- 1Department of Marine Sciences, University of Gothenburg, Strömstad, Sweden
- 2Division of Food and Nutrition Science, Department of Biology and Biological Engineering, Chalmers University of Technology, Gothenburg, Sweden
- 3Fibre and Polymer Technology, KTH Royal Institute of Technology, Stockholm, Sweden
- 4Swedish Food Agency, Uppsala, Sweden
Seaweed biomass is a renewable resource with multiple applications. Sea-based cultivation of seaweeds can provide high biomass yields, low construction, operation, and maintenance costs and could offer an environmentally and economically sustainable alternative to land-based cultivations. The biochemical profile of sea-grown biomass depends on seasonal variation in environmental factors, and the optimization of harvest time is important for the quality of the produced biomass. To identify optimal harvest times of Swedish sea-based cultivated sea lettuce (Ulva fenestrata), this study monitored biomass yield, morphology, chemical composition, fertility, and biofouling at five different harvesting times in April – June 2020. The highest biomass yields (approximately 1.2 kg fw [m rope]–1) were observed in late spring (May). The number and size of holes in the thalli and the amount of fertile and fouled tissue increased with prolonged growth season, which together led to a significant decline in both biomass yield and quality during summer (June). Early spring (April) conditions were optimal for obtaining high fatty acid, protein, biochar, phenolic, and pigment contents in the biomass, whereas carbohydrate and ash content, as well as essential and non-essential elements, increased later in the growth season. Our study results show that the optimal harvest time of sea-based cultivated U. fenestrata depends on the downstream application of the biomass and must be carefully selected to balance yield, quality, and desired biochemical contents to maximize the output of future sea-based algal cultivations in the European Northern Hemisphere.
Introduction
A central, recurring, and utmost important issue of the 21st century is the urgent need for new, sustainable future resources to supply a constantly growing world population. The necessity of the provision of renewable and novel materials and nutritious food sources – especially vegetarian and vegan protein – was emphasized by the United Nations Sustainable Development Goals (SDGs) (UN General Assembly, 2015). These goals were intensely reflected by the European Commission’s Green Deal to “transform the EU into a fair and prosperous society, with a modern, resource-efficient and competitive economy” (European Commission 2019/909, 2019). As a part of the Blue Economy, the emerging European seaweed aquaculture sector addresses the EU’s strategic priorities (Araújo et al., 2021) and there is a growing interest in seaweed cultivation for food and non-food commodities (Holdt and Kraan, 2011). Currently, the vast portion of food production comes from terrestrial agriculture, however, trends of crop yields predict an insufficient production growth by 2050 to cover food demands (Ray and Foley, 2013). Predictions for future agricultural expansion emphasize that aquaculture, especially mariculture, remain the only viable alternative for extensive growth of global food production (e.g., Duarte et al., 2009). Van Krimpen et al. (2013) showed that seaweed farms can produce more protein per hectare compared to soy: 2.5–7.5 tons/ha vs. 0.6–1.2 tons/ha (Van Krimpen et al., 2013), making it a very interesting protein crop. Another important benefit of seaweed biomass is its multi-purpose application in different economic sectors (Hafting et al., 2015; FAO, 2018). For example, seaweeds contain polyunsaturated fatty acids (PUFAs), as well as high-value carbohydrates and phytochemicals, which make them interesting for the cosmeceutical and pharmacological sectors and as functional foods. Furthermore, seaweed can be used to produce biochar with great potential in soil amelioration as it resists decomposition, effectively sequesters carbon and mitigates anthropogenic CO2 emissions (Lehmann and Joseph, 2009; Biederman and Harpole, 2013). Even bioenergy, generated from seaweeds, offers noticeable advantages and profitability can be achieved by selecting fast growing seaweed species (Gao et al., 2020). Furthermore, the cultivation of extractive aquatic species, like seaweeds, is accompanied by various ecosystem and bioremediation services such as the uptake of dissolved nutrients, coastal defense, and carbon sequestration (e.g., Araújo et al., 2021).
During the past years, sea lettuces, scientifically known as the genus Ulva, have received increasing interest from the aquaculture sector due to their compelling traits as crops (Colombo et al., 2006; Abreu et al., 2014; Santos et al., 2015; Califano et al., 2020; Toth et al., 2020; Steinhagen et al., 2021). A Northern Hemisphere Ulva species that gained increasing attention due to its in-door (Olsson et al., 2020a; Toth et al., 2020) and large-scale outdoor cultivability (Steinhagen et al., 2021) is Ulva fenestrata Postels and Ruprecht. This foliose, marine species is widely distributed in Europe (Hughey et al., 2019; Steinhagen et al., 2019; Fort et al., 2020), shows promising biochemical profiles (Olsson et al., 2020a; Toth et al., 2020; Steinhagen et al., 2021) for diverse economic applications such as the food industry (Harrysson et al., 2018; Toth et al., 2020; Trigo et al., 2021) and its polysaccharides have been successfully used for innovative future materials (Wahlström et al., 2020a,b).
Most cultivation of European Ulva spp. is until date land- or near-shore based and performed in bioreactors, tanks, ponds, or basins (Buchholz et al., 2012; Lubsch and Timmermans, 2018; Sebök et al., 2019; Califano et al., 2020; Roleda et al., 2021). Land-based seaweed cultivation offers several advantages such as full control of cultivation parameters, easy and permanent access of the biomass, including harvest, and constant production. Nevertheless, the commercial scale production of land-based cultivated seaweed biomass is challenged by its high costs of construction, operation, and maintenance (Lapointe et al., 1976; Lüning and Pang, 2003; Sebök et al., 2019). The demand for high power and resource inputs further challenges the sustainability of the on-shore produced biomass. To ensure that Ulva biomass can compete with terrestrial crop species there is a need for cost-efficient cultivation and large-scale biomass production beyond pilot-scale (Steinhagen et al., 2021). European temperate coastal- and off-shore regions are excellent farming grounds for native kelp seaweed species, as well as for U. fenestrata (e.g., Stévant et al., 2017; Visch et al., 2020; Steinhagen et al., 2021). Furthermore, sea-based cultivation of Ulva biomass requires only a minimum of land-based operational units during seedling hatcheries or post-harvest processes and could enable low production costs (Steinhagen et al., 2021).
Apart from low production costs, the yield and quality of the harvested biomass is important in order to optimize the economic output from seaweed cultivation. Crucial factors for the sea-based cultivation of seaweeds at high latitudes are biofouling pressure, seawater temperature, and irradiance, which are all strongly season-dependent (Førde et al., 2015; Rolin et al., 2017; Visch et al., 2020). Biofouling in seaweed aquaculture is accompanied by decreased productivity due to competition for surrounding nutrients (Hurd, 2000), reduced light availability (Cancino et al., 1987), increased incidence of breaking fronds (Dixon et al., 1981), and leads to a loss of commercial value of the farmed biomass (Park and Hwang, 2012). Although efforts have been made to predict best practices in avoiding high biofouling pressures on kelp species, such knowledge is lacking for sea-based farming of Ulva spp. (Bannister et al., 2019).
The biochemical profile and quality of farmed European Ulva spp. are also modulated by seasonal changes in environmental factors (e.g., light, salinity, and temperature) (Moreira et al., 2020; Olsson et al., 2020a,b; Toth et al., 2020). Especially light and temperature were identified to have strong effects on the growth as well as on protein, fatty acid, and carbohydrate composition of Northern Hemisphere U. fenestrata (Olsson et al., 2020a; Toth et al., 2020; Steinhagen et al., 2021). This implies that harvest time in sea-based cultivation systems is a crucial factor for the large-scale aquaculture potential of Ulva and the production of biomass with desired biochemical traits. Another central part for the success in Ulva cultivation is the avoidance of large, unpredictable fertility events and the consequent disintegration of the thalli which causes a significant biomass loss. Whereas strains from South Africa have not been observed to undergo such events, the unpredictable fertility intervals of northern European Ulva strains have previously prohibited their large-scale cultivability (Bolton et al., 2009). Whereas periodic biomass losses caused by reproduction have greatly limited the expansion of Ulva, obtaining sterile strains could increase the capacity to exploit Ulva for commercial applications (Gao et al., 2017a). However, the prediction of fertility and thus loss of biomass of non-sterile strains can be fostered by a full-life-cycle cultivation which is mostly afforded when applying seeding techniques in classical rope-cultivation systems (Carl et al., 2014).
The aim of the present study was to assess the yield and quality of large-scale, sea-based cultivated U. fenestrata biomass along a harvest period from early spring to early summer in the Northern Hemisphere. Growth, fertility, and biomass yield were quantified, and the quality of the cultivated biomass was assessed by measuring fouling coverage and through analyses of biochemical composition (protein, fatty acid, carbohydrate, phenolic, pigment, biochar, and ash content, as well as analysis of elements including inorganic arsenic).
Materials and Methods
Production and Deployment of Seedlings
Gametes were obtained from a clonal gametophytic U. fenestrata strain kept for long-term indoor tank cultivation at the Tjärnö Marine Laboratory, University of Gothenburg, Sweden (TML, 58°52′36.4′′N 11°6′42.84′′E). The clonal strain was established by parthenogenetic propagation of a single individual gametophyte and has been maintained by parthenogenetic propagation since. Fertility was induced by mincing and washing maturated parental material and followed the detailed description of fertility induction in U. fenestrata explained by Steinhagen et al. (2021). Molecular identification of the parental biomass can be found in Toth et al. (2020). Five days after fertility induction, a change of cultivation medium in the morning triggered the gametangia to immediately release motile gametes. Gametes were immobilized by keeping the solution in complete darkness at 15°C for 24 h and the density was calculated with a hemocytometer. A solution of 10,000 gametes mL–1 seawater was equally applied onto one twine (ø = 2–3 mm) which was coiled around a sterile PVC spool. A total of three twines were obtained to produce seed lines.
The spools were incubated for 6 weeks in 2 L aquaria filled with sterile filtered (0.2 μm + UV, 9 L h–1) nutrient-enriched seawater, following the recipe of Provasoli (1968). The seedling hatchery was performed in a light- and temperature-controlled cabinet at 15°C with an average irradiance of 80–100 μmol m–2 s–1 under a 12:12 h L:D light regime (LuxaLight LED). To prevent diatom growth 1 mg L–1 GeO2 was added. The culture medium was exchanged weekly. After 6 weeks the juvenile thalli reached a size of 0.5–1 cm and were acclimatized to the prevailing outside conditions. During acclimatization the seedlings grew in filtrated (0.2 μm) seawater and the temperature was decreased to 8°C with steps of 1°C per day over 1 week.
Cultivation Site
The 2 ha seafarm (200 m × 200 m) used for Ulva cultivation was a classical rope construction (see Figures 1A,B) which was situated in the Koster archipelago on the Swedish west coast within the Skagerrak region of the North Sea (58°85.93′ N, - 11°06.82′ E). The Koster archipelago is characterized by rocky shores, and it is one of the most species-rich marine areas in Sweden. The area is almost a tidal, but atmospheric pressure and wind-driven differences of high and low water levels of ±2 m appear along the coastline (Johannesson, 1989). The depth underneath the seafarm is ca. 10 m and the bottom substrate consists primarily of mud and sand.
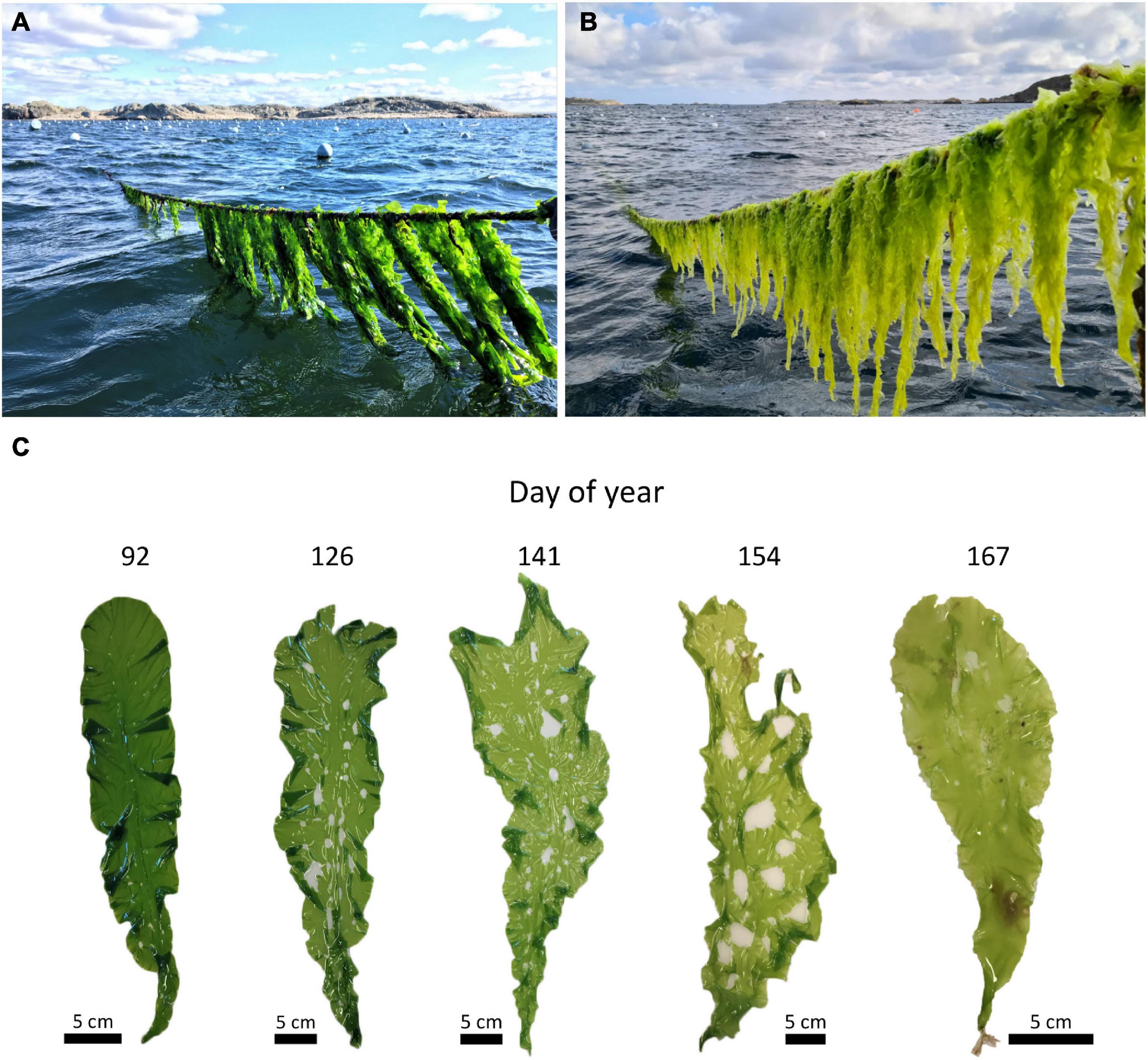
Figure 1. Overview about the off-shore cultivation of U. fenestrata located in the Swedish Koster archipelago, Skagerrak. (A) Picture of the farmed biomass at the first harvest time point of the experiment in May (DOY 92) and (B) before the final harvest in June (DOY 167). (C) Overview about the habitus of cultivated individuals at the five different harvesting points of the experiment.
The seeded cultivation twine was deployed in the seafarm 20th of October 2019 and the twine with attached seedlings was coiled around fabric long lines (each 200 m), which were attached to buoys which were anchored to the seafloor (see Figures 1A,B). The seedlings grew until their respective harvest time without further need of maintenance action. The growth substrate was suspended at 1.5 m depth by attaching buoys to the substrate every 10 m, with 4 m distances between each parallel running long line. For additional information on the farm site and surrounding environmental conditions see Visch et al. (2020). Furthermore, a schematic drawing of the farm site can be found in Steinhagen et al. (2021). To determine the effect of harvest time on U. fenestrata biomass yield and thallus habitus (frond length and width, number and size of thalli holes), biofouling (total cover and community structure), fertility (total amount of fertile frond tissue), and biochemical content (protein, fatty acids, carbohydrates, phenolic, pigments, biochar, and ash) were investigated at five defined harvest time points (01.04.2020 = DOY [day of year] 92; 05.05.2020 = DOY 126; 20.05.2020 = DOY 141; 02.06.2020 = DOY 154; 15.06.2020 = DOY 167). Furthermore, light intensity (Lux) and temperature (°C) were recorded during the whole cultivation period every 30 min using HOBO®§ pendant loggers installed at the seaweed cultivation depth. Loggers were cleaned on every sampling date to minimize the impact of potential foulers.
Biomass Yield, Thallus Habitus, and Biofouling
At each of the five harvesting time points, biomass yield measurements were carried out. Therefore, measurements at 1 m intervals were carried out at all three 200 m ropes including three replicates per rope (thus, per harvest time point three randomly selected spots – each 1 m in length – were harvested at each of the three 200 m ropes). The yield measured as fresh weight [fw] and dry weight [dw] per m rope was determined on a lab-balance (Sartorius TE1502S, Göttingen, Germany) after removing excess water by spinning and lyophilization, respectively. The lyophilized biomass was stored at –80°C until further analysis of biochemical composition. To assess mean thallus length, width, and area ten randomly selected individuals per replicate 1 m rope were photographed directly after each harvest time point (a total of 30 individuals per harvest time) and pictures were analyzed in ImageJ (Schneider et al., 2012). The same pictures were used to quantify the number and size of holes, percentage of biofouling and fertile tissue, which generally appears on the frond margins of Ulva and is detectable by a color change from a green to a brownish color (Wichard, 2015).
Biochemical Composition
Crude Protein Content
Total nitrogen content of U. fenestrata was determined by the combustion method (Dumas) using a LECO Trumac nitrogen analyzer (LECO, St. Joseph, MI, United States). Subsequently, the protein content was calculated using a nitrogen to protein conversion factor of 5 (Angell et al., 2016).
Fatty Acid Content and Composition
Fatty acids were analysed by GC-MS (Agilent 7890 A GC coupled to Agilent 5975 C mass detector, Agilent Technologies, Santa Clara, CA, United States) on a VF-WAX column (30 cm × 0.250 mm × 0.25 μm) according to the method described in detail by Harrysson et al. (2018). The fatty acids in ∼25 mg lyophilized and homogenized U. fenestrata were extracted and directly trans-esterified with toluene containing C17:0 as internal standard and 10% acetylchloride in methanol. Liquid partitioning with ether was used to extract the derivatized fatty acids and the ether extract was injected after evaporation and re-dissolution in isooctane on the GC-MS system. Fatty acids were quantified against the internal standard. Identification of fatty acids was done by using GLC Reference Standard 463 (Nu-Chek Prep, Inc., Elysian, MN, United States), except for C16:1, n9 C16:2 n9, C16:4 n3, C18:4 n3, and C20:4 n3, which were identified using the MS-library. Results are expressed as percent total fatty acids per seaweed dry weight, and as percent of each fatty acid out of the total amount of fatty acids.
Carbohydrate Content and Composition Including Starch
The total carbohydrate content including the monosaccharide composition was calculated as previously described by Bikker et al. (2016). A two-step sulfuric acid hydrolysis was performed on triplicate samples with 6 mg of lyophilized biomass. The monosaccharide and uronic acid compositions of the hydrolyzates were identified using high-performance anion exchange chromatography (HPAEC, Dionex, Sunnyvale, CA, United States) equipped with a pulsed amperometric detector (PAD, Dionex ICS-3000) and a CarboPac PA1 column (4 × 250 mm) using a 260 nM NaOH and 170 mM acetate gradient and Milli Q water as the mobile phase.
The starch content was measured by the GOPOD-method using the Total Starch Assay Kit (Megazyme, Ireland). 20 μl of 80% EtOH was added to 1 mg lyophilized and homogenized U. fenestrata followed by the addition of 300 μl of thermostable α-amylase (3,000 U/mL, diluted 1:30 in 100 mM acetate buffer, pH = 5). Samples were mixed by vortex for 10 s, incubated for 12 min in a boiling water bath, and cooled to room temperature after which 10 μL of amyloglucosidase (3300 U/mL) was added. Samples were then mixed for another 10 s by vortex and incubated at 50°C for 30 min under stirring. The samples were cooled to room temperature, diluted with 670 μl of milliQ water to obtain a final volume of 1.0 mL and then centrifuged at 5000 rpm for 5 min. To 40 μL of the supernatant 960 μL GOPOD-reagent was added. The samples were incubated at 40°C for 20 min. The absorbance of the resulting solution was measured at 510 nm and the starch content was estimated from a calibration curve prepared with glucose standards (0.005–1.0 mg/mL). All measurements were done in duplicates.
Total Phenolic Content
Total phenolic content in U. fenestrata was extracted from lyophilized and homogenized tissue material, following the procedure described by Steinhagen et al. (2021) which is based on the colorimetrical estimation using the Folin-Ciocalteu phenol reagent (Merck, Darmstadt, Germany) while applying gallic acid (Sigma-Aldrich, St. Louis, MO, United States) as a standard. The total phenolic content was subsequently assessed spectrophotometrically at 765 nm (Lambda XLS+, Perkin Elmer, Waltham, MA, United States) and calculated as% of dw.
Pigment (Chlorophyll a,b, Carotenoids) Content
Total contents of chlorophyll a,b and carotenoids of U. fenestrata were assessed using 90% aqueous acetone extraction followed by spectrophotometric assessment using the formula and wavelength given in Jeffrey and Humphrey (1975) for chlorophyll and Parsons et al. (1984) for total carotenoids.
Biochar and Ash Content
To assess the biochar and ash contents of U. fenestrata, TGA measurements were performed using a Mettler Toledo TGA/DSC (Columbus, OH, United States). Approximately 4 mg of each lyophilized sample was heated in ceramic cups from 25°C to 800°C at a heating rate of 10°C min–1 under an N2 atmosphere. After being heated to 800°C, O2 was introduced instead of N2 and the temperature was kept at 800°C for 15 min. The gas flow rate was set to 50 mL min–1. The biochar content was calculated as the weight that remained after the samples were heated to 800°C in N2, and the ash content was calculated as the mass remaining after finalizing the heating program. The data were processed and analyzed using STARe software.
Element Content and Inorganic Arsenic
Total elements were analyzed at the Swedish Food Agency with inductively coupled plasma mass spectrometry (ICP-MS, Agilent 7700x) according to the standard procedures of NMKL no. 186 and EN 15763. This includes total microwave acid digestion of the samples using a mixture of concentrated nitric acid and hydrochloric acid (6 + 1 mL). The method is accredited for foodstuffs by Swedish Board for accreditation and conformity assessment (SWEDAC) in accordance with ISO/IEC 17025. The correctness of the method is controlled by participation in international proficiency tests (PT) for food. In addition, certified reference materials (CRM) and in-house reference materials (IRM) are routinely analysed within each batch to follow both short- and long-term quality of the results. For comparison, also levels in traditional foodstuffs for samples in a Swedish market basket survey are presented (Swedish Food Agency, 2017). The food categories Fish products, Vegetables, and Cereal products were selected to refer to marine food and/or food categories that seaweed products could be related to. The elements are grouped in non-essential elements (more or less toxic) and essential elements. The market basket samples are composite samples of several foodstuffs within each category and correspond to the approximate adult average consumption in Sweden 2015 (e.g., the market basket sample of Cereals contain bread, pasta, breakfast cereals, etc.). The determination of iAs was performed according to the European standard EN16802 at the Swedish Food Agency. The method is based on extraction with dilute nitric acid and hydrogen peroxide in a hot water bath followed by analysis with strong anion exchange HPLC-ICP-MS (high-performance liquid chromatography – inductively coupled plasma mass spectrometry, Agilent 1260 Infinity Quaternary LC and Agilent 7700x ICP-MS). The analytical method is accredited in accordance with ISO/IEC 17025 by the SWEDAC for iAs for foodstuffs and seaweed within the range 1–25,000 μg/kg. The correctness of the method is continuously controlled by participation in international PT, and by routinely analyzing CRM and IRM within each batch, both for long-term quality control and when available for relevant sample matrices. The performance of the method is described in detail in Kollander et al. (2019).
Statistics and Data Analyses
Data on growth, biofouling, fertility, and biochemical composition of ocean-based cultivated U. fenestrata from the experiment in the present study were statistically analyzed using analysis of variance (ANOVA). The effect of harvest period was analyzed for each of the variables using an orthogonal one-way ANOVA with a Harvest time as a five-level, fixed factor. The data were tested for normality using the Shapiro–Wilk test (Shapiro and Wilk, 1965) and was additionally tested for homogeneity of variances using Cochran’s test (Underwood, 1981). When non-normality or heterogeneous variances were found, a robust ANOVA based on 20% trimmed means was performed in the WRS2 package, implemented in R (Mair and Wilcox, 2018). Significant differences among means were compared using Tukey’s HSD test.
Results
Biomass Yield, Thallus Habitus, and Biofouling
A strong difference of the thallus habitus and optical appearance of the sea-based cultivated biomass of U. fenestrata was observed during the harvest period (Figures 1A–C). The thalli had an intense green color at the start of the harvest period (DOY 92) (Figures 1A,C) and gradually became paler with cultivation time until a yellowish appearance was reached at the last harvesting date (DOY 167) (Figures 1B,C). Based on this first morphological variation of the biomass, the total yield, general thallus habitus, and the amount of biofoulers was determined:
The biomass yields significantly increased during the early growth season and reached its maximum (fw = 1216.94 g [m rope]–1 [not shown in figure], dw = 96.90g [m rope]–1) at the third harvest time at DOY = 141. Afterward, the biomass yield continuously declined at the following harvest time points (Figure 2A and Tables 1A,B). Similar patterns have been observed for the thallus area which significantly increased from 682.07 cm2 at the first harvest point to 1216.63 cm2 at the third harvest time point at DOY = 141 (Figures 1C, 2B and Table 1C). After the third harvest time point, the thallus area decreased significantly and reached a minimum (370.71 cm2) at the fifth harvest time point in June (Figures 1C, 2B and Table 1C).
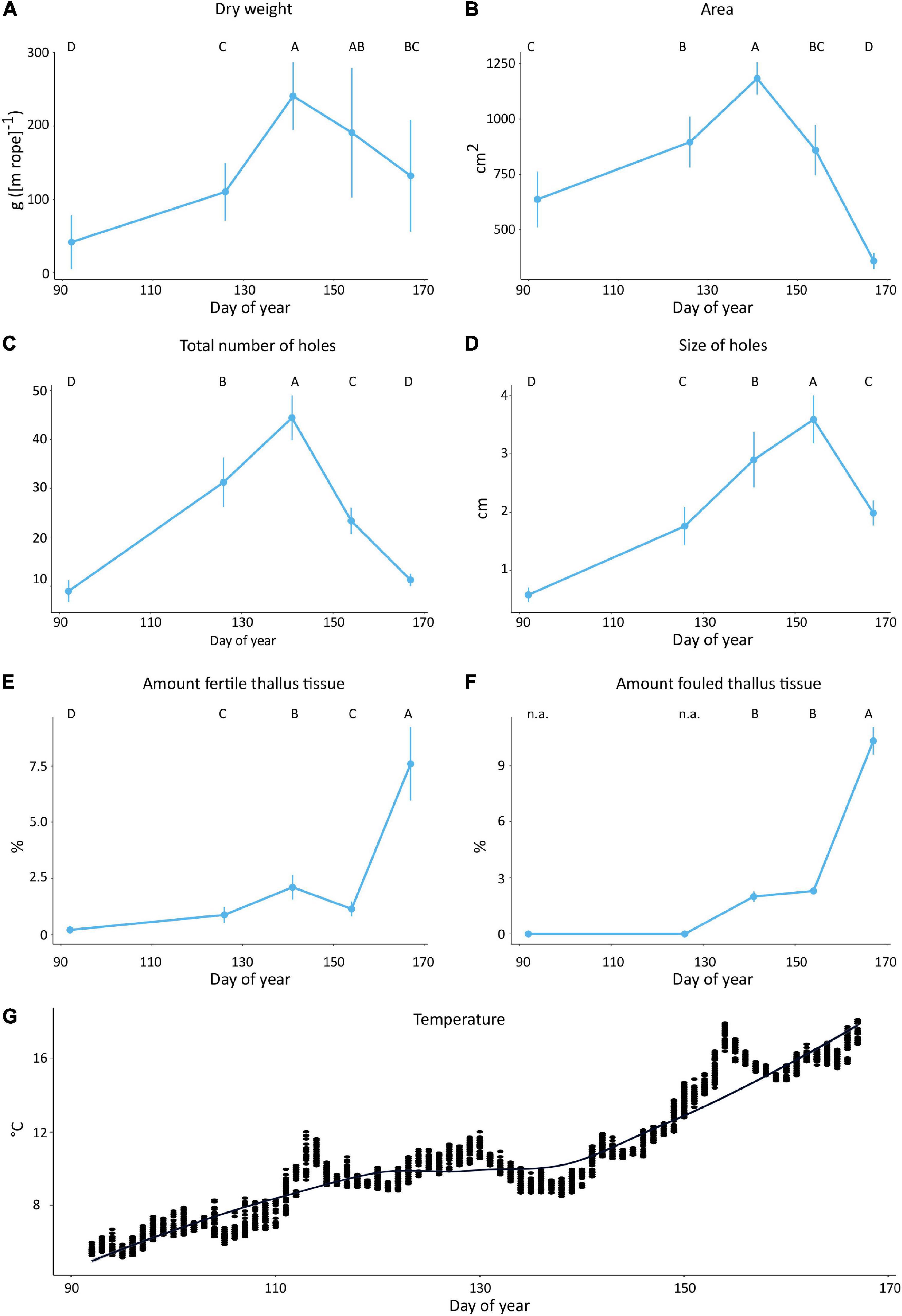
Figure 2. Mean (A) total dry weight (g [m rope]–1), (B) mean thallus area (cm2), (C) total number of holes, (D) size of holes (cm), (E) amount of fertile thallus tissue (%), (F) and amount of fouled thallus tissue of off-shore cultivated U. fenestrata recorded at five different harvest dates (DOY 92, 126, 141, 154, 167). Error bars show 95% confidence interval and capital letters above bars show significant differences between means based on Tukey’s HSD test (p < 0.05). Additionally, the recorded temperature throughout the cultivation time is displayed (G).
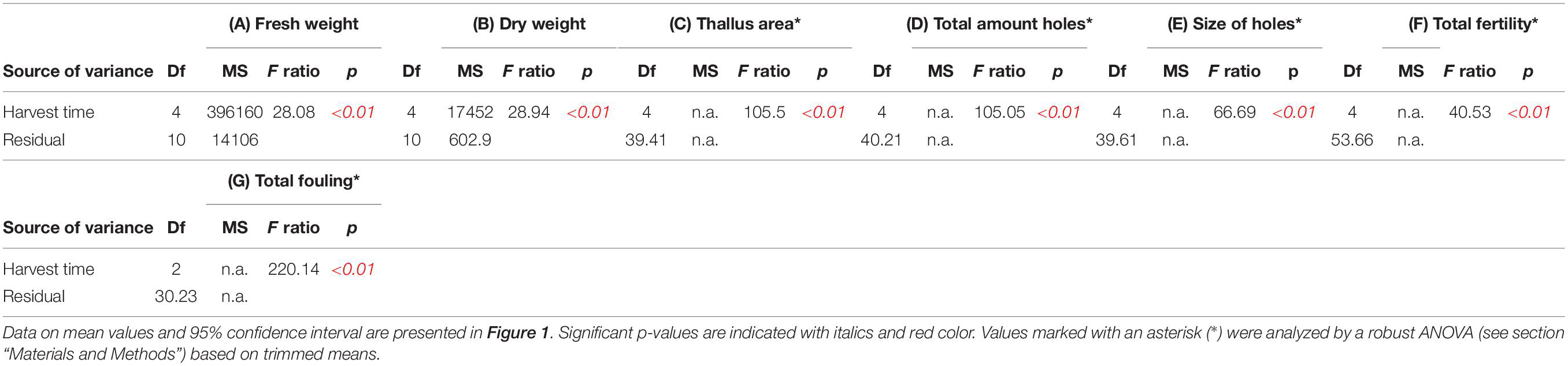
Table 1. ANOVAs of (A) fresh weight and (B) dry weight (g [m rope]–1), (C) thallus area (cm2), (D) total amount of holes, (E) size of holes (cm), (F) total amount of fertile thallus tissue (%), and (G) total amount of fouled thallus tissue (%) of sea-based cultivated Ulva fenestrata biomass harvested at different dates (see also Figure 1).
The total number of holes – measured across the whole thallus frond – significantly increased from 8.96 at the first harvest date (DOY 92) to a maximum of 44.03 at the third harvest date (DOY 141) and then continuously decreased to 11.26 at the end of the experiment (DOY 167) (Figures 1C, 2C and Table 1D). Similarly, the size of holes observed across the thallus frond significantly increased from its minimum measured at the first harvest time point (DOY 92) with 0.58 cm in diameter to its maximum of 3.58 cm in diameter at the fourth harvest time point (DOY 154). Notably, the size of holes significantly decreased from its maximum observed at the fourth harvest date (DOY 154) to 1.98 cm at the fifth harvest date (DOY 167) (Figures 1C, 2D and Table 1E). The amount of fertile thallus tissue significantly varied between the minimum of 0.2% at DOY 92 and the maximum of 7.6% at DOY 167 (Figure 2E and Table 1F).
For biofouling analyses mobile organisms such as amphipods (mainly Caprellidae), isopods, gastropods, and fish species (mainly juvenile Cyclopterus lumpus) were registered but excluded from further analyses. In decreasing order of abundance, we recorded the following epibionts: bryozoan species Electra pilosa and Membranipora membranacea, Aplysia spp. (eggs), filamentous algae (Ceramium sp., Polysiphonia sp.), and hydrozoa (Obelia sp.). Percentage biofouling covering the thallus fronds increased with time (Figures 1C, 2F). There was no biofouling observed until DOY 126 and thus the first two sampling dates were excluded from statistical analyses. There was a 2 and 2.3% coverage of biofoulers at DOY 141 and 154 respectively, followed by a significant increase of fouled thallus tissue of up to 10.33% at DOY 167 (Figures 1C, 2F and Table 1G). Whereas the biofouling community was initially (DOY 141) dominated by filamentous algae – which were predominantly found at the tips and rhizoidal zone of the thalli, those were less abundant at the following harvest dates and were later (DOY 154,167) replaced by the bryozoan epibionts Membranipora membranacea and Electra pilosa which were found across the whole blade (e.g., Figure 1C).
Notably, most of the morphological variables measured at the Northern Hemisphere strain of U. fenestrata (Figures 2A–F) have shown a decrease or a respective increase after the third harvest time point. These changes seem to correlate with the rapid increase in water temperature (Figure 2G) pointing out an important seasonal transition period from Scandinavian spring to summer conditions.
Biochemical Composition
Crude Protein Content
The mean crude protein content of the U. fenestrata biomass was the highest (20.79%) at the first harvest date (DOY 92) (Figure 3A and Table 2E), significantly decreased at the following harvest points (DOY 126, 141; 7.12–7.14% dw), and reached its minimum with 4.67% in June (DOY 167) (Figure 3A and Table 2E).
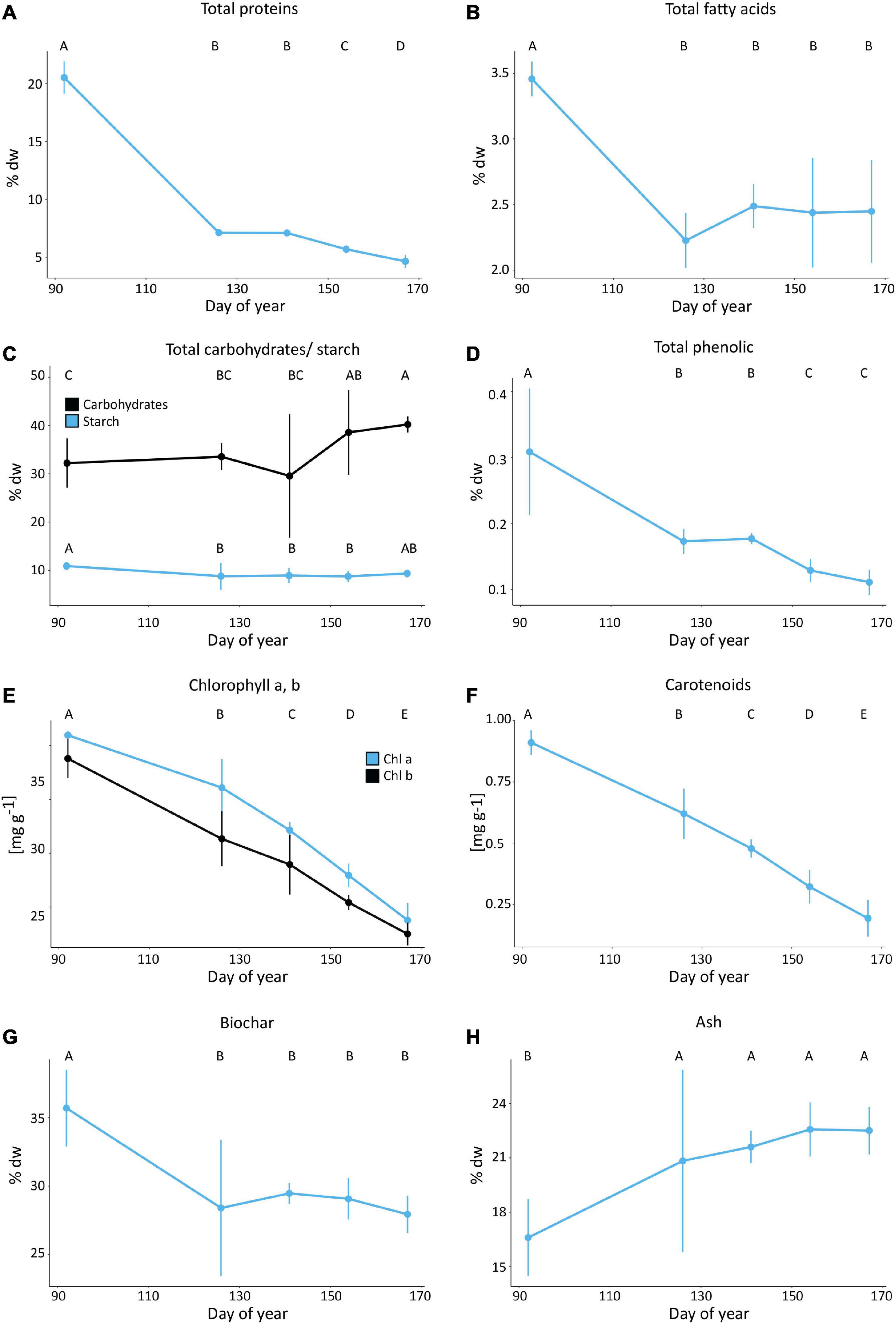
Figure 3. Mean (A) protein (% dw), (B) total fatty acid (% dw), (C) total carbohydrates including starch, (D) phenolic (% dw), (E) chlorophyll a, and b (mg g–1), (F) carotenoids (mg g–1), (G) biochar (% dw), and (H) ash (% dw) content of off-shore cultivated U. fenestrata recorded at five different harvest dates (DOY 92, 126, 141, 154, 167). Error bars show 95% confidence interval and capital letters above bars show significant differences between means based on Tukey’s HSD test (p < 0.05).
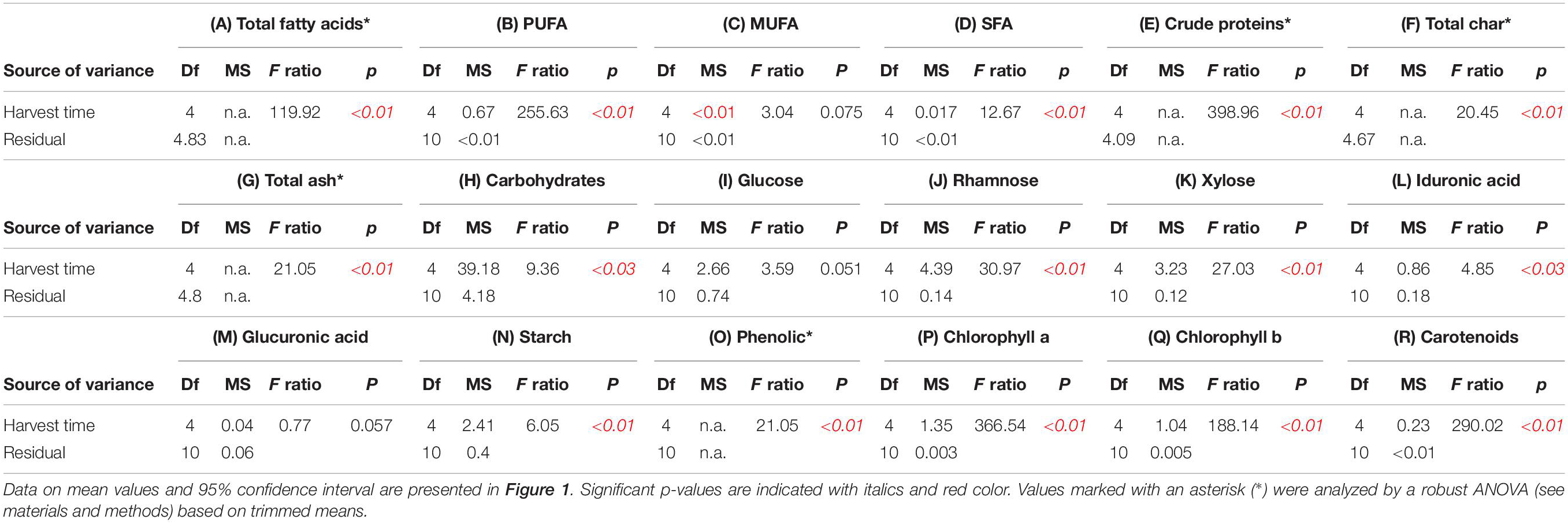
Table 2. ANOVAs of (A) fatty acid content (% dw), (B) PUFA (% dw), (C) MUFA (% dw), (D) SFA (% dw), (E) crude protein content (% dw), (F) total char (% dw), (G) total ash (% dw), (H) carbohydrate content (% dw), (I) glucose content (% dw), (J) rhamnose content (% dw), (K) xylose content (% dw), (L) iduronic acid content (% dw), (M) glucuronic acid content (% dw), (N) starch content, (O) phenolic content (% dw), (P) chlorophyll a (μg mg–1), (Q) chlorophyll b (μg mg–1), and (R) carotenoids (μg mg–1) of sea-based cultivated Ulva fenestrata biomass harvested at different dates (see also Figure 1).
Fatty Acid Content and Composition
The highest amount of total fatty acids 3.45% dw was observed in U. fenestrata at the first harvest point (DOY 92) (Figure 3B and Table 2A) followed by an abrupt and significant decrease at the following harvest points (DOY 126–167) where the total amount of fatty acids varied from 2.22 – 2.48% dw (Figures 3A,B and Table 2A). The total (Figure 4A and Tables 2B–D) and relative amount (Figure 4B) of polyunsaturated fatty acids (PUFA), monounsaturated fatty acids (MUFA), and saturated fatty acids (SFA), as well as the relative content of the 22 most prevalent FA, were analyzed (Figure 4C and Table 3).
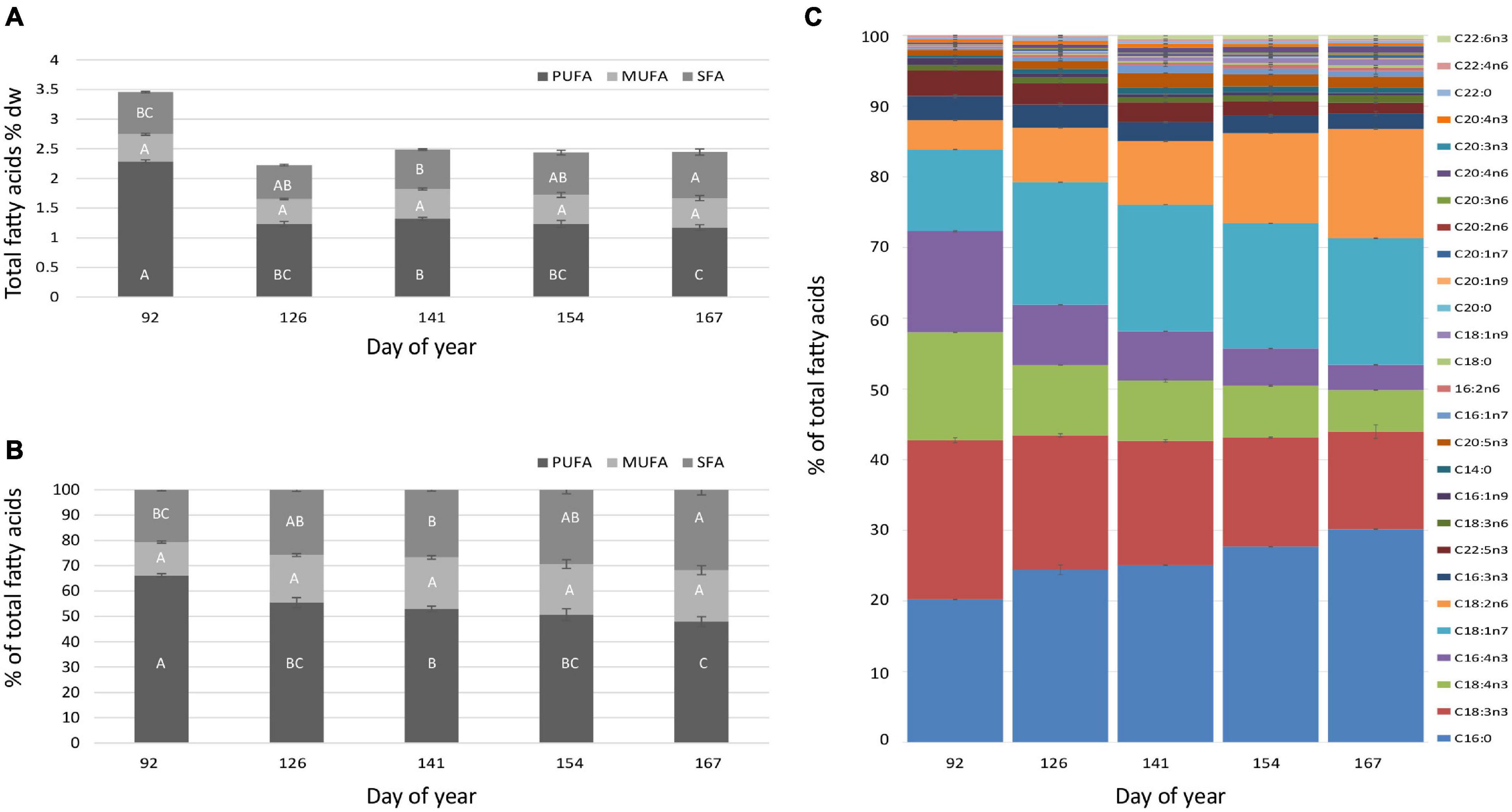
Figure 4. Overview of the (A) total (% dw) and (B) relative (% of total fatty acids) fatty acid composition in off-shore cultivated U. fenestrata divided into the respective amount of PUFA, MUFA, and SFA and recorded at five different harvest dates (DOY 92, 126, 141, 154, 167). (C) Fatty acid composition of the most prevalent fatty acids (% of total fatty acids) in off-shore cultivated U. fenestrata recorded at five different harvest dates. Error bars show SD and capital letters above bars show significant differences between means based on Tukey’s HSD test (p < 0.05).
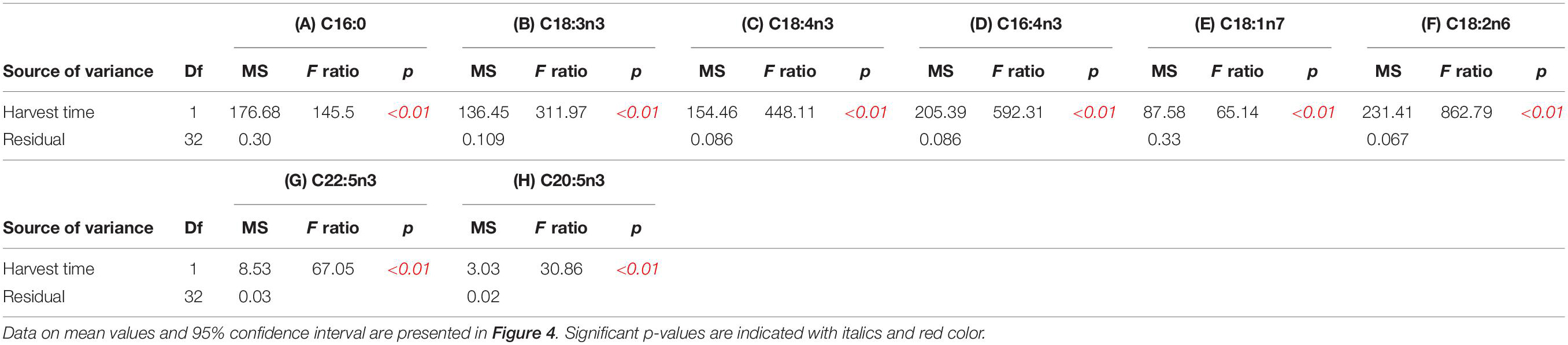
Table 3. ANOVAs of (A) C16:0% of total fatty acids, (B) C18:3n3% of total fatty acids, (C) C18:4n3% of total fatty acids (D) C16:4n3% of total fatty acids, (E) C18:1n7% of total fatty acids, (F) C18:2n6%, (G) C22:5n3, and (H) C20:5n3 of total fatty acids in of sea-based cultivated U. fenestrata biomass harvested at different dates (see also Figure 1).
Whereas the amount of PUFA is significantly higher (2.29% of total fatty acids) at the first harvest point (DOY 92) it successively decreased with prolonged cultivation periods where the amount varied from 1.17% to 1.32% (Figure 4 and Table 2B). Harvest time was not found to significantly affect the MUFA content (Figure 4 and Table 2C). Although significant differences in the amount of SFA were detected, only a trend of increasing SFA with prolonged cultivation time was detectable (Figure 4 and Table 2D).
The variation in fatty acid composition became even more obvious when the 22 most prevalent fatty acids were analysed (Figure 4C and Table 3). Our results pointed out that palmitic acid (C16:0), alpha-linolenic acid (C18:3n3), stearidonic acid (C18:4n3), hexadecatetraenoic acid (C16:4n3), vaccenic acid (C18:1n7), and linoleic acid (C18:2n6) were the dominant fatty acids in Ulva (Figure 4C). Palmitic acid (C16:0) and linoleic acid (C18:2n6) significantly increased throughout the cultivation period, whereas a significant decrease of alpha-linolenic acid (C18:3n3), stearidonic acid (C18:4n3), and hexadecatetraenoic acid (C16:4n3) was observed throughout the whole cultivation time (Figure 4C and Table 3). Vaccenic acid (C18:1n7) had a significantly lower amount during the first harvest (DOY 92) compared to the following sampling points (DOY 126–167) (Figure 4C and Table 3).
Specific health-beneficial long-chain n-3 PUFA’s (LC n-3 PUFA) were detected, among others docosapentaenoic acid (DPA, C22:5n3) and eicosapentaenoic acid (EPA, C20:5n3) (Figure 4). Whereas DPA (C22:5n3) significantly (p < 0.01) decreased from 3.69% (DOY 92) to 1.53% (DOY 167) of total fatty acids throughout the cultivation period, highest values of EPA (C20:5n3) (2.09%) were observed at DOY 141 (Figure 4C and Table 3).
Carbohydrate Content and Composition Including Starch
The total carbohydrate content significantly increased with prolonged cultivation time (Figures 3C, 5 and Table 2H) from 32.21% dw on the first harvest date (DOY 92) to 40.21% dw at the last harvest date of the experiment (DOY 167) resulting in a 24.84% increase of total carbohydrates in the U. fenestrata biomass.
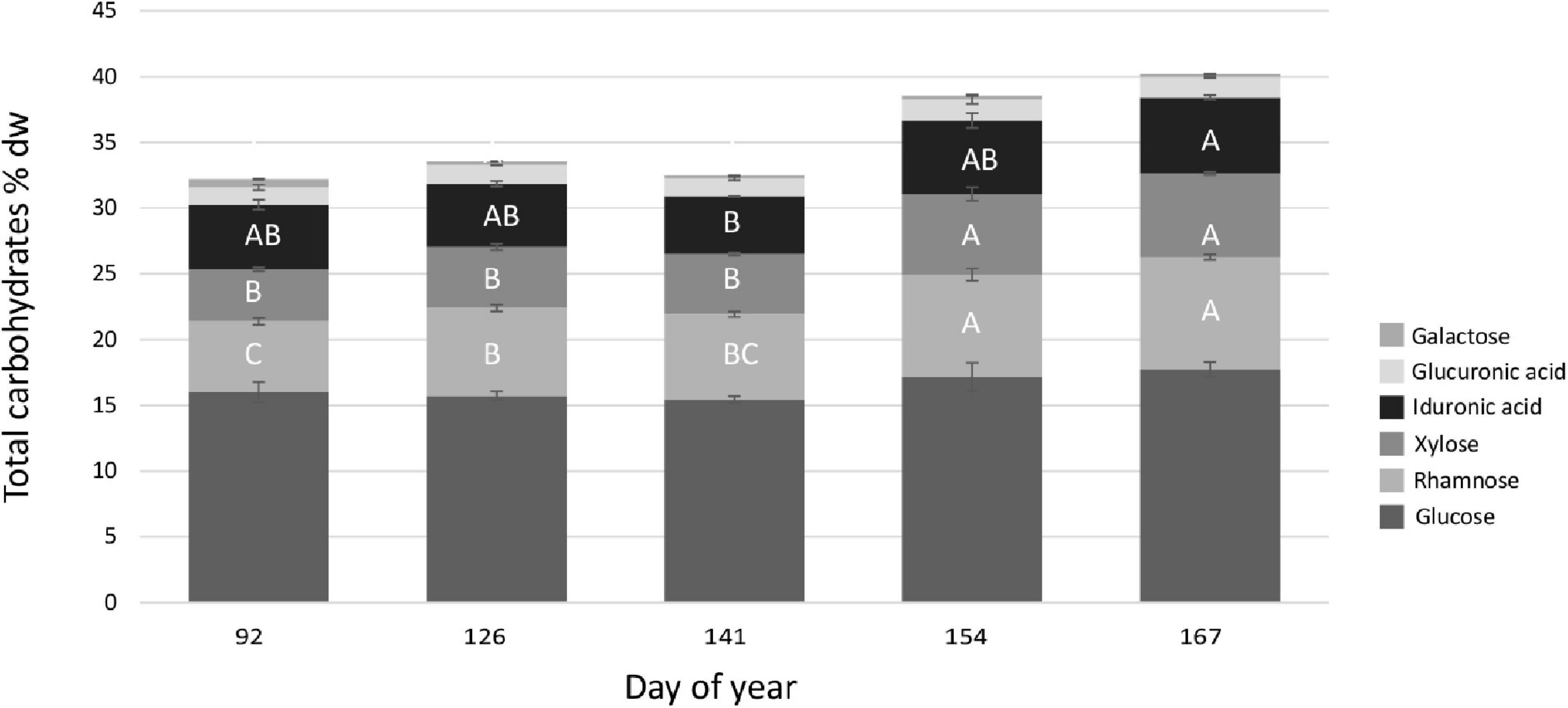
Figure 5. Overview of the total (% dw) carbohydrate composition in off-shore cultivated U. fenestrata divided into the respective amount of glucose, rhamnose, xylose, iduronic acid, glucuronic acid, and galactose at five different harvest dates (DOY 92, 126, 141, 154, 167). Error bars show SD and capital letters above bars show significant differences between means based on Tukey’s HSD test (p < 0.05).
Glucose was the dominating monosaccharide in the carbohydrates, with contents in the range of 15.17–18.16% dw, whereas rhamnose was the second most abundant monosaccharide at 5.17–48.71% dw, followed by xylose at 3.77–6.69% dw. The high-value monosaccharide iduronic acid was detected at 4.35–6.3% dw, and glucuronic acid was detected in the range of 1.15–2.12% dw, whereas galactose was found at relatively low values of 0.23–0.68% dw (Figure 5 and Tables 2I–M). The harvest time point had a significant effect (p < 0.01) on the amount of rhamnose, xylose, and iduronic acid in the off-shore cultivated U. fenestrata biomass, and a general increase of the respective monosaccharides was observed with prolonged cultivation time (Figure 5 and Tables 2J–L). Glucose and glucuronic acid amounts were not affected by harvest time (Figure 5 and Tables 2I,M).
Starch content ranged between 8.93 and 11.07% dw (Figure 3C) and was significantly affected by the time of harvest. The starch content at the first harvest time point (DOY 92) was significantly higher (11.07% dw) compared to that of DOY 126, 141, and 154 (8.97–9.1% dw), but no significant difference among the first and last harvest time point (DOY 167) (9.53% dw) was found (Figure 3C and Table 2N).
Phenolic Content
The highest phenolic content (0.31% dw) of the cultivated U. fenestrata was measured at the first sampling point of our experiment (DOY 92) (Figure 3D and Table 2O). It significantly decreased with prolonged cultivations (DOY 126, 141) and reached its minimum of 0.11 – 0.12% dw at the last harvest dates (DOY 154, 167) (Figure 3D and Table 2O).
Pigment Content
The pigment content, including chlorophyll a, b and carotenoids showed a significant (p < 0.01) and continuous decrease with prolonged cultivation time (Figures 3E,F and Tables 2P–R). Whereas highest chlorophyll a (2.09 mg g–1), chlorophyll b (1.79 mg g–1), and carotenoid (0.91 mg g–1) contents were observed at the first harvest time point in early spring (DOY 92), the lowest chlorophyll a (0.377 mg g–1), chlorophyll b (0.25 mg g–1), and carotenoid (0.19 mg g–1) contents were observed at the end of the experiment (DOY 167) in June (Figures 3E,F and Tables 2P–R).
Biochar and Ash Content
The biochar content was significantly (p < 0.01) higher during the first sampling (DOY 92; 35.7% dw) than compared to all other sampling dates (27.93–29.47% dw) (Figure 3G and Table 2F). The ash content was significantly (p < 0.01) lower during the first sampling (DOY 92; 16.6% dw) than compared to all other sampling dates (20.83–22.56% dw) (Figure 3H and Table 2G). Additionally, samples collected at the first time point (DOY 92) had a higher temperature stability in the sense that major thermal degradation occurred at higher temperature compared to the other sampling dates.
Element Content and Inorganic Arsenic
The results from the analysis of total elements and iAs in U. fenestrata are presented in Table 4 in μg/kg fresh weight. Some non-essential elements that may pose a risk of increased intake when consuming U. fenestrata compared to other food sources are inorganic arsenic, lead, nickel, and vanadium. The results for vanadium show almost a factor of thousand higher levels than the levels of the compared foodstuffs. Most of the non-essential elements increase with prolonged cultivation time (Table 4). For most of the essential elements U. fenestrate seems to be a good source and show values in the range of the compared foodstuffs.
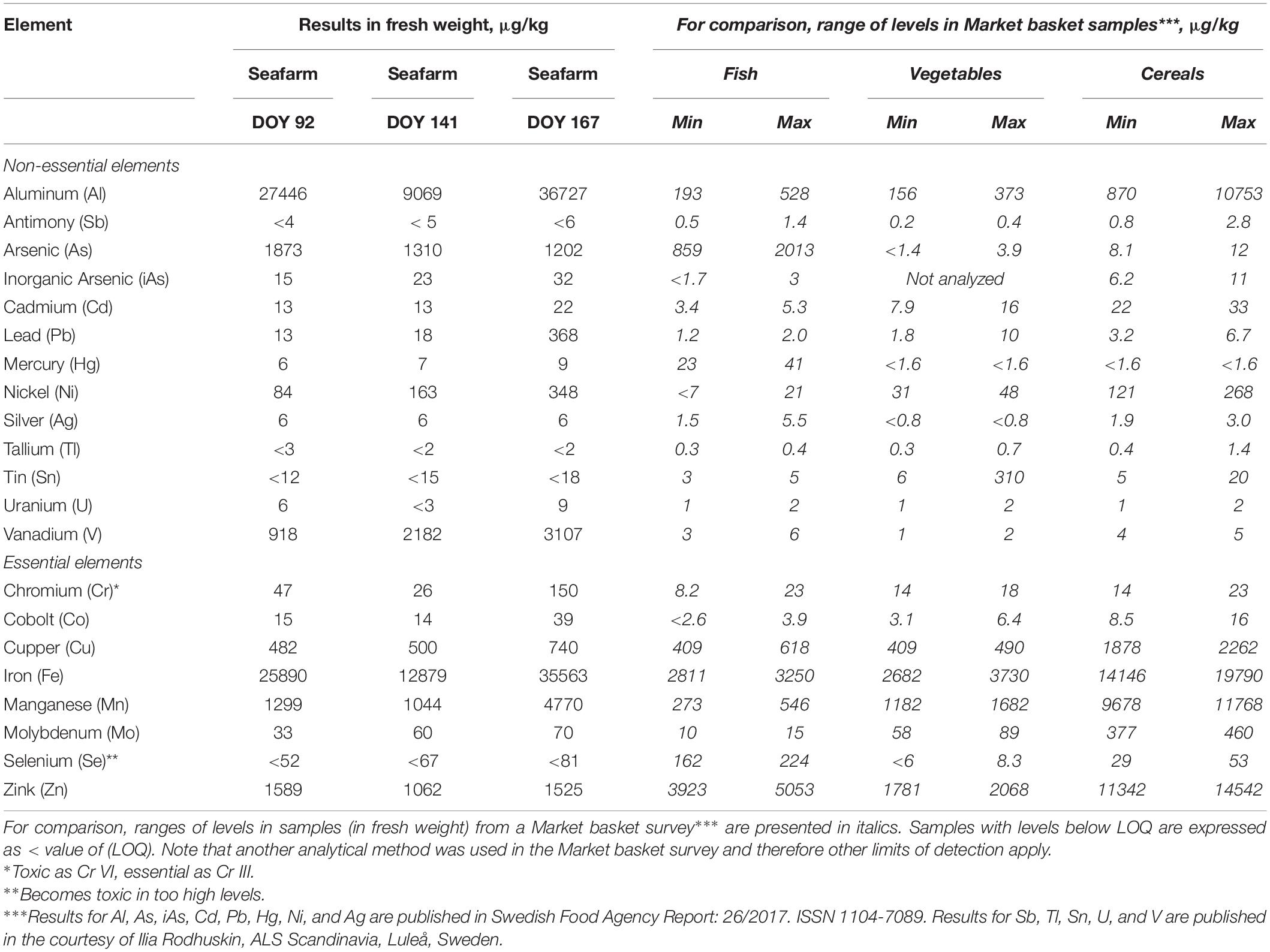
Table 4. Levels of non-essential (more or less toxic) and essential elements in sea-based cultivated U. fenestrata (fresh weight) from three different harvest time points (01.04.2020 = DOY 96; 20.05.2020 = DOY 141; 15.06.2020 = DOY 167).
Discussion
Our results show the necessity of controlling harvest times in a sea-based cultivation of U. fenestrata, in terms of obtaining a desired biomass yield and/or quality. This conclusion is based on (1) an increase of total biomass of up to 349%, (2) a successive increase of thallus fertility and biofouling, and (3) a strongly varying biochemical composition of the produced biomass. Since the biomass yield and biochemical composition determine the respective economic value of the sea-based produced biomass of U. fenestrata, our results emphasize the importance of harvest time selection to enable for a commercial usage of the seaweed biomass. Although our study yields first important data on the economic value of sea-based cultivated U. fenestrata in the Northern Hemisphere, a long-term monitoring is needed to assess annual variation especially with the background of climate change and changing abiotic factors (IPCC, 2021).
Biomass Yield, Thallus Habitus, and Biofouling
We found considerable seasonal variations in the total biomass yield, which peaked at the third harvest date in late spring (1217 g fw m rope–1 corresponding to 97 g dw m rope–1). Due to a notoriously difficult taxonomy of the genus Ulva (e.g., Hayden et al., 2003; Steinhagen et al., 2019) and the absence of comparable studies focusing on sustainable sea-based cultivation of Ulva spp. in the Northern Hemisphere, it is difficult to put results in a more global context. Generally, the exact taxonomic identity of Ulva spp. used in mariculture literature is widely ambiguous due to the lack of molecular identification (Bolton et al., 2016; Roleda and Heesch, 2021). To disentangle the effect of genetic or environmental factors and to enable geographic and site-specific selections of suitable Ulva spp. and strains we underline the importance of molecular identification of source material. However, a study on off-shore cultivation of tropical, filamentous Ulva species (Carl et al., 2014) yielded 228.7 ± 115.4 g fw [m rope]–1 (corresponding to 23.0 ± 8.8 g dw [m rope]–1). Although the species, nursery and cultivation periods of both studies are distinctively different, also Carl et al. (2014) emphasized that the timing of harvest is critical for an optimal biomass yield. The continuous loss of biomass after the third harvest time found in the present study can partly be explained by the weakened and instable thalli due to the increased number and size of holes, as well as by the increase of fertile thallus tissue during the last sampling date. As observed by Nilsen and Nordby (1975), fertility was mainly present at the thallus margins and at frond parts with high number of holes. Since fertility events lead to the complete disintegration of the affected distromatic thallus parts, the harvest should be adjusted to avoid massive biomass loss.
As typical for the Skagerrak region, the amount of biofoulers is relatively low in winter and early spring and increases with rising seawater temperatures at the beginning of summer (see also Visch et al., 2020). Biofoulers were absent from the sea-based cultivated U. fenestrata until late spring, and when biomass production peaked at the third harvest time point, the total amount of biofoulers was still relatively low (2%) and fouling organisms were mainly found at the very basal thallus parts. Fouling taxa overlapped with previous findings in ocean-based kelp aquacultures in the Skagerrak (Visch et al., 2020) and the Norwegian Sea (Forbord et al., 2020). Biofouling has been identified as a key constraint and depreciation factor in seaweed farms worldwide (Lüning and Mortensen, 2015; Kim et al., 2017), especially when seaweed biomass is used in food- and feed processing. Hence, a harvest in spring is recommended if the biomass is intended to be used for food- and feed application, which is also reflected by the protein, fatty acid and phytochemical data. To further increase the total biomass yield of sea-cultivated U. fenestrata, the influence of different farm sites should be evaluated to enable optimal site selection (e.g., Visch et al., 2020). In addition, breeding strategies of native strains should be adapted, which have shown to yield very productive crops not only in terrestrial crop systems but also in marine seaweeds (e.g., Hwang et al., 2012).
Due to the relatively low maintenance costs compared to on-shore cultivation (Titlyanov and Titlyanova, 2010; Palatnik and Zilberman, 2017; Steinhagen et al., 2021), sea-based cultivation settings could address the profitability of seaweed biomass production in Europe. Especially the high biomass yields of Ulva biomass in off-shore rope cultivation could benefit the industrial exploitation of this sustainably produced biomass source and offers an alternative to on-shore tank or pond cultivation.
Protein and Fatty Acid Content and Composition
Our study shows that the protein and total fatty acid content in early spring was higher compared to the other harvest dates. A lab-based study on the same strain of U. fenestrata showed that increased temperature and irradiance negatively affected the total protein and fatty acid content (Toth et al., 2020), which is in accordance with the findings of our study. Furthermore, decreased dissolved inorganic nitrogen and phosphorous concentrations in seawater during summer could also result in low protein and fatty acid levels (c.f. Toth et al., 2020). Moreover, since the maximum biomass yield was achieved at the third harvest time, the amount of protein and fatty acids per m–1 rope was higher in early summer (17.14 and 5.97 g m–1 rope, respectively) compared to early spring (8.57 and 1.44 g m–1 rope) (values are calculated based on the relative amount of biomass per m rope per season and the average amount of protein per g dw; see Figures 2, 3A,B). It is therefore depending on downstream biorefinery processes if a higher biomass yield could be more beneficial economically than maximizing the protein or fatty acid content of the crop. Furthermore, the value of sea-based cultivated U. fenestrata biomass for the food industry could strongly depend on the fatty acid profile. We found that the composition of the most common fatty acids in U. fenestrata was strongly seasonal dependent and thus the harvest date should be considered in regard to the desired fatty acid profile. In accordance with the conclusions by Khotimchenko (1991) for other seaweeds, the amount of PUFAs in U. fenestrata biomass was highest in early spring. Whereas the amount of MUFAs stayed relatively stable and no significant differences among the harvest dates were detectable, the amount of SFAs slowly increased with prolonged cultivation periods. The influence of harvest time on the amount of PUFAs is particularly interesting in respect to functional foods, which generally aim for high PUFA contents (Holdt and Kraan, 2011).
Carbohydrate Content and Composition Including Starch
Seaweeds are generally containing relatively high amounts of polysaccharides, which can be found as structural components of the cell wall, as storage polysaccharides, and as mucopolysaccharides (Holdt and Kraan, 2011). Our mean values of total carbohydrates (32.21 – 40.21% dw) of sea-based cultivated U. fenestrata are in the middle range of what has previously been reported for Ulva spp. (max. 65%, Holdt and Kraan, 2011; 25–38%, Olsson et al., 2020a,b). With prolonged cultivation time, total carbohydrates increased by 29% at the end of the experiment at summer conditions compared to spring conditions. Previous studies confirm that elevated temperature has a positive effect on the carbohydrate content in Ulva spp. (He et al., 2018; Olsson et al., 2020a). The seasonal variation in carbohydrate content can have implications for industrial-scale extraction of carbohydrates from U. fenestrata biomass. However, as discussed above it depends on the subsequent biorefining and extraction processes if higher biomass yields are more economic than increasing the carbohydrate content of the biomass.
In addition to the total content, carbohydrate composition differed between different harvest times. Here, the high-value monosaccharides rhamnose and iduronic acid are especially relevant. Rhamnose is widely used in the food industry for the production of flavoring aroma compounds and additionally functions as potential raw material to produce rhamnolipids (Holdt and Kraan, 2011; Muller et al., 2012). Iduronic acid is used in the synthesis of heparin fragment analogs with anti-thrombotic activities. The extraction of iduronic acid from U. fenestrata offers an attractive alternative to the commonly applied synthetic procedure which is time- and cost-intensive (Holdt and Kraan, 2011; Mohamed and Ferro, 2015). The rhamnose content of U. fenestrata increased by 54.73% at the last harvest in summer, but no clear seasonal trend was found for the iduronic acid content in U. fenestrata in this study. However, compared to previous studies (>1% dw, Olsson et al., 2020a; 1.77–3.51% dw, Olsson et al., 2020b; 1.4–1.8% dw, Steinhagen et al., 2021) the iduronic acid content in this study (4.39–5.77% dw) was distinctly higher, which adds to the beneficial effects of sea-based cultivation of U. fenestrata. This study shows that summer conditions are favorable for the optimization of the carbohydrate content in sea-cultivated U. fenestrata, but again, the total biomass yield has to be considered to optimize future commercial production schedules.
Total Phenolic and Pigment Content
Phenolics, chlorophyll, and carotenoids – so-called phytochemicals - are known for their health benefiting properties as they have antioxidant (Lanfer-Marquez et al., 2005) and anti-inflammatory effects (Abd El-Baky et al., 2009). The anti-tumoral activity of chlorophyll (Egner et al., 2003) and the prevention of free radical damage associated with the aging processes by carotenoids (Khairy and El-Sheikh, 2015) make seaweed biomass especially interesting for the cosmeceutical and pharmacological sector and as functional foods.
Early spring conditions clearly favored the phenolic and pigment (i.e., chlorophyll a, b, and carotenoids) content in the ocean-cultivated U. fenestrata whereas prolonged cultivation time had a negative effect on the phenolic and pigment content. Our findings agree with Khairy and El-Sheikh (2015), who stated that seasonality and consequently changing abiotic factors strongly influence the total phenol content. Similar seasonal dependent variations in chlorophyll and carotenoid content have been observed in other seaweed species as well (Holdt and Kraan, 2011) and our study underlines that harvest time is crucial to take into consideration to optimize the phenolic and pigment content in Northern Hemisphere U. fenestrata. As stated above, the highest amount of bioactive phytochemicals aligns with the highest amount of protein, and a harvest of the biomass for food- and feed application in early spring is recommended.
Biochar and Ash Content
Biochar is a carbon-rich co-product produced when pyrolyzing biomass and pyrolized seaweed biomass has a great potential to be used in soil amelioration (Lehmann and Joseph, 2009; Biederman and Harpole, 2013). Our results show that the biochar content was higher during early spring at the first harvest and notably, the values measured during the first harvest of this study (35.7% dw) were in the upper range of what has been reported in previous studies of the same species (30.3–33.82% dw, Toth et al., 2020) and other Ulva species (Ulva ohnoi 34–35% dw, Roberts et al., 2015). Whereas the biochar content was higher in early spring, the ash content was significantly lower at the first harvest time point. This implies a significant shift from a more organic biomass content with higher temperature stability in early spring to a higher ash content and higher temperature instability of the biomass with prolonged cultivation time. Therefore, our results agree with previous studies (Gao et al., 2017b, 2018a,b; Mhatre et al., 2019), which emphasized a positive effect of increased irradiance and temperature on the ash content of Ulva spp.
Element Content and Inorganic Arsenic
If “traditional” foodstuffs are to be fully or partly substituted by seaweeds it is of utmost importance to elucidate how this will affect the intake of toxic elements as well as nutritional elements. With the rising interest in seaweed as food- and feed source, different studies have acknowledged the need to identify the safety of seaweed (van den Burg et al., 2013; van der Spiegel et al., 2013; Banach et al., 2019). The European Union determines maximum levels (ML:s) for several toxic elements in foodstuffs in Commission Regulation (EC) No. 1881/2006. However, it is important to stress that ML:s in EU legislation are not fixed levels but are under continuous evaluation adapting to revised standards and newly registered foodstuffs. Our study shows that most of the non-essential elements increase with prolonged cultivation time. Seaweeds are known to rapidly accumulate elements from their surrounding environment and this process is for example used in bioremediation approaches (Neveux et al., 2018). Thus, an increase of elements, including iAs, with an increased growth period is to be expected, however, this emphasizes careful selection of suitable farm grounds, growth seasons, and harvest time points to reduce toxic elements in seaweed biomass aimed for the food- and feed sector. Moreover, concentrations of all essential elements except for Zn were highest at the last harvest date. With this study we provide the first knowledge on the quantity of non-essential elements and iAS which might function as safety hazards as well as essential elements in sea-cultivated U. fenestrata. From a food- and feed perspective we recommend an early harvest of the biomass in spring to keep amounts of non-essential elements and iAS at the lowest rate.
Conclusion
In conclusion, our findings together with those of another recent study (Steinhagen et al., 2021) identifies U. fenestrata as a suitable crop for large-scale production in marine Scandinavian waters and gives a first knowledge basis for the selection of optimal harvest times, depending on desired yield and/or biochemical composition of the biomass to support subsequent refining processes. To obtain the best quality of cultivated, sea-based U. fenestrata for general food and feed applications (i.e., high LC n3 fatty acids, total protein, and phytochemical concentration) as well as to avoid fouling and toxic elements, a harvest in early spring is recommended.
Data Availability Statement
The original contributions presented in the study are included in the article/supplementary materials, further inquiries can be directed to the corresponding author/s.
Author Contributions
SST: conceptualization of the study, implementation of experiment, investigation, data analyses, visualization, and original draft. SE: data collection, data analyses, and original draft. GC: data collection and refining of draft. KL, UE, AS, and NW: data analyses and refining of draft. BK: data analyses and original draft. HP: funding acquisition and refining of draft. IU and GT: funding acquisition, data analyses, and refining of draft. All authors: read and agreed to the published version of the manuscript.
Funding
The authors thank the Swedish Foundation for Strategic Research (SSF)-funded “SWEAWEED” project (project number RBP14-0045), as well as the Formas-funded ‘CirkAlg’ project (Grant no. 2018-01839) for financial support.
Conflict of Interest
The authors declare that the research was conducted in the absence of any commercial or financial relationships that could be construed as a potential conflict of interest.
Publisher’s Note
All claims expressed in this article are solely those of the authors and do not necessarily represent those of their affiliated organizations, or those of the publisher, the editors and the reviewers. Any product that may be evaluated in this article, or claim that may be made by its manufacturer, is not guaranteed or endorsed by the publisher.
References
Abd El-Baky, H. H., El-Baz, F. K., and El-Baroty, G. S. (2009). Natural preservative ingredient from marine alga Ulva lactuca L. Int. J. Food Sci. Technol. 44, 1688–1695. doi: 10.1111/j.1365-2621.2009.01926.x
Abreu, M. H., Pereira, R., and Sassi, J. F. (2014). “Marine algae and the global food industry,” in Marine Algae: Biodiversity, Taxonomy, Environmental Assessment, and Biotechnology, eds L. Pereira and J. Magalhaes Neto (Boca Raton, FL: CRC Press), 300–319. doi: 10.1201/b17540
Angell, A. R., Mata, L., de Nys, R., and Paul, N. A. (2016). The protein content of seaweeds: a universal nitrogen-to-protein conversion factor of five. J. Appl. Phycol. 28, 511–524. doi: 10.1007/s10811-015-0650-1
Araújo, R., Vázquez Calderón, F., Sánchez López, J., Azevedo, I. C., Bruhn, A., Fluch, S., et al. (2021). Current status of the algae production industry in Europe: an emerging sector of the blue bioeconomy. Front. Mar. Sci. 7:626389. doi: 10.3389/fmars.2020.626389
Banach, J. L., Hoek-van den Hill, E. F., and van der Fels-Klerx, H. J. (2019). Food safety hazards in the European seaweed chain. Compr. Rev. Food Sci. Food Saf. 19, 322–364. doi: 10.1111/1541-4337.12523
Bannister, J., Sievers, M., Bush, F., and Bloecher, N. (2019). Biofouling in marine aquaculture: a review of recent research and developments. Biofouling 35, 631–648. doi: 10.1080/08927014.2019.1640214
Biederman, L. A., and Harpole, W. S. (2013). Biochar and its effects on plant productivity and nutrient cycling: a meta-analysis. GCB Bioenergy 5, 202–214. doi: 10.1111/gcbb.12037
Bikker, P., van Krimpen, M. M., van Wikselaar, P., Houweling-Tan, B., Scaccia, N., van Hal, J. W., et al. (2016). Biorefinery of the green seaweed Ulva lactuca to produce animal feed, chemicals and biofuels. J. Appl. Phycol. 28, 3511–3525. doi: 10.1007/s10811-016-0842-3
Bolton, J. J., Cyrus, M. D., Brand, M. J., Joubert, M., and Macey, B. M. (2016). Why grow Ulva? Its potential role in the future of aquaculture. Perspect. Phycol. 3, 113–120. doi: 10.1127/pip/2016/0058
Bolton, J. J., Robertson-Andersson, D. V., Shuuluka, D., and Kandjengo, L. (2009). Growing Ulva (Chlorophyta) in integrated systems as a commercial crop for abalone feed in South Africa: a SWOT analysis. J. Appl. Phycol. 21, 575–583. doi: 10.1007/s10811-008-9385-6
Buchholz, C. M., Krause, G., and Buck, B. H. (2012). “Seaweed and Man,” in Seaweed Biology: Ecological Studies, 219, eds C. Wiencke and K. Bischof (Berlin: Springer), 471–493. doi: 10.1007/978-3-642-28451-9_22
Califano, G., Kwantes, M., Abreu, M. H., Costa, R., and Wichard, T. (2020). Cultivating the macroalgal holobiont: effects of integrated multi-trophic-aquaculture on the microbiome of Ulva rigida (Chlorophyta). Front. Mar. Sci. 7:52. doi: 10.3389/fmars.2020.00052
Cancino, J., Mufioz, J., Mufioz, M., and Orellana, M. (1987). Effects of the bryozoan Membranipora tuberculata (Bosc.) on the photosynthesis and growth of Gelidium rex Santelices et Abbott. J. Exp. Mar. Biol. Ecol. 113, 105–112.
Carl, C., de Nys, R., Lawton, R. J., and Paul, N. A. (2014). methods for the induction of reproduction in a tropical species of filamentous Ulva. PLoS One 9:e97396. doi: 10.1371/journal.pone.0097396
Colombo, M. L., Risè, P., Giavarini, F., Angelis, D. E. L., Galli, C., and Bolis, C. L. (2006). Marine macroalgae as sources of polyunsaturated fatty acids. Plant Foods Hum. Nutr. 61, 67–72.
Dixon, J., Schroeter, S. C., and Kastendiek, J. (1981). Effects of the encrusting bryozoan, Membranipora membranacea, on the loss of blades and fronds by the giant kelp, Macrocystis pyrifera (Laminariales). J. Phycol. 17, 341–345.
Duarte, C. M., Holmer, M., Olsen, Y., Soto, D., Marbà, N., Guiu, J., et al. (2009). Will the oceans help feed humanity? Bioscience 59, 967–976. doi: 10.1525/bio.2009.59.11.8
Egner, P. A., Muñoz, A., and Kensler, T. W. (2003). Chemoprevention with chlorophyllin in individuals exposed to dietary aflatoxin. Mutat. Res. 523, 209–216. doi: 10.1016/s0027-5107(02)00337-8
European Commission 2019/909 (2019). Commission Implementing Decision (EU) 2019/909 of 18 February 2019 Establishing the List of Mandatory Research Surveys and Thresholds for the Purposes of the Multiannual Union Programme for the Collection and Management of data in the Fisheries and Aquaculture Sectors. Available online at: http://dcf.mir.gdynia.pl/wp-content/uploads/2019/10/COM_Impl-Decision_2019-909_EU-MAP_surveys-thresholds.pdf (accessed February, 2021).
FAO (2018). The State of World Fisheries and Aquaculture 2018 – Meeting the Sustainable Development Goals. Rome: FAO.
Forbord, S., Matsson, S., Brodahl, G. E., Bluhm, B. A., Broch, O. J., Handå, A., et al. (2020). Latitudinal, seasonal and depth-dependent variation in growth, chemical composition and biofouling of cultivated Saccharina latissima (Phaeophyceae) along the Norwegian coast. J. Appl. Phycol. 32, 2215–2232. doi: 10.1007/s10811-020-02038-y
Førde, H., Forbord, S., Handå, A., Fossberg, J., Arff, J., Johnsen, G., et al. (2015). Development of bryozoan fouling on cultivated kelp (Saccharina latissima) in Norway. J. Appl. Phycol. 28, 1225–1234.
Fort, A., McHale, M., Cascella, K., Potin, P., Perrineau, M. M., Kerrison, P., et al. (2020). Exhaustive Reanalysis of Barcode Sequences from Public Repositories Highlights Ongoing Misidentifications and Impacts Taxa Diversity and Distribution: A Case Study of the Sea Lettuce. Available online at: https://d197for5662m48.cloudfront.net/documents/publicationstatus/53373/preprint_pdf/a8eb78ca0540179f09881a1031f0fbc9.pdf (accessed December, 2020).
Gao, G., Burgess, G., Wu, M., Wang, S., and Gao, K. (2020). Using macroalgae as biofuel: current opportunities and challenges. Bot. Mar. 63, 355–370. doi: 10.1016/j.ymben.2022.01.005
Gao, G., Clare, A. S., Chatzidimitriou, E., Rose, C., and Caldwell, G. (2018a). Effects of ocean warming and acidification, combined with nutrient enrichment, on chemical composition and functional properties of Ulva rigida. Food Chem. 258, 71–78. doi: 10.1016/j.foodchem.2018.03.040
Gao, G., Clare, A. S., Rose, C., and Caldwell, G. S. (2018b). Ulva rigida in the future ocean: potential for carbon capture, bioremediation and biomethane production. GBC Bioenergy 10, 39–51. doi: 10.1111/gcbb.12465
Gao, G., Clare, A. S., Rose, C., and Caldwell, G. S. (2017a). Reproductive sterility increases the capacity to exploit the green seaweed Ulva rigida for commercial applications. Algal Res. 24, 64–71. doi: 10.1016/j.algal.2017.03.008
Gao, G., Clare, A. S., Rose, C., and Caldwell, G. S. (2017b). Eutrophication and warming-driven green tides (Ulva rigida) are predicted to increase under future climate change scenarios. Mar. Pollut. Bull. 114, 439–447. doi: 10.1016/j.marpolbul.2016.10.003
Hafting, J. T., Craigie, J. S., Stengel, D. B., Loureiro, R. R., Buschmann, A. H., Yarish, C., et al. (2015). Prospects and challenges for industrial production of seaweed bioactivities. J. Phycol. 51, 821–837. doi: 10.1111/jpy.12326
Harrysson, H., Hayes, M., Eimer, F., Carlsson, N. G., Toth, G. B., and Undeland, I. (2018). Production of protein extracts from Swedish red, green, and brown seaweeds, Porphyra umbilicalis Kützing, Ulva lactuca Linnaeus, and Saccharina latissima (Linnaeus) JV Lamouroux using three different methods. J. Appl. Phycol. 30, 3565–3580. doi: 10.1007/s10811-018-1481-7
Hayden, H. S., Blomster, J., Maggs, C. A., Silva, P. C., Stanhope, M. J., and Waaland, J. R. (2003). Linnaeus was right all along: Ulva and Enteromorpha are not distinct genera. Eur. J. Phycol. 38, 277–294.
He, Y., Hu, C., Wang, Y., Cui, D., Sun, X., Li, Y., et al. (2018). The metabolic survival strategy of marine macroalga Ulva prolifera under temperature stress. J. Appl. Phycol. 30, 3611–3621. doi: 10.1007/s10811-018-1493-3
Holdt, S. L., and Kraan, S. (2011). Bioactive compounds in seaweed: functional food applications and legislation. J. Appl. Phycol. 23, 543–597. doi: 10.1007/s10811-010-9632-5
Hughey, J. R., Maggs, C. A., Mineur, F., Jarvis, C., Miller, K. A., Shabaka, S. H., et al. (2019). Genetic analysis of the Linnaean Ulva lactuca (Ulvales, Chlorophyta) holotype and related type specimens reveals name misapplications, unexpected origins, and new synonymies. J. Phycol. 55, 503–508. doi: 10.1111/jpy.12860
Hurd, C. L. (2000). Water motion, marine macroalgal physiology, and production. J. Phycol. 36, 453–472. doi: 10.1046/j.1529-8817.2000.99139.x
Hwang, E. K., Gong, Y. G., and Park, C. S. (2012). Cultivation of a hybrid of free-living gametophytes between Undariopsis peterseniana and Undaria pinnatifida: morphological aspects and cultivation period. J. Appl. Phycol. 24, 401–408. doi: 10.1007/s10811-011-9727-7
IPCC (2021). “Climate Change 2021: the Physical Science Basis,” in Contribution of Working Group I to the Sixth Assessment Report of the Intergovernmental Panel on Climate Change, eds V. Masson-Delmotte, P. Zhai, A. Pirani, S. L. Connors, C. Péan, S. Berger, et al. (Cambridge: Cambridge University Press). doi: 10.1007/s10584-021-03233-7
Jeffrey, S., and Humphrey, G. (1975). New spectrophotometric equations for determining a, b, c1 and c2 in higher plants, algae and natural phytoplankton. Biochem. Physiol. Pflanzen 167, 191–194. doi: 10.1016/s0015-3796(17)30778-3
Johannesson, K. (1989). The bare zone of swedish rocky shores: Why is it there? Oikos 54, 77–86. doi: 10.2307/3565899
Khairy, H. M., and El-Sheikh, M. A. (2015). Antioxidant activity and mineral composition of three Mediterranean common seaweeds from Abu-Qir Bay. Egypt. Saudi J. Biol. Sci. 5, 623–630. doi: 10.1016/j.sjbs.2015.01.010
Khotimchenko, S. V. (1991). Fatty-acid composition of 7 Sargassum species. Phytochemistry 30, 2639–2641. doi: 10.1016/0031-9422(91)85113-e
Kim, J. O., Kim, W. S., Jeong, H. N., Choi, S. J., Seo, J. S., Park, M. A., et al. (2017). A survey of epiphytic organisms in cultured kelp Saccharina japonica in Korea. Fish. Aquat. Sci. 20:1.
Kollander, B., Sand, S., Almerud, P., Halldin Ankarberg, E., Concha, G., Barregard, L., et al. (2019). Inorganic arsenic in food products on the swedish market and a risk-based intake assessment. Sci. Total Environ. 672, 525–535. doi: 10.1016/j.scitotenv.2019.03.289
Lanfer-Marquez, U. M., Barros, R. M., and Sinnecker, P. (2005). Antioxidant activity of chlorophylls and their derivatives. Food Res. Int. 38, 885–891. doi: 10.1016/j.foodres.2005.02.012
Lapointe, B. E., Williams, L. D., Goldman, J. C., and Ryther, J. H. (1976). The mass outdoor culture of macroscopic marine algae. Aquaculture 8, 9–21.
Lehmann, J., and Joseph, S. (eds) (2009). “Biochar for environmental management: an introduction,” in Biochar for Environmental Management: Science and Technology (Abingdon: Routledge), 33–46. doi: 10.4324/9780203762264-8
Lubsch, A., and Timmermans, K. (2018). Uptake kinetics and storage capacity of dissolved inorganic phosphorus and corresponding N:P dynamics in Ulva lactuca (Chlorophyta). J. Phycol. 54, 215–223. doi: 10.1111/jpy.12612
Lüning, K., and Mortensen, L. (2015). European aquaculture of sugar kelp (Saccharina latissima) for food industries: iodine content and epiphytic animals as major problems. Bot. Mar. 58, 449–455.
Lüning, K., and Pang, S. J. (2003). Mass cultivation of seaweed: current aspects and approaches. J. Appl. Phycol. 15, 115–119. doi: 10.1023/a:1023807503255
Mair, P., and Wilcox, R. (2018). ”Robust Statistical Methods Using WRS2.” The WRS2 Package. Available online at: https://cran.r-project.org/src/contrib/Archive/WRS2/ (accessed August, 2020).
Mhatre, A., Patil, S., Agrawal, A., Pandit, R., and Lali, A. M. (2019). Influence of nitrogen source on photochemistry and antenna size of the photosystems in marine green macroalgae, Ulva lactuca. Photosynth. Res. 139, 539–551. doi: 10.1007/s11120-018-0554-4
Mohamed, S., and Ferro, V. (2015). “Synthetic approaches to l-Iduronic acid and l-Idose: key building blocks for the preparation of glycosaminoglycan oligosaccharides,” in Advances in Carbohydrate Chemistry and Biochemistry, eds D. C. Baker and D. Horton (New York, NY: Academic Press), 21–61. doi: 10.1016/bs.accb.2015.07.001
Moreira, A. S., da Costa, E., Melo, T., Sulpice, R., Cardoso, S. M., Pitarma, B., et al. (2020). Seasonal plasticity of the polar lipidome of Ulva rigida cultivated in a sustainable integrated multi-trophic aquaculture. Algal Res. 49:101958.
Muller, M. M., Kugler, J. H., Henkel, M., Gerlitzki, M., Hormann, B., Pohnlein, M., et al. (2012). Rhamnolipids-next generation surfactants? J. Biotech. 162, 366–380. doi: 10.1016/j.jbiotec.2012.05.022
Neveux, N., Bolton, J. J., Bruhn, A., Roberts, D. A., and Ras, M. (2018). The bioremediation potential of seaweeds: recycling nitrogen, phosphorus, and other waste products. Blue Biotechnol. 1, 217–239. doi: 10.1002/9783527801718.ch7
Nilsen, G., and Nordby, O. (1975). Sporulation inhibiting substance from vegetative thalli of green alga Ulva mutabilis Føyn. Planta 125, 127–139. doi: 10.1007/BF00388699
Olsson, J., Toth, G. B., Oerbekke, A., Cvijetinovic, S., Wahlström, N., Harrysson, H., et al. (2020a). Cultivation conditions affect the monosaccharide composition in Ulva fenestrata. J. Appl. Phycol. 32, 3255–3263.
Olsson, J., Raikova, S., Mayers, J. J., Steinhagen, S., Chuck, C. J., Nylund, G. M., et al. (2020b). Effects of geographical location on potentially valuable components in Ulva intestinalis sampled along the Swedish coast. Appl. Phycol. 1, 80–92. doi: 10.1080/26388081.2020.1827454
Palatnik, R. R., and Zilberman, D. (2017). “Economics of natural resource utilization - the case of macroalgae,” in Modeling, Dynamics, Optimization and Bioeconomics II, eds A. A. Pinto and D. Zilberman (Cham: Springer), 1–21.
Park, C. S., and Hwang, E. K. (2012). Seasonality of epiphytic development of the hydroid Obelia geniculata on cultivated Saccharina japonica (Laminariaceae, Phaeophyta) in Korea. J. Appl. Phycol. 24, 433–439.
Parsons, T. R., Maita, Y., and Lalli, C. M. (1984). A Manual for Chemical and Biological Methods for Seawater Analysis. Oxford: Pergamon Press.
Provasoli, L. (1968). “Media and prospects for the cultivation of marine algae,” in Cultures and Collections of Algae,” in Proceedings of the 1968 US-Japan Conference Society of Plant Physiology, Hakone, 12–15.
Ray, D. K., and Foley, J. A. (2013). Increasing global crop harvest frequency: recent trends and future directions. Environ. Res. Lett. 8:044041.
Roberts, D. A., Paul, N. A., Dworjanyn, S. A., Bird, M. I., and de Nys, R. (2015). Biochar from commercially cultivated seaweed for soil amelioration. Sci. Rep. 5:9665. doi: 10.1038/srep09665
Roleda, M. Y., and Heesch, S. (2021). Chemical profiling of Ulva species for food applications: What is in a name? Food Chem. 361:130084. doi: 10.1016/j.foodchem.2021.130084
Roleda, M. Y., Lage, S., Aluwini, D. F., Rebours, C., Brurberg, M. B., Nitschke, U., et al. (2021). Chemical profiling of the Arctic sea lettuce Ulva lactuca (Chlorophyta) mass-cultivated on land under controlled conditions for food applications. Food Chem. 341:127999. doi: 10.1016/j.foodchem.2020.127999
Rolin, C., Inkster, R., Laing, J., and McEvoy, L. (2017). Regrowth and biofouling in two species of cultivated kelp in the Shetland Islands, UK. J. Appl. Phycol. 29, 2351–2361.
Santos, S. A. O., Vilela, C., Freire, C. S. R., Abreu, M. H., Rocha, S. M., and Silvestre, A. J. D. (2015). Chlorophyta and Rhodophyta macroalgae: a source of health promoting phytochemicals. Food Chem. 183, 122–128. doi: 10.1016/j.foodchem.2015.03.006
Schneider, C. A., Rasband, W. S., and Eliceiri, K. W. (2012). NIH image to imagej: 25 years of image analysis. Nat. Methods 9, 671–675. doi: 10.1038/nmeth.2089
Sebök, S., Herppich, W. B., and Hanelt, D. (2019). Outdoor cultivation of Ulva lactuca in a recently developed ring-shaped photobioreactor: effects of elevated CO2 concentration on growth and photosynthetic performance. Bot. Mar. 62, 179–190.
Shapiro, S. S., and Wilk, M. B. (1965). An analysis of variance test for normality (complete samples). Biometrika 52, 591–611.
Steinhagen, S., Enge, S., Larsson, K., Olsson, J., Nylund, G. M., Albers, E., et al. (2021). Sustainable large-scale aquaculture of the northern hemisphere sea lettuce, Ulva fenestrata, in an off-shore seafarm. J. Mar. Sci. Eng. 9:615.
Steinhagen, S., Karez, R., and Weinberger, F. (2019). Cryptic, alien and lost species: molecular diversity of Ulva sensu lato along the German coasts of the North and Baltic Seas. Eur. J. Phycol. 54, 466–483. doi: 10.1080/09670262.2019.1597925
Stévant, P., Rebours, C., and Chapman, A. (2017). Seaweed aquaculture in Norway: recent industrial developments and future perspectives. Aquacult. Int. 25, 1373–1390.
Swedish Food Agency (2017). Market Basket 2015 – Chemical Analysis, Exposure Estimation and Health-Related Assessment of Nutrients and Toxic Compounds in Swedish Food Basket, Swedish National Food Agency Report: 26/2017. ISSN 1104-7089. Uppsala: Swedish National Food Agency.
Titlyanov, E. A., and Titlyanova, T. V. (2010). Seaweed cultivation: methods and problems. Russ. J. Mar. Biol. 36, 227–242. doi: 10.1134/s1063074010040012
Toth, G. B., Harrysson, H., Wahlström, N., Olsson, J., Oerbekke, A., Steinhagen, S., et al. (2020). Effects of irradiance, temperature, nutrients, and pCO2 on the growth and biochemical composition of cultivated Ulva fenestrata. J. Appl. Phycol. 32, 3243–3254. doi: 10.1007/s10811-020-02155-8
Trigo, J. P., Engström, N., Steinhagen, S., Juul, L., Harrysson, H., Toth, G. B., et al. (2021). In vitro digestibility and Caco-2 cell bioavailability of sea lettuce (Ulva fenestrata) proteins extracted using pH-shift processing. Food Chem. 356:129683. doi: 10.1016/j.foodchem.2021.129683
UN General Assembly (2015). Sustainable Development Goals. SDGs Transform Our World, 2030. New York, NY: UN General Assembly.
Underwood, A. J. (1981). Techniques of analysis of variance in experimental marine biology and ecology. Oceanogr. Mar. Biol. Annu. Rev. 19, 513–603.
van den Burg, S. W. K., Stuiver, M., Veenstra, F. A., Bikker, P., Lopez Contreras, A. M., Palstra, A. P., et al. (2013). A Triple P Review of the Feasibility of Sustainable Offshore Seaweed Production in the North Sea. Wageningen: Wageningen University & Research.
van der Spiegel, M., Noordam, M. Y., and van der Fels-Klerx, H. J. (2013). Safety of novel protein sources (insects, microalgae, sea-weed, duckweed, and rapeseed) and legislative aspects for their application in food and feed production. Compr. Rev. Food Sci. Food Saf. 12, 662–678. doi: 10.1111/1541-4337.12032
Van Krimpen, M. M., Bikker, P., Van der Meer, I. M., Van der Peet-Schwering, C. M. C., and Vereijken, J. M. (2013). Cultivation, Processing and Nutritional Aspects for Pigs and Poultry of European Protein Sources as Alternatives for Imported Soybean Products (No. 662). Wageningen: University & Research Livestock Research.
Visch, W., Nylund, G., and Pavia, H. (2020). Growth and biofouling in kelp aquaculture (Saccharina latissima): the effect of location and wave exposure. J. Appl. Phycol. 32, 3199–3209.
Wahlström, N., Steinhagen, S., Toth, G., Pavia, H., and Edlund, U. (2020a). Ulvan dialdehyde-gelatine hydrogels for removal of heavy metals and methylene blue from aqueous solution. Carbohydr. Polym. 249:116841. doi: 10.1016/j.carbpol.2020.116841
Wahlström, N., Edlund, U., Pavia, H., Toth, G., Jaworski, A., Pell, A. J., et al. (2020b). Cellulose from the green macroalgae Ulva lactuca: isolation, characterization, optotracing, and production of cellulose nanofibrils. Cellulose 27, 3707–3725. doi: 10.1007/s10570-020-03029-5
Keywords: aquaculture, biochemical composition, biomass yield, biofouling, protein, seasonal variation, seaweed, Ulva fenestrata
Citation: Steinhagen S, Enge S, Cervin G, Larsson K, Edlund U, Schmidt AEM, Wahlström N, Kollander B, Pavia H, Undeland I and Toth GB (2022) Harvest Time Can Affect the Optimal Yield and Quality of Sea Lettuce (Ulva fenestrata) in a Sustainable Sea-Based Cultivation. Front. Mar. Sci. 9:816890. doi: 10.3389/fmars.2022.816890
Received: 17 November 2021; Accepted: 27 January 2022;
Published: 25 February 2022.
Edited by:
Cataldo Pierri, University of Bari Aldo Moro, ItalyReviewed by:
Antonella Petrocelli, National Research Council (CNR), ItalyGuang Gao, Xiamen University, China
Copyright © 2022 Steinhagen, Enge, Cervin, Larsson, Edlund, Schmidt, Wahlström, Kollander, Pavia, Undeland and Toth. This is an open-access article distributed under the terms of the Creative Commons Attribution License (CC BY). The use, distribution or reproduction in other forums is permitted, provided the original author(s) and the copyright owner(s) are credited and that the original publication in this journal is cited, in accordance with accepted academic practice. No use, distribution or reproduction is permitted which does not comply with these terms.
*Correspondence: Sophie Steinhagen, c29waGllLnN0ZWluaGFnZW5AZ3Uuc2U=
†ORCID: Sophie Steinhagen, orcid.org/0000-0001-8410-9932; Swantje Enge, orcid.org/0000-0003-4292-0051; Gunnar Cervin, orcid.org/0000-0002-2240-6525; Karin Larsson, orcid.org/0000-0002-4265-9044; Ulrica Edlund, orcid.org/0000-0002-1631-1781; Alina E. M. Schmidt, orcid.org/0000-0002-0492-0395; Niklas Wahlström, orcid.org/0000-0003-1986-1516; Barbro Kollander, orcid.org/0000-0003-2004-2323; Henrik Pavia, orcid.org/0000-0001-7834-6026; Ingrid Undeland, orcid.org/0000-0002-9732-3644; Gunilla B. Toth, orcid.org/0000-0002-1225-7773