uptake and subcellular distribution in different marine phytoplankton by using stable Cu tracing method. sp. Five phytoplankton belonging to two taxa Chrysophyta (Isochrysis zhanjiangensis, Phaeocystis sp., Isochrysis galbana) and Bacillariophyta (Chaetoceros sp. and Phaeodactylum tricornutum) were employed. sp. sp.They are common species in coastal seawater or widely used in aquaculture sp. Moreover, as the trophic transfer of Cu from phytoplankton to zooplankton was also important for Cu biogeochemistry, a trophic transfer experiment was conducted in an estuary model zooplankton, rotifer Brachionus plicatilis.
Material and method
Phytoplankton and zooplankton cultivation
Five marine phytoplankton species were isolated from the South China Sea or obtained from the National Center of Marine Algae and Microbes (NCMA), including three Chrysophyta (Isochrysis zhanjiangensis, Phaeocystis sp., Isochrysis galbana CCMP 1323), and two Bacillariophyta (Chaetoceros sp. and Phaeodactylum tricornutum CCMP 630), and kept in lab for generations. The information of Isochrysis zhanjiangensis and Phaeocystis sp. were introduced by Hu et al. (2014) and Chaetoceros sp. SCSIO-45851 were isolated from the coastal water of Wenchang, Hainan, China. The algae were maintained in the f/2 medium under the illumination of 4500 Lux and a 14:10 h light/dark (L/D) cycle at 23°C. The 30‰ seawater used in culture was collected in Daya Bay, South China Sea, filtered by a 0.45 μm polycarbonate membrane, and autoclaved before use.
The euryhaline rotifer, Brachionus plicatilis (L-type strain), were cultured in 30‰ filtered seawater as described before and fed Chlorella sp. (Liu et al., 2022). The fresh seawater for animal culturing was aerated before used.
Short-term and long-term uptake
To ensure the cleanliness of experiment conditions, artificial seawater was prepared in phytoplankton exposure experiments following the prescription by Zhou et al. (2021). During the rotifer feeding experiments seawater was treated through a Chelex-100 resin column (Bio-Rad, CA) to remove the background Cu. Both the artificial seawater and Chelex-treated seawater were autoclaved before use. All the membranes, glass beakers and bottles were soaked in 10% HNO3 and 8% HCl for at least 24 h and rinsed with Milli-Q water (18.2 mΩ) 7 times before used.
Phytoplankton in the logarithmic growth phase was used for these experiments (Harding et al., 1981). During the collection, the phytoplankton was filtered by 0.45 μm polycarbonate membrane gently under 5 mmHg vacuum (Wu et al., 2019). The collected cells were resuspended in the modified f/2 media (f/2 for N, P, Si and vitamin components, f/20 for metal component, eliminating Na2EDTA, Cu and Zn) (Miao and Wang, 2007).
Cu solutions used for exposure were made by 65Cu isotope (99.8%, International Atomic Energy Agency Office, New York) dissolved in the form of Cu(NO3)2. To regulate ion activity of 65Cu, nitrilotriacetic acid (NTA, 0.1 mmol L-1, Sigma) was added during the exposure periods. [Cu2+] in the exposure solutions were calculated by the software VISUAL MINTEQ 3.1 (Jon Petter Gustafsson, Sweden). According to the realistic [Cu2+] in the coastal seawater is usually lower than 10-12 mol L-1 (Li et al., 2011; Kong, 2022), in the short-term uptake experiment, 65Cu solution was added to make a series of concentrations as 5, 25, 50, 75, 100 μg L-1 and [Cu2+] of each treatment were 4.82 × 10-13, 2.42 × 10-12, 4.85 × 10-12, 7.31 × 10-12, 9.78 × 10-12 mol L-1, respectively. While in the long-term uptake experiment, three [Cu2+] were set, including 4.82 × 10-13, 4.85 × 10-12 and 9.78 × 10-12 mol L-1. Each sample had three replications.
Afterwards, a 2 h uptake experiment was conducted. The initial algae density was 1 × 105 cell mL-1. For this measurement, a 5 mL algae sample from each replication was filtered using a GF/C membrane (Whatman, British), then rinsed by Na2EDTA solution (0.1 mM, in the artificial seawater) twice to remove the weakly binding Cu. Samples were further rinsed by ammonium formate solution (36 g L-1) once to remove the salts remained in the filter membrane (Breuer et al., 2013). Finally, samples were stored in 15 mL centrifuge tubes (Corning, USA) and dried for analyses. The cell size was calculated by Coulter Counter (Beckman, USA) and the volume was calculated following Quigg et al. (2006).
A 6 days uptake experiment was also conducted in a batch culture system,. The initial algae density was 1 × 105 cell mL-1. Algae and seawater were sampled once per day at 24, 48, 72, 96, 120, and 144 hour. The cell densities of the algae samples were recorded. The other experimental conditions and sampling treatments were the same as those in the short-term uptake experiment described above. The average algae specific growth rates were calculated by the following equation:
where μ is the average cell specific growth rate (per day); Ct and C0 represent the final and initial cell density, respectively (Miao et al., 2005).
Subcellular fractionation and trophic transfer
Phytoplankton were exposed in 5 μg L-1 65Cu for 6 days to analyze the subcellular fractionation of Cu and to be used in the feeding experiment. The exposure treatment was the same as the method described above. The collected algae were separated into two parts.
A part of collected algae was used to determine Cu subcellular distribution following the modified methods described by Wallace et al. (1998). The alga cells were homogenized by sonication in ice bath. The cells were homogenized in 2 mL buffer containing Tris-HCl (3.15 g L-1), 2-mercaptoethanol (0.39 g L-1) and phenylmethanesulfonyl fluoride (PMSF, 0.017 g L-1) (Zhou et al., 2018). The samples were centrifuged at 1500 g at 4°C for 15 min. The primary supernatant was heated at 80°C for 10 min, ice-cooled for 1 h, centrifuged at 26,000 g at 4°C for 30 min, and then the HDP + Organelle fraction and HSP fraction were obtained as the secondary pellet and supernatant, respectively. The primary pellet was added with 1 mL 40 g L-1 NaOH solution, heated at 100°C for 2 min and centrifuged at 5000 g at 4°C for 10 min, and then the MRG fraction and a supernatant of CD fraction were obtained as the third pellet and supernatant, respectively. Different from Wallance’s method, HDP + Organelle were pooled as a single fraction due to the small proportion of Cu in the organelles in the preliminary experiments. Cu distributed in TAM fraction was also analyzed as the sum of Cu in HDP + Organelle and HSP fractions.
The other part of algae used for rotifer feeding was diluted to 2 × 105 cell mL-1. The protocol of feeding experiment followed the method in Wang et al. (2001), Before feeding, the predator rotifers were starved for 12 h and pulse-chase fed the 65Cu labeled living phytoplankton for 10 min. Then the rotifers were transferred into clean seawater for 5 hours. 1000 individuals of rotifers were sampled through a 70 μm mesh at 0, 5, 10, 20, 30, 40, 60, 90, 120, 180, 240, 300 min. Samples were washed by artificial seawater containing Na2EDTA twice and ultrapure-water once, and stored by frozen. Each sample had four replications, and the calculation of AE followed the equation:
Where A0 is the AE, A is the percentage of Cu retained in rotifers, k is the depuration rate constant.
Cu quantification
All the samples were dried and digested in 2 mL HNO3 (68%, ultrapure, CNW, German) at 80°C for 24 h. Then, the digested samples were diluted with ultrapure water to an appropriate range of Cu concentration for measurement. The 65Cu content was analyzed using Inductively Coupled Plasma Mass Spectrometer (ICP-MS, NexION350X, PerkinElmer, USA). The recovery of Cu was 80%–120% and 45Sc was selected as the internal standard to correct the sensitivity of ICP-MS. The calculation of 65Cu concentration was used following the equation reported by Croteau et al. (2004) and Guo et al. (2016). The certified reference material of mussels (GBW08571) was used for quality control and a quality control sample was analyzed every 20 samples. The dissolved uptake rate constant (ku, L h-1 cell-1) of Cu described the relationship between Cu uptake rate and free Cu2+ activity, which was calculated following the equation:
Where Iw was the Cu uptake rate and Cw was [Cu2+].
Statistical analyses
The statistical data analyzation and graphic presentation were operated based on R, including packages of xlsx, plyr, Rmisc, gridExtra and ggplot2. Briefly, two-way analysis of variance and Hierarchical analysis of variance were applied to analyze the difference in short term Cu uptake rates among treatments and species. One-way analysis of variance was applied to analyze the difference in long term growth among treatments. Pearson correlation coefficient (r) was applied to test the relationship between TAM and Cu assimilation efficiency. Data were presented as the mean ± standard error, *P< 0.05, **P< 0.01, ***P< 0.001.
Results and discussion
Short-term Cu uptake in different phytoplankton
In the short-term Cu exposure, all the phytoplankton absorbed Cu in a linear pattern over time at environmentally relevant [Cu2+] levels, which meant that phytoplankton can take ambient Cu efficiently (Figure 1). The Cu uptake rates in I. zhanjiangensis, Phaeocystis sp., I. galbana, Chaetoceros sp. and P. tricornutum ranged from 60.3–85.7, 20.8–84.8. 56.0–82.0, 49.0–60.6, and 35.1–51.0 amol h-1 cell-1, respectively. Two-way ANOVA analysis showed that Cu uptake in different phytoplankton was influenced by the interaction between the two variables, species and [Cu2+] (P< 0.001). After eliminating the influence of the interaction, the results showed the uptake rates among species were significantly different (P< 0.001).
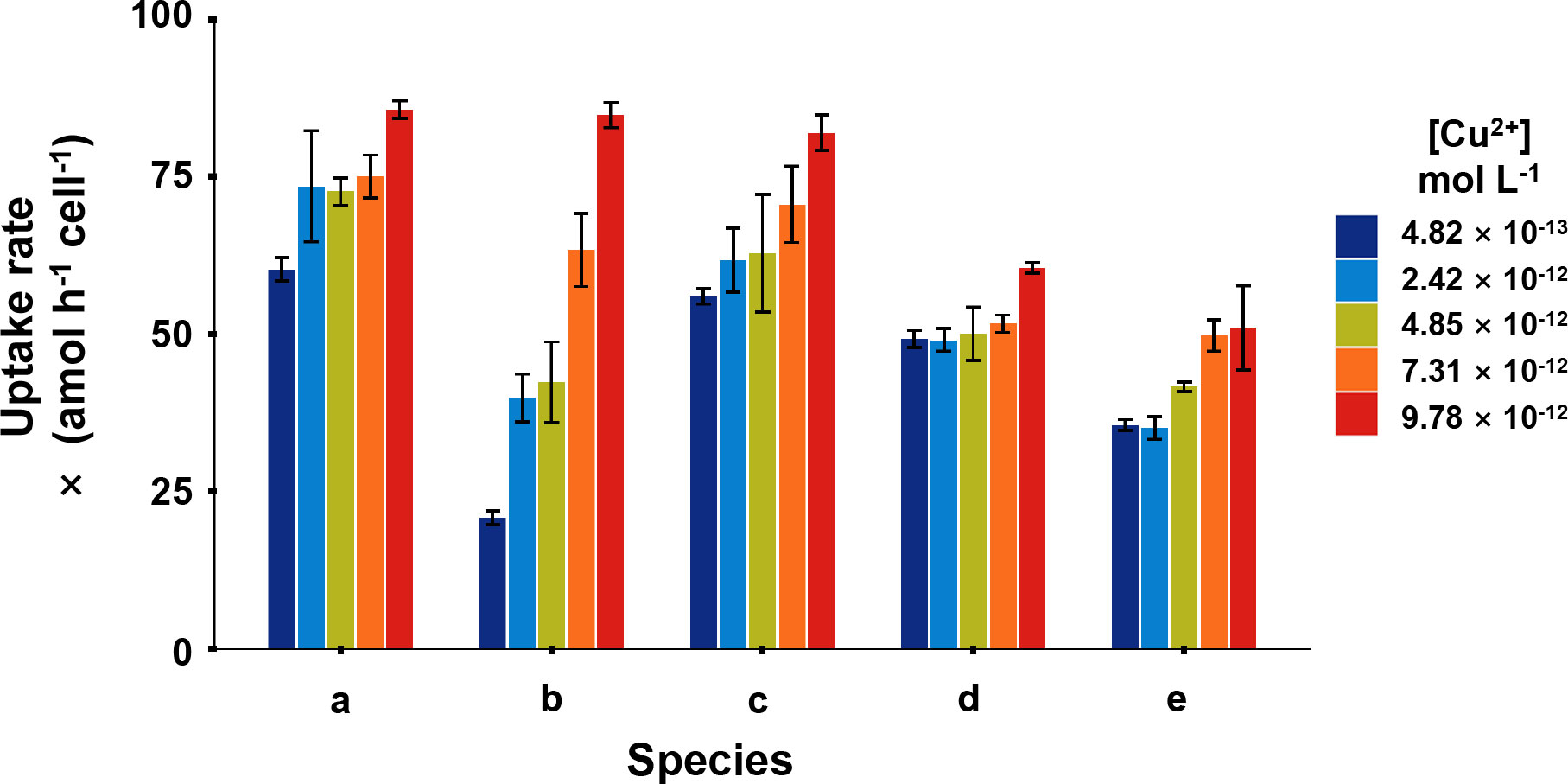
Figure 1 Cu uptake rates of five phytoplankton, in which (A), Isochrysis zhanjiangensi, (B), Phaeocystis sp., (C), Isochrysis galbana, (D), Chaetoceros sp. and (E), Phaeodactylum tricornutum at 4.82 × 10-13, 2.42 × 10-12, 4.85 × 10-12, 7.31 × 10-12, 9.78 × 10-12 mol L-1 [Cu2+] for 2-hour exposure.
At the lowest [Cu2+] (4.82 × 10-13 mol L-1), Phaeocystis sp. had the lowest uptake rate of 20.8 amol h-1 cell-1, while, I. zhanjiangensis had the highest uptake rate of 60.3 amol h-1 cell-1. At the highest [Cu2+] (9.78×10-12 mol L-1), P. tricornutum had the lowest uptake rate 51.0 amol h-1 cell-1, while I. zhanjiangensis still had had the highest uptake rate of 85.7 amol h-1 cell-1. By classifying the species in Bacillariophyta (P. tricornutum, Chaetoceros sp.) and Chrysophyta (I. galbana, I. zhanjiangensis, Phaeocystis sp.), the uptake rate of Chrysophyta was 84.2 amol h-1 cell-1, which was higher than 55.8 amol h-1 cell-1 for Bacillariophyta at [Cu2+] 9.78×10-12 mol L-1. As shown in Table 1, the uptake rate constants (ku) of I. zhanjiangensis, I. galbana, Phaeocystis sp., Chaetoceros sp. and P. tricornutum were 2.20 ± 0.59, 6.46 ± 0.79, 2.60 ± 0.42, 1.10 ± 0.41, 1.96 ± 0.33 (× 10-6 L h-1 cell-1) respectively. Also, the three Chrysophyta species had higher Cu kus than the two Bacillariophyta species, suggesting that Bacillariophyta might have relatively weak ability in Cu uptake.
Usually, cell size is considered as one of the most important factors for metal uptake in phytoplankton. Larger cell has more surface area but less surface/volume, so larger cell may have more metal uptake sites per cell but less metal uptake sites per weight or carbon content of cell. The relationship was much more obvious between Cyanobacteria and Eukaryotic algae (Fawaz et al., 2019), as the Cyanobacteria has the largest ratio of surface/volume or the minimum surface area, and these small particles usually preformed a better capacity for Cu uptake and a more sensitivity to Cu (Brand et al., 1986). Cyanobacteria showed an enhancement of tolerance with the increase of cell size (Debelius et al., 2010). Quigg et al. (2006) had reported the surface area of Thalassiosira weissflflogii and Synechococcus sp. was 460 and 4.7 μm2, and Cu uptake rates of them were 6.1 and 0.33 amol cell-1 min-1, respectively.
However, size effects on Cu uptake and toxicity were not obvious among Eukaryotic algae (Genter and Lehman, 2000). Levy et al. (2007) reported that the Copper partition coefficient of Emiliania huxleyi and Dunaliella tertiolecta was similar at 8 × 10−10 L cell−1 and the surface area was different in 34 and 220 μm2. The 3 Haslea ostrearia strains were defined by cell size, 85 μm, 65 μm and 40 μm, and 85 μm strain showed most sensitive to Cu while 65 μm strain was of most tolerance (Joux-Arab et al., 2000). The Cu EC50 of Tetraselmis chuii, Rhodomonas salina, Chaetoceros sp., Isochrysis galbana, Nannochloropsis gaditana was 330 ± 100, 48 ± 10, 58 ± 30, 137 ± 20 μg L−1 respectively, while the difference cannot be explained by cell size (Debelius et al., 2009).
Table 1 also showed cell size of the five phytoplankton. Except for P. tricornutum, other four species had similar cell size. There was no relationship between cell size and Cu uptake among the five species. Among the four species with similar cell size, the three Chrysophyta species also had significantly higher Cu kus than the Bacillariophyta Chaetoceros sp. Besides the cell size, the different characteristics of algae surface may also affect metal uptake. Metals usually go through the cell wall firstly, bind in the uptake site and then be transported into algae cell (Davis et al., 2003; Nanda et al., 2019). The three Chrysophyta species in this study don’t have any frustules while the Bacillariophyta have silicon frustules, which might be the reason to cause the difference in Cu uptake. It was demonstrated that Cu adsorption was larger in the high-silicon cells than the low-silicon cells of P. tricornutum through AFM images, suggesting that the frustules of Bacillariophyta could affect Cu uptake (Zhou et al., 2020; Ma et al., 2021).
Long-term Cu bioaccumulation in different phytoplankton
Phytoplankton was bioactive through microscopic examination after 6-day exposure. In the long-term Cu exposure, the growth of the five phytoplankton species at the environmental relevant [Cu2+] conditions (4.82 × 10-13–9.78 × 10-12 mol L-1) were shown in Figure 2. It was obvious that the five phytoplankton species had different SGRs, and the SGR of each species kept constant among different [Cu2+], indicating Cu was not deficient nor toxic to the species at this experimental condition. Lopez et al. (2019) investigated different phytoplankton growth rates at [Cu2+] ranging from 10-16–10-11 mol L-1, and results showed the growth rate increased with [Cu2+] and reached platform around 10-11 mol L-1 [Cu2+]. To be mentioned, our in-situ conditions demonstrated no deleterious influence on the population, and the algae had not reflected forceful Cu resistance, in which Cu could act in an essential function.
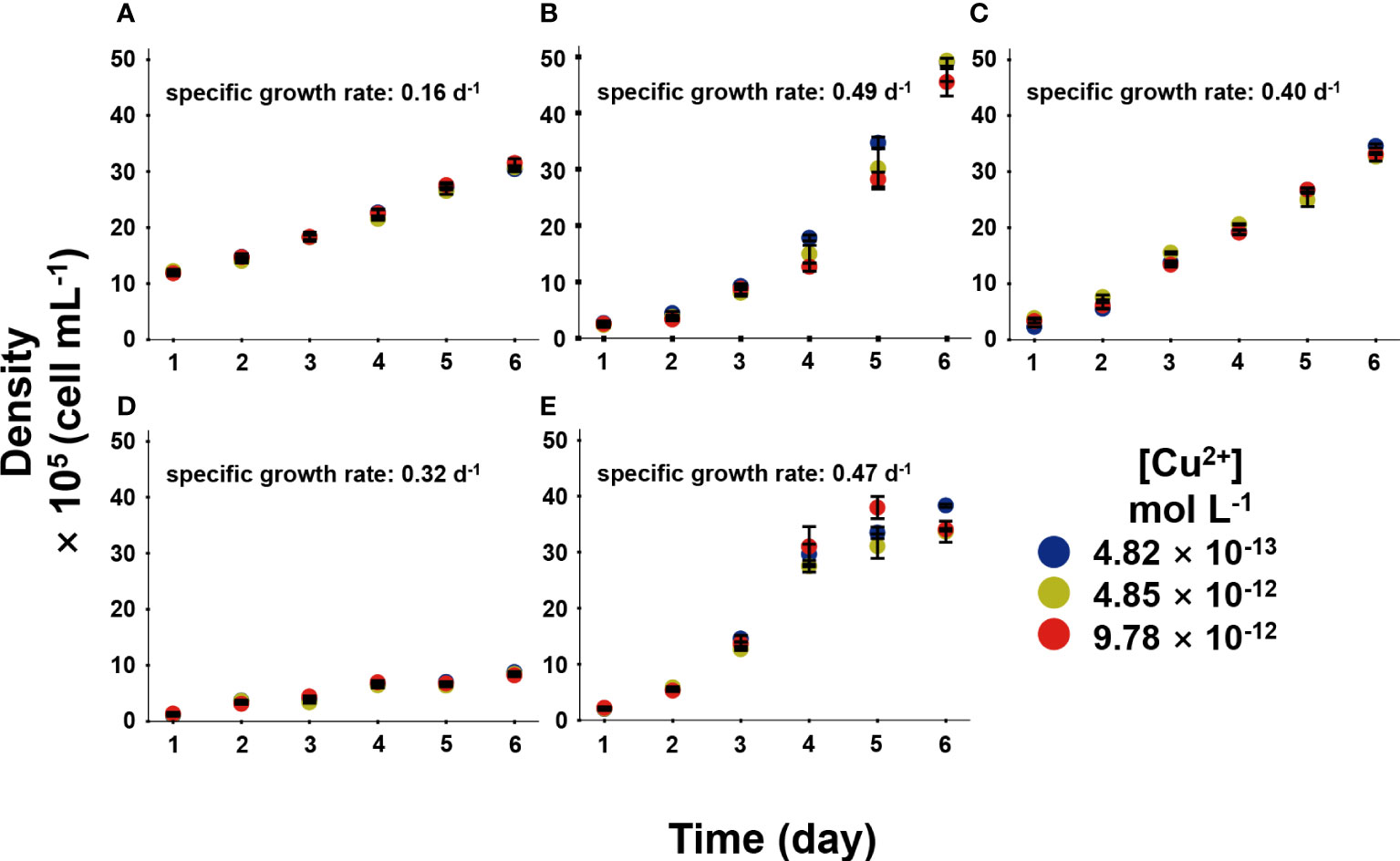
Figure 2 The growth of five phytoplankton, in which (A), Isochrysis zhanjiangensi, (B), Phaeocystis sp., (C), Isochrysis galbana, (D), Chaetoceros sp. and (E), Phaeodactylum tricornutum sp. sp., during 6 day exposure of 4.82 × 10-13, 4.85 × 10-12, and 9.78 × 10-12 mol L-1 [Cu2+].
Figure 3 exhibited the Cu bioaccumulation in the phytoplankton during the 6 days of exposure. Different species accumulated different amount of Cu. Cu accumulation increased continuously within the 6 days in Chrysophyta, while Cu accumulation reached the platform in Bacillariophyta. At the end of exposure, the Cu concentrations in I. zhanjiangensis, Phaeocystis sp., I. galbana, Chaetoceros sp. and P. tricornutum were 99.6 ± 6.0, 446 ± 89, 652 ± 35, 2438 ± 136, 1724 ± 99 amol cell−1 at 9.78 × 10-12 mol L-1 [Cu2+], respectively. Bacillariophyta accumulated significantly more Cu than Chrysophyta. The average Cu concentrations were 275 ± 5, 1132 ± 49, 1281 ± 118 amol cell−1 in Bacillariophyta, and 128 ± 2, 357 ± 7, 399 ± 43 amol cell−1 in Chrysophyta at 4.82 × 10-13, 4.85 × 10-12, 9.78 × 10-12 mol L-1 [Cu2+] respectively at the end of exposure. These results demonstrated that Bacillariophyta might have more Cu capacities than Chrysophyta. And the results of subcellular distribution of Cu might partially explain it.
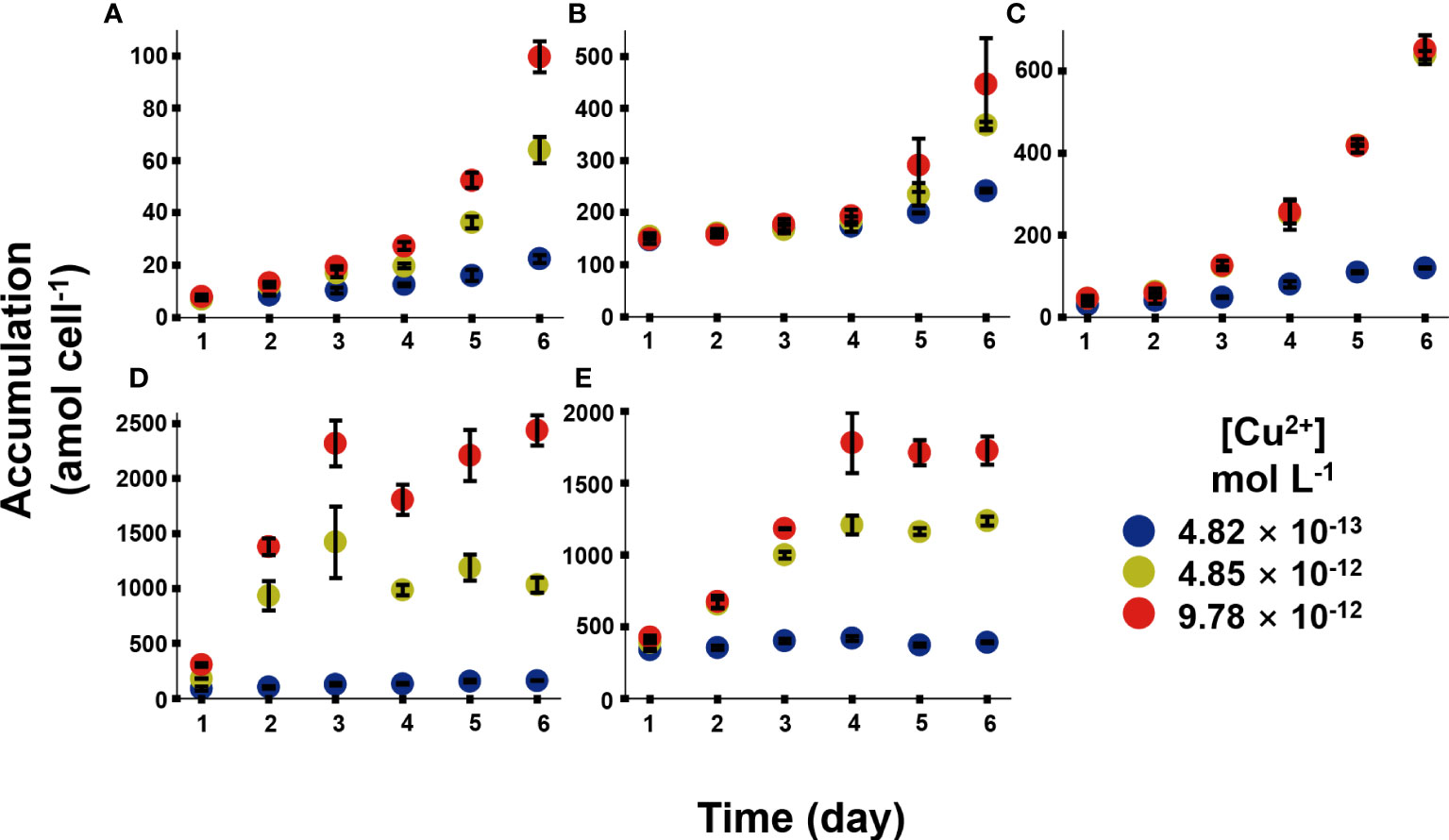
Figure 3 Cu accumulation in five phytoplankton species, in which (A), Isochrysis zhanjiangensi, (B), Phaeocystis sp., (C), Isochrysis galbana, (D), Chaetoceros sp. and (E), Phaeodactylum tricornutum sp. sp., during 6 day exposure of 4.82 × 10-13, 4.85 × 10-12, and 9.78 × 10-12 mol L-1 [Cu2+].
Although it is hardly to compare Cu bioaccumulation in different algae species among different literatures, previous studies demonstrated that Cu accumulation could be affected by various factors, like culturing conditions, exposure duration, Cu concentrations and so on. Cu accumulation in Dunaliella salina was 0.43, 0.79, 0.39, 1.09 fmol cell-1 under different N/P in 12, 16, 25, 110 after 0.55 μmol L-1 Cu exposure for 8 days, which indicated Cu accumulation increased with the N/P (Li et al., 2013). Cu accumulation in Cylindrotheca fusiformis were 3.8 and 0.7 mg ml-1 cell volume under 0.2 mg L-1 Cu exposure for 7 days and 21 days, respectively, and almost 40% Cu could be washed by EDTA (Pistocchi et al., 1997). Tetraselmis suecica could accumulate 29.3 and 110.7 mg kg-1 Cu (dry weight) after 15 and 50 μg L-1 Cu exposure for 7-8 days, respectively (Akcha et al., 2022). In our long-term accumulation, although Cu has not affacted the growth of algae, the Cu accumulations increased with the time in the 6 days culture, which may indicate that different species has different Cu demand. Generally, long-term Cu accumulation is a gross result of uptake, storage, demand, and elimination to balance the request and toxic effects in algae.
Subcellular distribution of Cu in different phytoplankton
The subcellular distribution of Cu was considered to be stable at the endpoint. As it was showed in Figure 4, HDP + Organelle and HSP were the Cu dominated subcellular fractions among all the five phytoplankton. I. zhanjiangensis and Phaeocystis sp. had 66.6 ± 1.1% and 58.4 ± 2.8% of the total Cu in HDP + Organelle, while I. galbana, Chaetoceros sp. and P. tricornutum had 61.2 ± 1.5%, 62.5 ± 3.6%, 40.9 ± 8.5% of the total Cu in HSP. Basically, different Cu subcellular distribution related to the storage and detoxification mechanisms. HDP consisted of enzyme and functional proteins, a metabolism keystone which was influenced by environmental stress and vulnerable to metal cation. Meanwhile, HSP consisted of metallothionein (MT) or MTs-Like proteins, an ordinary antidote which combined metal ions for storage and detoxification.
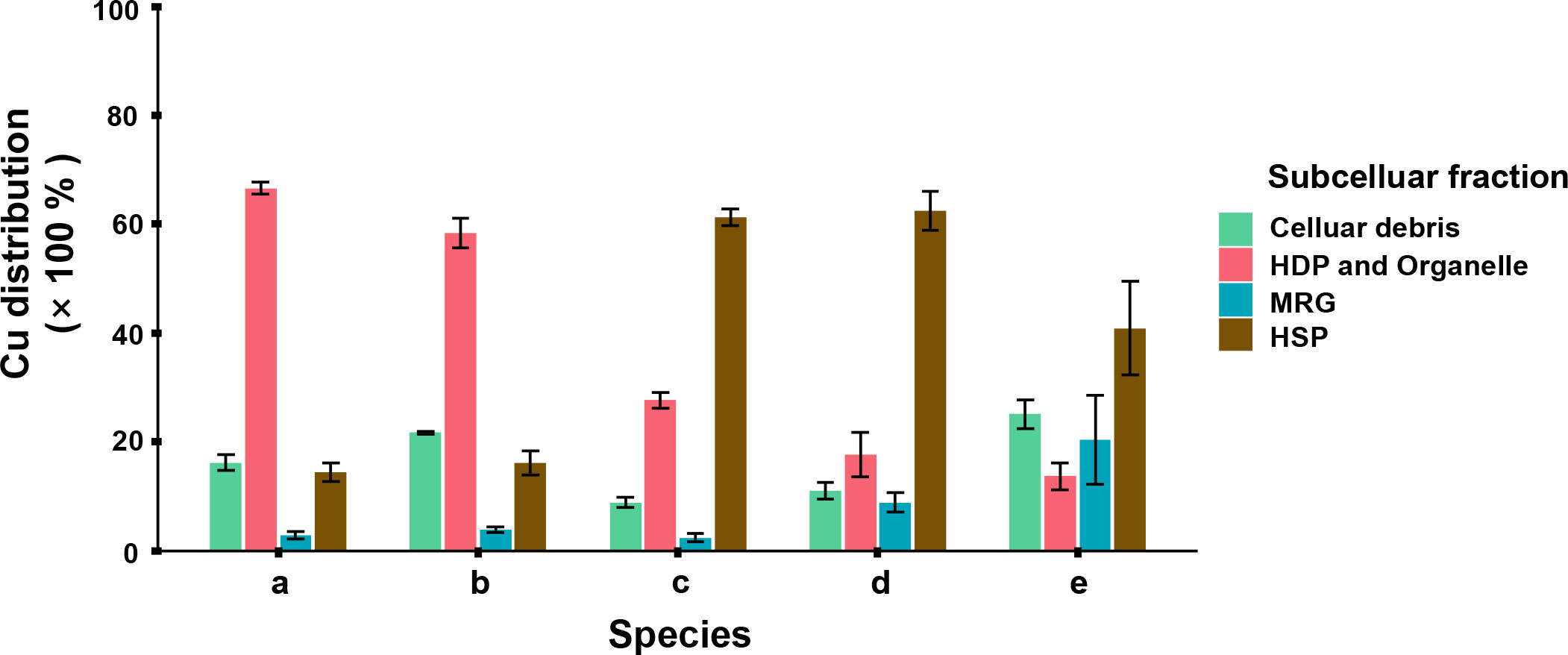
Figure 4 Subcellular distribution of Cu in 5 phytoplankton species, in which (A) Isochrysis zhanjiangensi, (B) Phaeocystis sp., (C) Isochrysis galbana, (D) Chaetoceros sp. and (E) Phaeodactylum tricornutum sp. sp., after 6-day exposure.
The subcellular distributions of Cu were corresponded to the Cu bioaccumulation in different phytoplankton species (Figure 3). In the species accumulating more Cu, such as P. tricornutum and Chaetoceros sp., more Cu was distributed in storage fractions HSP and MRG. In contrast, in the species accumulating less Cu, such as I. zhanjiangensis and Phaeocystis sp., more Cu was distributed in metabolic fractions HDP+Organelle. The previous studies showed that some heat stable proteins were induced by metal and stored metal operatively (Wei et al., 2003). Phytoplankton used to produce protein containing sulfhydryl dealing with metal pressure. HSP contained prosperous mercapto proteins, among which phytochelatins (PCs) was important in phytoplankton. PCs was a kind of sulfhydryl protein containing γ-(Glu-Cys)n-Gly structure, usually induced by metallic cation like Cd, Cu, Hg and so on (Wu et al., 2016). In mainstream, the qualities of sulfhydryl protein reflected the resistance handling divalent ions, however it depended on the protein’s existence form and physiological role. On the other hand, HDP was more vulnerable than HSP. Guo et al. (2013) reported that HSP family’s DbHSP70/90 were significantly upregulated by CuSO4. It made sense that part of phytoplankton used to induced more HSP in defense response against metal stress.
Trophic transfer of Cu from different phytoplankton to rotifer
As it showed in Figure 5, Cu contents in rotifer started to depurate 20 min later after feeding and the remained to stable levers between 90–300 min. The Cu AEs in rotifer feeding I. zhanjiangensis, Phaeocystis sp., I. galbana, Chaetoceros sp. and P. tricornutum were 63.4%, 57.6%, 60.2%, 77.4% and 14.6%, respectively. The Cu AEs for different species were significantly different (P<0.05). It was obvious that Cu AEs were correlated to Cu distribution in TAM of different species, with a spearman correlation coefficient of 0.87, suggesting that TAM had a strong influence on Cu trophic transfer from phytoplankton to the predators (Figure 6). In previous study, TAM had been found to play an important role in metal transfer. Trophic transfer of Cd in bivalves was affected by TAM, in which way AE-Cd in predator increased with TAM-Cd % in prey enhanced, suggesting that more Cd in TAM resulted in higher Cd assimilation (Wallace and Luoma, 2003). Rainbow et al. (2006) reported that TAM component could explain 8%–17% of assimilation change.
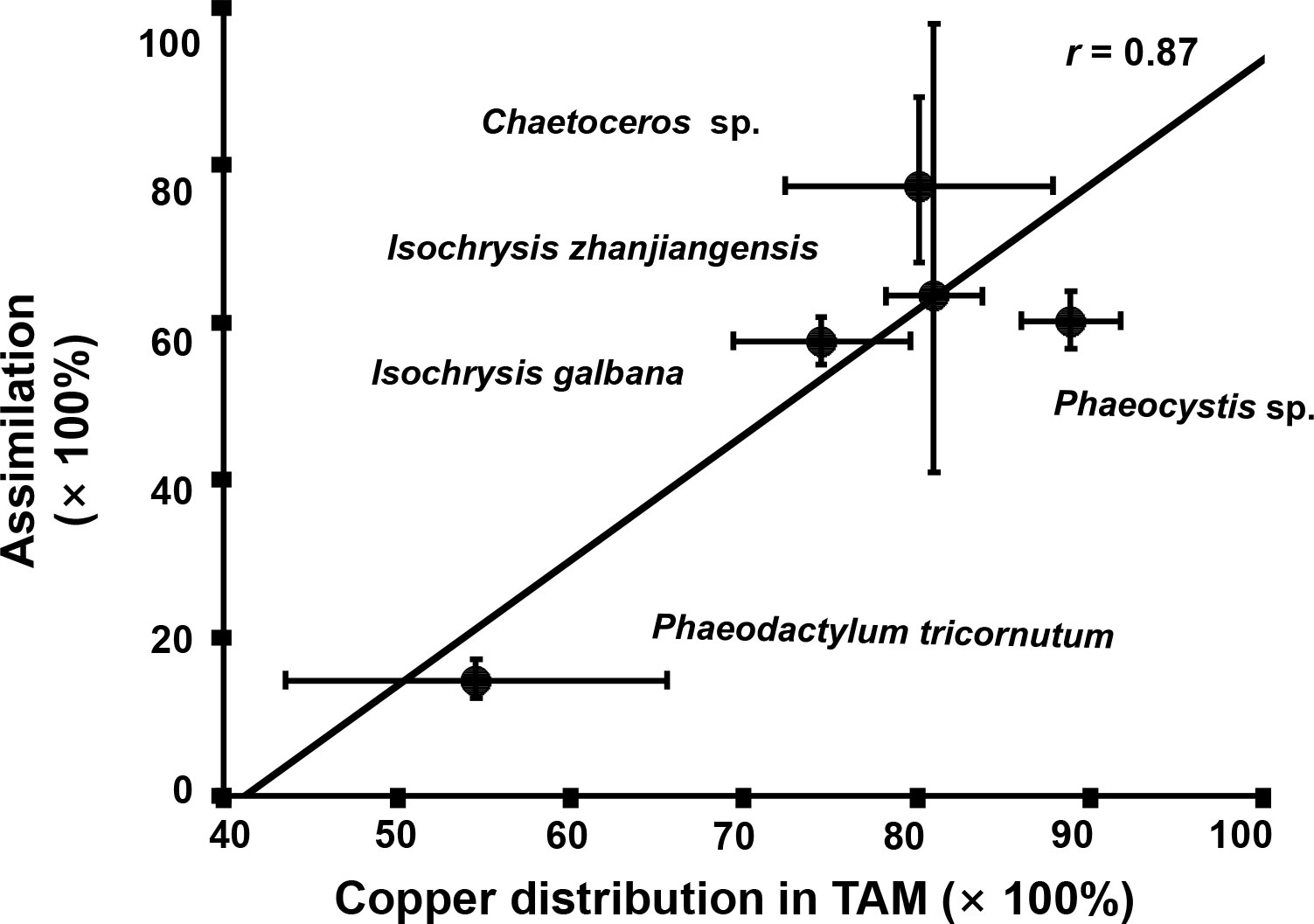
Figure 6 The relationship between Cu assimilation efficiencies (AEs) in rotifer after feeding different phytoplankton species and the Cu distribution in the trophically available metal fraction (TAM) in the respective phytoplankton species.
Normally, aquatic food chains transmit energy and matter with the different efficiencies and make big function in the metal biogeochemical cycling in ecosystems (Tomczyk et al., 2018). The metal transfer through food-chain depended on plenty of factors, such as gut passage time (GPT), species’ life-style, food resource qualities and so on (Wang, 2002). This study firstly demonstrated Cu AEs in marine zooplankton, and found they could be much higher than those in other marine organisms (for example, lower than 20% in fish (Guo et al., 2016)). In natural habitats, zooplankton’s food components were chronologically complex, depending on the seasonal regulative supply. Ye et al. (2018) reported a monthly survey on the species composition of Daya Bay, which showed that diatoms and dinoflagellates exchanged with temperature. It suggests that Cu transfer from phytoplankton to zooplankton is highly diverse in the complicated natural environment.
Conclusion
In general, this study revealed that the different phytoplankton taxa/species had big diversities in Cu uptake, capacity of Cu accumulation, subcellular distribution and trophic transfer potentials. Bacillariophyta might have lower Cu uptake rate but higher capacity of Cu accumulation than Chrysophyta due to their silicon-frustules and the strategy in Cu storage in HSP. Also, the subcellular distribution of Cu in different species greatly influenced the trophic transfer of Cu to zooplankton. Therefore, this study implies that the change of phytoplankton community will change the biogeochemistry of some nutrient trace metals, and influences the bioaccumulation of those metals in many marine organisms.
Data availability statement
The original contributions presented in the study are included in the article/supplementary material. Further inquiries can be directed to the corresponding author.
Author contributions
PZ and LZ contributed to the design/conception of the work, data analysis, reviewing and editing of manuscript. PZ collected and analyzed samples. LZ provided resource support and project administration. PZ and YL contributed to the writing of original draft. All authors contributed to the article and approved the submitted version.
Funding
This research was financially supported by the National Natural Science Foundation of China (NSFC, 41922042, 41876133), Key Special Project for Introduced Talents Team of Southern Marine Science and Engineering Guangdong Laboratory-Guangzhou (GML2019ZD0405), the Natural Science Foundation of Guangdong Province (2021A1515012533), Science and Technology Planning Project of Guangdong Province, China (2020B1212060058).
Acknowledgments
We thank Professor Sheng Liu and Hualian Wu at the Institution of South China Sea Ecology and Environmental Engineering for the present of all the phytoplankton, including Isochrysis zhanjiangensis, Phaeocystis sp., Isochrysis galbana, Chaetoceros sp. and Phaeodactylum tricornutum.
Conflict of interest
The authors declare that the research was conducted in the absence of any commercial or financial relationships that could be construed as a potential conflict of interest.
Publisher’s note
All claims expressed in this article are solely those of the authors and do not necessarily represent those of their affiliated organizations, or those of the publisher, the editors and the reviewers. Any product that may be evaluated in this article, or claim that may be made by its manufacturer, is not guaranteed or endorsed by the publisher.
References
Akcha F., Coquille N., Sussarellu R., Rouxel J., Chouvelon T., Gonzalez P., et al. (2022). Trophic transfer of copper decreases the condition index in Crassostrea gigas spat in concomitance with a change in the microalgal fatty acid profile and enhanced oyster energy demand. Sci. Total. Environ. 824, 153841. doi: 10.1016/j.scitotenv.2022.153841
Andresen E., Peiter E., Kupper H. (2018). Trace metal metabolism in plants. J. Exp. Bot. 69, 909–954. doi: 10.1093/jxb/erx465
Brand L. E., Sunda W. G., Guillard R. R. L. (1986). Reduction of marine-phytoplankton reproduction rates by copper and cadmium. J. Exp. Mar. Biol. Ecol. 96, 225–250. doi: 10.1016/0022-0981(86)90205-4
Breuer G., Evers W. A., De Vree J. H., Kleinegris D. M., Martens D. E., Wijffels R. H., et al. (2013). Analysis of fatty acid content and composition in microalgae. J. Vis. Exp. 80, 50628. doi: 10.3791/50628
Chen H., Shen X., Ying Y., Li X., Chen L., Shen C., et al. (2022). Effect of trace elements in the toxicity of copper to Chlamydomonas reinhardtii. Environ. Sci. Process. Impacts. 24, 576–585. doi: 10.1039/d1em00521a
Croot P. L., Karlson B., Van Elteren J. T., Kroon J. J. (1999). Uptake of 64Cu-oxine by marine phytoplankton. Environ. Sci. Technol. 33, 3615–3621. doi: 10.1021/es9807901
Croot P. L., Karlson B., Van Elteren J. T., Kroon J. J. (2003). Uptake and efflux of 64Cu by the marine cyanobacterium Synechococcus (WH7803). Limnol. Oceanogr. 48, 179–188. doi: 10.4319/lo.2003.48.1.0179
Croteau M. N., Luoma S. N., Topping B. R., Lopez C. B. (2004). Stable metal isotopes reveal copper accumulation and loss dynamics in the freshwater bivalve Corbicula. Environ. Sci. Technol. 38, 5002–5009. doi: 10.1021/es049432q
Davis T. A., Volesky B., Mucci A. (2003). A review of the biochemistry of heavy metal biosorption by brown algae. Water Res. 37, 4311–4330. doi: 10.1016/s0043-1354(03)00293-8
Debelius B., Forja J. M., Delvalls T. A., Lubian L. M. (2009). Toxicity of copper in natural marine picoplankton populations. Ecotoxicology 18, 1095–1103. doi: 10.1007/s10646-009-0377-3
Debelius B., Forja J. M., Delvalls A., Lubian L. M. (2010). Toxic effect of copper on marine picophytoplankton populations isolated from different geographic locations. Sci. Mar. 74, 133–141. doi: 10.3989/scimar.2010.74s1133
Fawaz E. G., Kamareddine L. A., Salam D. A. (2019). Effect of algal surface area and species interactions in toxicity testing bioassays. Ecotox. Environ. Safe. 174, 584–591. doi: 10.1016/j.ecoenv.2019.03.032
Genter R. B., Lehman R. M. (2000). Metal toxicity inferred from algal population density, heterotrophic substrate use, and fatty acid profile in a small stream. Environ. Toxicol. Chem. 19, 869–878. doi: 10.1002/etc.5620190413
Gonzalez-Davila M., Santana-Casiano J. M., Perez-Pena J., Millero F. J. (1995). Binding of Cu(II) to the surface and exudates of the alga Dunaliella tertiolecta in seawater. Environ. Sci. Technol. 29, 289–301. doi: 10.1021/es00002a004
Guo J. A., Annett A. L., Taylor R. L., Lapi S., Ruth T. J., Maldonado M. T. (2010). Copper-uptake kinetics of coastal and oceanic diatoms. J. Phycol. 46, 1218–1228. doi: 10.1111/j.1529-8817.2010.00911.x
Guo J., Lapi S., Ruth T. J., Maldonado M. T. (2012). The effects of iron and copper availability on the copper stoichiometry of marine phytoplankton. J. Phycol. 48, 312–325. doi: 10.1111/j.1529-8817.2012.01133.x
Guo R., Lee M. A., Ki J. S. (2013). Different transcriptional responses of heat shock protein 70/90 in the marine diatom Ditylum brightwellii exposed to metal compounds and endocrine-disrupting chemicals. Chemosphere 92, 535–543. doi: 10.1016/j.chemosphere.2013.03.052
Guo Z., Zhang W., Du S., Zhou Y., Gao N., Zhang L., et al. (2016). Feeding reduces waterborne Cu bioaccumulation in a marine rabbitfish Siganus oramin. Environ. pollut. 208, 580–589. doi: 10.1016/j.envpol.2015.10.032
Harding L. W., Meeson B. W., Prezelin B. B., Sweeney B. M. (1981). Diel periodicity of photosynthesis in marine phytoplankton. Mar. Biol. 61, 95–105. doi: 10.1007/Bf00386649
Hu S., Guo Z., Li T., Carpenter E. J., Liu S., Lin S. (2014). Detecting in situ copepod diet diversity using nolecular technique: development of a copepod/symbiotic ciliate-excluding eukaryote-inclusive PCR protocol. PloS One 9, e103528. doi: 10.1371/journal.pone.0103528
Joux-Arab L., Berthet B., Robert J. M. (2000). Do toxicity and accumulation of copper change during size reduction in the marine pennate diatom Haslea ostrearia? Mar. Biol. 136, 323–330. doi: 10.1007/s002270050690
Knauer K., Behra R., Sigg L. (1997). Adsorption and uptake of copper by the green alga Scenedesmus subspicatus (Chlorophyta). J. Phycol. 33, 596–601. doi: 10.1111/j.0022-3646.1997.00596.x
Kong L. (2022). Copper requirement and acquisition by marine microalgae. Microorganisms 10, 1853. doi: 10.3390/microorganisms10091853
Kong L. L., Price N. M. (2020). A reduction-dependent copper uptake pathway in an oceanic diatom. Limnol. Oceanogr. 65, 601–611. doi: 10.1002/lno.11329
Levy J. L., Stauber J. L., Jolley D. F. (2007). Sensitivity of marine microalgae to copper: The effect of biotic factors on copper adsorption and toxicity. Sci. Total. Environ. 387, 141–154. doi: 10.1016/j.scitotenv.2007.07.016
Li S. X., Liu F. J., Zheng F. Y., Zuo Y. G., Huang X. G. (2013). Effects of nitrate addition and iron speciation on trace element transfer in coastal food webs under phosphate and iron enrichment. Chemosphere 91, 1486–1494. doi: 10.1016/j.chemosphere.2012.12.018
Li L., Pala F., Haskins J., Sukola K., Wallace G. T. (2011). Measurement of free Cu ion activity in seawater using a passive-equilibrium sonic-assisted free ion recorder (SAFIR). Environ. Sci. Technol. 45, 5660–5667. doi: 10.1021/es200367e
Liu Q., Zhou L., Wu Y., Huang H., He X., Gao N., et al. (2022). Quantification of the carbon released by a marine fish using a carbon release model and radiocarbon. Mar. pollut. Bull. 181, 113908. doi: 10.1016/j.marpolbul.2022.113908
Lopez J. S., Lee L., Mackey K. R. M. (2019). The toxicity of copper to Crocosphaera watsonii and other marine phytoplankton: A systematic review. Front. Mar. Sci. 5. doi: 10.3389/fmars.2018.00511
Ma J., Zhou B., Chen F., Pan K. (2021). How marine diatoms cope with metal challenge: Insights from the morphotype-dependent metal tolerance in Phaeodactylum tricornutum. Ecotox. Environ. Safe. 208, 111715. doi: 10.1016/j.ecoenv.2020.111715
Miao A. J., Wang W. X. (2007). Predicting copper toxicity with its intracellular or subcellular concentration and the thiol synthesis in a marine diatom. Environ. Sci. Technol. 41, 1777–1782. doi: 10.1021/es0613963
Miao A. J., Wang W. X., Juneau P. (2005). Comparison of cd, Cu, and zn toxic effects on four marine phytoplankton by pulse-amplitude-modulated fluorometry. Environ. Toxicol. Chem. 24, 2603–2611. doi: 10.1897/05-009r.1
Nanda M., Kumar V., Sharma D. K. (2019). Multimetal tolerance mechanisms in bacteria: The resistance strategies acquired by bacteria that can be exploited to ‘clean-up’ heavy metal contaminants from water. Aquat. Toxicol. 212, 1–10. doi: 10.1016/j.aquatox.2019.04.011
Ng T. Y., Wang W. X. (2005). Dynamics of metal subcellular distribution and its relationship with metal uptake in marine mussels. Environ. Toxicol. Chem. 24, 2365–2372. doi: 10.1897/04-637r.1
Pistocchi R., Guerrini F., Balboni V., Boni L. (1997). Copper toxicity and carbohydrate production in the microalgae Cylindrotheca fusiformis and gymnodinium sp. Eur. J. Phycol. 32, 125–132. doi: 10.1017/s0967026297001170
Quigg A., Reinfelder J. R., Fisher N. S. (2006). Copper uptake kinetics in diverse marine phytoplankton. Limnol. Oceanogr. 51, 893–899. doi: 10.4319/lo.2006.51.2.0893
Rainbow P. S., Poirier L., Smith B. D., Brix K. V., Luoma S. N. (2006). Trophic transfer of trace metals from the polychaete worm nereis diversicolor to the polychaete n-virens and the decapod crustacean palaemonetes varians. Mar. Ecol. Prog. Ser. 321, 167–181. doi: 10.3354/meps321167
Sunda W. G., Guillard R. R. L. (1976). Relationship between cupric ion activity and toxicity of copper to phytoplankton. J. Mar. Res. 34, 511–529. doi: 10.1575/1912/1275
Tomczyk N. J., Parr T. B., Gray E., Iburg J., Capps K. A. (2018). Trophic strategies influence metal bioaccumulation in detritus-based, aquatic food webs. Environ. Sci. Technol. 52, 11886–11894. doi: 10.1021/acs.est.8b04009
Tsvetkov P., Coy S., Petrova B., Dreishpoon M., Verma A., Abdusamad M., et al. (2022). Copper induces cell death by targeting lipoylated TCA cycle proteins. Science 375, 1254–1261. doi: 10.1126/science.abf0529
Wallace W. G., Lopez G. R., Levinton J. S. (1998). Cadmium resistance in an oligochaete and its effect on cadmium trophic transfer to an omnivorous shrimp. Mar. Ecol. Prog. Ser. 172, 225–237. doi: 10.3354/meps172225
Wallace W. G., Luoma S. N. (2003). Subcellular compartmentalization of cd and zn in two bivalves. II. significance of trophically available metal (TAM). Mar. Ecol. Prog. Ser. 257, 125–137. doi: 10.3354/meps257125
Walsh M. J., Ahner B. A. (2014). Copper export contributes to low copper levels and copper tolerance in Emiliania huxleyi. Limnol. Oceanogr. 59, 827–839. doi: 10.4319/lo.2014.59.3.0827
Wang W. X. (2002). Interactions of trace metals and different marine food chains. Mar. Ecol. Prog. Ser. 243, 295–309. doi: 10.3354/meps243295
Wang K., Chen B., Gao Y., Lin H. (2021). Harmful algal blooms caused by phaeocystis globosa from 1997 to 2018 in Chinese coastal waters. Mar. pollut. Bull. 173, 112949. doi: 10.1016/j.marpolbul.2021.112949
Wang W. X., Dei R. C. H., Xu Y. (2001). Responses of zn assimilation by coastal plankton to macronutrients. Limnol. Oceanogr. 46, 1524–1534. doi: 10.4319/lo.2001.46.6.1524
Wang M. J., Wang W. X. (2008). Temperature-dependent sensitivity of a marine diatom to cadmium stress explained by subcelluar distribution and thiol synthesis. Environ. Sci. Technol. 42, 8603–8608. doi: 10.1021/es801470w
Wei L., Donat J. R., Fones G., Ahner B. A. (2003). Interactions between cd, Cu, and zn influence particulate phytochelatin concentrations in marine phytoplankton: Laboratory results and preliminary field data. Environ. Sci. Technol. 37, 3609–3618. doi: 10.1021/es0340026
Wu Y., Guo Z., Zhang W., Tan Q., Zhang L., Ge X., et al. (2016). Quantitative relationship between cadmium uptake and the kinetics of phytochelatin induction by cadmium in a marine diatom. Sci. Rep-UK. 6, 35935. doi: 10.1038/srep35935
Wu Y., Yuan Y., Yuan H., Zhang W., Zhang L. (2019). Predicting cadmium toxicity with the kinetics of phytochelatin induction in a marine diatom. Aquat. Toxicol. 207, 101–109. doi: 10.1016/j.aquatox.2018.12.008
Ye Y. Y., Chen K. B., Zhou Q. Q., Xiang P., Huo Y. L., Lin M. (2018). Impacts of thermal discharge on phytoplankton in daya bay. J. Coast. Res. 83, 135–147. doi: 10.2112/Si83-022.1
Zeng J., Yang L., Wang W. X. (2010). High sensitivity of cyanobacterium Microcystis aeruginosa to copper and the prediction of copper toxicity. Environ. Toxicol. Chem. 29, 2260–2268. doi: 10.1002/etc.266
Zhang L., Wang W. X. (2006). Significance of subcellular metal distribution in prey in influencing the trophic transfer of metals in a marine fish. Limnol. Oceanogr. 51, 2008–2017. doi: 10.4319/lo.2006.51.5.2008
Zhou L. B., Liu F. J., Liu Q. X., Fortin C., Tan Y. H., Huang L. M., et al. (2021). Aluminum increases net carbon fixation by marine diatoms and decreases their decomposition: Evidence for the iron-aluminum hypothesis. Limnol. Oceanogr. 66, 2712–2727. doi: 10.1002/lno.11784
Zhou B., Ma J., Chen F., Zou Y., Wei Y., Zhong H., et al. (2020). Mechanisms underlying silicon-dependent metal tolerance in the marine diatom Phaeodactylum tricornutum. Environ. pollut. 262, 114331. doi: 10.1016/j.envpol.2020.114331
Keywords: copper, phytoplankton, bioaccumulation, assimilation, stable isotope
Citation: Zhang P, Liu Y and Zhang L (2022) Copper uptake and subcellular distribution in five marine phytoplankton species. Front. Mar. Sci. 9:1084266. doi: 10.3389/fmars.2022.1084266
Received: 30 October 2022; Accepted: 11 November 2022;
Published: 24 November 2022.
Edited by:
Huan Zhong, Nanjing University, ChinaReviewed by:
Jie Ma, Shenzhen University, ChinaMinghua Wang, Xiamen University, China
Yuelu Jiang, Tsinghua University, China
Copyright © 2022 Zhang, Liu and Zhang. This is an open-access article distributed under the terms of the Creative Commons Attribution License (CC BY). The use, distribution or reproduction in other forums is permitted, provided the original author(s) and the copyright owner(s) are credited and that the original publication in this journal is cited, in accordance with accepted academic practice. No use, distribution or reproduction is permitted which does not comply with these terms.
*Correspondence: Li Zhang, emhhbmdsaUBzY3Npby5hYy5jbg==