- Instituto de Oceanografía y Cambio Global, IOCAG, Universidad de Las Palmas de Gran Canaria, Las Palmas, Spain
Ocean acidification impacts the iron (Fe) biogeochemistry both by its redox and its complexation reactions. This has a direct effect on the ecosystems due to Fe being an essential micronutrient. Polyphenols exudated by marine microorganisms can complex Fe(III), modifying the Fe(II) oxidation rates as well as promoting the reduction of Fe(III) to Fe(II) in seawater. The effect of the polyphenol gallic acid (GA; 3,4,5-trihydroxy benzoic acid) on the oxidation and reduction of Fe was studied. The Fe(II) oxidation rate constant decreased, increasing the permanence of Fe(II) in solutions at nM levels. At pH = 8.0 and in the absence of gallic acid, 69.3% of the initial Fe(II) was oxidized after 10 min. With 100 nM of gallic acid (ratio 4:1 GA:Fe), and after 30 min, 37.5% of the initial Fe(II) was oxidized. Fe(III) is reduced to Fe(II) by gallic acid in a process that depends on the pH and composition of solution, being faster as pH decreases. At pH > 7.00, the Fe(III) reduction rate constant in seawater was lower than in NaCl solutions, being the difference at pH 8.0 of 1.577 × 10–5 s–1. Moreover, the change of the Fe(III) rate constant with pH, within the studied range, was higher in seawater (slope = 0.91) than in NaCl solutions (slope = 0.46). The Fe(III) reduction rate constant increased with increasing ligand concentration, being the effect higher at pH 7.0 [k′ = 1.078 × 10–4 s–1; (GA) = 250 nM] compared with that at pH 8.0 [k′ = 3.407 × 10–5 s–1; (GA) = 250 nM]. Accordingly, gallic acid reduces Fe(III) to Fe(II) in seawater, making possible the presence of Fe(II) for longer periods and favoring its bioavailability.
Highlights
Gallic acid promotes the permanence of Fe(II) in seawater.
Introduction
The increase in atmospheric carbon dioxide (CO2) by human activity and its uptake by seawater has resulted in substantive changes in ocean chemistry over the last few decades (Orr et al., 2005; Bates et al., 2014). Ocean acidification results from the decrease in ocean pH and calcium carbonate saturation state (Caldeira and Wickett, 2003) and future projections of CO2 chemistry (Orr et al., 2005), raise potential consequences for marine organisms, ecosystems, and chemical properties (Royal Society, 2005; Gattuso and Hansson, 2011; Bates, 2017). The organic and inorganic speciation of trace metal chemistry is affected by ocean acidification (Millero et al., 2009; Shi et al., 2010; Gledhill et al., 2015; Ivanina and Sokolova, 2015; Avendaño et al., 2016). According to the present evolution of pH in the ocean (Orr et al., 2005; Santana-Casiano et al., 2007; Byrne et al., 2010), it will suffer intense changes out of those in the last 20 million years (Caldeira and Wickett, 2003; Raven et al., 2005). The pH of surface seawater may decrease from its current value of 8.1–7.8 by the end of the 21st century (IPCC, 2014, 2021). Decreasing the pH in the oceans increases the residence time of the reduced form of essential trace metals, such as Fe and Cu, by decreasing their oxidation rate (Millero, 1996). In addition, ocean acidification could change the type and amount of dissolved organic material released by phytoplankton (Spilling et al., 2016), as seawater pH/pCO2 conditions regulate inorganic carbon concentration mechanisms and photosynthetic carbon fixation rates in autotrophic microorganisms (Riebesell et al., 2007).
Iron is an essential micronutrient required for important physiological processes in phytoplankton, such as nutrient assimilation and electron transport during respiration and photosynthesis (Geider and La Roche, 1994; Morel and Price, 2003; Behrenfeld and Milligan, 2013). In the marine environment, Fe(III) is the predominant form which is highly insoluble (Liu and Millero, 2002), however, the presence of Fe-binding ligands dominates its speciation (99% of Fe is organically bound) and increases its solubility (Rue and Bruland, 1995; van den Berg, 1995; Boye et al., 2010; Caprara et al., 2016). Therefore, these organic ligands play a key role in Fe biogeochemical cycles, controlling its speciation, solubility, and mobility in seawater and determining Fe bioavailability to organisms (Liu and Millero, 2002; Croot et al., 2011; Croot and Heller, 2012; Shaked and Lis, 2012).
Numerous studies have reported that, in seawater, the redox chemistry of inorganic Fe is intimately linked to the pH (Moffett and Zika, 1987; Miller et al., 1995; King and Farlow, 2000; González-Dávila et al., 2006; González et al., 2016; Pérez-Almeida et al., 2019). When the pH decreases the speciation of inorganic Fe(III) changes, increasing the solubility of Fe oxyhydroxides, thus also affecting their reactivity. In addition, a change in pH also affects the oxidation rate constants of Fe(II), which decreases with decreasing pH. The redox chemistry of Fe is also affected by the presence of organic ligands in seawater (Santana-Casiano et al., 2000, 2010, 2014; Rose and Waite, 2002, 2003; Craig et al., 2009; Roy and Wells, 2011; Gonzaìlez et al., 2012; González et al., 2014; Lee et al., 2017; Samperio-Ramos et al., 2018; Arreguin et al., 2021). On other hand, recent investigations studied the effect of ocean acidification on the organic processes where iron is involved (Gledhill et al., 2015; Avendaño et al., 2016).
However, there is a lack of information on the functional groups that form the ligand pool in the ocean and their role in Fe chemistry. The concentration of dissolved organic compounds (DOC) in the ocean ranges from nM to μM levels (Benner et al., 1992; Hopkinson and Vallino, 2005; Hansell and Carlson, 2014). Organic matter in the ocean consists of a pool of individual compounds. Among all possible organic compounds, polyphenols are relevant because they form complexes with Fe, by forming strong or weak bonds, modifying its chemical speciation and bioavailability (Brown et al., 1998; Witter et al., 2000; Lodovici et al., 2001; Mira et al., 2002; Sroka and Cisowski, 2003; Andjelković et al., 2006; Santana-Casiano et al., 2010, 2014; González et al., 2019; Arreguin et al., 2021). Polyphenols can reach the ocean via terrestrial supply and can be considered as model ligands within the group of humic substances (Krachler et al., 2005, 2010, 2012, 2015, 2016, 2019; Blazevic et al., 2016; Orlowska et al., 2016, 2017a,b; Rathgeb et al., 2017). In the ocean, they are excreted by marine diatoms and green algae, particularly under metallic stress conditions (Rico et al., 2013; López et al., 2015). Microalgae cultures are capable of producing polyphenols with values of 43 nM for a total of 18 individual compounds (Rico et al., 2013; López et al., 2015). In addition, they are strongly involved in the transport of dissolved Fe from terrestrial to aquatic systems (Wan et al., 2018).
Polyphenols react with oxygen (De et al., 2018) and reactive oxygen species such as superoxide and hydroxyl radicals (Joshi et al., 2012; Velika and Kron, 2012), acting as free radical scavengers. Numerous phenolic compounds can form stable compounds with dissolved Fe(III) (Moran et al., 1997; González et al., 2019; Arreguin et al., 2021) and Fe(II) (Andjelković et al., 2006). The Fe(III)-phenol compounds formed can be reduced to a Fe(II) complex (Strlič et al., 2002; Santana-Casiano et al., 2010, 2014; Arreguin et al., 2021). Conversely, Fe(II)-phenol complex can be also oxidized to Fe(III) complex (Santana-Casiano et al., 2000, 2014). Interactions between natural organic matter, including phenolic compounds, and Fe involve varied and, in many cases, complex chemistry.
Among the diversity of polyphenols, Gallic acid (GA; 3,4,5-trihydroxy benzoic acid), (GA, Figure 1A), is a low molecular weight triphenolic compound with strong antioxidant activity (Khan et al., 2000; Zheng and Wang, 2001; Kim et al., 2002; Schlesier et al., 2002; Abdelwahed et al., 2007; Priscilla and Prince, 2009; Giftson et al., 2010). GA is a planar molecule containing an aromatic ring, three hydroxyl groups, and a carboxylic group (Badhani et al., 2015). The three groups are bonded to the aromatic ring in an ortho position relative to each other. Four stable conformers have been described for GA depending on the orientation of the hydroxyl group (Figure 1B). GA(I) is the most stable conformer and has the position of the hydrogen atoms in the same orientation (Cappelli et al., 2005; Pardeshi et al., 2013). Gallic acid can form both intra- (between hydroxyl groups) and intermolecular hydrogen bonds (Pardeshi et al., 2013). In this regard, GA(I), GA(II), GA(III), and GA(IV) (Figure 1B) can have two, one, one, and two intramolecular hydrogen bonds, respectively.
Both the antioxidant capacity and antiradical activity of polyphenols increase with the number of OH– groups attached to the aromatic ring (Galato et al., 2001; Sroka and Cisowski, 2003; Palafox-Carlos et al., 2012). Therefore, GA exhibits high oxidative capacity compared to most polyphenols (Palafox-Carlos et al., 2012). On the other hand, the high complexation ability of Fe with polyphenols has been related to the position of the OH– group in the benzene ring, forming Fe-phenol complexes, those polyphenols having at least two OH– groups in consecutive positions (Wu et al., 2016). In this sense, gallic acid forms very stable complexes with Fe(III), in different media (Li et al., 2000; Masoud et al., 2014; González et al., 2019; Luther et al., 2021) and with Fe(II), increasing its permanence and bioavailability in the medium (Wu et al., 2016). In the pH range 1–3, the formation of the Fe(III)-gallic acid complex leads to the oxidation of gallic acid to hydroxyquinone with a simultaneous reduction of Fe(III) to Fe(II) (Hynes and O’Coinceanainn, 2001). Moreover, Wu et al. (2016) and, previously, Andjelković et al. (2006) showed, by UV-Vis spectra, that Fe(II)-GA solutions presented absorption peaks in the UV region, indicating the chelation abilities of gallic acid, where the presence of the third hydroxyl group could stabilize the electronic delocalization process within the benzene ring.
Given the importance that ocean acidification can have on Fe biogeochemistry and that the presence of organic matter, including polyphenols, is a major factor in the mechanisms affecting Fe bioavailability in marine ecosystems, we have investigated the role of gallic acid in the oxidation and reduction of Fe in seawater, as well as the different factors that can affect these redox processes. Changes in temperature of the process, that affect the oxidation process (Santana-Casiano et al., 2005), were not considered. To this end, the role of gallic acid on the redox chemistry of Fe in NaCl and seawater solutions has been studied as a function of pH (7.0–8.2), organic ligand concentration (0–500 nM), and the presence of Ca2+ and Mg2+ as major ions in seawater.
Experimental
Chemical
Fe(II) stock solution (10–4 M) was prepared from ammonium iron (II) sulfate hexahydrate (Sigma) in 0.7 M NaCl acidified to pH 2 with supra pure HCl. Gallic acid (GA, 3 × 10–4 M) stock (Sigma-Aldrich) was prepared weekly in HPLC grade methanol. The second stock of 10–4 M GA in an aqueous solution was prepared before each experiment. Experiments in NaCl were carried out in 0.7 M NaCl with 2 mM NaHCO3, to reach average seawater ionic strength. The experiments on the effect exerted by the major seawater ions (Millero, 1996) were carried out in final ionic strength of 0.7 M, keeping the amount of NaHCO3 constant (2 mM). All aqueous solutions were prepared with ultra-pure water (UPW, 18 MΩ) and the reagents used were of analytical grade.
The seawater used in all the experiments was collected from the ESTOC Time Series station (29° 10′ N 15° 30′ W), an oligotrophic area situated in the North Atlantic subtropical gyre, using trace metal clean Teflon pump (PFD2 316F, AstiPure®) and in line filtered by 0.2 μm dual pore-size trace metal clean filters (AcropackTM) and kept in darkness to remove any reactive oxygen species (ROS) present in the seawater.
Oxidation and Reduction Experiments
Fe oxidation and reduction kinetic experiments were performed in a 200 mL thermostated flask. The temperature was set at 25 ± 0.02 °C using a thermostatic bath (AG-2TM, Julabo™). The pH was measured on the free scale (Millero, 1986) and was fixed and monitored throughout each experiment (with a variation interval of ± 0.02 pH units) with small additions of 0.1 M HCl using an automatic titration system (Titrino plus 848 Metrohm™). Samples were bubbled with pure air before and during each experiment and stirred with a Teflon magnetic stirrer. The initial iron concentration was always set to 25 nM.
Determination of Fe(II)
The Fe(II) concentration was determined spectrophotometrically using the modified ferrozine method (Viollier et al., 2000; Santana-Casiano et al., 2005) and the same experimental details that previous studies (Santana-Casiano et al., 2010, 2014; Arreguin et al., 2021). A 200 mL reaction vessel is connected to a 5 m long capillary column (World Precision Instruments™) by using a peristaltic pump (EXPETEC Perimax 12) with a flux of 1 mL min–1. The S4000 UV detector (Ocean Optics) is connected to the column with a 400 μm fiber optics and the lamp (HL-2000-FHSA from Mikropack).
Each experiment is always done under darkness and in continuous flux to avoid photoreactions, where the solution passes through the capillary column and goes to waste. The detection limit of this technique was 0.1 nM at pH 8.0–0.2 nM at pH 7.0, with a limit of quantification that ranged from 0.3 nM to 0.7 nM at pH 8.0 and 7.0, respectively (Santana-Casiano et al., 2010, 2014; González et al., 2019; Arreguin et al., 2021). It has been computed by the preparation of calibration plots at different pH from 7 to 8 and a range of Fe(II) from 0 to 100 nM. The accuracy of the Fe(II) determination was carried out by triplicate and the Fe(II) measured against a standard solution freshly prepared was always less than 6% (in triplicate). The linearity of the system was over two orders of magnitude Fe(II) (González-Dávila et al., 2005; Santana-Casiano et al., 2005).
Fe(II) Oxidation Experiments
These studies were performed in artificial seawater (ASW) (Millero, 1996) by using trace metal grade reagents. An amount of 200 mL of ASW was introduced into the reaction flask and purged for 1 h with pure air. The solution was then brought to the desired pH, GA was added, and the blank was obtained. Fe(II) blank levels were always below the detection limit. Next, 50 μL of the Fe(II) stock solution (10–4 M) was added (t = 0 of the reaction time). At set periods, 10 mL aliquots of the reaction flask were added to a glass flask containing 50 μL of 0.01 M ferrozine, 2 mL of acetate buffer at pH 5.5 and 50 μL of NaF 7.1 × 10–4 M (to avoid Fe(III) interference), proceeding to the Fe(II) determination (Viollier et al., 2000; Santana-Casiano et al., 2005). Each data is the result of at least two replicates.
Fe(III) Reduction Experiments
Following the method employed in previous studies (Santana-Casiano et al., 2010, 2014; Arreguin et al., 2021), 25 μl of the Fe(II) stock solution (10–4 M) were added to 100 ml of the solution (seawater or NaCl with ions) contained in the reaction flask and bubbled with pure air for 1 h. In the case of natural seawater and under such conditions, Fe(II) is totally oxidized to Fe(III) where the equilibrium for the hydrolysis of Fe(III) species and possible Fe-binding ligands was achieved. Ferrozine (FZ) was then added; the pH was adjusted to the desired value and a blank was obtained. The addition of the GA aliquot corresponded to t = 0 of the reaction time. The Fe(II)-FZ3 complex formed was followed continuously and the measured solution, once it passes through the capillary column, does not return to the reaction flask. The detection limit of this technique was 0.1 nM at pH 8.0 and 0.2 nM at pH 7.0 (Arreguin et al., 2021), as has been mentioned above. Fe(II) was not detected before GA addition. In the presence of GA, no interference with the Fe(II)-FZ3 peak was observed. Under these conditions, the amount of Fe(III) present in the solution is given by, [Fe(III)]t = [Fe(III)]0 - [Fe(II)]t (subscripts 0 and t indicate, respectively, initial and time Fe(III) concentration; Santana-Casiano et al., 2010).
For all the experiments in both NaCl and SW solutions using GA, the plot of ln [Fe(III)] vs. time gave a straight line (R2 values greater than 0.97). From Equation 1, the pseudo-first-order constant (k′, min–1) is obtained and the apparent reduction rate constant (kapp) is calculated from k′ and the GA concentration.
where k′ = kapp[GA].
Results
Oxidation of Fe(II) in the Presence of Gallic Acid
The oxidation experiments were carried with an initial concentration of Fe(II) of 25 nM and with a concentration of GA from 0 to 100 nM and at fixed temperature and pH of 25°C and 8.0, respectively, in ASW. The results obtained are shown in Figure 2 where it is observed that the presence of GA decreased the Fe(II) oxidation rate constants.
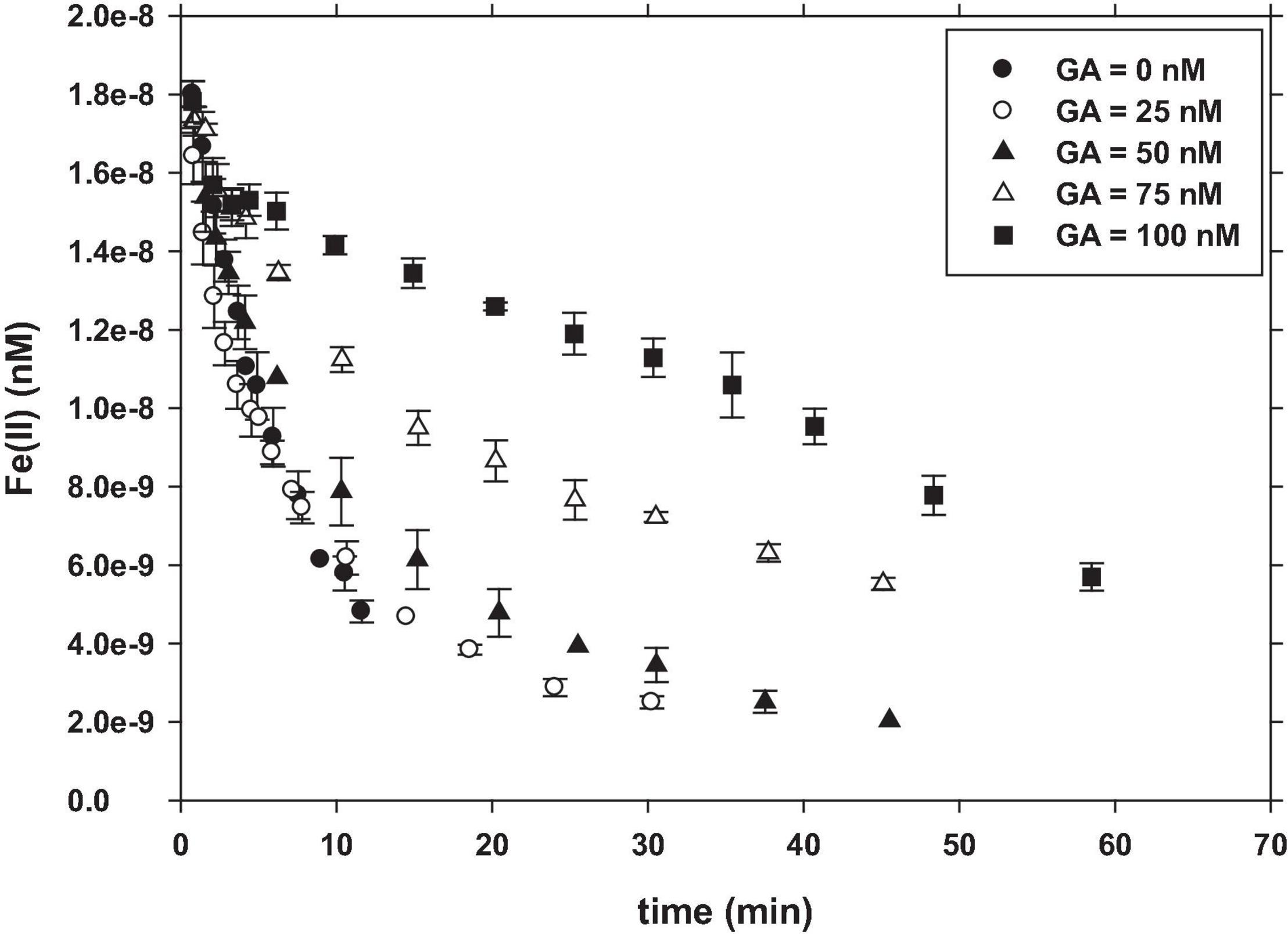
Figure 2. Oxidation of Fe(II) in the presence of GA. Experimental conditions were: [Fe(II)]0 = 25 nM; [GA] = 0–100 nM; pH = 8.0; T = 25°C.
Figure 2 shows that the concentration of Fe(II) remains longer in solution when the GA concentration increases at pH 8. The initial concentration of Fe(II) (25 nM) decreased until 5 nM after 11.6 min of reaction when GA is not present in the solution. However, the addition of GA produced a slowdown of the reaction time, reaching 5 nM after 13.6 min (25 nM GA) and 16.4 min (50 nM GA), while in the presence of 75 and 100 nM GA (GA:Fe > 3), Fe(II) did not reach 5 nM after the maximum period of study of 45 min and 59 min, respectively (Figure 2).
Analysis of the Spectra to Calculate the Fe(III) Reduction by Gallic Acid
To properly compute the Fe(II)-FZ3 peak, the analysis of the spectra should be done. The evolution of the spectra of GA with the time in NaCl and SW solutions in oxygen saturated conditions at different pH (7.0 and 8.0) and concentration of GA (100 and 500 nM) (Figure 3), was initially considered. The peak observed at wavelengths near 400 nm corresponds to the presence of the benzoquinone (Hynes and O’Coinceanainn, 2001; Santana-Casiano et al., 2010; Masoud et al., 2014) and the peak/shoulder above 600 nm corresponds to the semiquinone radical (Slawiñska et al., 2007). The Fe(II)-FZ3 peak at 562 nm was determined without interferences (Figure 4). At the experimental conditions considered in this current investigation (low Fe and GA levels), the previously reported peak at 690 nm for Fe(III) and Gallic acid (Dong et al., 2016) was not observed. This difference was also reported for Fe(III) and Gentisic acid at high and low levels (Porwal et al., 2015; Arreguin et al., 2021).
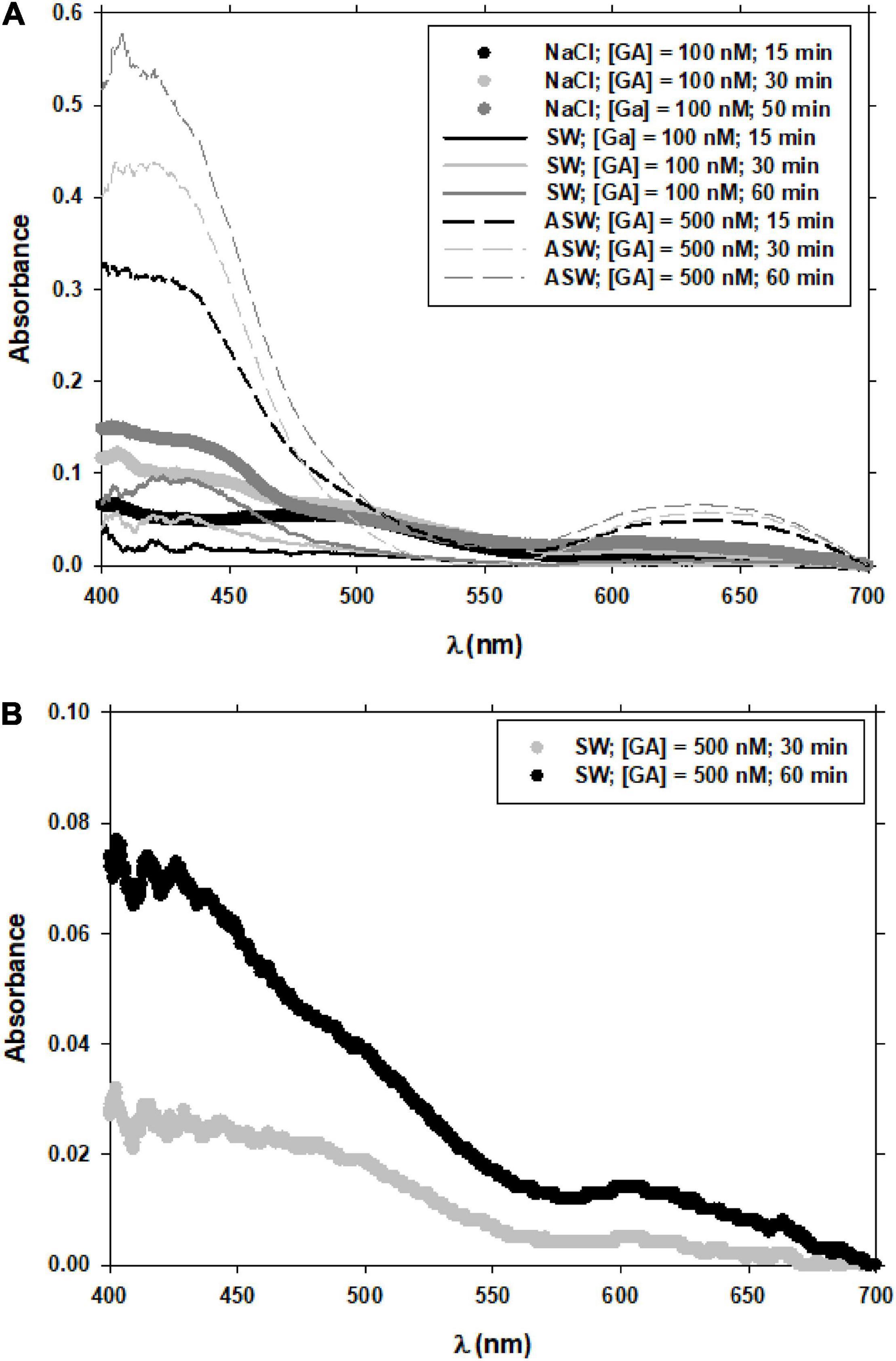
Figure 3. Spectra of GA at 100 and 500 nM in different reaction media. Experimental conditions were T = 25°C, pH = 8.0 (A). Spectra of GA at 500 nM at different times and in seawater. Experimental conditions were T = 25°C, pH = 7.0 (B).
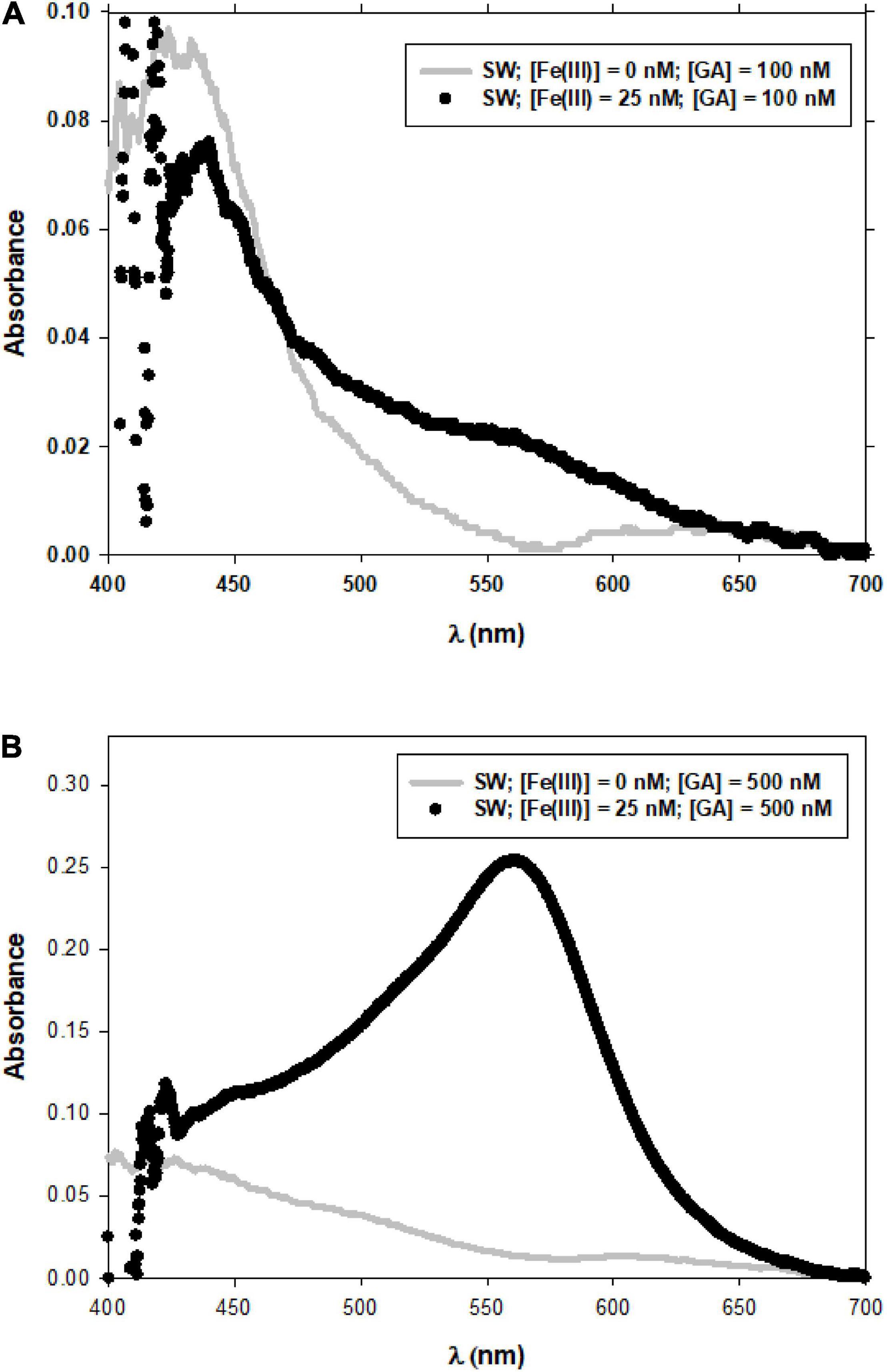
Figure 4. Spectra of GA at 100 nM and of the Fe(II)-FZ3 complex at 25 nM Fe. Experimental conditions were T = 25°C, pH = 8.0 (A). Spectra of GA at 500 nM and of the Fe(II)-FZ3 complex at 25 nM Fe. Experimental conditions were T = 25°C, pH = 7.0 (B).
The Effect of pH on the Reduction of Fe(III) in the Presence of Gallic Acid
In the presence of GA in both NaCl and SW solutions, Fe(III) was reduced to Fe(II) (Table 1). According to González et al. (2019), Fe(III) is organically complexed with GA increasing its solubility (Liu and Millero, 2002). In addition, the current experiments demonstrate that the GA-Fe(III) complex is also electron-transfer reduced to Fe(II). Then, the complex of Fe(III)-GA favors the Fe(II) regeneration in seawater. The Fe(III) reduction to Fe(II) in the presence of GA was studied at different pH (7.0–8.2) in NaCl and SW solutions (Figure 5) with [GA]0 = 100 nM, [Fe(III)]0 = 25 nM and T = 25°C. The Fe(III) reduction to Fe(II) was a pH-dependent process where the rate constant linearly decreased with increasing pH in both NaCl and SW solutions (R2 greater than 0.98 and a standard error of estimate of 0.029 in NaCl and 0.10 in SW, Equations 2, 3).
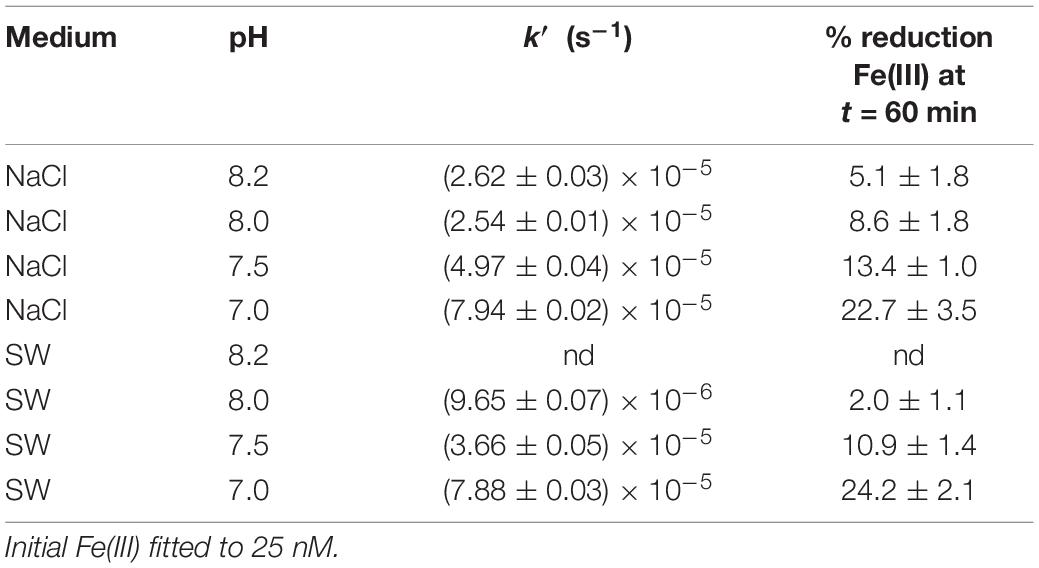
Table 1. Fe(III) reduction rate constants in the presence of GA 100 nM at T = 25°C, different pH and in NaCl and seawater media.
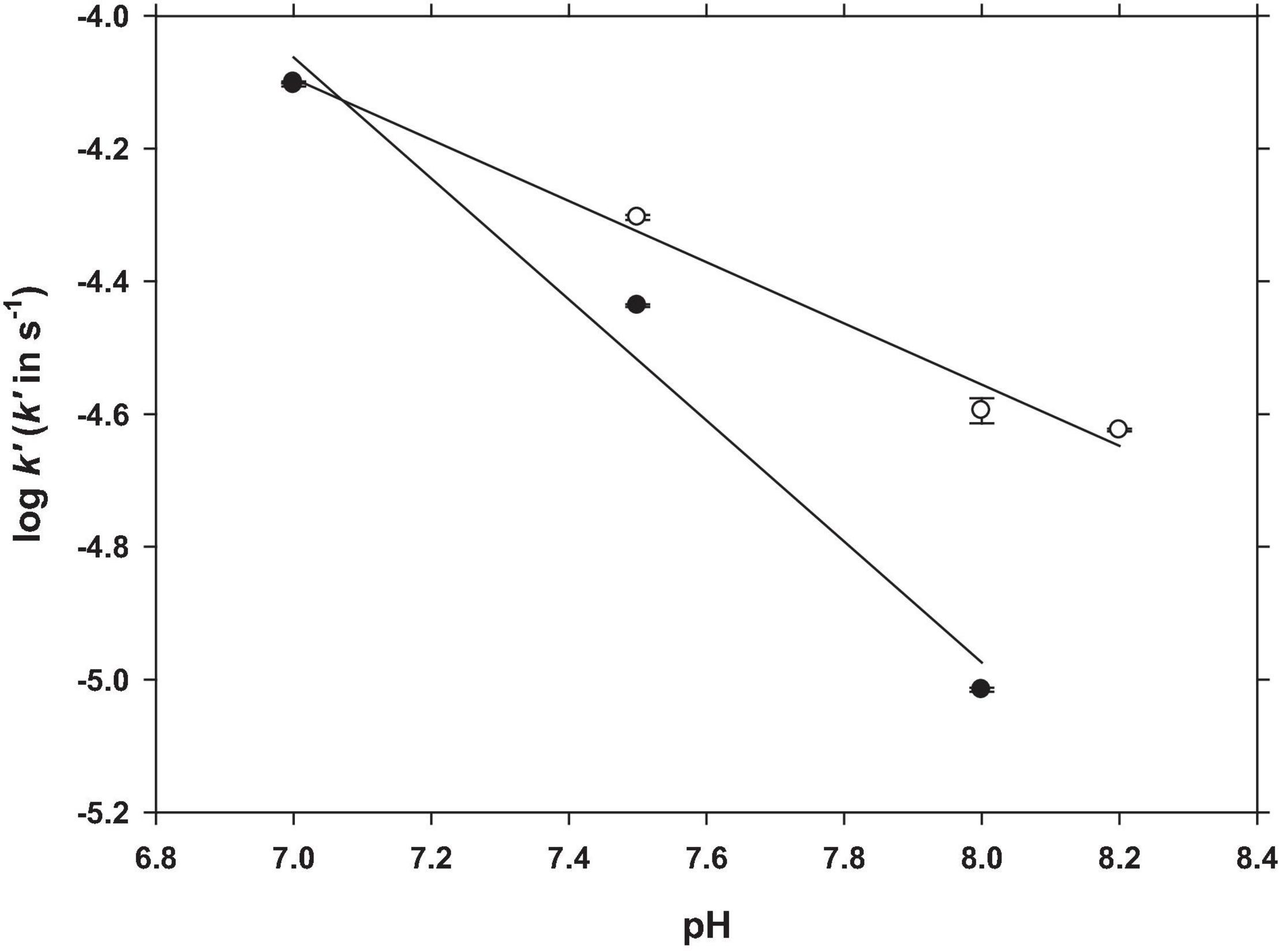
Figure 5. pH dependence of the Fe(III) reduction rate constant in SW (filled symbols) and NaCl-NaHCO3 (unfilled symbols). Experimental conditions were: [Fe(III)]0 = 25 nM; [GA] = 100 nM; T = 25°C.
At pH 7.0, the reduction rate constant was similar in both solutions (7.94 × 10–5 s–1 in NaCl and 7.88 × 10–5 s–1 in SW) and at higher pH, the constants decreased at different rates. In seawater, the slope was close to 1 and twice that determined in NaCl. The Fe(III) reduction percentages after 60 min of reaction are shown in Table 1. At pH 8.2, the amount of Fe reduced in NaCl was 5.1% of the initial {[Fe(III)0] = 25 nM}, increasing to 22.7% at pH 7.0. In seawater, the Fe(III) reduction was quantified until pH 8.0, because at higher pH the peak was not measurable. In SW at pH 8.0, the percentage of Fe(III) reduction was 2.0% of the initial one, increasing to 24.2% at pH 7.0.
The different slope showed by the reduction of Fe(III) to Fe(II) in NaCl and SW solutions (Equations 2, 3) indicated that major ions in seawater affect the process. Two of the main major ions present in seawater, Ca2+ and Mg2+, were studied at pH 7.0 and 8.0 (Figure 6) with [GA]0 = 100 nM and T = 25°C.
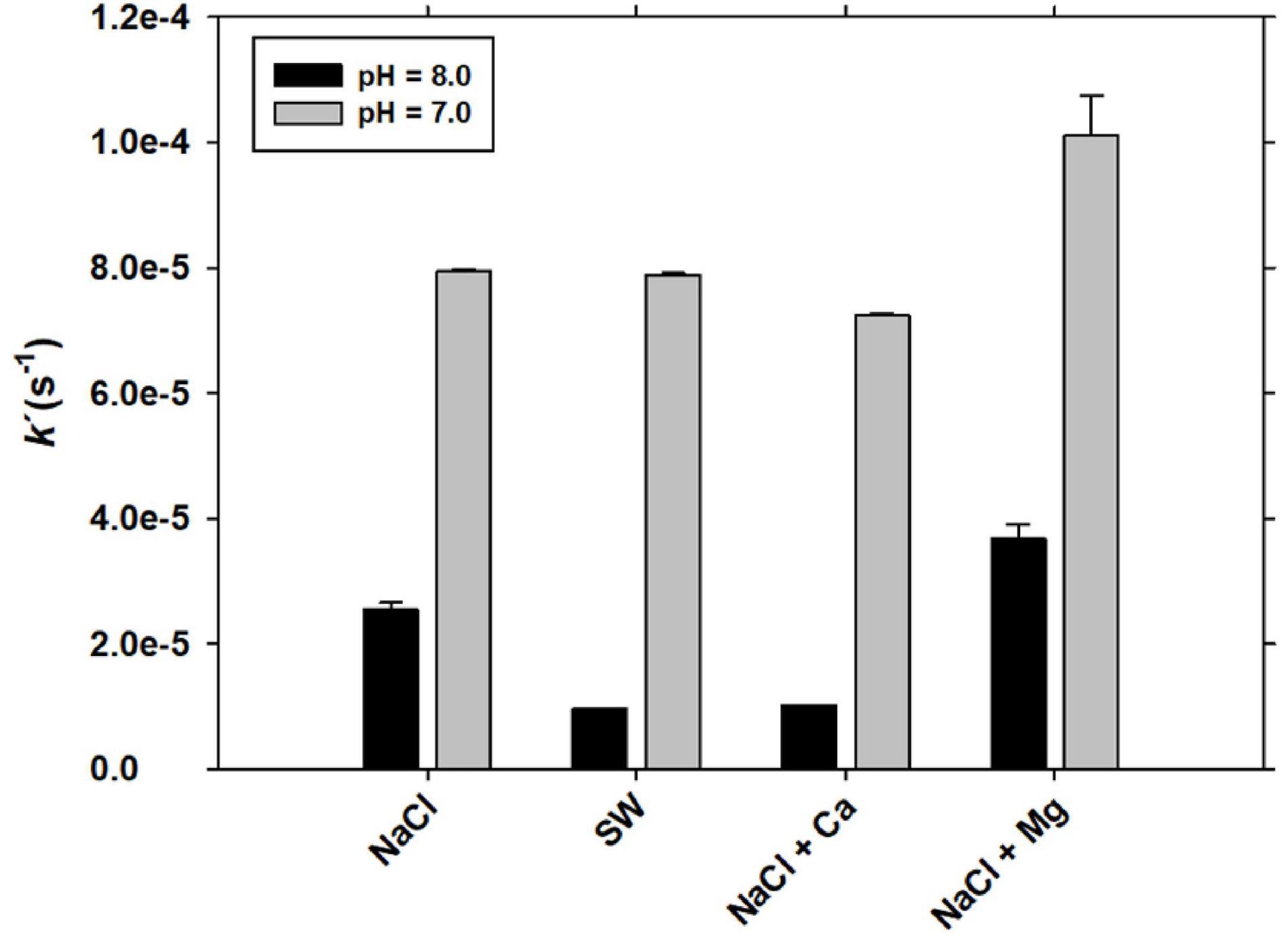
Figure 6. The Fe(III) reduction rate constant in the presence of 100 nM of GA at T = 25°C in NaCl (+2 mM NaHCO3), seawater and NaCl with Ca2+ and Mg2+.
At both pH 7.0 and pH 8.0, the Fe(III) reduction rate constants increased in the presence of NaCl-Mg2+. When it was compared with the Fe(III) reduction rate constant in SW, the presence of Ca2+ provides Fe(III) reduction rate constants similar to those in SW at both pH values. At pH 7.0, the percentage of Fe(III) that is reduced after 60 min reached 30 ± 3% in NaCl-Mg2+, 24 ± 4% in SW, 23 ± 3% in NaCl and 21 ± 3% in NaCl-Ca2+, decreasing to 9 ± 3%, 2 ± 1%, 7 ± 2% and 3 ± 1%, respectively, at pH 8.0.
The Fe(III) Reduction at Different Gallic Acid Concentrations
The Fe(III) reduction at different GA concentrations (50–500 nM) was performed in SW at pH 7.0 and 8.0 (Figure 7). The relationship between Fe(III) reduction rate constant (k′, s−1) and GA concentration (nM) followed a second-degree polynomial equation (Equations 4, 5) with an R2 greater than 0.97 and an error of estimation of 1.9 × 10–5 at pH 7.0 and 3.5 × 10–6 at pH 8.0.
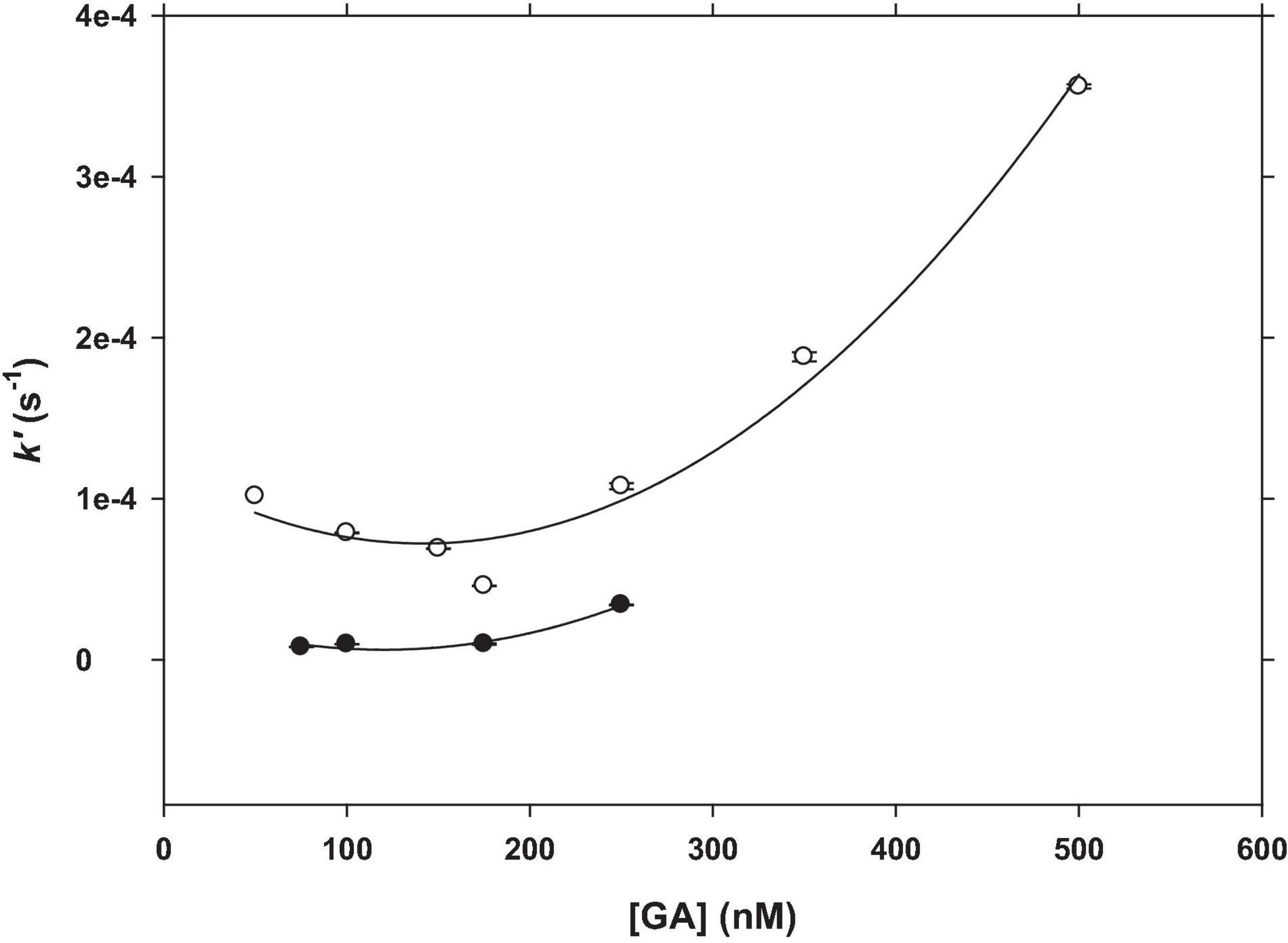
Figure 7. Dependence of the Fe(III) reduction rate constant on GA concentration at pH = 7.0 (unfilled symbols) and pH = 8.0 (filled symbols). The experimental conditions were [Fe(III)]0 = 25 nM; [GA] = 50–500 nM; T = 25°C.
The Fe(III) reduction rate constant increased with the GA concentration at both pH values. At pH 7.0 and between 50 and 250 nM of GA (GA:Fe(III) ratio from 2 to 10), the Fe(III) rate constant varied from 1.02 × 10–4 s–1 to 1.08 × 10–4 s–1 (1.4-fold). However, from 250 nM to 500 nM (ratio of 20), this variation on the Fe(III) rate was much pronounced, k′ = 3.56 × 10–4 s–1 at 500 nM of GA (3.3-fold). That is, 26.9% and 63% of Fe(III) were reduced to Fe(II) when added GA changed from 50 to 500 nM. At pH 8.0, the Fe(III) reduction rate is lower than that at pH 7.0. At pH 8, under 50 nM GA concentration, the Fe(II)-FZ3 peak was not detected, even after 80 min of reaction. The Fe(II)-FZ3 was only detected when GA was over a 3:1 GA:Fe(III) ratio, between 75 and 250 nM of GA. Above this GA concentration, it was not possible to detect Fe(II) because the Fe(II)-FZ3 peak was masked by the GA peaks observed at 400 nm and 600 nm (Figure 3A) that increased with the GA concentration. This interference at pH 7.0 (Figure 8) does not affect as the height of these peaks is lower than at pH 8.0 (Figure 3B).
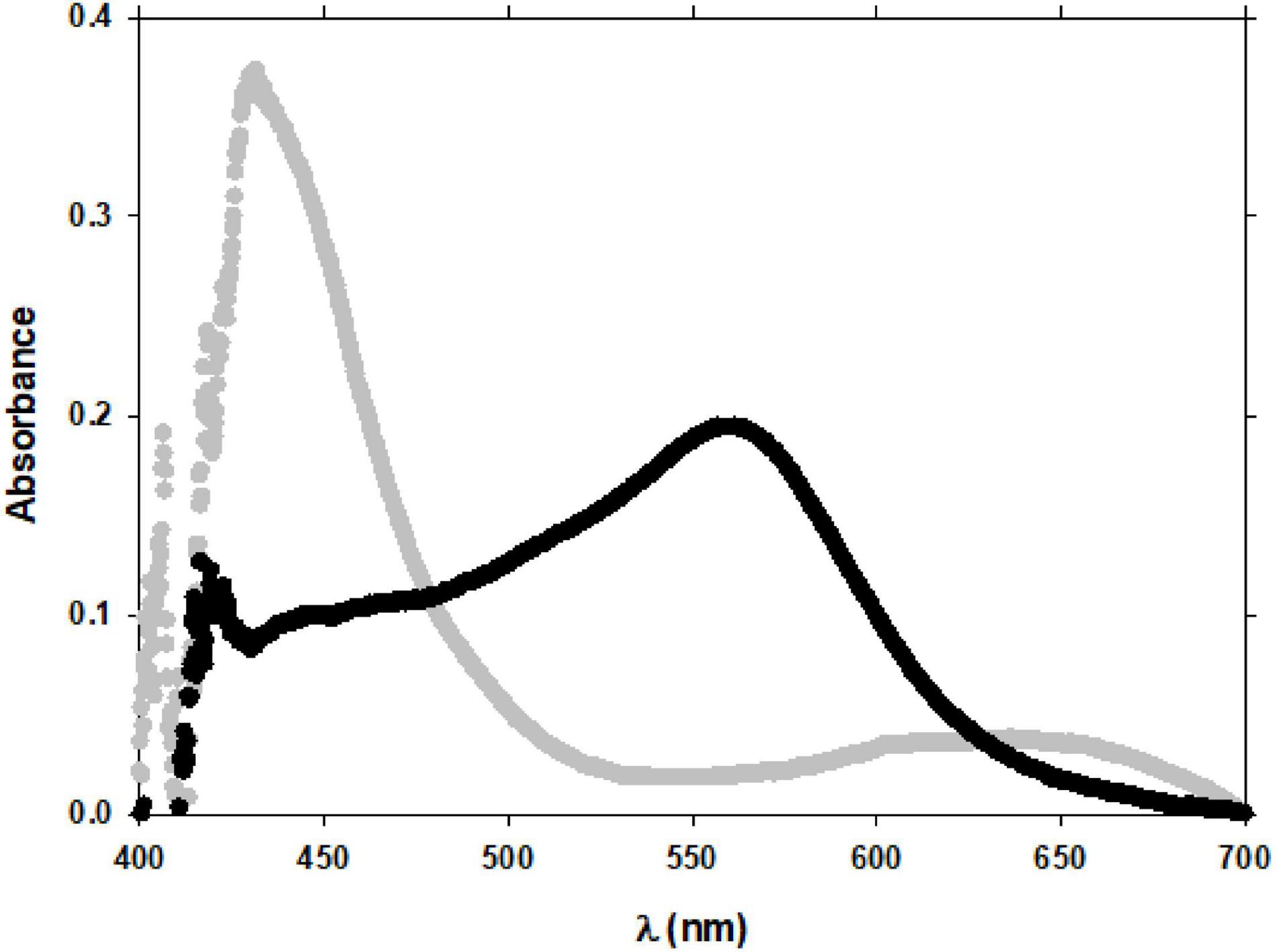
Figure 8. Spectrum of the Fe(II)-FZ3 peak in the presence of GA at pH = 7.0 (black) and 8.0 (gray). Experimental conditions were: [Fe(III)]0 = 25 nM; [GA] = 350 nM; T = 25°C.
Discussion
The presence of Gallic acid promoted the permanence of Fe(II) in seawater according to the current investigation, where the oxidation rate constant decreases as the GA concentration increases (Figure 2). The decrease in the Fe(II) oxidation rate constant has also been reported by natural exudates produced by phytoplankton species (Gonzaìlez et al., 2012; González et al., 2014; Santana-Casiano et al., 2014), humic substances (Ghosh et al., 1967; Theis and Singer, 1974). In this sense, other phenolic compounds such as catechin and sinapic acid, which are part of the organic compounds exuded by P. tricornutum, have been previously shown to decrease the oxidation rate constant of Fe(II), reducing Fe(III) to Fe(II) (Santana-Casiano et al., 2014). However, at different experimental conditions in deionized water at μM levels of Fe and pH lower than 7, catechol and galloyl groups have been shown to increase the Fe(II) oxidation because of their strong chelating ability toward ferric ion, even when catechol partially formed ferrous ions in the presence of ferrozine (Chvátalová et al., 2008; Perron et al., 2010). These same authors indicated that the presence of the third hydroxyl in the benzene ring made the oxidation less effective.
There are various mechanisms by which phenols can inhibit oxidation processes: metal complexation (Melidou et al., 2005; Zhao et al., 2005) or free radical scavenging (Hanasaki et al., 1994; Cos et al., 1998). By scavenging free radical donating one H atom (Equation 6) or acting as electron donor (Equation 7) (Wright et al., 2001) their propagation is interrupted by chain reactions, delaying the onset of the reaction, or slowing down the rate of the reaction.
where Ar is the aromatic ring. Galato et al. (2001) studied the antioxidant activity of various polyphenolic compounds and determined that this activity increased with increasing the number and position of OH groups bonded to the aromatic ring. In this sense, gallic and pyrogallol-derived polyphenols have a higher chelating capacity than catechol derivatives (Andjelković et al., 2006; Wan et al., 2018). The three hydroxyl groups are prone to oxidation generating hydrogen peroxide, quinones, and semiquinones (Rice-Evans et al., 1996; Pannala et al., 2001; Silva et al., 2002; Eslami et al., 2010; Severino et al., 2011).
Metal complexation by organic compounds affect the redox chemistry. In the case of phenols, and according to our results, the Fe(III) reduction and Fe(III) complexation are occurring (González et al., 2019). Neither all organic compounds nor all phenols possess these antioxidant and complexing capacities. In this sense, Wu et al. (2016) studied the interaction between Fe and ten phenols at pH = 8.0, finding that only three of them, caffeic acid, gallic acid, and protocatechuic acid showed protective effects on Fe(II), protecting 69, 64 and 33% of the initial Fe(II) content. This behavior was related to the chelating capacity of the catechol and galloyl groups with Fe(II), which provided high stability to the Fe(II)-GA complex which is oxidized to a lower rate. The latter agrees with the results of Andjelković et al. (2006). Due to the number and position of hydroxyl groups, phenols with galloyl group (3,4,5-trihydroxy) form more stable complexes with Fe(II) than those with catechol group (3,4-dihydroxy) (Rice-Evans et al., 1997; Wu et al., 2016).
However, complexes of Fe(II) with oxygen-containing ligands, such as -OH and carboxyl groups, are weak (Theis and Singer, 1974; Santana-Casiano et al., 2000, 2004; Rose and Waite, 2003), so that a decrease in the rate of Fe(II) oxidation due to the formation of a Fe(II)-GA complex could be contributing to the observed process but unlikely controlling the mechanism. A third mechanism can be considered: the complexation of Fe(III), formed both from Fe(III) in solution or from the oxidation of Fe(II), and the subsequent electron-transfer reduction of the Fe(III)-phenol complex through semiquinone and quinone derivatives (Santana-Casiano et al., 2014; González et al., 2019). Previous studies have reported that GA reduces Fe(III) to Fe(II) at experimental conditions different from those at this work and far from the typical conditions of oceanic environments. They were done in very acidic pH (between 1 and 3; Hynes and O’Coinceanainn, 2001) or higher GA and Fe(III) concentrations (Wan et al., 2018). Moreover, it should be considered that GA is also oxidized in the absence of any transition metal to semiquinone and quinone (Yoshioka et al., 1991; Guo et al., 1999; Oniki and Takahama, 2004), being the reaction to semiquinone radical slow except under strongly alkaline conditions (pH between 9 and 13; Ferreira-Severino et al., 2011). The electron transfer process could also imply the reduction of complexed Fe(III) in the solution. In this sense, Christoforidis et al. (2018) considering previous studies (Hynes and O’Coinceanainn, 2001; Dong et al., 2016; Pan et al., 2020) proposed a mechanism that involved the transformation of GA and Fe(III) in a Fenton system in which both Fe(III) is reduced to Fe(II) and the generated oxygen reactive species (H2O2, and the superoxide and hydroxyl radicals) from the reduction of GA and from the oxidation of Fe(II) react with the semiquinone and GA regenerating oxygen and producing GA and degradation products.
where Fe(III) represents any organic complex formed between Fe(III) and GA and other organic ligands in solution, a pH-dependent reaction that in a 1:1 complex Fe is bound to the adjacent hydroxyl groups of the GA molecule. Fe(II) represents also any inorganic and organic complex GA-Fe(II) that is oxidized to the corresponding Fe(III). SQ and BQ are the semiquinones and quinones formed from GA. According to our results, the oxidation of the GA-Fe(II) complex is slower than the oxidation of inorganic complexed Fe(II).
The different slope shown by Equations 2, 3 indicates that the Fe(III) to Fe(II) reduction process is pH-dependent, being greater in SW than in NaCl as observed for other phenolic compounds (Santana-Casiano et al., 2010, 2014; Arreguin et al., 2021). This indicates a major role of ionic interaction with organic ligands on the redox process. In the presence of GA, the amount of Fe(III) that is reduced is similar at pH = 7.0 in both media and increases with pH higher for NaCl solutions than for SW. This behavior is the opposite of that observed with gentisic acid (Arreguin et al., 2021) and catechol (Santana-Casiano et al., 2010). Moreover, the amount of Fe(III) being reduced is significantly higher with GA than with gentisic acid and catechol. In seawater, gentisic acid in a ratio Fe(III) : gentisic acid of 1 : 10, 4.3% of the initial Fe(III) was reduced at pH = 7.0, decreasing to 0.43% at pH = 8.0 (Arreguin et al., 2021). Under the same conditions, 24.2% of the initial Fe(III) was reduced by GA at pH = 7.0, decreasing to 2.0% at pH = 8.0. González et al. (2019) found that catechin, sinapic acid and gallic acid (in ratio Fe(III): organic ligand = 1:1, where100 nM of Fe(III) and organics was used at pH = 8.0) reduced Fe(III) from 0.05% with sinapic acid to 11.92% with gallic acid.
The combined effects of complexation and reduction ability seem to control the differences in Fe(III) reduction processes by the phenols type compounds. In this sense, Fe redox chemistry by GA can be explained by the Fenton chemistry (Equations 8–15) and the GA antioxidative activity (Equations 6, 7). At ratios Fe(III):GA > 2:1, prooxidative activity dominates due to the reduction of Fe(III) to Fe(II), producing HO radicals. At ratios < 2:1, the antioxidative effect controls due to the important scavenging of HO radicals by GA (Equation 15) (Strlič et al., 2002; Yen et al., 2002). The oxidation of GA to hydroxyquinone is simultaneous with a reduction from Fe(III) to Fe(II) (Hynes and O’Coinceanainn, 2001). This process is affected by the composition of seawater, where at pH 8.0 the presence of Mg2+ blocks the semiquinone to quinone electron transfer and consequently the Fe(III) reduction to Fe(II) (Santana-Casiano et al., 2010).
Other aspects that may influence Fe(III) reduction by GA are the acidic species of GA predominant in the medium pH and the degree of GA oxidation at that pH. The acidity constants of GA are: pKa1 = 4.39, due to the carboxylic group and pKa2 = 8.50, pKa3 = 10.3 and pKa4 = 13 due to the three phenolic groups (Eslami et al., 2010; Jabbari, 2015). In the pH range of this study (7.0–8.2) the predominance of the acidic species varies from a practically total predominance of the H3A– species (GA that has given up one proton) at pH = 7.0 to a predominance of approximately 50% for each of the H3A– and H2A2– species (H2A2–: GA that has given up two protons) at pH = 8.0. Considering the different predominance of acidic GA species with increasing pH, the oxidation of GA by oxygen that increases with increasing pH (Strlič et al., 2002; Pant et al., 2019) was important above pH = 7.0 (Strlič et al., 2002), and may also influence the decrease in the rate of Fe(III) reduction with increasing pH. The spectrum of GA after 1 h of bubbling with pure air differs at pH 8.0 and 7.0 (Figures 3A,B, respectively). At pH 7.0 (Figure 3B) and 500 nM GA, the signals at wavelengths close to 430 nm typical of benzoquinone (Hynes and O’Coinceanainn, 2001; Santana-Casiano et al., 2010; Masoud et al., 2014) and 600 nm typical related with quinone (Slawiñska et al., 2007) are much smaller than at pH = 8.0 (Figure 3A), even when compared to the signal with only 100 nM GA.
The different behavior of Fe(III) reduction in NaCl-NaHCO3 and SW can be related to the presence of the major ions in seawater. Figure 6 showed that, in the studied pH range, Mg2+ considerably increased the rate constant of Fe(III) reduction (with respect to NaCl) while Ca2+ decreased it, being practically equal k′Fe(III) in SW and NaCl-NaHCO3-Ca2+ at pH = 8.0 [9.65 (±0.07) × 10–6 and 1.01 (±0.01) × 10–5 s–1, respectively]. Therefore, Ca2+ by own can account for the observed differences in NaCl-NaHCO3 and SW while the increase observed by Mg2+ should be compensated by other major ions. This behavior is different from that observed in catechol, catechin, sinapic acid, and gentisic acid (Santana-Casiano et al., 2010, 2014; Arreguin et al., 2021). Santana-Casiano et al. (2010) reported that the presence of Mg2+ blocked Fe(III) reduction by catechol in a pH-dependent process, while Ca2+ decreased it. Catechin and sinapic acid (Santana-Casiano et al., 2014) decreased Fe(III) reduction in the presence of Mg2+ and Ca2+. However, Gentisic acid (Arreguin et al., 2021) increased the Fe(III) reduction with Ca2+ and Mg2+.
Mg2+ ion has been shown to exert a great influence on the oxidation of some phenols at slightly basic pH by influencing the general mechanism of catechol (Nikolić et al., 1988) and GA (Nikolić et al., 2011) oxidation. In Figure 9, where the spectra of gallic acid at 100 nM concentration after 60 min of aeration with pure air in NaCl-NaHCO3, NaCl-NaHCO3-Ca2+, and NaCl-NaHCO3-Mg2+ are represented, it is observed that with Mg2+ the signals of benzoquinone and semiquinone are by far the lowest. The decrease in the signal of the GA spectrum was also observed when the pH changed from 8.0 to 7.0 (Figures 3A,B) and was related to the increase in k′Fe(III) with decreasing pH. That is, a decrease in the oxidation rate constant of gallic acid increased the reduction of Fe(III) and, as observed in Figure 9, Mg2+ decreased such oxidation. All these results demonstrated that the interaction of Fe with polyphenols and major ions of seawater is highly complex.
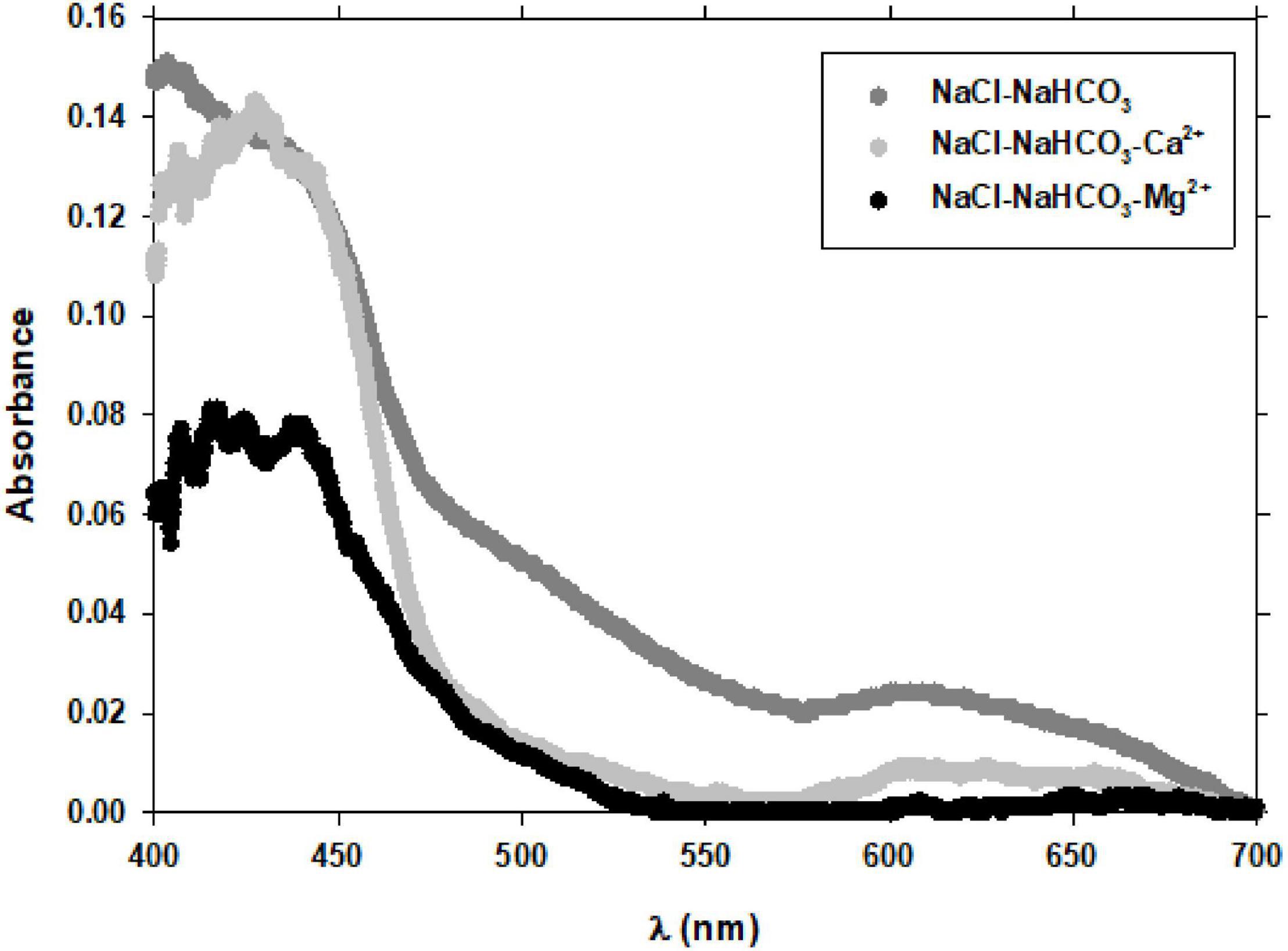
Figure 9. Effect of Ca2+ and Mg2+ ions on the GA spectrum in NaCl (+2 mM NaHCO3). Experimental conditions were: [Fe]0 = 0 nM; [GA] = 100 nM; pH = 8.0; T = 25°C.
The rate constant of Fe(III) reduction is also affected by the GA concentration. At pH = 7.0, an increase in the constants was observed over the entire range of Fe:GA ratios (from 1:2 to 1:20). At pH = 8.0, the Fe(II) was only detected at ratios lower than 1:10. An increase in Fe(III) reduction rate constant was also obtained by Arreguin et al. (2021) with gentisic acid. Wan et al. (2018) for river and peat (pH close to 8) also found that when the concentration of gallic acid increases, the amount of Fe(II) produced increases. The amount increased as the Fe:GA ratio increased from 1:2 to 1:10, with no differences observed between the ratios 1:10 and 1:20. As reduced Fe(II) is formed and get back oxidized, it can be again complexed with excess GA and kept longer in solution, increasing the amount of reduced Fe(III).
The present study indicates that GA in seawater can promote the presence of Fe(II) through a redox electron-transfer process of the Fe(III) complexed through a semiquinone/quinone pair to Fe(II) increasing the persistence of bioavailable iron in seawater in a process that can be favored under nowadays ocean acidification scenario.
Conclusion
The permanence of Fe(II) in seawater increased by the presence of GA, a polyphenol produced by marine phytoplankton. GA promoted the Fe(III) reduction and retard the Fe(II) oxidation in SW and NaCl-NaHCO3 as a function of pH and GA concentration.
In this sense, the presence of GA produced a significant decrease in the Fe(II) oxidation rate constant in SW (Fe:GA ratio from 1:1 to 1:4), favoring the Fe(II) permanence in SW with increasing GA concentration. The decrease in Fe(II) oxidation rate constants is related to the reduction process of Fe(III) present in the solution mediated by GA oxidation.
The present results demonstrated that Fe(III) is reduced to Fe(II) in a pH-dependent process when GA is in solution and both SW and NaCl-NaHCO3. The Fe(III) reduction rate increased with the pH decreasing, with a slope of 0.46 ± 0.03 in NaCl-NaHCO3 and 0.91 ± 0.14 in SW. The addition of major ions of SW such as Ca2+ and Mg2+ to the NaCl-NaHCO3 solution affected the electron transfer redox process to the semiquinone/quinone, retarding the processes the presence of Ca2+. The Fe(III) reduction rate increased with the GA concentration. At pH = 7.0, it was observed over the entire range of Fe: GA ratios. At pH = 8.0, Fe(II) was only detected at ratios from 1:3 to 1:10, because the Fe(II)-FZ3 peak is masked by the characteristic GA peaks at 400 and 600 nm, which increase with higher GA levels and pH.
The results of this study show that the presence of GA significantly increases the residence time of Fe(II) in SW because it can reduce Fe(III) to Fe(II). Likewise, a decrease in pH favors this reduction, showing that ocean acidification may contribute to a higher amount of bioavailable Fe in the global ocean through this redox process.
Data Availability Statement
The raw data supporting the conclusions of this article will be made available by the authors, without undue reservation.
Author Contributions
NP-A and AG carried out the experiments and data treatments. MG-D, JS-C, and AG performed the work design. All authors made significant contributions toward the writing of the manuscript.
Funding
This study received funding from the European Union’s Horizon 2020 research and innovation program under grant agreement no. 820989 (project COMFORT, Our common future ocean in the Earth system–quantifying coupled cycles of carbon, oxygen, and nutrients for determining and achieving safe operating spaces with respect to tipping points). We also acknowledge the financial support for the ATOPFe project (CTM2017-83476-P) from the Ministerio de Ciencia e Innovación (Spain). The participation of AG was within the Programa de Cooperación INTERREG V-A España-Portugal MAC (Madeira-Azores-Canarias) 2014e2020 (PLANCLIMAC project).
Conflict of Interest
The authors declare that the research was conducted in the absence of any commercial or financial relationships that could be construed as a potential conflict of interest.
Publisher’s Note
All claims expressed in this article are solely those of the authors and do not necessarily represent those of their affiliated organizations, or those of the publisher, the editors and the reviewers. Any product that may be evaluated in this article, or claim that may be made by its manufacturer, is not guaranteed or endorsed by the publisher.
References
Abdelwahed, A., Bouhlel, I., Skandrani, I., Valenti, K., Kadri, M., Guiraud, P., et al. (2007). Study of antimutagenic and antioxidant activities of Gallic acid and 1, 2, 3, 4, 6-pentagalloylglucose from Pistacia lentiscus: confirmation by microarray expression profiling. Chem. Biol. Interact. 165, 1–13. doi: 10.1016/j.cbi.2006.10.003
Andjelković, M., Van Camp, J., De Meulenaer, B., Depaemelaere, G., Socaciu, C., Verloo, M., et al. (2006). Iron-chelation properties of phenolic acids bearing catechol and galloyl groups. Food Chem. 98, 23–31. doi: 10.1016/j.foodchem.2005.05.044
Arreguin, M. L., González, A. G., Pérez-Almeida, N., Arnone, V., González-Dávila, M., and Santana-Casiano, J. M. (2021). The role of gentisic acid on the Fe(III) redox chemistry in marine environments. Mar. Chem. 234:104003. doi: 10.1016/j.marchem.2021.104003
Avendaño, L., Gledhill, M., Achterberg, E. P., Rerolle, V., and Schlosser, C. (2016). Influence of ocean acidification on the organic complexation of iron and copper in Northwest European shelf seas; a combined observational and model study. Front. Mar. Sci. 3:58. doi: 10.3389/fmars.2016.00058
Badhani, B., Sharma, N., and Kakkar, R. (2015). Gallic acid: a versatile antioxidant with promising therapeutic and industrial applications. RSC Adv. 5, 27540–27557. doi: 10.1039/C5RA01911G
Bates, N. R. (2017). Twenty years of marine carbon cycle observations at Devils Hole Bermuda provide insights into seasonal hypoxia, coral reef calcification, and ocean acidification. Front. Mar. Sci. 4:36. doi: 10.3389/fmars.2017.00036
Bates, N. R., Astor, Y. M., Church, M. J., Currie, K., Dore, J. E., González-Dávila, M., et al. (2014). A time-series view of changing surface ocean chemistry due to ocean uptake of anthropogenic CO. Oceanography 27, 126–141. doi: 10.5670/oceanog.2014.16
Behrenfeld, M. J., and Milligan, A. J. (2013). Photophysiological expressions of iron stress in phytoplankton. Ann. Rev. Mar. Sci. 5, 217–246. doi: 10.1146/annurev-marine-121211-172356
Benner, R., Palulski, J. D., Mccarthy, M., Hedges, I., and Hatcher, P. (1992). Bulk chemical characteristics of dissolved organic matter in the ocean. Science 255, 1561–1564. doi: 10.1126/science.255.5051.1561
Blazevic, A., Orlowska, E., Kandioller, W., Jirsa, F., Keppler, B. K., Tafili-Kryeziu, M., et al. (2016). Photoreduction of terrigenous Fe-humic substances leads to bioavailable iron in oceans. Angew. Chem. 128, 6527–6532. doi: 10.1002/anie.201600852
Boye, M., Nishioka, J., Croot, P., Laan, P., Timmermans, K. R., and Strass, V. H. (2010). Significant portion of dissolved organic Fe complexes in fact is Fe colloids. Mar. Chem. 122, 20–27. doi: 10.1016/j.marchem.2010.09.001
Brown, J. E., Khodr, H., Hider, R. C., and Rice-Evans, C. A. (1998). Structural dependence of flavonoid interactions with Cu2+ ions: implications for their antioxidant properties. Biochem. J. 330, 1173–1178. doi: 10.1042/bj3301173
Byrne, R. H., Mecking, S., Feely, R. A., and Liu, X. (2010). Direct observations of basin-wide acidification of the North Pacific ocean. Geophys. Res. Lett. 37, 1–5. doi: 10.1029/2009GL040999
Caldeira, K., and Wickett, M. (2003). Anthropogenic carbon and ocean pH. Nature 425:365. doi: 10.1038/425365a
Cappelli, C., Mennucci, B., and Monti, S. (2005). Environmental effects on the spectroscopic properties of gallic acid: a combined classical and quantum mechanical study. J. Phys. Chem. A 109, 1933–1943. doi: 10.1021/jp044781s
Caprara, S., Buck, K. N., Gerringa, L. J. A., Rijkenberg, M. J. A., and Monticelli, D. (2016). A compilation of iron speciation data for open oceanic waters. Front. Mar. Sci. 3:221. doi: 10.3389/fmars.2016.00221
Christoforidis, K. C., Vasiliadou, I. A., Louloudidand, M., and Deligiannakis, Y. (2018). Gallic acid mediated oxidation of pentachlorophenol by the Fenton reactionunder mild oxidative conditions. J Chem. Technol. Biotechnol. 93, 1601–1610. doi: 10.1002/jctb.5529
Chvátalová, K., Slaninová, I., Březinová, L., and Slanina, J. (2008). Influence of dietary phenolic acids on redox status of iron: ferrous iron autoxidation and ferric iron reduction. Food Chem. 106, 650–660. doi: 10.1016/j.foodchem.2007.06.028
Cos, P., Ying, L., Calomme, M., Hu, J. P., Cimanga, K., Van Poel, B., et al. (1998). Structure-activity relationship and classification of flavonoids as inhibitors of xanthine oxidase and superoxide scavengers. J. Nat. Prod. 61, 71–76. doi: 10.1021/np970237h
Craig, P. S., Shaw, T. J., Miller, P. L., Pellechia, P. J., and Ferry, J. L. (2009). Use of multiparametric techniques to quantify the effects of naturally occurring ligands on the kinetics of Fe(II) oxidation. Environ. Sci. Technol. 43, 337–342. doi: 10.1021/es802005p
Croot, P. L., and Heller, M. I. (2012). The importance of kinetics and redox in the biogeochemical cycling of iron in the surface ocean. Front. Microbiol. 3:219. doi: 10.3389/fmicb.2012.00219
Croot, P. L., Heller, M. I., Schlosser, C., and Wuttig, K. (2011). “Utilizing radio-isotopes trace metals speciation measurements in seawater,” in Radioisotopes – Applications in Physical Sciences, ed. Singh N. (Intech), 247–278. doi: 10.5772/23182
De, A., Dey, D., Das, A., Kole, N., and Biswas, B. (2018). Gentisate-1, 2-dioxygenase activity by an iron (II)-phenanthroline complex. J. Chem. Sci. 130:26. doi: 10.1007/s12039-018-1425-4
Dong, H., Sans, C., Li, W., and Qiang, Z. (2016). Promoted discoloration of methyl orange in H2O2/Fe(III) Fenton system: effects of gallic acid on iron cycling. Sep. Purif. Technol. 171, 144–150. doi: 10.1016/j.seppur.2016.07.033
Eslami, A. C., Pasanphan, W., Wagner, B. A., and Buettner, G. R. (2010). Free radicals produced by the oxidation of gallic acid: an electron paramagnetic resonance study. Chem. Cent. J. 4, 1–4. doi: 10.1186/1752-153X-4-15
Ferreira-Severino, J. F., Goodman, B. A., Reichenauer, T. G., and Pirker, K. F. (2011). Is there a redox reaction between Cu (II) and gallic acid? Free Radic. Res. 45, 123–132.
Galato, D., Ckless, K., Susin, M. F., Giacomelli, C., Ribeiro-do-Valle, R. M., and Spinelli, A. (2001). Antioxidant capacity of phenolic and related compounds: correlation among electrochemical, visible spectroscopy methods and structure–antioxidant activity. Redox Rep. 6, 243–250. doi: 10.1179/135100001101536391
Gattuso, J., and Hansson, L. (eds) (2011). Ocean Acidification. Oxford: Oxford University press, 326.
Geider, R. J., and La Roche, J. (1994). The role of iron in phytoplankton photosynthesis, and the potential for iron-limitation of primary productivity in the sea. Photosynth. Res. 39, 275–301. doi: 10.1007/BF00014588
Ghosh, M. M., O’Connor, J. T., and Engelbrecht, R. S. (1967). Bathophenanthroline method for the determination of ferrous iron. J. Am. Water Works Assoc. 59, 897–905. doi: 10.1002/j.1551-8833.1967.tb03425.x
Giftson, J. S., Jayanthi, S., and Nalini, N. (2010). Chemopreventive efficacy of gallic acid, an antioxidant and anticarcinogenic polyphenol, against 1, 2-dimethyl hydrazine induced rat colon carcinogenesis. Investig. New Drugs 28, 251–259. doi: 10.1007/s10637-009-9241-9
Gledhill, M., Achterberg, E. P., Li, K., Mohamed, K. N., and Rijkenberg, M. J. A. (2015). Influence of ocean acidification on the complexation of iron and copper by organic ligands in estuarine waters. Mar Chem. 177, 421–433. doi: 10.1016/j.marchem.2015.03.016
González, A. G., Cadena-Aizaga, M. I., Sarthou, G., González-Dávila, M., and Santana- Casiano, J. M. (2019). Iron complexation by phenolic ligands in seawater. Chem. Geol. 511, 380–388. doi: 10.1016/j.chemgeo.2018.10.017
González, A. G., Pérez-Almeida, N., Santana-Casiano, J. M., Millero, F. J., and González-Dávila, M. (2016). Redox interactions of Fe and Cu in seawater. Mar. Chem. 179, 12–22. doi: 10.1016/j.marchem.2016.01.004
Gonzaìlez, A. G., Santana-Casiano, J. M., Gonzaìlez-Daìvila, M., and Peìrez, N. (2012). Effect of organic exudates of Phaeodactylum tricornutum on the Fe(II) oxidation rate constant. Cienc. Mar. 38, 245–261. doi: 10.7773/cm.v38i1b.1808
González, A. G., Santana-Casiano, J. M., González-Dávila, M., Pérez-Almeida, N., and Suárez de Tangil, M. (2014). Effect of Dunaliella tertiolecta organic exudates on the Fe(II) oxidation kinetics in seawater. Environ. Sci. Technol. 48, 7933–7941. doi: 10.1021/es5013092
González-Dávila, M., Santana-Casiano, J. M., and Millero, F. J. (2005). Oxidation of iron (II) nanomolar with H2O2 in seawater. Geochim. Cosmochim. Acta 69, 83–93. doi: 10.1016/j.gca.2004.05.043
González-Dávila, M., Santana-Casiano, J. M., and Millero, F. J. (2006). Competition between O2 and H2O2 in the oxidation of Fe(II) in natural waters. J. Solut. Chem. 35, 95–111. doi: 10.1007/s10953-006-8942-3
Guo, Q., Zhao, B., Shen, S., Hou, J., Hu, J., and Xin, W. (1999). ESR study on the structure–antioxidant activity relationship of tea catechins and their epimers. Biochim. Biophys. Acta Gen. Subj. 1427, 13–23. doi: 10.1016/S0304-4165(98)00168-8
Hanasaki, Y., Ogawa, S., and Fukui, S. (1994). The correlation between active oxygens scavenging and antioxidative effects of flavonoids. Free Radic. Biol. Med. 16, 845–850. doi: 10.1016/0891-5849(94)90202-X
Hansell, D. A., and Carlson, C. A. (eds) (2014). Biogeochemistry of Marine Dissolved Organic Matter. Cambridge, MA: Academic Press.
Hopkinson, C., and Vallino, J. (2005). Efficient export of carbon to the deep ocean through dissolved organic matter. Nature 433, 142–145. doi: 10.1038/nature03191
Hynes, M. J., and O’Coinceanainn, M. (2001). The kinetics and mechanisms of reactions of iron(III) with gallic acid, gallic methyl ester and catechin. J. Inorg. Biochem. 85, 131–142. doi: 10.1016/S0162-0134(01)00205-7
IPCC (2014). “Climate change 2014: synthesis report,” in Contribution of Working Groups I, II and III to the Fifth Assessment Report of the Intergovernmental Panel on Climate Change, eds R. K. Pachauri and L. A. Meyer (Geneva: IPCC).
IPCC (2021). “Summary for Policymakers,” in Climate Change 2021: The Physical Science Basis. Contribution of Working Group I to the Sixth Assessment Report of the Intergovernmental Panel on Climate Change, eds V. Masson-Delmotte, P. Zhai, A. Pirani, S. L. Connors, C. Péan, S. Berger, et al. (Cambridge: Cambridge University Press).
Ivanina, A. V., and Sokolova, I. M. (2015). Interactive effects of metal pollution and ocean acidification on physiology of marine organisms. Curr. Zool. 61, 653–668. doi: 10.1093/czoolo/61.4.653
Jabbari, M. (2015). Solvent dependence of protonation equilibria for gallic acid in water and different acetonitrile–water cosolvent systems. J. Mol. Liquids 208, 5–10. doi: 10.1016/j.molliq.2015.03.055
Joshi, R., Gangabhagirathi, R., Venu, S., Adhikari, S., and Mukherjee, T. (2012). Antioxidant activity and free radical scavenging reactions of gentisic acid: in-vitro and pulse radiolysis studies. Free Radic. Res. 46, 11–20. doi: 10.3109/10715762.2011.633518
Khan, N. S., Ahmad, A., and Hadi, S. M. (2000). Anti-oxidant, pro-oxidant properties of tannic acid and its binding to DNA. Chem. Biol. Interact. 125, 177–189. doi: 10.1016/S0009-2797(00)00143-5
Kim, D. O., Lee, K. W., Lee, H. J., and Lee, C. Y. (2002). Vitamin C equivalent antioxidant capacity (VCEAC) of phenolic phytochemicals. J. Agric. Food Chem. 50, 3713–3717. doi: 10.1021/jf020071c
King, D. W., and Farlow, R. (2000). Role of carbonate speciation on the oxidation of Fe(II) by H2O2. Mar. Chem. 70, 201–209. doi: 10.1016/S0304-4203(00)00026-8
Krachler, R., Jirsa, F., and Ayromlou, S. (2005). Factors influencing the dissolved iron input by river water to the open ocean. Biogeosciences 2, 311–315. doi: 10.5194/bg-2-311-2005
Krachler, R., Krachler, R. F., von der Kammer, F., Süphandag, A., Jirsa, F., Ayromlou, S., et al. (2010). Relevance of peat-draining rivers for the riverine input of dissolved iron into the ocean. Sci. Total Environ. 408, 2402–2408. doi: 10.1016/j.scitotenv.2010.02.018
Krachler, R., Krachler, R. F., Wallner, G., Hann, S., Laux, M., Recalde, M. F. C., et al. (2015). River-derived humic substances as iron chelators in seawater. Mar. Chem. 174, 85–93. doi: 10.1016/j.marchem.2015.05.009
Krachler, R., Krachler, R. F., Wallner, G., Steier, P., El Abiead, Y., Wiesinger, H., et al. (2016). Sphagnum-dominated bog systems are highly effective yet variable sources of bio-available iron to marine waters. Sci. Total Environ. 556, 53–62. doi: 10.1016/j.scitotenv.2016.03.012
Krachler, R., Krachler, R., Valda, A., and Keppler, B. K. (2019). Natural iron fertilization of the coastal ocean by “blackwater rivers”. Sci. Total Environ. 656, 952–958. doi: 10.1016/j.scitotenv.2018.11.423
Krachler, R., von der Kammer, F., Jirsa, F., Süphandag, A., Krachler, R. F., Plessl, C., et al. (2012). Nanoscale lignin particles as sources of dissolved iron to the ocean. Glob. Biogeochem. Cycles 26:GB3024. doi: 10.1029/2012GB004294
Lee, Y. P., Fujii, M., Kikuchi, T., Natsuike, M., Ito, H., Watanabe, T., et al. (2017). Importance of allochthonous and autochthonous dissolved organic matter in Fe(II) oxidation: a case study in Shizugawa Bay watershed, Japan. Chemosphere 180, 221–228. doi: 10.1016/j.chemosphere.2017.04.008
Li, A. S., Bandy, B., Tsang, S. S., and Davison, A. J. (2000). DNA-breaking versus DNA-protecting activity of four phenolic compounds invitro. Free Radic. Res. 33, 551–566. doi: 10.1080/10715760000301091
Liu, X., and Millero, F. J. (2002). The solubility of iron in seawater. Mar. Chem. 77, 43–54. doi: 10.1016/S0304-4203(01)00074-3
Lodovici, M., Guglielmi, F., Casalini, C., Meoni, M., Cheynier, V., and Dolara, P. (2001). Antioxidant and radical scavenging properties in vitro of polyphenolic extracts from red wine. Eur. J. Nutr. 40, 74–77. doi: 10.1007/PL00007386
López, A., Rico, M., Santana-Casiano, J. M., González, A. G., and González-Dávila, M. (2015). Phenolic profile of Dunaliella tertiolecta growing under high levels of copper and iron. Environ. Sci. Pollut. Res. 22, 14820–14828. doi: 10.1007/s11356-015-4717-y
Luther, G. W. III, Mullaugh, K. M., Hauser, E. J., Rader, K. J., and Di Toro, D. M. (2021). Determination of ambient dissolved metal ligand complexation parameters via kinetics and pseudo-voltammetry experiments. Mar. Chem. 234:103998. doi: 10.1016/j.marchem.2021.103998
Masoud, M. S., Ali, A. E., Haggag, S. S., and Nasr, N. M. (2014). Spectroscopic studies on acid gallic and their iron (III) complexes. Biomol. Spectr. 120, 505–551. doi: 10.1016/j.saa.2013.10.054
Melidou, M., Riganakos, K., and Galaris, D. (2005). Protection against nuclear DNA damage offered by flavonoids in cells exposed to hydrogen peroxide: the role of iron chelation. Free Radic. Biol. Med. 39, 1591–1600. doi: 10.1016/j.freeradbiomed.2005.08.009
Miller, W. L., King, D. W., Lin, J., and Kester, D. R. (1995). Photochemical redox cycling of iron in coastal seawater. Mar. Chem. 50, 63–77. doi: 10.1016/0304-4203(95)00027-O
Millero, F. J. (1986). The pH of estuarine waters. Limnol. Oceanogr. 31, 839–847. doi: 10.4319/lo.1986.31.4.0839
Millero, F. J., Woosley, R., Ditrolio, B., and Waters, J. (2009). Effect of ocean acidification on the speciation of metals in seawater. Oceanography 22, 72–85. doi: 10.5670/oceanog.2009.98
Mira, L., Fernandez, M. T., Santos, M., Rocha, R., Florencio, M. H., and Jennings, K. R. (2002). Interactions of flavonoids with iron and copper ions: a mechanism for their antioxidant activity. Free Radic. Res. 36, 1199–1208. doi: 10.1080/1071576021000016463
Moffett, J. W., and Zika, R. G. (1987). Reaction kinetics of hydrogen peroxide with copper and iron in seawater. Environ. Sci. Technol. 21, 804–810. doi: 10.1021/es00162a012
Moran, J. F., Klucas, R. V., Grayer, R. J., Abian, J., and Becana, M. (1997). Complexes of iron with phenolic compounds from soybean nodules and other legume tissues: prooxidant and antioxidant properties. Free Radic. Biol. Med. 22, 861–870. doi: 10.1016/S0891-5849(96)00426-1
Morel, F. M. M., and Price, N. M. (2003). The biogeochemical cycles of trace metals in the oceans. Science 300, 944–947. doi: 10.1126/science.1083545
Nikolić, G. M., Promovic, P. I., and Nikolić, R. S. (1988). Spectrophotometric study of catechol oxidation by aerial O2 in alkaline aqueous solutions containing Mg(II), Ions. Spectr. Lett. 31, 327–333. doi: 10.1080/00387019808003257
Nikolić, G. M., Veselinović, A. M., and Nikolić, R. S. (2011). Spectroscopic study of Mg(II) ion influence on the autoxidation of gallic acid in weakly alkaline aqueous solutions. Russ. J. Phys. Chem. 85, 2270–2273. doi: 10.1134/S0036024411130176
Oniki, T., and Takahama, U. (2004). Free radicals produced by the oxidation of gallic acid and catechin derivatives. J. Wood Sci. 50, 545–547. doi: 10.1007/s10086-003-0591-1
Orlowska, E., Enyedy, E. A., Pignitter, M., Jirsa, F., Krachler, R., Kandioller, W., et al. (2017a). β-O-4 type dilignol compounds and their iron complexes for modeling of iron binding to humic acids: synthesis, characterization, electrochemical studies and algal growth experiments. New J. Chem. 41, 11546–11555. doi: 10.1039/c7nj02328f
Orlowska, E., Roller, A., Pignitter, M., Jirsa, F., Krachler, R., Kandioller, W., et al. (2017b). Synthetic iron complexes as models for natural iron-humic compounds: synthesis, characterization and algal growth experiments. Sci. Total Environ. 577, 94–104. doi: 10.1016/j.scitotenv.2016.10.109
Orlowska, E., Roller, A., Wiesinger, H., Pignitter, M., Jirsa, F., Krachler, R., et al. (2016). Benzoic hydroxamate-based iron complexes as model compounds for humic substances: synthesis, characterization and algal growth experiments. RSC Adv. 6, 40238–40249. doi: 10.1039/c5ra25256c
Orr, J., Fabry, V., Aumont, O., Bopp, L., Doney, S. C., Feely, R. A., et al. (2005). Anthropogenic ocean acidification over the twenty-first century and its impact on calcifying organisms. Nature 437, 681–686. doi: 10.1038/nature04095
Palafox-Carlos, H., Gil-Chávez, J., Sotelo-Mundo, R., Namiesnik, J., Gorinstein, S., and González-Aguilar, G. A. (2012). Antioxidant Interactions between major phenolic compounds found in ‘Ataulfo’ mango pulp: chlorogenic, gallic, protocatechuic and vanillic acids. Molecules 17, 12657–12664. doi: 10.3390/molecules171112657
Pan, T., Wang, Y., Yang, X., Huang, X., and Qiu, R. (2020). Gallic acid accelerated BDE47 degradation in PMS/Fe(III) system: oxidation intermediates autocatalyzed redox cycling of iron. Chem. Eng. J. 384:123248. doi: 10.1016/j.cej.2019.123248
Pannala, A. S., Chan, T. S., O’Brien, P. J., and Rice-Evans, C. A. (2001). Flavonoid B-ring chemistry and antioxidant activity: fast reaction kinetics. Biochem. Biophys. Res. Commun. 282, 1161–1168. doi: 10.1006/bbrc.2001.4705
Pant, A. F., Özkasikci, D., Fürtauer, S., and Reinelt, M. (2019). The effect of deprotonation on the reaction kinetics of an oxygen scavenger based on gallic acid. Front. Chem. 7:680. doi: 10.3389/fchem.2019.00680
Pardeshi, S., Dhodapkar, R., and Kumar, A. (2013). Quantum chemical density functional theory studies on the molecular structure and vibrational spectra of Gallic acid imprinted polymers. Spectrochim. Acta Part A Mol. Biomol. Spectrosc. 116, 562–573. doi: 10.1016/j.saa.2013.07.067
Pérez-Almeida, N., González, A. G., Santana-Casiano, J. M., and González-Dávila, M. (2019). Iron and copper redox interactions in UV-seawater: a kinetic model approach. Chem. Geol. 506, 149–161. doi: 10.1016/j.chemgeo.2018.12.041
Perron, N. R., Hodges, J. N., Jenkins, M., and Brumaghim, J. L. (2010). Kinetics of iron oxidation upon polyphenol binding. Inorg. Chem. 47, 6153–6161. doi: 10.1039/c0dt00752h
Porwal, S. K., Furia, E., Harris, M. E., Viswanathan, R., and Devireddy, L. (2015). Synthetic, potentiometric and spectroscopic studies of chelation between Fe(III) and 2,5-DHBA supports salicylate-mode of siderophore binding interactions. J. Inorg. Biochem. 145, 1–10. doi: 10.1016/j.jinorgbio.2014.12.010
Priscilla, D. H., and Prince, P. S. M. (2009). Cardioprotective effect of gallic acid on cardiac troponin-T, cardiac marker enzymes, lipid peroxidation products and antioxidants in experimentally induced myocardial infarction in Wistar rats. Chem. Biol. Interact. 179, 118–124. doi: 10.1016/j.cbi.2008.12.012
Rathgeb, A., Causon, T., Krachler, R., and Hann, S. (2017). From the peat bog to the estuarine mixing zone: common features and variances in riverine dissolved organic matter determined by non-targeted analysis. Mar. Chem. 194, 158–167. doi: 10.1016/j.marchem.2017.06.012
Raven, J., Caldeira, K., Elderfield, H., Hoegh-Guldberg, O., Liss, P., Riebesell, U., et al. (2005). Ocean Acidification due to Increasing Atmospheric Carbon Dioxide. London: The Royal Society, 60. doi: 10.1080/02688690801911598
Rice-Evans, C. A., Miller, N. J., and Paganga, G. (1996). Structure-antioxidant activity relationships of flavonoids and phenolic acids, 20. Free Radic. Biol. Med. 20, 933–956. doi: 10.1016/0891-5849(95)02227-9
Rice-Evans, C. A., Miller, N. J., and Paganga, G. (1997). Antioxidant properties of phenolic compounds. Trends Plant Sci. 2, 152–159. doi: 10.1016/S1360-1385(97)01018-2
Rico, M., López, A., Santana-Casiano, J. M., González, A. G., and González-Dávila, M. (2013). Variability of the phenolic profile in the diatom Phaeodactylum tricornutum growing under copper and iron stress. Limnol. Oceanogr. 51, 144–152. doi: 10.4319/lo.2013.58.1.0144
Riebesell, U., Schulz, K. G., Bellerby, R. G. J., Botros, M., Fritsche, P., Meyerhöfer, M., et al. (2007). Enhanced biological carbon consumption in a high CO2 ocean. Nature 450, 545–548. doi: 10.1038/nature06267
Rose, A. L., and Waite, T. D. (2002). Kinetic model for Fe (II) oxidation in seawater in the absence and presence of natural organic matter. Environ. Sci. Technol. 36, 433–444. doi: 10.1021/es0109242
Rose, A. L., and Waite, T. D. (2003). Kinetic of iron complexation by dissolved natural organic matter in coastal waters. Mar. Chem. 84, 85–103. doi: 10.1016/S0304-4203(03)00113-0
Roy, E. G., and Wells, M. L. (2011). Evidence for regulation of Fe (II) oxidation by organic complexing ligands in the Eastern Subarctic Pacific. Mar. Chem. 127, 115–122. doi: 10.1016/j.marchem.2011.08.006
Royal Society. (2005). Ocean Acidification Due to Increasing Atmospheric Carbon Dioxide. Policy document 12/05. London: The Royal Society.
Rue, E. L., and Bruland, K. W. (1995). Complexation of iron (III) by natural organic ligands in the central North Pacific as determined by a new competitive ligand equilibration/adsorptive cathodic stripping. Mar. Chem. 50, 117–138. doi: 10.1016/0304-4203(95)00031-l
Samperio-Ramos, G., González-Dávila, M., and Santana-Casiano, J. M. (2018). Impact on the Fe redox cycling of organic ligands released by Synechococcus PCC 7002, under different iron fertilization scenarios. Model. Appr. J. Mar. Syst. 182, 67–78. doi: 10.1016/j.jmarsys.2018.01.009
Santana-Casiano, J. M., González-Dávila, M., and Millero, F. J. (2004). The oxidation of Fe(II) in NaCl–HCO3- and seawater solutions in the presence of phthalate and salicylate ions: a kinetic model. Mar. Chem. 85, 27–40. doi: 10.1016/j.marchem.2003.09.001
Santana-Casiano, J. M., González-Dávila, M., and Millero, F. J. (2005). Oxidation of nanomolar levels of Fe (II) with oxygen in natural waters. Environ. Sci. Technol. 39, 2073–2079. doi: 10.1021/es049748y
Santana-Casiano, J. M., González-Dávila, M., González, A. G., Rico, M., López, A., and Martel, A. (2014). Characterization of phenolic exudates from Phaeodactylum tricornutum and their effects on the chemistry of Fe(II)-Fe(III). Mar. Chem. 158, 10–16. doi: 10.1016/j.marchem.2013.11.001
Santana-Casiano, J. M., González-Dávila, M., Rodríguez, M. J., and Millero, F. J. (2000). The effect of organic compounds in the oxidation kinetics of Fe(II). Mar. Chem. 70, 211–222. doi: 10.1016/S0304-4203(00)00027-X
Santana-Casiano, J. M., González-Dávila, M., Rueda, M. J., Llinás, O., and González-Dávila, E. F. (2007). The interannual variability of oceanic CO2 parameters in thenortheast Atlantic subtropical gyre at the ESTOC site. Glob. Biogeochem. Cycles 21:GB1015. doi: 10.1029/2006GB002788
Santana-Casiano, J., González-Dávila, M., González, A., and Millero, F. (2010). Fe (III) Reduction in the presence of catechol in seawater. Aquat. Geochem. 16, 467–482. doi: 10.1007/s10498-009-9088-x
Schlesier, K., Harwat, M., Böhm, V., and Bitsch, R. (2002). Assessment of antioxidant activity by using different in vitro methods. Free Radic. Res. 36, 177–187. doi: 10.1080/10715760290006411
Severino, J. F., Goodman, B. A., Reichenauer, T. G., and Pirker, K. F. (2011). Is there a redox reaction between Cu (II) and gallic acid? Free Rad. Res. 45, 123–132. doi: 10.3109/10715762.2010.515220
Shaked, Y., and Lis, H. (2012). Disassembling iron availability to phytoplankton. Front. Microbiol. 3:123. doi: 10.3389/fmicb.2012.00123
Shi, D., Xu, Y., Hopkinson, B. M., and Morel, F. M. M. (2010). Effect of ocean acidification on iron availability to marine phytoplankton. Science 327, 676–679. doi: 10.1126/science.1183517
Silva, M. M., Santos, M. R., Caroço, G., Rocha, R., Justino, G., and Mira, L. (2002). Structurantioxidant activity relationships of flavonoids: a reexamination. Free Radic. Res. 36, 1219–1227. doi: 10.1080/198-1071576021000016472
Slawiñska, D., Polewski, K., Rolewski, P., and Slawinski, J. (2007). Synthesis and properties of model humic substances derived from gallic acid. Int. Agrophys. 21, 199–208.
Spilling, K., Schulz, K. G., Paul, A. J., Boxhammer, T., Achterberg, E. P., Hornick, T., et al. (2016). Effects of ocean acidification on pelagic carbon fluxes in a mesocosm experiment. Biogeosciences 13, 6081–6093. doi: 10.5194/bg-13-6081-2016
Sroka, Z., and Cisowski, W. (2003). Hydrogen peroxide scavenging, antioxidant and anti-radical activity of some phenolic acids. Food Chem. Toxicol. 41, 753–758. doi: 10.1016/S0278-6915(02)00329-0
Strlič, M., Radovič, T., Kolar, J., and Pihlar, B. (2002). Anti- and prooxidative properties of gallic acid in Fenton-type systems. J. Agric. Food Chem. 50, 6313–6317. doi: 10.1021/jf025636j
Theis, T. L., and Singer, P. C. (1974). Complexation of iron(II) by organic matter and its effect on iron(II) oxygenation. Environ. Sci. Technol. 8, 569–573. doi: 10.1021/es60091a008
van den Berg, C. M. G. (1995). Evidence for organic complexation of iron in seawater. Mar. Chem. 50, 139–157. doi: 10.1016/0304-4203(95)00032-M
Velika, B., and Kron, I. (2012). Antioxidant properties of benzoic acid derivatives against superoxide radical. Free Radic. Antioxid. 2, 62–67. doi: 10.5530/ax.2012.4.11
Viollier, E., Inglett, P., Hunter, K., Roychoudhury, A. N., and van Capellan, P. (2000). The ferrozine method revisited: Fe(II)/Fe(III) determination in natural waters. Appl. Geochem. 15, 785–790. doi: 10.1016/S0883-2927(99)00097-9
Wan, X., Xiang, W., Wan, N., Yan, S., Bao, Z., and Wang, Y. (2018). Complexation and reduction of iron by phenolic substances: implications for transport of dissolved Fe from peatlands to aquatic ecosystems and global iron cycling. Chem. Geol. 498, 128–138. doi: 10.1016/j.chemgeo.2018.09.019
Witter, A. E., Hutchins, D. A., Butler, A., and Luther, G. W. (2000). Determination of conditional stability constants and kinetic constants for strong model Fe-binding ligands in seawater. Mar. Chem. 69, 1–17. doi: 10.1016/S0304-4203(99)00087-0
Wright, J. S., Johnson, E. R., and DiLabio, G. A. (2001). Predicting the activity of phenolic antioxidants: theoretical method, analysis of substituent effects, and application to major families of antioxidants. J. Am. Chem. Soc. 123, 1173–1183. doi: 10.1021/ja002455u
Wu, Y., Xiang, W., Fu, X., Yan, S., Su, J., Liu, J., et al. (2016). Geochemical interactions between iron and phenolics originated from peatland in Hani, China: implications for effective transport of iron from terrestrial systems to marine. Environ. Earth Sci. 75:336. doi: 10.1007/s12665-015-5189-6
Yen, G. C., Duh, P. D., and Tsai, H. L. (2002). Antioxidant and pro-oxidant properties of ascorbic acid and gallic acid. Food Chem. 79, 307–313. doi: 10.1016/S0308-8146(02)00145-0
Yoshioka, H., Sugiura, K., Kawahara, R., Fujita, T., Making, M., Kamiya, M., et al. (1991). Formation of radicals and chemiluminescence during the autoxidation of tea catechins. Agric. Biol. Chem. 55, 2717–2723. doi: 10.1271/bbb1961.55.2717
Zhao, C., Dodin, G., Yuan, C., Chen, H., Zheng, R., Jia, Z., et al. (2005). “In vitro” protection of DNA from Fenton reaction by plant polyphenol verbascoside. Biochim. Biophys. Acta. Gen. Subj. 1723, 114–123. doi: 10.1016/j.bbagen.2005.02.004
Keywords: gallic acid, iron, complexation, redox process, seawater, ocean acidification
Citation: Pérez-Almeida N, González AG, Santana-Casiano JM and González-Dávila M (2022) Ocean Acidification Effect on the Iron-Gallic Acid Redox Interaction in Seawater. Front. Mar. Sci. 9:837363. doi: 10.3389/fmars.2022.837363
Received: 16 December 2021; Accepted: 25 January 2022;
Published: 11 February 2022.
Edited by:
Marta Plavsic, Rudjer Boskovic Institute, CroatiaReviewed by:
Aikaterini Sakellari, National and Kapodistrian University of Athens, GreeceVlado Cuculić, Rudjer Boskovic Institute, Croatia
Martha Gledhill, Helmholtz Association of German Research Centers (HZ), Germany
Copyright © 2022 Pérez-Almeida, González, Santana-Casiano and González-Dávila. This is an open-access article distributed under the terms of the Creative Commons Attribution License (CC BY). The use, distribution or reproduction in other forums is permitted, provided the original author(s) and the copyright owner(s) are credited and that the original publication in this journal is cited, in accordance with accepted academic practice. No use, distribution or reproduction is permitted which does not comply with these terms.
*Correspondence: Melchor González-Dávila, bWVsY2hvci5nb256YWxlekB1bHBnYy5lcw==