- 1Algal Technologies Program, Centre for Sustainable Development, College of Arts and Sciences, Qatar University, Doha, Qatar
- 2Department of Biological and Environmental Sciences, College of Arts and Sciences, Qatar University, Doha, Qatar
At a time when worldwide water shortage is increasing, seawater is being viewed as an inexhaustible supply of freshwater via the process of seawater desalination. As a result, seawater desalination is becoming more popular, especially in areas where freshwater is scarce, such as the Middle East and North Africa (MENA), which accounts for half of all global saltwater desalination activities. To enhance the efficiency of saltwater desalination, thermal and membrane-based desalination technologies are continually being developed and hybridized systems established. Brine is an unavoidable product of seawater desalination and is commonly disposed of in oceans and seas, where it has negative effects on the surrounding marine environment and its biodiversity due to the resultant increased salinity and temperature, as well as the presence of chemicals. Furthermore, the quality and amount of brine are influenced by several parameters, including the input quality and quantity, the desalination process, and the discharge method. The intensity of brine’s influence on the marine biota is determined by a number of factors; nevertheless, marine species differ in their tolerance to brine’s effects. Desalination technology is improving to maximize water recovery and reduce the volume of brine produced, with the objective of eventually reaching zero liquid discharge and limiting harmful effects on the marine environment. Meanwhile, proper systems for analyzing the effects of seawater desalination facilities on the marine ecology must be implemented. This review study will look at all of the factors that determine the physicochemical features of desalination brine, with a focus on its impact on marine chemistry and biodiversity. More crucially, the most cutting-edge brine management methods will be investigated for long-term desalination and a healthy marine ecosystem.
Introduction
Water security has deteriorated globally as a result of increased water demand due to population growth, as well as contamination and climate change depleting water supplies. (Jones et al., 2019). As a result, most countries are focusing on implementing existing ways for collecting conventional water resources, which is a major worry for humanity’s future that must be addressed. (Panagopoulos et al., 2019). Desalination techniques are currently regarded as the most effective means of acquiring freshwater in many parts of the globe, while saltwater desalination is the sole method of obtaining freshwater in many nations (Blanco-Marigorta et al., 2017).
Desalination is a multi-stage process that splits saltwater into two products: the product stream (freshwater) and the by-product stream (highly concentrated brine) (Mavukkandy et al., 2019). The worldwide desalination capacity expanded from 95.6 M m3 per day in 2016 to 99.8 M m3 per day in 2017 (Al-Kaabi and Mackey, 2019), with the MENA region accounting for 47.5% of global desalination capacity (Kress et al., 2020). According to Jones et al. (2019) findings, 142 M m3 of brine is created daily throughout the world, with Saudi Arabia, the United Arab Emirates (UAE), Kuwait, and Qatar accounted solely 55%. Desalination provides a limitless and continual supply of freshwater, but creating enough freshwater in a sustainable manner is a challenging task (Al-Kaabi and Mackey, 2019). Although much emphasis has been placed on improving the technical aspects of desalination processes, which has resulted in cost savings and increased efficiency, the accompanying environmental issues have been overlooked, in particular the consequences of the discharge of desalination by-products, such as the effects of concentrate effluent on the marine coastal ecosystem (Gacia et al., 2007).
This review paper will provide a complete examination of the many elements influencing desalination brine’s physicochemical characteristics with emphasis on its influence on marine chemistry and biodiversity. More importantly, the most modern brine management solutions for sustainable desalination and a healthy marine environment will also be explored.
Desalination Processes: Case study of the Arabian Gulf
The Arabian Gulf region is hyper-arid and subtropical; it is a shallow semi-enclosed sea with an average depth of 35 m and salinity of 39 psu (Al-Barwani and Purnama, 2019). Low precipitation, freshwater input from land, and a high evaporation rate, combined with its topographical features, have resulted in extreme seawater temperatures and salinity (Sheppard et al., 2010). Seawater desalination accounts for 61% of all freshwater produced globally (Kress et al., 2020).
Desalination technologies are currently divided into two groups: thermal and membrane-based, which are mostly represented by multi-stage flash (MSF) and reverse osmosis (RO), respectively. Hybrid desalination process combining both technologies in the same plant was described by Loutatidou et al. (2017). When compared to current desalination technologies, RO is the most cost-effective, reliable, and energy-efficient at producing freshwater (Shaaban and Yahya, 2017). Accordingly, MSF technology dominates the desalination facilities in the Arabian Gulf, accounting for about 86.7%of total desalination capacity, owing to its reliability and simplicity (Al-Kaabi and Mackey, 2019; Anis et al., 2019). The dominance of MSF in the Arabian Gulf has shifted in favour of RO, as only four of the 38 planned desalination plants in 2012 were MSF, while 26 were RO. This is largely resulting from the technological advancements in RO technologies, which have enabled RO to operate in the Arabian Gulf’s extreme water conditions, thus lowering the cost of desalination treatment (Dawoud, 2012; Ahmad and Baddour, 2014).
Reverse Osmosis
The RO process works by applying hydraulic pressure to the chamber with the highest salt concentration, forcing water molecules to pass through a semipermeable membrane into the chamber with the lowest salt concentration (Lazarides and Katsanidis, 2003). Subsequently, the salt is prevented from passing through the membrane, and concentrated brine is formed in the feed chamber (Panagopoulos et al., 2019). The capacity of RO for feed water containing total dissolved solids (TDS) of up to 70,000 mg/L, RO may desalinate with a maximum water recovery level of 50%. Hence, one of the two major drawbacks of RO is its ineffectiveness at operating as an independent technology for brine treatment (Matin et al., 2019).
Multi-Stage Flash
The MSF desalination plant heats the feed saltwater to its evaporation point using condensing vapor from the flash units as well as an external source of heat, the brine heater, which raises the feed temperature to a maximum of 120°C. Some of the feed evaporates and condenses in the feed pre-heat exchangers when the high-temperature feed brine travels through a sequence of reduced vapor pressure and temperature flash components. Consequently, freshwater is created from condensed water vapor, and highly concentrated brine is discharged from the final flash unit stage (Heidary et al., 2018).
MSF has the capacity to generate high-quality freshwater while also requiring little pre−treatment. Another advantage of MSF is that the technology is commercially accessible, allowing it to be enhanced to create freshwater from high-TDS brine. The treatment efficiency of high-TDS brine is limited by the corrosion of the normal stainless-steel types used because of high chlorine environment. Another issue is the high specific energy consumption, which can range from 12.5–24 kWh/m3, making it an energy-intensive technology (Calì et al., 2008).
Hybrid Technologies
A hybrid desalination plant combines two or more desalination methods, such as RO and thermal-based technologies such as MSF or Multiple Effect Distillation (MED). These hybrid desalination plants are particularly common in the Middle East Region (Missimer and Maliva, 2018). When compared to individual desalination plants, hybridization allows the combined desalination plants to operate in a more sustainable and cost-effective manner (Calì et al., 2008; Heidary et al., 2018). The hybrid systems combine the benefits of both plants, such as combining the low specific energy consumption of RO with the ability of MSF to produce high-quality freshwater, allowing the use of energy and water as needed, which brings large savings on maintenance costs (El-Sayed et al., 2000; Marcovecchio et al., 2005; Marcovecchio et al., 2005; Al-hotmani et al., 2019).
In addition to the above, because hybrid desalination systems share the same feed intake and brine discharge systems, combining effluents from both thermal and membrane-based desalination plants lowers the temperature of MSF or MED brines and dilutes the higher-salinity brine of RO (Missimer and Maliva, 2018). Hybrid desalination systems save energy, reduce carbon dioxide emissions, and comply with environmental laws and regulations (Al-hotmani et al., 2019).
When compared to other thermal-based desalination technologies, a combination of multi−effect distillation thermal vapor compression (MED TVC) and RO has the potential to be implemented in the future, because performance of the MED TVC technique has better efficiency due to fewer scaling problems and lower fouling, in addition to functional economic features and reduced requirement for scheduled maintenance (Al-hotmani et al., 2019). Although RO is regarded as a sustainable desalination technology, it has some drawbacks, such as membrane degradation and fouling issues. Combining the freshwater produced by the two desalination technologies will result in an efficient seawater desalination process, because of the high-water recovery and lower energy consumption at a higher production flow rate, as well as the environmental benefit of lowering the salinity and flow rate of the brine produced (Al-hotmani et al., 2019).
The largest MED and RO hybrid complex was built as a second stage in the UAE, as part of the Fujairah project. The MED and RO hybrid desalination system is capable of generating 591,000 m3/day of freshwater; however, the first phase of this project combining the MSF and RO hybrid desalination systems was capable of generating 591,000 m3/day (Esmaeilion, 2020).
Characteristics of Desalination Brine
Physical Characteristics
The quality and amount of the brine are governed by the quality of the feed water, pre-treatment process, desalination process, water recovery rate, and disposal technique (Panagopoulos et al., 2019) (Table 1). Brine effluents released from MSF and RO seawater desalination facilities have physical properties (such as salinity and temperature); cleaning, bio-fouling, scale, and foam control chemicals; coagulants added to remove suspended solids; and pollutants resulting from corrosion, such as heavy metals (Lin et al., 2013). Although RO relies on hydraulic pressure and does not change the temperature of the seawater used, it does have highly complex pre-treatment steps that include antiscalants and coagulants that may change the pH of the water, resulting in brine that is the same temperature as ambient water but with a much higher salinity (Missimer and Maliva, 2018; Frank et al., 2019; Matin et al., 2019).
MSF desalination, on the other hand, relies on evaporating and condensing the input saltwater, resulting in brine effluent with higher temperature and salinity levels, although the salinity is generally significantly lower than with RO (Hashim and Hajjaj, 2005; Bandi et al., 2016).
Chemical and Heavy Metal Characteristics
Desalination brine effluents include significant levels of Cl- and Na+, as well as other ions such as Ca2+, Mg2+, and (Panagopoulos et al., 2019). Several studies have been published on the presence of heavy metals such as copper (Cu) in desalination brine effluents and seawater. MSF, on the other hand, relies on the use of stainless steel with strong corrosion resistance and non-metal equipment. Brine discharges from RO often include metals at trace quantities, such as iron (Fe), nickel (Ni), chromium (Cr), and molybdenum (Mo). Copper contamination is more frequent in MSF effluents and is derived from Cu-based alloys used as components in the distillation process (Lin et al., 2013).
Furthermore, chemicals such as biocides, surface−active agents, anti-scale additives, and solid residues from filter backflushing may be present in the effluent discharge on a continuous or periodic basis, posing a risk to the environment (Frank et al., 2019). Brine effluents from RO desalination plants not only have a high salt content but typically also contain compounds from the desalination process, such as phosphonate-based antiscalants and ferric (or alum) sulphate-based coagulants (Frank et al., 2019).
Factors Influencing the Physiochemical Composition of Brine
Intake Water Quality
The quality and amount of the brine are governed by the quality of the desalinated input water, pre-treatment, the desalination process, and the water recovery rate (Hashim and Hajjaj, 2005) (Figure 1). The amount of brine produced is determined by the capacity of the desalination plant and the water recovery rate, which refers to the percentage of freshwater produced from the total volume of feed water used. As a result, feed water with higher salinity levels will produce more concentrated brine if the water recovery rate remains constant, and thus, better feed water quality will lead to a higher recovery rate (Jones et al., 2019). The brine generated will become more concentrated and lesser as the water recovery rate increases.
The design and operation of seawater RO desalination pre-treatment is highly dependent on the quality of feed water, which has an impact on the quality of brine discharged, as lower-quality feed water with high biological activity necessitates more pre-treatment, which necessitates the use of more chemicals such as anti-fouling agents, chlorine, and acids, which are critical for treatment of the feed water and pipelines, and which ultimately ends up being discharged at a lower quality (Hashim and Hajjaj, 2005; Dupavillon and Gillanders, 2009; Missimer and Maliva, 2018). Membrane biofouling is a major issue for seawater RO desalination plants, as it leads to higher maintenance and operation costs. Because pre-treatment of the feed water is required for an efficient desalination process, using high−quality feed water at the intake stage will reduce the complexity and need for pre-treatment processes.
Surface intake is the most popular method used by seawater RO desalination plants, and typically delivers lower-quality feed water that requires more pre-treatment and is highly affected by seasonal changes, as harmful algal blooms can result in closure of the desalination plant, whereas a sub-surface intake system avoids these problems by using geological media as a natural filter (Dehwah and Missimer, 2016)
Climatic and Seasonal Variation
Due to low precipitation and high evaporation rates, the Arabian Gulf is known for being a shallow sea with extreme water temperatures and salinity levels (Sheppard et al., 2010). Furthermore, the seasonal variation in the Arabian Gulf’s water temperature is high, with temperatures reaching 36°C in the summer and less than 15°C in the winter (Hashim and Hajjaj, 2005; Zhao et al., 2017). The likelihood of biofouling and membrane scaling increases as the temperature of the input water to the saltwater RO desalination plant rises (Al-Bahri et al., 2001; Shaaban and Yahya, 2017).
More chemicals will be needed to pre-treat the feed water in order to reduce biofouling and allow for a more efficient and consistent desalination process. Consequently, the chemicals used in the pre-treatment process will end up in the discharged brine, which will not be treated for these impurities. Because the performance of saltwater reverse osmosis (SWRO) is dependent on permeate flux, salt rejection, and specific power consumption, the optimum temperature and salinity of feed water differs depending on the membrane used in seawater RO desalination plants. Salt rejection decreases as feed water temperature and salinity rise, resulting in lower-quality freshwater and less concentrated brine (Shaaban and Yahya, 2017).
Desalination Techniques
The temperature of brine produced by membrane-based technologies is very similar to that of ambient seawater, whereas brine produced by thermal-based technologies can be 1.37–1.82 times higher (Missimer and Maliva, 2018; Panagopoulos et al., 2019). Furthermore, brine produced by normal seawater RO can contain up to 50% of total dissolved solids, which includes different chemical residues from the pre-treatment and cleaning processes (Ahmad and Baddour, 2014). NaOCl, FeCl3, AlCl3, H2SO4, HCl, and NaHSO3 are commonly used in the pre-treatment phase to treat water to limit algae growth, minimize corrosion, avoid scaling, chlorinate the water, and adjust the pH (Mavukkandy et al., 2019). The water recovery rate is determined by the desalination process and the salinity of the feed water. Because SWRO has a water recovery rate ranging from 40% to 55%, the brine generated will have greater salinity and lower volume as the water recovery rate increases.
Discharge Approach
Surface water discharge, deep well injection, sewage discharge, evaporation ponds, and land applications are some of the ways in which brine from desalination facilities is disposed of inland and into the sea (Mavukkandy et al., 2019). The quality, content, and quantity of brine, as well as the geographical location at the point of discharge, the validity of the choice, and the availability of an acquisition site, all influence the optimal brine disposal strategy (Mansour et al., 2017; Mavukkandy et al., 2019). More than 90% of seawater desalination facilities use the surface water approach to discharge brine into open water bodies (Panagopoulos et al., 2019). This method is commonest owing to the short distance between the desalination plant and the sea. Accordingly, the inland disposal option has become undesirable (Ahmad and Baddour, 2014).
Surface discharge and sub-surface discharge are the two types of brine discharge to open water bodies. The first type discharges brine via an outfall structure very near to the coast, resulting in a build-up of highly concentrated saltwater in that area (Ahmad and Baddour, 2014). In contrast, submerged brine discharge, which is used by the majority of large desalination plants, allows brine to be discharged deeper and further into the mixing zone of the receiving water body via pipes with diffusers or vertical risers embedded at the discharging end. This method allows for better dilution of brine with ambient seawater to reduce salinity, thus minimizing the impact on the marine environment (Ahmad and Baddour, 2014; Mansour et al., 2017; Missimer and Maliva, 2018).
The vertical riser can be used in deep-sea brine discharge. It is positioned vertically and equipped with a nozzle that increases the momentum of the discharged brine, resulting in dilution of the brine with seawater. The brine is discharged vertically with great momentum, causing it to ascend to a certain height then descend due to its negative buoyancy, eventually dispersing around the discharge point on the sea floor (Ahmad and Baddour, 2014; Missimer and Maliva, 2018). Diffusers are commonly used in sub-surface brine discharges, which can include rosette or multi-port diffusers, which are essentially multiple nozzles on the end of the discharge pipe that promote mixing of brine with ambient saltwater in the water column to prevent brine accumulation on the sea bottom (Missimer and Maliva, 2018).
The most common method of brine disposal in the Arabian gulf is disposal back into the sea. Most of the desalination plants are located closer to the sea itself, making it easier to dispose the waste effluent back directly through pipes leading to the sea. Ahmed et al. (2001) investigated brine disposal methods in Oman and UAE. It was found that various disposals were used including sea disposal, small bores and evaporation ponds, located within 200 m of the plant itself. Furthermore, all plants investigated in UAE disposed the brine back into the sea either directly or by disposing into creeks which were linked closely to the sea.
The salinity of brine is reduced by diluting it with regular seawater, municipal wastewater, and cooling water from a neighboring power station prior to disposal (Ahmad and Baddour, 2014; Mansour et al., 2017; Missimer and Maliva, 2018). However, because the temperature and salinity of water discharged from cooling and desalination plants are both higher than that of the receiving seawater, the cooling power plant discharge can float on the surface, although because the desalination discharge is more salinic and heavier, it drags the cooling power plant water with it as it descends. Hence, the entire water column is involved in the salinity and heat dissipation process, which speeds up the dilution of discharged water (Missimer and Maliva, 2018).
Impact of Desalination Brine on Marine Health
In general, anthropogenic activities pollute the coastal marine environment, altering the environment’s physiochemical properties and resulting in changes in marine communities. Physiochemical conditions can be altered by the presence of pollutants, hypoxia, organic enrichment, decreased hydrodynamic conditions, and, more recently, brine discharge (de-la-Ossa-Carretero et al., 2016). Salinity elevation in receiving soil and water bodies and the territorial consequences of brine with high total dissolved solids on benthic marine life close to the discharge site are the most important environmental challenges associated with brine disposal (Miri and Chouikhi, 2005; Panagopoulos et al., 2019).
It is important to note that the production of brine as a by-product of seawater desalination is almost unavoidable, and it is typically released into the marine environment (Meerganz von Medeazza, 2005). The harmfulness of brine to the marine environment can occur as a result of abnormally high salinity, or the occurrence of pollutants that do not naturally exist in the receiving water body. Apart from the high salinity of the brine, other substances that may be present include dangerous pre-treatment chemicals, anti-fouling agents, heavy metals, organics, chlorine, and acids, all of which are important for treatment of the feed water and pipelines.
In addition to the above, before being dumped into the water, these elements are seldom treated to remove their toxicity. Heavy metals and toxic compounds can be harmful to marine creatures, but salinity is the most significant physiochemical influence they face (Dupavillon and Gillanders, 2009). The heavy metals and other harmful chemicals degrades the water quality and local hydrography of the receiving water body, interfering with physiological processes of the biotope such as enzymatic activity, nutrition, photosynthesis, respiration, and reproduction, as well as unnoticed behavioral changes within the ecological community, such as increased stress levels in organisms and increased susceptibility to diseases due to changes in gas solubility and the occurrence of toxic ions (Kenigsberg et al., 2020 and Frank et al., 2017)
Two of the main factors that determine the vulnerability of marine organisms to salinity changes are their ability to regulate osmotic pressure and their mobility. Osmotic regulators, such as most marine fish, can regulate the content of salt within their cells when salinity changes, unlike osmotic conformers; and mobile marine organisms can abandon areas when the osmotic pressure changes. Sessile organisms such as corals and plants, on the other hand, lack this ability and are more susceptible to increased salinity (Dupavillon and Gillanders, 2009; Missimer and Maliva, 2018).
Even though natural marine ingredients are found in desalination brine, disposing of brine without dilution will cause the brine to descend to the sea floor due to its higher density compared to ambient seawater, forming a stratified system whose effects can reach hundreds of meters, thus harming benthic organisms (Dupavillon and Gillanders, 2009). Furthermore, if the effluent brine is not properly mixed before release, the quantity of dissolved oxygen in the water may change (Dupavillon and Gillanders, 2009). Moreover, because the toxicity of chemicals and metals increases as the temperature rises, the discharge of brine with a temperature 30–40°C higher than incoming seawater can have a variety of consequences on marine life (Panagopoulos et al., 2019) (Table 2).
Potential Impacts of the Brine’s Salinity on Fauna (Vertebrate and Invertebrate)
Mobile marine species can adapt to changes in their environment, such as changes in salinity or disruptions in their habitat, by migrating to more suitable sites (Missimer and Maliva, 2018). Many species evade salinities higher than 50 psu according to an experiment conducted by Remaili et al. (2018). Tolerance to high salinity is species-specific, with polychaete and crab species having the highest tolerability (surviving a salinity of 60 psu for an extended period), gastropods and bivalves species showing moderate tolerance, and shrimp, copepod, and amphipod species being the least tolerant Figure 2.
Two fish species and a clam species were used in one of the preliminary experiments to measure the effects of discharged desalination brine on marine organisms. The results showed that there were no effects for salinities under 45 parts per thousand (ppt), but as the salinity increased to 50 ppt, sea bream juveniles were affected within 30 minutes (body coloration darkened) and the first death occurred within 24 hours, whereas juveniles exposed to 70 ppt died after one hour (Iso et al., 1994). When the salinity reached 60 ppt, the hatching of flounder eggs slowed down, and no eggs hatched after the salinity reached 100 ppt.
A study of the toxicity of artificial seawater and desalination brine on embryos and larvae of a euryhaline species, the Japanese medaka, found no difference between the toxicity of the salt water levels. However, as salinity increased to more than 35 ppt, the swim bladder inflation of the larvae decreased, impairing the fish and forcing them to consume more oxygen than larvae with inflated swim bladders (Kupsco et al., 2017). Furthermore, when exposed to salinities greater than 35 ppt, the number of days required for the eggs to hatch rose significantly, reducing larval survival and competitiveness in the wild (Kupsco et al., 2017).
Another study used prepared brine to test the hypersalinity toxicity thresholds for seven marine organisms, including vertebrates and invertebrates, as well as reject brine from the Monterey Bay Aquarium’s (MBA) RO desalination plant, to test three marine species. The results showed that salinity tolerance was species-specific (Voorhees et al., 2013). The euryhaline topsmelt was the most salinity-tolerant species, followed by mysid shrimp and the giant kelp, with mean half maximal effective concentrations (EC50) on the survival of topsmelt and mysid shrimp of 61.9 ppt and 47.8 ppt, respectively, whereas the highest salinity effects occurred in the marine larval development tests, with EC50 for red abalone, purple urchin, and sand dollar of 36.8 ppt, 38.1 ppt and 39.6 ppt, respectively, while the mussel’s larval development EC50 was 43.3 ppt. The EC50 of sand dollar and purple urchin fertilization was 40.3 ppt and 44.2 ppt, respectively. It is worth noting that there were no variations in mussel larval growth of EC50 = 43.3 ppt between prepared brine and brine effluent from the MBA RO desalination facility, showing that salinity was the only factor determining toxicity (Voorhees et al., 2013).
Desalination brine can have double the salinity of seawater, reaching up to 70 ppt. As the salinity increased, the hatching rate, growth, and larval development of the cuttlefish noticeably decreased when compared to ambient seawater, salinities above 40 ppt decreased the embryo’s survival and also caused osmotic stress on the egg (Dupavillon and Gillanders, 2009).
Increased salinity also reduces gas diffusion in the eggs, resulting in smaller eggs and decreased hatching survival. Pathogenic infections also rise with increased salinity (Dupavillon and Gillanders, 2009). Another effect of desalination brine is the concentration of metals and trace elements, which can cause mortality as metals prevent embryos from hatching. Elevated levels of magnesium reduce the activity of newly hatched eggs, inhibiting their movement and defensive mechanisms; and because brine is negatively buoyant and cuttlefish spend a part of their life cycle as benthic organisms, especially as benthic eggs, they are extremely vulnerable to bacterial infections (Dupavillon and Gillanders, 2009).
Brine discharges have resulted in the depletion of fish populations as well as the death of corals and plankton in the Red Sea, while the Ras Hunjurah lagoon in the UAE suffered increased mortality of its mangroves and marine angiosperm, as well as increased pollution caused by inflated copper and nickel levels as a result of brine discharges (Del-Pilar-Ruso et al., 2007). Table 2 shows a summary of all studies conducted in the ME region outlining effects of brine disposal on the various fauna.
Flora (Sea Grass)
Desalination brine discharges have severely impacted Posidonia oceanica seagrass for a prolonged period (Gacia et al., 2007; Ahmad and Baddour, 2014). Seagrass plays a crucial role in the marine ecosystem; meadows of Posidonia oceanica can shelter associated algae, invertebrates and vertebrates in areas of high biodiversity, and contribute to improving water quality, preventing coastal erosion, and regulating biochemical fluxes along the coast (Gacia et al., 2007). Thalassia testudium was unaffected by a salinity increase of up to 4 ppt from an RO desalination discharge, and both Cymodocea nodosa seagrass and Caulerpa prolifera green algae were unaffected by a discharge plume as long as the salinity remained below 37.5 ppt, which is within their natural salinity range (Gacia et al., 2007); whereas a 1–2 ppt increase above ambient salinity of 37–38 ppt caused significant effects on P. oceanica (Sánchez-Lizaso et al., 2008). P. oceanica is sensitive to increased salinity due to brine discharge and was substantially impacted. The plant’s tissues suffered an increase in nitrogen content and reduced glutamine synthetase activity and its health was impaired, particularly in the area next to the discharge where the salinity is higher, the deleterious impacts on the plant’s health being because of a higher occurrence of necrosis, a reduction in total non-structural carbohydrate content, and reduced leaf growth (Gacia et al., 2007; Sánchez-Lizaso et al., 2008). The structure and vitality of P. onceanica were severely affected at a salinity of 39.1 ppt and 38.4 ppt, respectively, and the plant’s mortality considerably increased above 40 ppt, as 50% of P. onceanica died upon the exposure to 45 ppt salinity within 15 days (Sánchez-Lizaso et al., 2008) (Figure 3).
Increased salinity of water can have detrimental consequences for seagrass, including increased morbidity and mortality, decreased root development, highly negative water potentials, an increase in osmoregulators such as amino acids and sucrose, and a drop in potassium and calcium ion concentrations (Cambridge et al., 2017). Increased concentrations of nutrients such as nitrates are another effect of desalination brine discharge, leading to an increase in epiphyte abundance (Gacia et al., 2007).
Microbiome (Bacteria, Archaea, Fungus, Microalgae)
Desalination brine disposal raises the salinity of seawater and introduces associated chemicals into the receiving water body, resulting in the deterioration of marine health and water quality due to changes in environmental physio-chemical characteristics, which alter the ecosystem’s diversity and productivity. Changes in biodiversity, as well as the succession of various species, can further degrade water quality by stimulating the production of detritus (Belkin et al., 2015; Hosseini et al., 2021). Because of its capacity to regulate functional performance, growth rates, and changes in the structure of the bacterial community, salinity is considered to play the most important role in defining the worldwide distribution patterns of bacteria and other microorganisms (Belkin et al., 2015).
A study to determine the toxicity of desalination brine on different marine organisms, including four phytoplankton species (Chlorella vulgaris, Isochrysis galbana, Skeletonema coastatum, and Tetraselmis suecica), found that salinity tolerance varies by species, with the half maximal effect concentration (EC50) of population growth inhibition (PGI) after 72 hours of exposure to C. vulgaris, I. galbana (Yoon and Park, 2011). Another study looked at the impact of desalination brine on the marine microbial community by increasing salinity to up to 5% and 15% of ambient water (41 and 45 ppt, respectively) while taking seasonal changes into account. The experiment was repeated for the spring and summer seasons, using a homogeneous water column in the spring and a thermally stratified water column with a slightly gradual temperature increase in the summer, according to the study report (Belkin et al., 2015). Photosynthesis was suppressed because salt stress deactivates both the Photosystem I (PSI) and Photosystem II (PSII) reaction centers of photosynthesis, as well as preventing new protein synthesis, particularly the D1 protein synthesis in PSII. Furthermore, cyanobacteria’s adaptation to salt stress can take 12 to 24 hours, during which time the cells initiate osmolyte synthesis (Belkin et al., 2015).
Furthermore, within the first two hours of increasing salinity, the indirect proxy of algal biomass, chlorophyll a (Chl a) and photosynthetic pigment was reduced. The reduction in Chl a levels was probably due to the immediate death of salinity-sensitive species of phytoplankton, whereas the heterotrophic organisms’ response resulted in increased bacterial productivity, as a result of higher use of organic carbon by bacteria to endure the changing osmotic stress of cells (Belkin et al., 2015). Over the course of the studies, there were two distinct reactions of the microbial community: The spring−driven experiment had a small change in community composition with strong metabolic activity in response to increased salinity, whereas the summer-driven experiment had a structural shift (Belkin et al., 2015). A field study was conducted in three seasons (spring, summer, and winter) to determine the effects of desalination brine diluted with power plant cooling water on a microbial community. Increased salinity and temperature of the impacted receiving water body resulted in a 60% reduction in average Chl a concentration during spring and winter, and a 32% reduction in average Chl a concentration during summer, as well as a reduction in total cell numbers compared to ambient waters, with impacted areas having 3.8, 2, and 6 times lower cell numbers during spring, summer, and winter, respectively (Drami et al., 2011).
When compared to the control values of 4.41 1.4, 4.79 0.85, and 5.53 0.37 mg cm-3 h-1 during spring, summer, and winter, respectively, the average primary production rates in the affected region were 0.60 0.56, 1.84 0.67, and 1.43 0.31 mg cm-3h-1 during spring, summer, and winter, respectively. Similarly, during spring and winter, the average rates of bacterial production were significantly lower at the impacted area; 0.03 0.03 mg cm-3 h-1 for both seasons, compared to control values of 0.19 0.10 mg cm-3 h-1 in spring and 0.46 0.05 mg cm-3 h-1 in winter, while summer values showed an increase in bacterial production rate from 0.58 0.25 mg cm-3 h-1 in the control area to 1.64 0.73 mg cm-3 h-1 in the affected region (Drami et al., 2011).
Brine Management Strategies
Brine Management and Zero Liquid Discharge Technology
Disposal of desalination brine and reduction of its associated environmental effects are two of the focal issues of new technologies (Mansour et al., 2017) (Figure 4). As a result, appropriate and efficient actions must be taken to ensure the safe disposal of desalination brine and its related toxic substances that occur in the brine from desalination processes (Loutatidou et al., 2017). In modern-day brine management, two basic techniques are used: The first is volume reduction, which aims to retrieve additional amounts of water equal to or beyond the saturation concentration, and the second is zero liquid discharge or crystallization approaches, which aims to retrieve water and various salts from the highly concentrated brine (Mavukkandy et al., 2019).
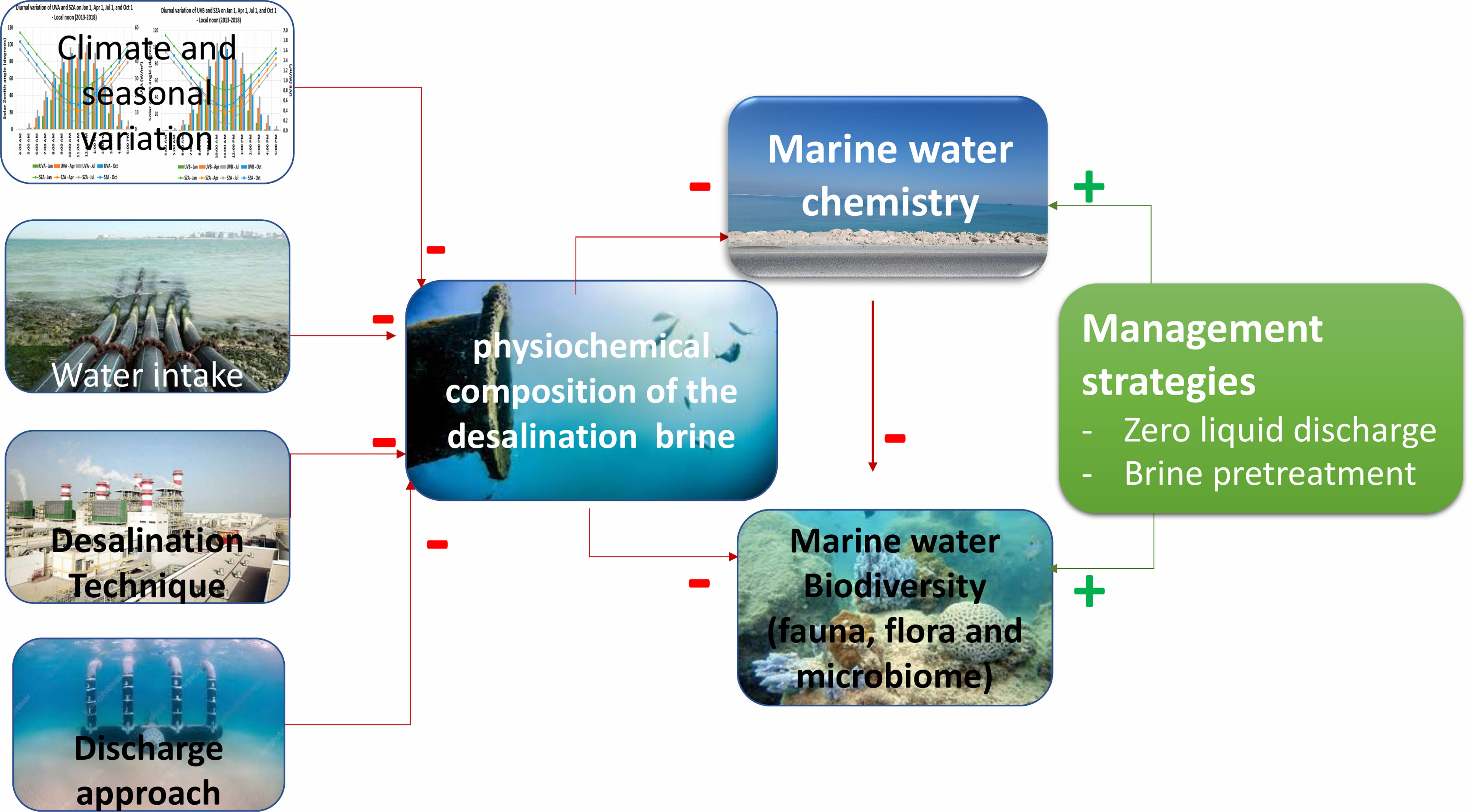
Figure 4 Overview of the factors influencing the desalination brine characteristics, and brine impacts and management strategies.
Zero liquid discharge is defined as a synthesis of desalination technologies that aims to generate high-quality water without producing any liquid waste at all. These processes achieve a water recovery rate of 95–99%, and the water produced is very pure and can be used for a variety of purposes including drinking and irrigation. In addition to the production of water, the solutes are compressed into their solid state, which can be processed further to produce a useful substance (Muhammad and Lee, 2019).
There is no universal zero liquid discharge system to be adopted by all desalination plants because zero liquid discharge systems differ in their design, arrangement, and operation, making each system unique. However, a standard zero liquid discharge system consists of three main stages, the first of which is pre-concentration, in which membrane-based technologies recover water and reduce brine volume. Various methods can extract resources from brine, including those that work alone or as part of a hybrid compound of processes (Mavukkandy et al., 2019).
Membrane-Based Technologies
As previously stated, RO is one of the most commonly used desalination technologies, capable of treating feed water with TDS of up to 70,000 mg/L. However, an enhanced HPRO technology exists that is capable of treating the brine from a typical RO desalination plant with TDS of up to 130,000 mg/L with 50% water recovery, though it does face challenges (Jenkins et al., 2012; Anis et al., 2019; Mavukkandy et al., 2019).
Forward osmosis differs from RO in that it is driven by osmotic pressure gradients, in which an extremely highly concentrated solution, also known as “draw solution”, is used against the feed water to generate osmotic pressure, forcing the water molecules of the less concentrated feed water to pass through the membrane, eventually removing the freshly produced water from the highly concentrated feed water (Maliva and Missimer, 2013; Mansour et al., 2017).
Membrane distillation (MD) is a membrane-based technology, the concept of which relies on a micro-porous hydrophobic membrane that allows water vapour to pass through while restricting the liquid water. This process is powered by vapour pressure, which is generated by a temperature difference across the membrane (Mavukkandy et al., 2019). The other side of the membrane condenses the water vapour to produce freshwater as a cold distillate (Panagopoulos et al., 2019).
Conclusions
The physicochemical qualities of desalination brine are influenced by a number of factors, including the quality of the input water, desalination processes, and discharge methods. These features determine how it affects the chemistry and health of the ocean. The tolerance of marine species to the impacts of salt varies. Several brine management solutions have been developed lately, with increasing water recovery to eventually achieve zero-liquid discharge being the most promising strategy for reducing the harmful impact on marine health.
Author Contributions
MO: writing – original draft, visualization. HAJ: conceptualization, writing – review and editing; SAS: writing – review and editing; IS: supervision, conceptualization, writing – review and editing, project administration, funding acquisition. All authors contributed to the article and approved the submitted version.
Funding
This report was made possible by the NPRP award [NPRP11S-0110-180248] from the Qatar National Research Fund (a member of The Qatar Foundation). The statements made herein are solely the responsibility of the authors.
Conflict of Interest
The authors declare that the research was conducted in the absence of any commercial or financial relationships that could be construed as a potential conflict of interest.
Publisher’s Note
All claims expressed in this article are solely those of the authors and do not necessarily represent those of their affiliated organizations, or those of the publisher, the editors and the reviewers. Any product that may be evaluated in this article, or claim that may be made by its manufacturer, is not guaranteed or endorsed by the publisher.
Acknowledgments
Special thanks are due to the Centre for Sustainable Development for their support.
References
Ahmad N., Baddour R. E. (2014). A Review of Sources, Effects, Disposal Methods, and Regulations of Brine Into Marine Environments. Ocean Coast. Manage. 87, 1–7. doi: 10.1016/j.ocecoaman.2013.10.020
Ahmed M., Shayya W. H., Hoey D., Al Handaly J. (2001). Brine Disposal From Reverse Osmosis Desalination Plants in Oman and United Arab Emirates. Desalination 133, 135–147. doi: 10.1016/S0011-9164(01)80004-7
Al-Bahri Z. K., Hanbury W. T., Hodgkiess T. (2001). Optimum Feed Temperatures for Seawater Reverse Osmosis Plant Operation in an MSF/SWRO Hybrid Plant. Desalination 138, 335–339. doi: 10.1016/S0011-9164(01)00282-X
Al-Barwani H. H., Purnama A. (2019). “The Hypersaline Gulf: A Model Study of Potential Impacts of Seawater Desalination on the Marine Environment,” in The Marine Environment: Ecology, Management and Conservation. Ed. Nemeth A. D. (Nova Science Publisher), pp.167–pp.177.
Al-hotmani O. M. A., Al-Obaidi M. A., Patel R., Mujtaba I. M. (2019). Performance Analysis of a Hybrid System of Multi Effect Distillation and Permeate Reprocessing Reverse Osmosis Processes for Seawater Desalination. Desalination 470, 114066. doi: 10.1016/j.desal.2019.07.006
Al-Kaabi A. H., Mackey H. R. (2019). Environmental Assessment of Intake Alternatives for Seawater Reverse Osmosis in the Arabian Gulf. J. Environ. Manage. 242, 22–30. doi: 10.1016/j.jenvman.2019.04.051
Anis S. F., Hashaikeh R., Hilal N. (2019). Reverse Osmosis Pretreatment Technologies and Future Trends: A Comprehensive Review. Desalination 452, 159–195. doi: 10.1016/j.desal.2018.11.006
Bandi C. S., Uppaluri R., Kumar A. (2016). Global Optimization of MSF Seawater Desalination Processes. Desalination 394, 30–43. doi: 10.1016/j.desal.2016.04.012
Belatoui A., Bouabessalam H., Hacene O. R., de-la-Ossa-Carretero J. A., Martinez-Garcia E., Sanchez-Lizaso J. L. (2017). Environmental Effects of Brine Discharge From Two Desalinations Plants in Algeria (South Western Mediterranean). Desalination And Water Treat 76, 311–318. doi: 10.5004/dwt.2017.20812
Belkin N., Rahav E., Elifantz H., Kress N., Berman-Frank I. (2015). Enhanced Salinities, as a Proxy of Seawater Desalination Discharges, Impact Coastal Microbial Communities of the Eastern Mediterranean Sea. Environ. Microbiol. 17, 4105–4120. doi: 10.1111/1462-2920.12979
Belkin N., Rahav E., Elifantz H., Kress N., Berman-Frank I. (2017). The Effect of Coagulants and Antiscalants Discharged With Seawater Desalination Brines on Coastal Microbial Communities: A Laboratory and In Situ Study From the Southeastern Mediterranean. Water Res. 110, 321–331. doi: 10.1016/j.watres.2016.12.013
Bello A. S., Zouari N., Da’ana D. A., Hahladakis J. N., Al-Ghouti M. A. (2021). An Overview of Brine Management: Emerging Desalination Technologies, Life Cycle Assessment, and Metal Recovery Methodologies. J.Env. Manage. 288, 112358. doi: 10.1016/j.jenvman.2021.112358
Benaissa M., Rouane-Hacene O., Boutiba Z., Habib D., Guibbolini-Sabatier M. E., Risso-De Faverney C. (2020). Ecotoxicological Effects Assessment of Brine Discharge From Desalination Reverse Osmosis Plant in Algeria (South Western Mediterranean). Reg. Stud. Marine Sci. 39, 101407. doi: 10.1016/j.rsma.2020.101407
Blanco-Marigorta A. M., Lozano-Medina A., Marcos J. D. (2017). A Critical Review of Definitions for Exergetic Efficiency in Reverse Osmosis Desalination Plants. Energy 137, 752–760. doi: 10.1016/j.energy.2017.05.136
Calì G., Fois E., Lallai A., Mura G. (2008). Optimal Design of a Hybrid RO/MSF Desalination System in a non-OPEC Country. Desalination 228, 114–127. doi: 10.1016/j.desal.2007.08.012
Cambridge M. L., Zavala-Perez A., Cawthray G. R., Mondon J., Kendrick G. A. (2017). Effects of High Salinity From Desalination Brine on Growth, Photosynthesis, Water Relations and Osmolyte Concentrations of Seagrass Posidonia Australis. Mar. Pollut. Bull. 115, 252–260. doi: 10.1016/j.marpolbul.2016.11.066
Dawoud M. A. (2012). Environmental Impacts of Seawater Desalination: Arabian Gulf Case Study. Int. J. Environ. Sustain. 1, 22–37. doi: 10.24102/ijes.v1i3.96
Dehwah A. H. A., Missimer T. M. (2016). Subsurface Intake Systems: Green Choice for Improving Feed Water Quality at SWRO Desalination Plants, Jeddah, Saudi Arabia. Water Res. 88, 216–224. doi: 10.1016/j.watres.2015.10.011
de-la-Ossa-Carretero J. A., Del-Pilar-Ruso Y., Loya-Fernández A., Ferrero-Vicente L. M., Marco-Méndez C., Martinez-Garcia E., et al. (2016). Response of Amphipod Assemblages to Desalination Brine Discharge: Impact and Recovery. Estuar. Coast. Shelf Sci. 172, 13–23. doi: 10.1016/j.ecss.2016.01.035
Del-Pilar-Ruso Y., la Ossa Carretero J. A. D., Casalduero F. G., Lizaso J. L. S. (2007). Spatial and Temporal Changes in Infaunal Communities Inhabiting Soft-Bottoms Affected by Brine Discharge. Mar. Environ. Res. 64, 492–503. doi: 10.1016/j.marenvres.2007.04.003
Drami D., Yacobi Y. Z., Stambler N., Kress N. (2011). Seawater Quality and Microbial Communities at a Desalination Plant Marine OutfallA Field Study at the Israeli Mediterranean Coast. Water Res. 45, 5449–5462. doi: 10.1016/j.watres.2011.08.005
Dupavillon J. L., Gillanders B. M. (2009). Impacts of Seawater Desalination on the Giant Australian Cuttlefish Sepia Apama in the Upper Spencer Gulf, South Australia. Mar. Environ. Res. 67, 207–218. doi: 10.1016/j.marenvres.2009.02.002
El-Sayed E., Abdel-Jawad M., Ebrahim S., Al-Saffar A. (2000). Performance Evaluation of Two RO Membrane Configurations in a MSF/RO Hybrid System. Desalination 128, 231–245. doi: 10.1016/S0011-9164(00)00038-2
Esmaeilion F. (2020). Hybrid Renewable Energy Systems for Desalination, Applied Water Science (Springer International Publishing). doi: 10.1007/s13201-020-1168-5
Frank H., Fussmann K. E., Rahav E., Bar Zeev E. (2019). Chronic Effects of Brine Discharge Form Large-Scale Seawater Reverse Osmosis Desalination Facilities on Benthic Bacteria. Water Res. 151, 478–487. doi: 10.1016/j.watres.2018.12.046
Frank H., Rahav E., Bar-Zeev E. (2017). Short-Term Effects of SWRO Desalination Brine on Benthic Heterotrophic Microbial Communities. Desalination 417, 52–59. doi: 10.1016/j.desal.2017.04.031
Gacia E., Invers O., Manzanera M., Ballesteros E., Romero J. (2007). Impact of the Brine From a Desalination Plant on a Shallow Seagrass (Posidonia Oceanica) Meadow. Estuar. Coast. Shelf Sci. 72, 579–590. doi: 10.1016/j.ecss.2006.11.021
Hashim A., Hajjaj M. (2005). Impact of Desalination Plants Fluid Effluents on the Integrity of Seawater, With the Arabian Gulf in Perspective. Desalination 182, 373–393. doi: 10.1016/j.desal.2005.04.020
Heidary B., Hashjin T. T., Ghobadian B., Roshandel R. (2018). Optimal Integration of Small Scale Hybrid Solar Wind RO-MSF Desalination System. Renew. Energy Focus 27, 120–134. doi: 10.1016/j.ref.2018.05.003
Hosseini H., Saadaoui I., Moheimani N., Al Saidi M., Al Jamali F., Al Jabri H., et al. (2021). Marine Health of the Arabian Gulf: Drivers of Pollution and Assessment Approaches Focusing on Desalination Activities. Mar. Pollut. Bull. 164 p.112085. doi: 10.1016/j.marpolbul.2021.112085
Iso S., Suizu S., Maejima A. (1994). The Lethal Effect of Hypertonic Solutions and Avoidance of Marine Organisms in Relation to Discharged Brine From a Destination Plant. Desalination 97, 389–399. doi: 10.1016/0011-9164(94)00102-2
Jenkins S., Paduan J., Roberts P., Schlenk D., Weis J. (2012). “Management of Brine Discharges to Coastal Waters: Recommendations of a Science Advisory Panel. Technical report 694,” in Submitt. Req. State Water Resour (Costa Mesa: Control Board 101).
Jones E., Qadir M., van Vliet M. T. H., Smakhtin V., Kang S. (2019). The State of Desalination and Brine Production: A Global Outlook. Sci. Total Environ. 657, 1343–1356. doi: 10.1016/j.scitotenv.2018.12.076
Kenigsberg C., Abramovich S., Hyams-Kaphzan O. (2020). The Effect of Long-Term Brine Discharge From Desalination Plants on Benthic Foraminifera. PloS One 15 (1), e0227589. doi: 10.1371/journal.pone.0227589
Kress N., Gertner Y., Shoham-Frider E. (2020). Seawater Quality at the Brine Discharge Site From Two Mega Size Seawater Reverse Osmosis Desalination Plants in Israel (Eastern Mediterranean). Water Res. 171, 115402. doi: 10.1016/j.watres.2019.115402
Kupsco A., Sikder R., Schlenk D. (2017). Comparative Developmental Toxicity of Desalination Brine and Sulfate-Dominated Saltwater in a Euryhaline Fish. Arch. Environ. Contam. Toxicol. 72, 294–302. doi: 10.1007/s00244-016-0354-9
Lazarides H. N., Katsanidis E. (2003). “MEMBRANE TECHNIQUES | Principles of Reverse Osmosis,” in Encyclopedia of Food Sciences and Nutrition, 2nd ed. (Academic Press), 3827–3833.
Lin Y. C., Chang-Chien G. P., Chiang P. C., Chen W. H., Lin Y. C. (2013). Potential Impacts of Discharges From Seawater Reverse Osmosis on Taiwan Marine Environment. Desalination 322, 84–93. doi: 10.1016/j.desal.2013.05.009
Loutatidou S., Mavukkandy M. O., Chakraborty S., Arafat H. A. (2017). Introduction: What is Sustainable Desalination?, Desalination Sustainability: A Technical, Socioeconomic, and Environmental Approach (Amsterdam: Elsevier Inc). doi: 10.1016/B978-0-12-809791-5.00001-8
Mabrook B. (1994). Environmental Impact of Waste Brine Disposal of Desalination Plants, Red Sea, Egypt. Desalination 97 (1–3), 453–465. doi: 10.1016/0011-9164(94)00108-1
Maliva R., Missimer T. (2013). Arid Lands Water Evaluation and Management, Choice Reviews Online, Environmental Science and Engineering 434, 198–215. (Berlin, Heidelberg: Springer Berlin Heidelberg). doi: 10.5860/choice.50-4453
Mansour S., Arafat H. A., Hasan S. W. (2017). Brine Management in Desalination Plants, Desalination Sustainability: A Technical, Socioeconomic, and Environmental Approach (Amsterdam: Elsevier Inc). doi: 10.1016/B978-0-12-809791-5.00005-5
Marcovecchio M. G., Mussati S. F., Aguirre P. A., Scenna N. J. (2005). Optimization of Hybrid Desalination Processes Including Multi Stage Flash and Reverse Osmosis Systems. Desalination 182, 111–122. doi: 10.1016/j.desal.2005.03.011
Matin A., Rahman F., Shafi H. Z., Zubair S. M. (2019). Scaling of Reverse Osmosis Membranes Used in Water Desalination: Phenomena, Impact, and Control; Future Directions. Desalination 455, 135–157. doi: 10.1016/j.desal.2018.12.009
Mavukkandy M. O., Chabib C. M., Mustafa I., Al Ghaferi A., AlMarzooqi F. (2019). Brine Management in Desalination Industry: From Waste to Resources Generation. Desalination 472, 114187. doi: 10.1016/j.desal.2019.114187
Meerganz von Medeazza G. L. (2005). Directand Socially-Induced Environmental Impacts of Desalination. Desalination 185, 57–70. doi: 10.1016/j.desal.2005.03.071
Miri R., Chouikhi A. (2005). Ecotoxicological Marine Impacts From Seawater Desalination Plants. Desalination 182, 403–410. doi: 10.1016/j.desal.2005.02.034
Missimer T. M., Maliva R. G. (2018). Environmental Issues in Seawater Reverse Osmosis Desalination: Intakes and Outfalls. Desalination 434, 198–215. doi: 10.1016/j.desal.2017.07.012
Muhammad Y., Lee W. (2019). Zero-Liquid Discharge (ZLD) Technology for Resource Recovery From Wastewater: A Review. Sci. Total Environ. 681, 551–563. doi: 10.1016/j.scitotenv.2019.05.062
Nasr H., Yousef M., Madkour H. (2019). Impacts of Discharge of Desalination Plants on Marine Environment at the Southern Part of the Egyptian Red Sea Coast (Case Study). Int. J. Ecotoxicol. Ecobiol. 4 (3), 66. doi: 10.11648/j.ijee.20190403.12
Panagopoulos A., Haralambous K. J., Loizidou M. (2019). Desalination Brine Disposal Methods and Treatment Technologies - A Review. Sci. Total Environ. 693, 133545. doi: 10.1016/j.scitotenv.2019.07.351
Petersen K. L., Paytan A., Rahav E., Levy O., Silverman J., Barzel O., et al. (2018). Impact of Brine and Antiscalants on Reef-Building Corals in the Gulf of Aqaba – Potential Effects From Desalination Plants. Water Res. 144, 183–191. doi: 10.1016/j.watres.2018.07.009
Remaili T. M., Simpson S. L., Bennett W. W., King J. J., Mosley L. M., Welsh D. T., et al. (2018). Assisted Natural Recovery of Hypersaline Sediments: Salinity Thresholds for the Establishment of a Community of Bioturbating Organisms. Environ. Sci. Process. Impacts 20, 1244–1253. doi: 10.1039/c8em00092a
Sánchez-Lizaso J. L., Romero J., Ruiz J., Gacia E., Buceta J. L., Invers O., et al. (2008). Salinity Tolerance of the Mediterranean Seagrass Posidonia Oceanica: Recommendations to Minimize the Impact of Brine Discharges From Desalination Plants. Desalination 221, 602–607. doi: 10.1016/j.desal.2007.01.119
Shaaban S., Yahya H. (2017). Detailed Analysis of Reverse Osmosis Systems in Hot Climate Conditions. Desalination 423, 41–51. doi: 10.1016/j.desal.2017.09.002
Sheppard C., Al-Husiani M., Al-Jamali F., Al-Yamani F., Baldwin R., Bishop J., et al. (2010). The Gulf: A Young Sea in Decline. Mar. Pollut. Bull. 60, 13–38. doi: 10.1016/j.marpolbul.2009.10.017
Voorhees J. P., Phillips B. M., Anderson B. S., Siegler K., Katz S., Jennings L., et al. (2013). Hypersalinity Toxicity Thresholds for Nine California Ocean Plan Toxicity Test Protocols. Arch. Environ. Contam. Toxicol. 65, 665–670. doi: 10.1007/s00244-013-9931-3
Yoon S. J., Park G. S. (2011). Ecotoxicological Effects of Brine Discharge on Marine Community by Seawater Desalination. Desalin. Water Treat. 33, 240–247. doi: 10.5004/dwt.2011.2644
Keywords: brine composition, brine management, desalination, marine health, seawater chemistry
Citation: Omerspahic M, Al-Jabri H, Siddiqui SA and Saadaoui I (2022) Characteristics of Desalination Brine and Its Impacts on Marine Chemistry and Health, With Emphasis on the Persian/Arabian Gulf: A Review. Front. Mar. Sci. 9:845113. doi: 10.3389/fmars.2022.845113
Received: 29 December 2021; Accepted: 22 March 2022;
Published: 26 April 2022.
Edited by:
Rathinam Arthur James, Bharathidasan University, Trichy, IndiaReviewed by:
Satheeswaran Thangaraj, Incheon National University, South KoreaSabarathinam Chidambaram, Kuwait Institute for Scientific Research, Kuwait
Copyright © 2022 Omerspahic, Al-Jabri, Siddiqui and Saadaoui. This is an open-access article distributed under the terms of the Creative Commons Attribution License (CC BY). The use, distribution or reproduction in other forums is permitted, provided the original author(s) and the copyright owner(s) are credited and that the original publication in this journal is cited, in accordance with accepted academic practice. No use, distribution or reproduction is permitted which does not comply with these terms.
*Correspondence: Imen Saadaoui, aW1lbi5zYWFkYW91aUBxdS5lZHUucWE=