- 1Energy and Environment Department, Center for American Progress, Washington, DC, United States
- 2Department of Earth and Environmental Science, University of Pennsylvania, Philadelphia, PA, United States
- 3GreenCollar, International Projects, Chicago, IL, United States
- 4Environment Department, The Pew Charitable Trusts, Washington, DC, United States
- 5School of Oceanography, University of Washington, Seattle, WA, United States
- 6Department of Strategy and International Affairs, CREAF (Centre for Research on Ecology and Forestry Applications), Barcelona, Spain
- 7Integrative Ecophysiology, Alfred Wegener Institute, Bremerhaven, Germany
The ocean is gaining prominence in climate change policy circles as a tool for addressing the climate crisis. Blue carbon, the carbon captured and stored by marine and coastal ecosystems and species, offers potential as a “nature-based solution” to climate change. The protection and restoration of specific ocean ecosystems can form part of a climate response within climate mitigation policies such as Nationally Determined Contributions under the United Nations Framework Convention on Climate Change. For mitigation policies that seek to implement management actions that drawdown carbon, ecosystem sequestration and emissions must be measurable across temporal and spatial scales, and management must be practical leading to improved sequestration and avoided emissions. However, some blue carbon interventions may not be suitable as a climate mitigation response and better suited for other policy instruments such as those targeted toward biodiversity conservation. This paper gives context to numerous blue carbon sequestration pathways, quantifying their potential to sequester carbon from the atmosphere, and comparing these sequestration pathways to point-source emissions reductions. The applicability of blue carbon is then discussed in terms of multiple international policy frameworks, to help individuals and institutions utilize the appropriate framework to reach ocean conservation and climate mitigation goals.
Introduction
Climate change is the preeminent policy and scientific challenge of this decade. Increasingly dire warnings are being sounded regarding systemic changes across the planet from rising sea levels, melting glaciers, shrinking sea ice, climate-related weather extremes, and irreversible loss of marine ecosystems such as coral reefs (IPCC, 2019a; DeConto et al., 2021). The urgency to deeply reduce greenhouse gas (GHG) emissions and to adapt to a warmer world is overwhelmingly evident in the most recent Intergovernmental Panel on Climate Change (IPCC) Sixth Assessment Reports (IPCC, 2021; IPCC, 2022), which reinforces that humans have unequivocally altered the atmosphere, land, and ocean. Efforts to limit the global temperature increase to 1.5°C above pre-industrial levels – a goal laid out by the Paris Agreement and reinforced by the Glasgow Climate Pact – require a global net anthropogenic CO2 emissions decline of about 45% from the 2010 level by 2030 and to net zero by 2050 (IPCC, 2018). The urgency of the climate crisis situates significant climate mitigation and adaptation actions very squarely within not only our own lifetimes, but within many institutions’ short-term planning horizons.
Long overlooked, the role of the ocean is receiving increasing focus within the climate policy landscape and there is growing interest in bringing ocean-related policy actions forward as climate solutions (Hoegh-Guldberg et al., 2019b). The release of the IPCC Special Report on the Ocean and Cryosphere in a Changing Climate (IPCC, 2019a), followed closely thereafter by the Chilean Presidency’s focus on oceans at the United Nations Framework Convention on Climate Change (UNFCCC) 25th Conference of Parties, which was dubbed the “Blue COP”, has created buzz around utilizing the ocean as a mitigation tool. The ocean and climate change dialogue convened in 2021 also considered how ocean-based climate solutions can strengthen the global climate response (SBSTA, 2021). These discussions include lowering sector-specific emissions from ocean industries like shipping and advancing clean energy options such as offshore wind, wave and tidal energy, and floating solar, providing clear examples of how countries can utilize ocean resources to reduce emissions (Hoegh-Guldberg et al., 2019a).
Employing ‘nature-based solutions’ can also expand our climate response to include natural systems more fully (IUCN, 2020). The management of blue carbon ecosystems – open ocean and coastal ecosystems that capture and measurably store carbon in the long term – has the potential to address both climate mitigation and adaptation challenges. However, these ecosystems are not equal in terms of their mitigation potential or readiness for inclusion within climate mitigation policies. Recent headline-grabbing reports on the promise of blue carbon provide limited context with regards to additionality, a key factor defined as the carbon sequestered and stored, and/or emissions avoided (i.e., net emission reductions), as a result of management or policy intervention beyond what occurs without that intervention. Further context regarding the true climate impact of interventions developed to protect, enhance, or restore marine ecosystems, and the associated timeline to achieve measurable climate benefits, is also needed.
It is our goal within this paper to put these ‘blue carbon solutions’ into the broader climate context, communicating disparate data found across disciplines and providing subsequent policy recommendations in a simplified format. Although review papers exist for some of the processes and ecosystems discussed below, this review fills a gap in the literature, identified by practitioners in the ocean-climate field, for a comprehensive discussion of blue carbon in the present scientific and policy context and clarification of the magnitude and applicability of these ‘solutions’ in climate and conservation frameworks.
We synthesized the recent state of the knowledge for blue carbon and emergent topics, both from a science and policy perspective, and identified challenges regarding measuring, comparing, and communicating blue carbon solutions across the authors’ fields in private, government, academic, and non-governmental organization sectors. The authors used a series of keywords to identify relevant literature; recent articles were prioritized, and where the state of the knowledge was well-established, other review articles were cited. The authors purposely did not cover all the blue carbon scientific literature in depth, as this would be beyond the scope of the review. Additionally, the manuscript was reviewed by practitioners in the ocean-climate field to identify any gaps in the synthesis or points for clarification, and in a second drafting period, information from the IPCC, 2022 report was added.
To compare the mitigation potential across blue carbon solutions, we standardized units of measurement, using multiples of tons of carbon as the main unit of stock throughout the paper. Megatons (MtC) and gigatons (GtC) is used depending on relevant scale, GtC per year and MtC per year were used for fluxes. While petagrams of carbon (PgC) are often used in the scientific literature for global carbon stocks, using GtC and MtC enable comparison to emissions sources and match metrics more commonly used in policy and communications for the public.
In this review, we first give a high-level overview of the biological and solubility pumps that drive carbon sequestration in the ocean, discuss connections between these systems, and provide context to the limiting factors in both processes. We then review the existing data for open ocean and coastal ecosystems and species noted for their potential contribution to climate mitigation. Lastly, we highlight the inclusion criteria for current mitigation policies and identify policy instruments outside of the mitigation context through which scientists, advocates, and policymakers can leverage their findings and deliver tangible climate and conservation gains.
Carbon Storage and Fluxes in the Ocean
Due to anthropogenic-driven emissions, atmospheric CO2 is increasing, influencing the fluxes of carbon among land, ocean, and atmosphere reservoirs. Some processes, whether natural or anthropogenic, remove atmospheric carbon and store it in a pool outside of the atmosphere long enough to be considered ‘sequestration’. Throughout this review, ‘carbon flux’ is used to refer to the movement of carbon from one carbon pool to another (Table 1).
Biological and Solubility Pumps in the Open Ocean
As the major global carbon reservoir, the ocean stores carbon in four primary pools: dissolved inorganic carbon (38,000 Gt C), organic carbon (700 Gt C, mostly dissolved organic carbon, excluding living marine biota and sediment floor), marine biota (6 Gt C) (Bar-On et al., 2018), and the sediment floor (2,322 Gt C in the top first meter) (Bar-On et al., 2018; Atwood et al., 2020; Friedlingstein et al., 2020) (Table 2 and Figure 2). The first is processed through what is known as the solubility pump, and the latter three through the biological pump, with both pumps interacting with each other.
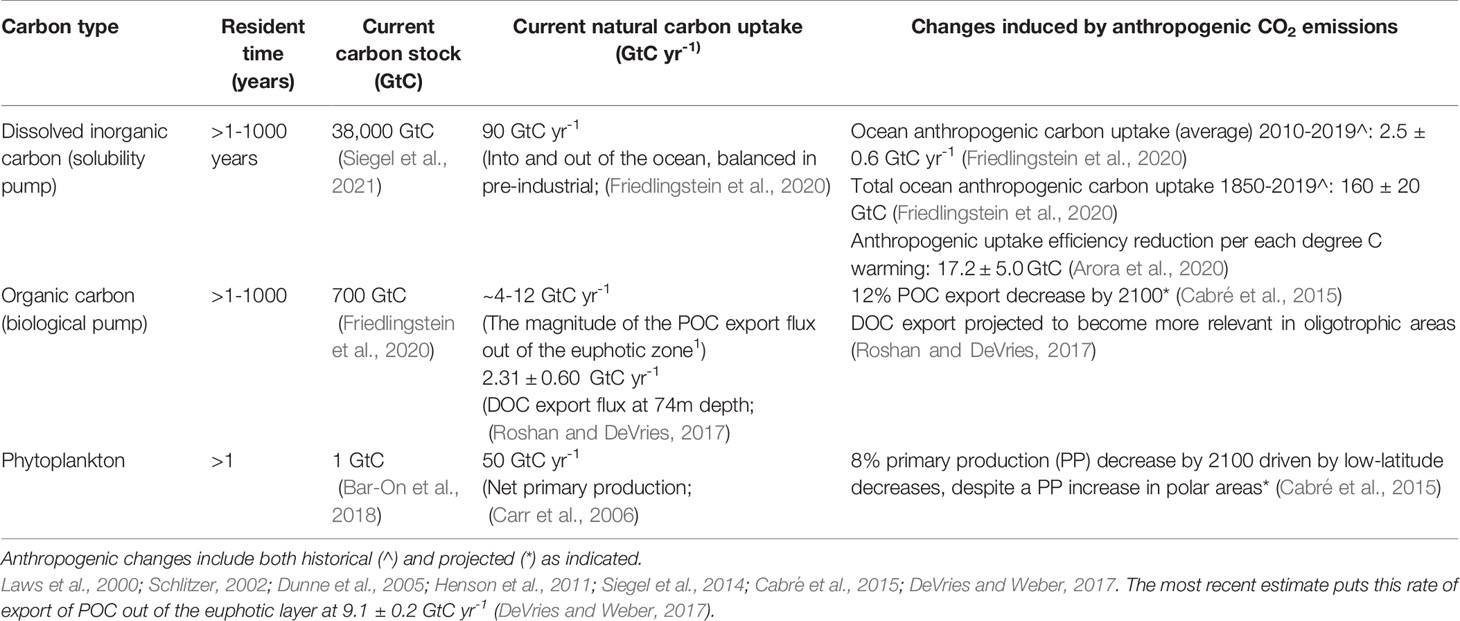
Table 2 Open ocean carbon stocks and sequestration through solubility and biological pumps. Resident time refers to the time that carbon stays in the ocean and out of the atmosphere.
The Solubility Pump
Through the solubility pump the ocean physically uptakes atmospheric CO2 and chemically converts it into dissolved inorganic carbon that is transported to the deep ocean via mixing and currents. As part of the natural pre-industrial carbon cycle, the solubility pump was in equilibrium with the atmosphere (the same amount of CO2 enters and goes out). However, anthropogenic emissions beginning in the industrial period have modified this equilibrium; for every 1 part per million (ppm) increase of atmospheric CO2, the ocean uptakes an extra 0.97 ± 0.40 GtC, comparable to the extra induced CO2 uptake by land vegetation via photosynthesis (Arora et al., 2020). Note, however, that this increased carbon sequestration through the anthropogenically-forced solubility pump is not considered additional sequestration as it has occurred naturally with no human intentional mitigation intervention. Without the solubility pump, atmospheric CO2 levels, which reached 414 ppm in 2020, would be 75 ppm higher (Arico et al., 2021). Via the solubility pump, the ocean has thus provided a buffer for human-caused climate change, absorbing 25% of all the human-induced carbon emissions over 1850-2019 (160 ± 20 Gt C) (Friedlingstein et al., 2020) (Table 2). Another 33% of anthropogenic carbon emissions were taken up by land vegetation, while 42% accumulated in the atmosphere (Figure 2). The ocean is thus taking up more carbon now than in the pre-industrial period, but this ocean sink is projected to become less effective with increasing cumulative CO2 emissions (Arora et al., 2020), which effectively translates into greater atmospheric CO2 (Canadell et al., 2021).
The efficiency of the solubility pump varies with physical factors such as pH, temperature, and ocean circulation. As atmospheric CO2 enters the ocean, ocean pH decreases. This causes more dissolved inorganic carbon to exist in the form of aqueous CO2, thus limiting the ocean’s ability to absorb more atmospheric CO2 while simultaneously leading to ocean acidification. It is estimated that ocean acidification has reduced the pH of the ocean by 0.1 units since the pre-industrial period, with negative consequences for corals and calcifying organisms, as well as the wider marine ecosystem (IPCC, 2021). The ocean has also taken up 91% of anthropogenic heat (IPCC, 2021), increasing ocean temperatures and reducing the solubility of CO2, as water’s capacity to hold dissolved gasses decreases with increasing water temperatures (McKinley et al., 2011). Faster warming of the surface ocean has also enhanced water column stratification, hindering the mixing and transport of CO2 from the surface to the deep ocean and therefore limiting solubility pump efficiency. Due to these factors, the solubility pump is decreasing by a rate of 17.2 ± 5.0 Gt C per °C of increased global near surface air temperature since pre-industrial times (Arora et al., 2020).
The Biological Pump
The ocean also absorbs carbon through the biological pump, in which phytoplankton photosynthesis transforms CO2 into organic matter (i.e., biomass) that is then exported from surface waters to the deep ocean. Though phytoplankton (~1 Gt C standing biomass) (Bar-On et al., 2018) account for less than 0.5% of the weight of all photosynthesizing plants of the world, they synthesize organic carbon at a net annual rate similar to that of all terrestrial plants combined (Carr et al., 2006, Table 1) and form the base of the food chain that sustains almost all marine animals (Bar-On et al., 2018).
From a carbon sequestration standpoint, it is critical to understand how much of this transformed organic carbon (also known as net primary production, or NPP) is exported out of the euphotic zone (the top ~100 m of the ocean receiving sunlight, constantly mixing with the atmosphere) and into deeper ocean layers, where it can remain stored for longer timescales away from atmospheric interaction. Exported material consists of both particulate organic carbon (POC) and dissolved organic carbon (DOC) (Table 1).
POC accounts for the majority of the biological carbon pump. The magnitude of the POC export flux out of the euphotic zone has been estimated to be between 8-24% of the annual NPP rate (Laws et al., 2000; Schlitzer, 2002; Dunne et al., 2005; Henson et al., 2011; Siegel et al., 2014; Cabré et al., 2015; DeVries and Weber, 2017). Importantly, this POC flux decreases with depth as particles fragment, decompose, and remineralize through microbial respiration (e.g., Martin et al., 1987). Globally, ~13% and ~5% of the POC exported out of the euphotic zone makes it to 1,000 m depth and 2,000 m depth, respectively (DeVries and Weber, 2017). Therefore, a very small fraction of the organic carbon formed at the surface ultimately sinks to the sea floor, where it can be sequestered for millennia (DeVries et al., 2012) (Table 2 and Figure 2).
Dissolved organic carbon (DOC) globally accounts for about 15% of export flux out of the euphotic zone (Hansell et al., 2009; Emerson, 2014). DOC is especially important in nutrient-depleted waters of the subtropical ocean (oligotrophic areas), which are projected to expand with climate change and where DOC can account for roughly half of total export (Roshan and DeVries, 2017). The open ocean biological pump is an important part of the natural carbon cycle, but unlike the solubility pump, has not played an appreciable role in anthropogenic carbon uptake. Indeed, the current strength of the biological pump is unaffected by direct changes in atmospheric CO2 concentrations because phytoplankton productivity is limited by availability of nutrients and light, rather than CO2 or dissolved inorganic carbon concentrations.
Even so, indirect changes to ocean conditions brought on by increases in atmospheric CO2 concentrations can affect the strength of the biological pump. For example, warmer temperatures will increase both phytoplankton primary productivity and bacterial remineralization rates, but remineralization rates are predicted to increase more quickly due to remineralization’s higher sensitivity to temperature changes, resulting in a net decrease in exported POC fluxes (e.g., Cavan et al., 2019). Moreover, global warming stratifies the water column, hindering the upwelling of nutrients needed by phytoplankton at the surface. This decreases NPP and favors small, slower-sinking phytoplankton that grow better in nutrient-depleted waters, both of which result in less carbon sinking to depth (Leung et al., 2021). At the global scale, POC export flux out of the euphotic zone is expected to decrease under a business-as-usual scenario (Cabré et al., 2015, Table 1), resulting in a relatively small reduction in ocean carbon storage by 2100 (~1 GtC yr-1 below the euphotic zone). These predicted changes in the strength of the biological pump due to climate warming-induced changes in light or nutrient availability will therefore be small compared to atmospheric CO2-induced changes in the solubility pump.
The biological pump would only offer additionality for climate mitigation if it were modified in a significant way that brings it out of its existing natural equilibrium into a new equilibrium where more net carbon is sequestered on long timescales. Carbon Dioxide Removal (CDR) methods to increase the efficiency of the solubility and biological pumps – such as ocean iron fertilization (Ilyina et al., 2013) – have been discussed elsewhere and are out of the scope of this paper. Large uncertainties remain around climate feedbacks and wider social and ecological implications of CDR.
Coastal Ecosystems
Mangroves, Salt Marshes, Seagrass Meadows
The ability of vegetated coastal ecosystems – such as mangroves, salt marshes, and seagrass meadows (Figure 1) – to capture and store large amounts of carbon is due to their high productivity, as well as to the saturated and saline conditions of their soils that slow down organic matter decomposition and limit the production of potent GHGs such as methane (CH4) that freshwater wetland ecosystems typically generate. Mangroves are significant carbon sinks, with a biomass productivity (mass per unit area and time) comparable to that of tropical forests (Alongi, 2009), in addition to typically high soil carbon (SOC) sequestration rates (103 g SOC/m2/y) (Alongi, 2020). Salt marshes are efficient in accumulating carbon in the soil (212 g SOC/m2/y) (Alongi, 2018), with a highly variable biomass productivity depending on climate and dominant species (Duarte and Chiscano, 1999). Seagrass carbon stocks and coverage are less well known than that of mangroves and salt marshes; estimated differences between seagrass productivity (Duarte and Chiscano, 1999) and total carbon stocks (Alongi, 2018), suggest significant export of the biomass produced, potentially into shelf sediments and the deep sea (Table 3).
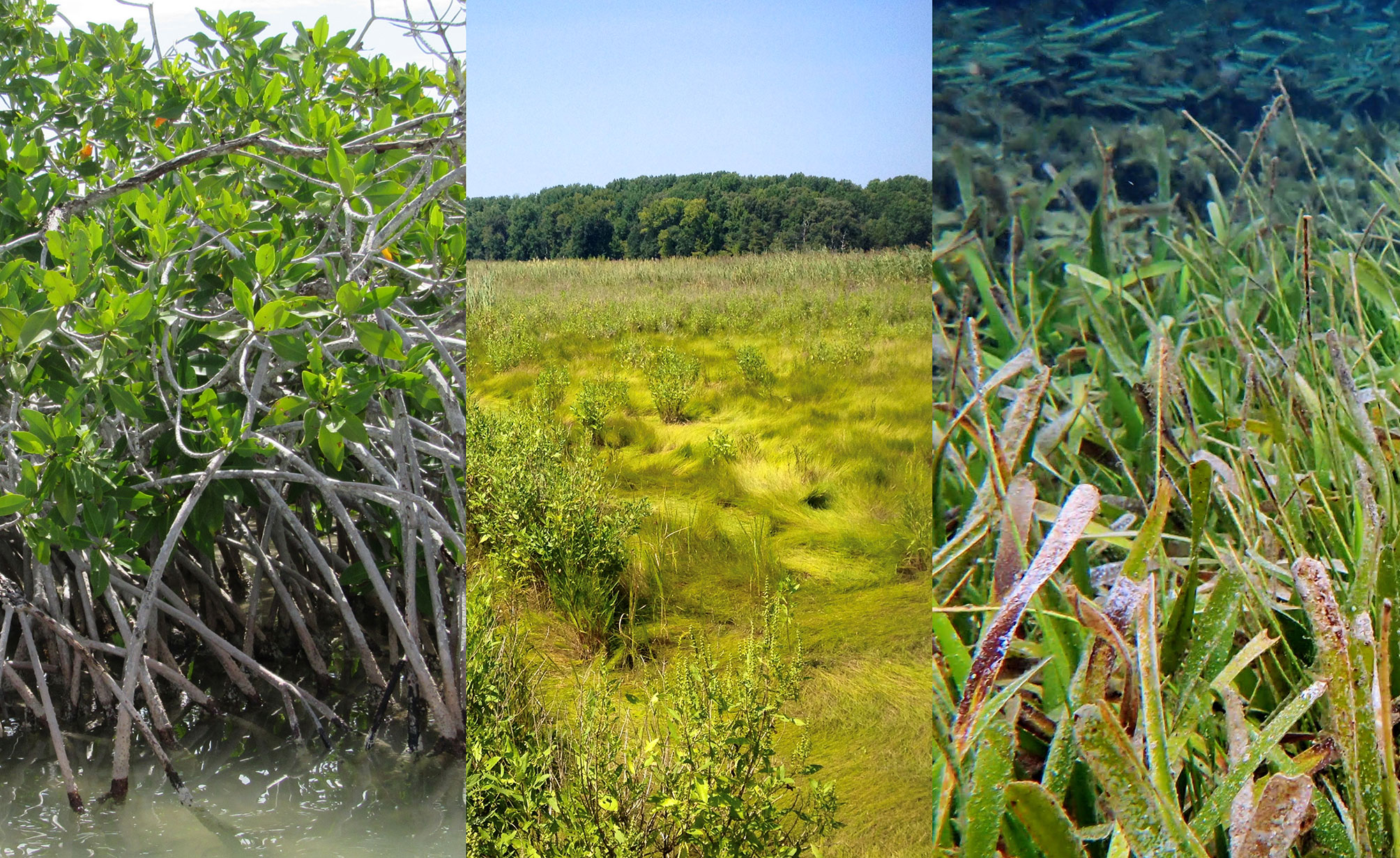
Figure 1 Mangrove forest, salt marsh, and seagrass meadow, the three coastal ecosystems currently recognized for measurable blue carbon mitigation potential (photo credits: S. Baez, (B) Bernal, (J) Lefcheck).
Due to their ability to sequester carbon in biomass and especially in soils, the preservation, conservation, and restoration of these ecosystems has been recognized as an action to contribute to climate change mitigation with many co-benefits for adaptation (IPCC, 2014; Howard et al., 2017). However, net additional emissions reductions can only be achieved through avoiding coastal ecosystem degradation and destruction – as the annual emissions from their loss were estimated as equivalent to 3–19% of annual GHG emissions from global deforestation (Pendleton et al., 2012) – and enhancing or restoring their carbon sequestration capacity, which varies across regions. Climate change is also impacting the ecosystems themselves, as climate hazards such as sea level rise, increasing temperatures, and extreme weather events can alter productivity and change species composition – thereby altering the extent of coastal ecosystem carbon stocks.
In addition to carbon sequestration, coastal ecosystems also provide numerous adaptation services (Barbier et al., 2011; Nordlund et al., 2016) such as buffering severe coastal storm impacts, mitigating floods, protecting against accelerated sea level rise, and supporting coastal water quality and food security. Effectively managing coastal ecosystems as carbon sinks while preserving their ecological integrity requires robust estimates of ecosystem extent and conditions (Friess et al., 2019). Mangrove mapping has improved in recent years (Hamilton and Casey, 2016), but the extent of seagrass bed coverage remains uncertain (McKenzie et al., 2020). Despite their ecological and climate regulating value, coastal ecosystems continue to disappear at a fast pace, releasing stored carbon into the atmosphere. Barriers to proper preservation, conservation, and restoration, such as habitat unsuitability and lack of community engagement (Lee et al., 2019; Lovelock and Brown, 2019; Ellison et al., 2020), must be addressed for these activities to succeed.
Macroalgae
Macroalgae comprise the most extensive and productive benthic (seafloor) vegetated marine habitats globally; widely distributed across coastal latitudes including kelp forests in cold, coastal waters and sargassum in tropical and temperate coastal areas, they are identified as potentially important carbon sinks (Krause-Jensen and Duarte, 2016; Krause-Jensen et al., 2018). Compared to rooted coastal vegetation, highly productive yet rootless macroalgal communities generate less on-site carbon burial and export more of their carbon away from the site of origination (Queirós et al., 2019; Filbee-Dexter and Wernberg, 2020; Hurd et al., 2022). Kelp and other macroalgae are gaining attention due to their highly recalcitrant (i.e. difficult to decompose) organic matter, and their ability to grow quickly and capture significant amounts of atmospheric carbon in their biomass (Table 3).
The global average stocks of macroalgae are only recently being assessed (Krause-Jensen and Duarte, 2016; Krause-Jensen et al., 2018) and the extent to which they currently exist compared to their potential area with ecosystem restoration and management is not well known. Recent estimates in Australia reveal that thanks to their high productivity, macroalgae account for 10-23 MtC or 30% of national blue carbon stocks (Filbee-Dexter and Wernberg, 2020), demonstrating how significant this carbon pool can be. These systems are not yet adequately managed or protected by current policies as only a portion of macroalgal-carbon is sequestered and stored in ocean sediments through on site burial, thus complicating management (Laffoley, 2020). It is estimated that 80-90% of macroalgal carbon production leaves the original site and is incorporated into the marine food web and eventually buried at depth (Krumhansl and Scheibling, 2012; Krause-Jensen and Duarte, 2016). Furthermore, Ortega et al. (2019) studied vertical macroalgal carbon export and estimated that 69% of the macroalgae carbon at surface will sink below 1,000 m, close to permanent sequestration and storage depths, and 24% will reach deep seafloor sediments (>4000 m). Similarly, Krause-Jensen and Duarte (2016) estimated that 19% of the macroalgae carbon production will stay in the coastal ocean as POC, of which 4.6% will be buried in the coastal and shelf sediments. Lateral carbon export of macroalgae can also be traced from polar to tropical regions (Krause-Jensen and Duarte, 2016). Understanding the linkages between macroalgae and coastal sediments is therefore key to assessing their potential as carbon sequestering systems (Queirós et al., 2019).
While these ecosystems do not yet fit well in current carbon accounting frameworks, as the amount of carbon carried away and buried into ocean sediments is not easily measured and monitored (Krause-Jensen et al., 2018; Queirós et al., 2019; Hurd et al., 2022), their high sequestration rates at depth are promising. Understanding of macroalgal carbon cycling and their potential role in ocean carbon dynamics is growing quickly as well as interest in seaweed farming for climate mitigation. Funding for research should be encouraged to better understand the suitability of macroalgae preservation efforts and farming options in carbon accounting and blue carbon strategies (Krause-Jensen and Duarte, 2016; Ortega et al., 2019).
Shelf Sediments
On a global scale, Luisetti et al. (2020) estimate that offshore or shelf sediments represent a long-term carbon stock comparable to that in tropical forests. However, the precise ability of these sediments to store carbon depends on the type of sediment (Laffoley, 2020), and there is a lack of site-specific knowledge necessary for accounting (Queirós et al., 2019; Hurd et al., 2022) (Table 5). Disturbing shelf sediments leads to sediment resuspension that results in remineralization of the component particles (Pusceddu et al., 2014), thereby releasing soil carbon that had accumulated over centuries to millennia. Sala et al. (2021) estimate that 4.9 million km2 or 1.3% of the global ocean is trawled each year, resulting in 1.47 Gt yr-1 of aqueous CO2 emissions for the first 9 years, which is at least an order of magnitude higher than the simulated global natural carbon accumulation into sediments through export of particulate organic carbon via the biological pump (Table 3). Other estimates note that industrial trawling may lead to sediment erosion comparable to highly deteriorated agricultural fields on land (Pusceddu et al., 2014). Although an unknown fraction of the aqueous CO2 is emitted to the atmosphere, the magnitude of disturbed sediment emissions from trawling is an ongoing threat to sediment carbon that highlights the need to both better quantify the rate of coastal sediment carbon accumulation and to discuss ways to protect these sediments. With clearer quantification of both coastal shelf sediment carbon accumulation rates and atmospheric carbon emissions due to trawling, prevention of further trawling could be considered avoided emissions.
Marine Organisms
Here we briefly summarize the current research on marine organisms’ role in the biological pump, and their blue carbon potential. Organisms such as krill, big fish, and whales are known to have a fertilizing effect on phytoplankton, hence influencing the biological carbon pump. Moreover, their diurnal migrations, fecal pellets, and dead carcasses also add to the downward vertical pump of carbon. Calcifying organisms like coral and oyster reefs have also been investigated to assess their potential to sequester and store carbon. Note that the biological pump is most widely reported as the export flux out of the euphotic zone, but only a fraction of this carbon export is kept out of the atmosphere for timescales relevant to climate mitigation, and even a smaller fraction gets to the seafloor, where carbon can remain for centuries to millennia (see biological pump section, above).
Zooplankton
Zooplankton graze on phytoplankton at the surface, then move down the water column and actively transport carbon via egestion, respiration, and mortality from the surface to depths of 1,000m or more as part of the biological pump, accounting for 16-18% of total carbon export flux (out of the top ~100 m) (Aumont et al., 2018; Archibald et al., 2019). Below 1,000 m, zooplankton active transport increases export by at least 0.44 Gt C/yr (though this includes contributions from migrating micronekton as well) (Hernández-León et al., 2020). Further quantification of the global contribution of total zooplankton active transport to the biological carbon pump is an active area of research.
Antarctic krill, a type of zooplankton and the keystone species of the Southern Ocean, are also an increasing focus of research for their role in the Antarctic biological carbon pump (Cavan et al., 2019). Although researchers theorize that krill carbon export may be significant, its quantification is still highly uncertain (Belcher et al., 2019; Cavan et al., 2019). Antarctic krill, while accounting for the largest krill population globally at 400 Mt, are being impacted by ocean acidification, warming water, and changes in sea ice dynamics (Kawaguchi et al., 2013; Klein et al., 2018; Meredith et al., 2019), which may be negatively impacting the population and shifting it southwards towards the continent (Atkinson et al., 2019; Meredith et al., 2019). Krill are further stressed by increasingly concentrated fishing in the region (Watters et al., 2020).
Fish
Fish also contribute to the biological carbon pump via the same vertical migration-induced active transport mechanisms as zooplankton, as well as passive transport via sinking fecal pellets formed at the surface, a relatively large flux compared to some of the other marine organisms discussed here (Table 4). It is unknown the extent to which protection and rebuilding of fish stocks would change the biological pump. However, the reduction in fish carcass deadfall to the seafloor due to industrial fishing is somewhat better studied. Mariani et al. (2020) estimate that industrial fishing of large, dense fish (including tuna, mackerel, billfish, and sharks) has prevented the sequestration of 21.8 ± 4.4 Mt C through deadfall since 1950 and emitted 0.2 Gt C (primarily through fuel consumption) into the atmosphere. This study estimates that if fishing levels were reduced to zero (taking 2014 levels as a reference), an additional 1.09 MtC/yr would be stored in large fish biomass with ~0.6 MtC/yr sequestered via carcass deadfall (Mariani et al., 2020). In addition to fishing pressures, fish populations are also impacted by climate change, which alters habitable zones and food web structures. Fish biomass is projected to decrease by 10 to 25% by 2011 under the IPCC AR6 high greenhouse gas emissions scenario SSP58.5 (Tittensor et al., 2021). These climate and fishing-driven decreases in fish stocks have serious repercussions for biodiversity, ecosystem resilience, and human well-being for coastal communities (IPCC, 2022). The direct additional impact of restoring fish on blue carbon sequestration is relatively low and is therefore unlikely to be an effective climate mitigation tool. In contrast, a reduction in industrial trawling could preserve significant amounts of carbon stored in the sediment floor (Sala et al., 2021).
Whales
Whales contribute to the biological pump through fertilization of the surrounding ocean with iron-rich excrement, which also contributes to fast-sinking particulate organic carbon, and to a lesser extent whale fall, when the carcass of a dead whale sinks to the seafloor and an unknown fraction is buried in ocean sediments. Researchers have estimated the restoration of whale populations to pre-whaling levels could generate additional carbon sequestration of 0.16 - 6.7 MtC yr-1 depending on the population (Lavery et al., 2010; Pershing et al., 2010; Durfort et al., 2020 preprint). However, whales are threatened by climate impacts as well as increased shipping traffic and pollution (Tulloch et al., 2019). Despite their potential importance in the biological carbon pump, whales’ role in marine carbon sequestration is minimal (Maldonado et al., 2016) (Table 4), and the time scale and uncertainties for population recovery, threatened by climate change, further complicates the calculation of additional carbon sequestration that could be attributed to these species (Durfort et al., 2020 preprint).
Calcifying Organisms
Calcifying organisms such as oysters and coral reef systems are also discussed in the context of carbon sequestration. Oysters are filter feeders that excrete organic carbon onto the seabed (Lee et al., 2020), but they also release CO2 through respiration and the shell formation (calcification) process. For this reason, oysters have been kept out of blue carbon frameworks for now, as the balance between calcification and respiration needs further investigation (Ullman et al., 2013).
Recent estimates indicate that coral reefs cover about 52.7 million hectares worldwide (Mora et al., 2006), potentially representing 31 Mt of organic carbon (Crossland et al., 1991). However, coral reefs are generally considered a small net source of CO2 rather than a net sink despite supporting photosynthetic algae, and are excluded from blue carbon frameworks (Howard et al., 2017). Like oysters, reefs undergo continuous calcification and dissolution, and their role as a carbon sink or source depends on the balance between calcification and dissolution. Calcification releases CO2 to the atmosphere, whereas dissolution represents an oceanic carbon sink. The relative rates of calcification and dissolution depend on ocean acidity and the concentration of dissolved inorganic carbon in the water. Under current conditions, the amount of CO2 released to the atmosphere by the reefs is slightly greater than that being captured through dissolution (Howard et al., 2017). This balance may change in the future as ocean acidification alters the biochemistry of the ocean column in favor of dissolution.
Calcification is common in other coastal ecosystems as well, as a restored subtidal habitat (e.g., seagrasses) can favor the proliferation of shelled molluscs. For accurate carbon accounting in all of the above ecosystems, site-specific assessments are necessary to assess the balance between organic and inorganic carbon production and sequestration (Macreadie et al., 2017). While the climate mitigation benefits of these systems might be negligible, oysters and coral reefs provide multiple ecosystem services (e.g., water quality improvement, coastal resilience, and fish habitat, among others) and warrant protections.
Polar Regions
Contrary to decreasing global blue carbon stocks elsewhere, blue carbon storage in Antarctic bottom sediments and benthic ecosystems is likely increasing due to sea ice loss, ice shelf disintegration, and retreat of coastal glaciers (Barnes, 2015; Barnes, 2018; Barnes et al., 2018; Zwerschke et al., 2022), which enlarges shallow areas where phytoplankton can bloom and thus enhances benthic biomass. This supports subsequent highly efficient sequestration and immobilization of additional biomass in sediments (meaning the fraction of NPP that is deposited on the seafloor is very high, as observed by Peck et al., 2010) and the hard skeletal parts of calcifying benthic organisms (particularly sponges and corals).
The potential spatial extent of Antarctic sediments and benthic ecosystems is large and growing, however this trend is regional (Pineda-Metz et al., 2020), and the percentage of global carbon burial occurring in Antarctic blue carbon sinks is currently insignificant compared to mangroves, salt marshes, and seagrass meadows (Bax et al., 2021). The Antarctic benefits from relatively little anthropogenic disturbance, so guaranteeing that it remains undisturbed should be the main priority for protection efforts and climate mitigation policies (Bax et al., 2021); however the criteria of additionality would not be met through preservation unless these ecosystems were under immediate threat (bottom trawling is currently restricted through the Antarctic Treaty System; see Brasier et al., 2021 for an overview of risks to Southern Ocean benthos). Due to its remoteness, accurate large-scale measurement of Antarctic blue carbon is expensive and difficult (Cavanagh et al., 2021).
The Arctic is also typically highly productive (Arrigo and van Dijken, 2015) with fast transfer of organic matter to the seafloor. As the Arctic becomes increasingly ice-free due to climate change, the biological storage of carbon is being altered and this may lead to increased export to the seafloor, though numerous uncertainties remain (Souster et al., 2020; März et al., 2022). Carbon store hotspots are at risk from increasing bottom trawling, as fishing activity and oil exploration expands into previously inaccessible areas (Fauchald et al., 2021; März et al., 2022). Overall, more research is needed to identify and quantify the most significant blue carbon hotspots in polar areas, which may inform how this system can fit within new or existing mitigation frameworks and to incorporate carbon benefits into regional preservation and management decision-making.
Discussion
Challenges to Blue Carbon Accounting
Numerous challenges to coastal and oceanic blue carbon accounting make comprehensive carbon sequestration quantification, and therefore management to maximize the mitigation benefits of nature-based solutions, difficult for certain systems. From a climate mitigation policy perspective, only the management interventions that lead to additional sequestration and long-term storage of carbon out of the atmosphere compared to scenarios without intervention are relevant (Crooks et al., 2018). Carbon storage is assessed in the literature on timescales from short-term (centennial) to long term (millennia). Only the long-term storage provides solutions that are considered permanent for mitigation.
Using shorter time scales for climate mitigation strategies, however, does provide benefits such as avoiding climatic tipping points (Jørgensen and Hauschild, 2013). The ability to continuously measure and monitor carbon and other GHG fluxes and stores over long periods is therefore crucial when considering whether a sequestration process is additional and meaningfully contributes to climate mitigation (Table 5).
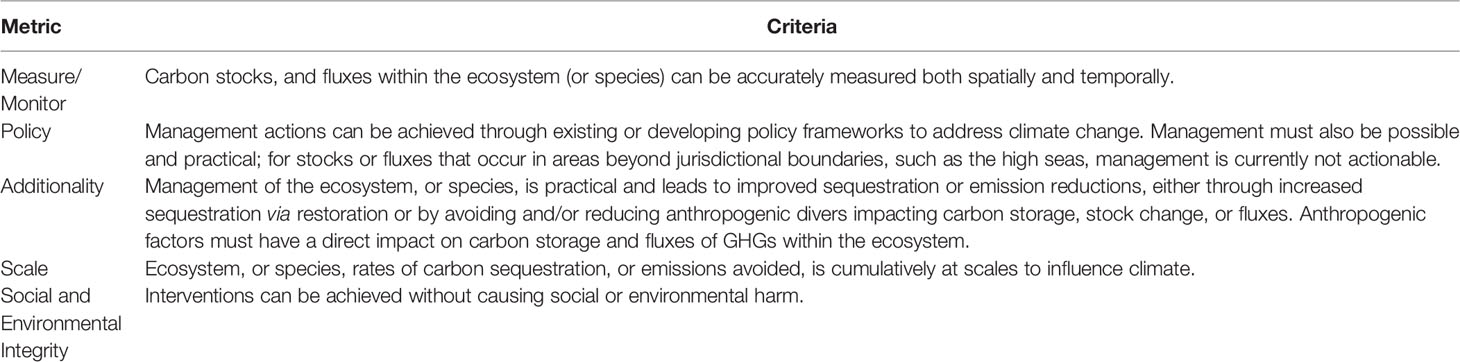
Table 5 Attributes of ecosystems recognized for their carbon sequestration benefits in the context of climate mitigation policies and financing as outlined in Crooks et al. (2018).
Methodologies for estimating national inventories of sinks and sources of GHG from coastal wetlands, and the associated human activities that affect them, such as harvesting, revegetation and draining, are becoming available (IPCC, 2019b). In some cases, conservation and restoration of coastal ecosystems may not be net “carbon positive” when all ecosystem components of management practices are considered, such as coastal regions where calcification outpaces carbonate dissolution (e.g., some seagrass meadows) (Howard et al., 2017; Macreadie et al., 2017). Accounting for all ecosystem synergies and fluxes is even more challenging in the open ocean. Monitoring and continued quantification of these processes and rates over time is needed but can be prohibitively expensive.
Proper spatial accounting of carbon sequestration is also a challenge. Spatially transported carbon can represent a significant portion of ecosystem productivity (e.g., kelp). Indeed, this carbon can be deposited within a few kilometers or as distant as thousands of kilometers from where it was produced, which makes accounting under a local framework difficult (Tzortziou et al., 2011; Duarte and Krause-Jensen, 2017; Huxham et al., 2018). While area-based protections (see below) can be a useful tool to conserve carbon pools, they might result in displacement of effort (fishing, trawling, habitat conversion) outside of spatial protection boundaries. Species such as whales and fish provide additional challenges for accounting, as their ranges can extend across national and other jurisdictional boundaries and whale and fish fall ultimately becomes a component of other carbon pools (such as sediment); both these factors make double counting under existing climate mitigation and carbon valuation frameworks a real possibility.
Lastly, robust temporal accounting can also be challenging. Solutions differ in the amount of time needed for implementation or net sequestration to begin, or the amount of time that carbon remains sequestered away from the atmosphere. For instance, DeVries et al. (2012) predict that only 10-30% of the carbon exported out of the euphotic layer is still in the ocean after 100 years depending on where that export occurred.
Ocean Climate Policy
The global ocean is critical to a stable climate and within the climate policy landscape there is growing interest in bringing ocean related actions forward as climate solutions (Hoegh-Guldberg et al., 2019b).
United Nations Framework Convention on Climate Change
Under the UNFCCC’s global objective of the Paris Agreement to mitigate anthropogenic GHG emissions, Parties can put forward nature-based solutions as part of their emission reduction and climate adaptation pledges known as nationally determined contributions (NDCs) (UNFCCC, 2015). The Agreement does not identify specific ocean-based interventions, but rather emphasizes the reduction of Parties’ GHG emissions as the central tenet to be implemented for mitigating and adapting to climate change.
As of October 2021, 71 countries have mentioned coastal and marine nature-based solutions within their new or updated NDCs. Of these, 45 countries mention the protection, management, and restoration of coastal and marine ecosystems to meet both mitigation and adaptation climate objectives (Lecerf et al., 2021). Costa Rica for example has committed to protecting all their coastal wetlands as recorded in the country’s National Wetland Inventory, while Seychelles has committed to protecting all its coastal wetlands by 2030, and Belize has committed to both protecting and restoring mangroves along with developing a seagrass management plan.
Although most countries have at least one type of coastal wetland, for a handful of countries that have substantial amounts of these ecosystems (Taillardat et al., 2018), proper conservation, restoration, and management of these ecosystems may result in sequestration rates significant enough to reduce overall country emissions (Bindoff et al., 2019). While there is burgeoning interest to include marine protections within climate mitigation policy frameworks, several key criteria must be met to ensure that these protections advance climate objectives (Table 5).
Recent papers have pointed to the potential monetary value of polar blue carbon (Armstrong et al., 2019; Bax et al., 2021), but without clear oversight, double-counting of emission reductions (when two entities claim the same carbon removal), could occur with unfixed blue carbon sources or sequestration that takes place on the high seas (e.g. two nations taking part in the multilateral governing body could both claim reductions from the same source). While deep-sea sediments were not discussed in this manuscript, this risk of double counting also extends to sediments located beyond countries’ exclusive economic zones; while additional data is needed to quantify these stocks, their disturbance could result in the remineralization of this carbon (Atwood et al., 2020). Rules to operationalize Article 6 of the Paris Agreement, the UNFCCC framework to establish a global mechanism for carbon markets to reduce emissions, were established at COP26 in November 2021, but action on the high seas is not included within the UNFCCC (Gallo et al., 2017). Article 6 does however specify that when parties transfer international mitigation outcomes to NDCs, they must avoid double counting (UNFCCC, 2021 see Article 6 paragraph 4). Furthermore, the risk of green-washing (or in this case blue-washing), where entities label environmentally deleterious actions as sustainable, is elevated in the climate space due to the monetization of carbon credits (In and Schumacher, 2021).
Alternative Policy Frameworks, Tools, and Research
While many marine ecosystems may not be suitable for climate mitigation policies at this time, they can be important to building climate adaptation and resilience, protecting biodiversity, and fostering societal well-being (Bindoff et al., 2019; IPBES-IPCC; Sala et al., 2021). The latest IPCC WGII report (IPCC, 2022) underlines the role of resilient biodiversity and ecosystems and their services in mitigation and adaptation and achieving climate resilient development. The effectiveness of ecosystem-based adaptation and approaches to climate change mitigation based on ecosystems are reduced as warming increases and limits to natural adaptation capacity are reached. This underscores that scientists, advocates, and policymakers should not have a singular focus on climate mitigation policies in the blue carbon context, but utilize, or create new, frameworks more appropriate to their conservation, adaptation, and well-being goals, while seeking connections between the frameworks that may strengthen them collectively.
In instances where carbon accounting towards inventories is not suitable, there are a suite of policy frameworks that may be more appropriate to achieve these broader goals. The Convention on Biological Diversity (CBD), which came into force in 1993 and includes 193 Parties to the agreement, sets up an overarching framework to address the underlying causes of biodiversity loss, reduce the direct pressures on biodiversity, and safeguard ecosystems. The CBD also acknowledges the interlinkages between biodiversity and climate change and has adopted voluntary guidelines for the design and implementation of ecosystem-based approaches to climate change adaptation and disaster risk reduction (CBD, 2019).
Other frameworks support the creation of marine protected areas, which can protect carbon capturing marine ecosystems and species, and help build resilience to climate change (Roberts et al., 2017). For example, a recent assessment found that marine World Heritage sites and their immediate surrounding areas comprise at least 21% of the global area of blue carbon ecosystems (UNESCO, 2020). The Commission for the Conservation of Antarctic Marine Living Resources (CCAMLR) has agreed to designate a representative network of marine protected areas (MPAs) in the Southern Ocean (Brooks et al., 2020), though progress in recent years towards this goal has stalled. The potential U.N. agreement for the conservation and sustainable use of marine biological diversity of areas beyond national jurisdiction (BBNJ) could lead to widespread protections from shipping, fishing, and deep-sea mining on the little understood high seas (Gjerde and Rulska-Domino, 2012; Visalli et al., 2020), an area covering half of the planet (UN 2017) and managed by over 20 varied governance organizations. Despite political challenges, the discussions within CCAMLR and the U.N. are a testament to the international scientific and diplomatic success that can be found through multilateral frameworks.
In addition to these frameworks, tools to identify and protect vital marine ecosystems for carbon storage have been developed by a number of scholars (see McLeod and Salm, 2006; Di Nitto et al., 2014; Laffoley, 2020). Institutions can further support the restoration and conservation of blue carbon ecosystems through creative carbon financing. Additional funding is needed to better understand the sequestration potential in other marine ecosystems, as noted above. The authors underscore that funding for ocean research remains inadequate (IOC-UNESCO, 2020), even as the percentage for ocean and climate funding grows (Our Shared Seas, 2022). Due to this shortfall, it is important to recognize that it is more cost-effective to preserve, rather than restore, blue carbon ecosystems (Jakovac et al., 2020; Su et al., 2021), and that the ecological integrity of a system supports its ability to store carbon and deliver other co-benefits. With the rapidly closing window for significant climate mitigation (IPCC, 2021) and adaptation action (IPCC, 2022), and continued shortfalls of climate financing, a near-term focus on enhancing current validated blue-carbon ecosystems may be justified (see Macreadie et al., 2019, for a set of blue carbon research priorities).
Lastly, to better enable policymakers to operationalize scientific research, results should be communicated in both the metrics used in the field, as well as put into the greater context of emissions reduction. As described previously, in this article we standardized units of measurements to enable comparisons between potential blue carbon gains and current emissions (Figure 2). Scientists can easily communicate their findings by similarly standardizing measurements, as well as utilizing emissions calculators (such as the one provided by the U.S. Environmental Protection Agency, see EPA, 2022), and including clear messages for policymakers.
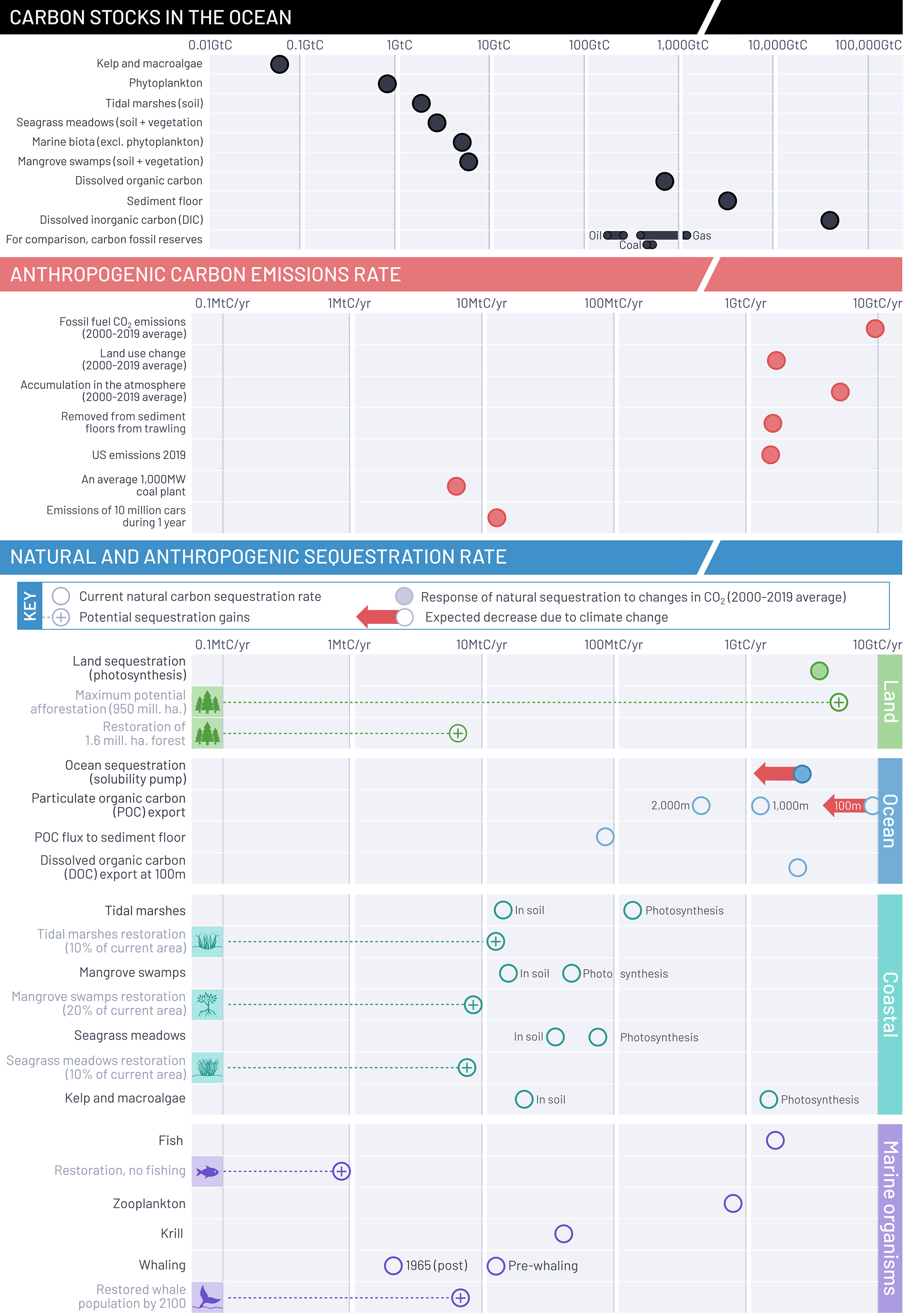
Figure 2 An overview of the annual potential sequestration gains of blue carbon interventions discussed in the manuscript, compared to current annual natural sequestration and emission rates of key greenhouse gas sources. Marine carbon stocks are also displayed in comparison to existing fossil fuel reserves. The values are plotted on logarithmic scales. A more detailed explanation of the processes can be found in Tables 1–3. We have not included the natural carbon sequestration rates for the solubility pump and for photosynthetic land sequestration. These numbers can be found in Friedlingstein et al. (2020). In the open ocean (POC, DOC, zooplankton, fish, krill, whales), the natural sequestration rate (i.e., ‘export flux’) is estimated and reported at the euphotic depth; only a portion of this export will be sequestered for long timescales within the deep ocean and on the sediment floor. The coastal restoration numbers do not include soil carbon, which would represent 75% of the total carbon stock in the ecosystem. The maximum carbon gain potential of afforestation and forest restoration was calculated as the average of tropical and temperate forests, following the CO2 removal rates in Bernal et al. (2018). The area of 950 mill. ha is the estimate of IPCC SR15 needed commitment to keep temperatures at 1.5°C. The forest restoration (1.6 mill. ha, average of tropical and temperate forests) is shown to compare to the potentially restorable mangrove global mangrove area (20% of current area, from Zeng et al., 2021). It is unknown what fraction of the carbon removed from the sediment floor due to trawling eventually leaves the ocean into the atmosphere. Figure illustrated by Stacey McCormack (Visual Knowledge).
Key Messages for Policymakers
1. From a climate mitigation policy perspective, only the blue carbon management interventions that lead to additional sequestration and long-term storage of carbon out of the atmosphere compared to scenarios without intervention are relevant to reach the 1.5°C goal of the Paris Agreement, set to avoid the most catastrophic impacts of climate change.
2. Coastal wetlands carbon accounting methodologies and management is practical and can lead to improved sequestration and storage, as well as climate adaptation benefits. Macroalgae, marine sediments, and polar benthic ecosystems require further research to determine whether they meet, or to establish new, criteria for inclusion within current mitigation policies.
3. Current data does not support the idea that zooplankton, fish, whales, and some calcifying organisms provide significant, readily measurable additionality; therefore, their inclusion at this time in mitigation and carbon credit frameworks would risk greenwashing. However, these organisms are an important part of the natural carbon cycle, and their reduction due to human pressures has serious implications for marine ecosystems and their services.
4. Scientists, advocates, and policymakers should not have a singular focus on climate mitigation policies but utilize, or create new, frameworks more appropriate to their conservation, adaptation, and well-being goals, while seeking connections between the frameworks that may strengthen them collectively.
5. With the 2030 deadline for significant climate mitigation and adaptation action, and continued shortfalls of climate financing, a near-term focus on protecting and enhancing current validated blue-carbon ecosystems may be justified. As work continues to address climate change after 2030, greater exploration and implementation of scalable ocean-based solutions is needed.
Conclusion
Research clearly shows that the climate crisis must be addressed through immediate and significant point-source greenhouse gas emissions reductions across sectors. The management of blue carbon ecosystems can help meet some climate challenges, although climate change is predicted to decrease open ocean sequestration rates associated with the biological and solubility pumps. However, by maintaining a narrow focus on carbon mitigation, scientists, practitioners, and policymakers can run the risk of overstating the climate benefits of some blue carbon ecosystems, undermining other important marine policy actions.
The ocean and its ecosystems are a major carbon reservoir, but carbon sequestration within and flux across different carbon pools is varied and dynamic, making some marine ecosystems more suitable for climate mitigation policies than others. Protecting and restoring coastal ecosystems including mangroves, salt marshes, and seagrass meadows, which helps avoid emissions and sequester carbon, should be a part of the climate solution toolbox. Protecting and restoring macroalgal communities and polar benthos, and protecting marine sediments, may be promising for additional carbon sequestration and avoided emissions, but currently face challenges for carbon accounting and require further research to prove their carbon mitigation potential. On the other hand, the mitigation benefits zooplankton, fish, whales, and calcifying organisms is less clear, and in many cases the data indicates limited to no additionality.
Despite their lack of carbon mitigation, the protection and restoration of open ocean systems and marine organisms provide clear environmental benefits and climate resilience gains. In these instances, aligning conservation objectives with appropriate policy frameworks outside of the UNFCCC has the potential to preserve these critical marine ecosystems and species without the risk of green-washing or drawing attention away from necessary point-source emissions reductions.
There is an urgent need for actors in the policy, advocacy, and especially scientific community to make the connections between overlapping frameworks, and support biodiversity conservation on its own merits without valuing it solely for carbon sequestration potential. Scientists can play a leading role in facilitating these conversations through improved connections to policy dialogues and communicating their results in terms approachable to non-experts. Importantly, scientists can develop standardized approaches to measure, monitor, and report carbon accounting across ecosystems that can generate transparent and robust data and use comparable metrics to quantify sequestration potential as is used to describe GHG emissions and emission reductions, in order to make appropriate comparisons and put additionality in perspective.
Ultimately, reducing global emissions should remain the top priority. Nature-based solutions cannot replace these actions, but should be seen as a complement to emissions reductions while also supporting adaptation and biodiversity conservation (Seddon et al., 2020; Seddon et al., 2021). Ocean based solutions to climate change must be robust and credible, provide multiple benefits across scales, and demonstrate additionality. Therefore, investing in large, scalable blue carbon preservation, conservation, and restoration interventions is an important step towards comprehensively addressing the climate crisis.
Author Contributions
ABC, AC, BB, AP-P, and EP conceived of and held initial development discussions for this review. AC and BB, with support from SB, SL, and EP, contributed to the Carbon Storage and Fluxes section. ABC and SB, with support from AP-P and EP, contributed to the discussion. All authors contributed to the Introduction and reviewed and approved the final manuscript.
Funding
Funding for open access publication was provided by The Pew Charitable Trusts.
Conflict of Interest
SB is currently employed by The Pew Charitable Trusts, which provided the funding for open-access publication of this review.
The remaining authors declare that the research was conducted in the absence of any commercial or financial relationships that could be construed as a potential conflict of interest.
Publisher’s Note
All claims expressed in this article are solely those of the authors and do not necessarily represent those of their affiliated organizations, or those of the publisher, the editors and the reviewers. Any product that may be evaluated in this article, or claim that may be made by its manufacturer, is not guaranteed or endorsed by the publisher.
Acknowledgments
The authors would like to thank Stacey McCormack at Visual Knowledge for her expert design and development of Figure 2.
Footnotes
- ^ Cabré et al., 2015; DeVries and Weber, 2017; Dunne et al., 2005; Henson et al., 2011; Laws et al., 2000; Schlitzer, 2002; Siegel et al., 2014. The most recent estimate puts this rate of export of POC out of the euphotic layer at 9.1 ± 0.2 GtC yr-1 (DeVries and Weber, 2017)
References
Alongi D. M. (2009). The Energetics of Mangrove Forests (Netherlands: Springer Science & Business Media).
Alongi D. M. (2018). Blue Carbon: Coastal Sequestration for Climate Change Mitigation (Cham, Switzerland: Springer).
Alongi D. M. (2020). Global Significance of Mangrove Blue Carbon in Climate Change Mitigation. Science 2 (3), 67. doi: 10.3390/sci2030067
Archibald K. M., Siegel D. A., Doney S. C. (2019). Modeling the Impact of Zooplankton Diel Vertical Migration on the Carbon Export Flux of the Biological Pump. Global Biogeochem. Cycles 33 (2), 181–199. doi: 10.1029/2018GB005983
Arico S., Arrieta J. M., Bakker D., Boyd P., Cotrim da Cunha L., Chai L., et al. (2021). Integrated Ocean Carbon Research: A Summary of Ocean Carbon Research, and Vision of Coordinated Ocean Carbon Research and Observations for the Next Decade. Eds. R W., C S., S Aricò (Paris: UNESCO). doi: 10.25607/h0gj-pq41.
Armstrong C. W., Foley N. S., Slagstad D., Chierici M., Ellingsen I., and Reigstad M (2019). Valuing Blue Carbon Changes in the Arctic Ocean. Front. Mar. Sci. 6, 331. doi: 10.3389/fmars.2019.00331
Arora V. K., Katavouta A., Williams R. G., Jones C. D., Brovkin V., Friedlingstein P., et al. (2020). Carbon–concentration and Carbon–Climate Feedbacks in CMIP6 Models and Their Comparison to CMIP5 Models. Biogeosci. 17 (16), 4173–4222. doi: 10.5194/bg-17-4173-2020
Arrigo K. R., van Dijken G. L. (2015). Continued Increases in Arctic Ocean Primary Production. Prog. Oceanogr. 136, 60–70. doi: 10.1016/j.pocean.2015.05.002
Atkinson A., Hill S. L., Pakhomov E. A., Siegel V., Reiss C. S., Loeb V. J., et al. (2019). Krill (Euphausia Superba) Distribution Contracts Southward During Rapid Regional Warming. Nat. Clim. Change 9 (2), 142–147. doi: 10.1038/s41558-018-0370-z
Atwood T. B., Witt A., Mayorga J., Hammill E., Sala E. (2020). Global Patterns in Marine Sediment Carbon Stocks. Front. Mar. Sci. 7, 165. doi: 10.3389/fmars.2020.00165
Aumont O., Maury O., Lefort S., Bopp L. (2018). Evaluating the Potential Impacts of the Diurnal Vertical Migration by Marine Organisms on Marine Biogeochemistry. Global Biogeochem. Cycles 32 (11), 1622–1643. doi: 10.1029/2018GB005886
Barbier E. B., Hacker S. D., Kennedy C., Koch E. W., Stier A. C., Silliman B. R. (2011). The Value of Estuarine and Coastal Ecosystem Services. Ecol. Monogr. 81 (2), 169–193. doi: 10.1890/10-1510.1
Barnes D. K. A. (2015). Antarctic Sea Ice Losses Drive Gains in Benthic Carbon Drawdown. Curr. Biol. 25 (18), R789–R790. doi: 10.1016/j.cub.2015.07.042
Barnes D. K. A. (2018). “Blue Carbon on Polar and Subpolar Seabeds," in Carbon Capture, Utilization and Sequestration. Ed. R.K A. (London: IntechOpen).
Barnes D. K. A., Fleming A., Sands C. J., Quartino M. L., Deregibus D. (2018). Icebergs, Sea Ice, Blue Carbon and Antarctic Climate Feedbacks. Philos. Trans. R. Soc 376, 20170176. doi: 10.1098/rsta.2017.0176
Bar-On Y. M., Phillips R., Milo R. (2018). The Biomass Distribution on Earth. PNAS 115 (25), 6506–6511. doi: 10.1073/pnas.1711842115
Bax N., Sands C. J., Gogarty B., Downey R. V., Moreau C. V., Moreno B., et al. (2021). Perspective: Increasing Blue Carbon Around Antarctica is an Ecosystem Service of Considerable Societal and Economic Value Worth Protecting. Glob. Change Biol. 27 (1), 5–12. doi: 10.1111/gcb.15392
Belcher A., Henson S. A., Manno C., Hill S. L., Atkinson A., Thorpe S. E., et al. (2019). Krill Faecal Pellets Drive Hidden Pulses of Particulate Organic Carbon in the Marginal Ice Zone. Nat. Commun. 10 (1), 889. doi: 10.1038/s41467-019-08847-1
Bernal B., Murray L. T., Pearson T. R. H. (2018). Global Carbon Dioxide Removal Rates from Forest Landscape Restoration Activities. Carbon Balance Manag. 13(1), 22. doi: 10.1186/s13021-018-0110-8
Bindoff N. L., Cheung W. W., Kairo J. G., Arístegui J., Guinder V. A., Hallberg R., et al. (2019). “Changing Ocean, Marine Ecosystems, and Dependent Communities,” in IPCC Special Report on the Ocean and Cryosphere in a Changing Climate. Eds. Pörtner H.-O., Roberts D. C., Masson-Delmotte V., Zhai P., Tignor M., Poloczanska E., et al. (Cambridge, UK and New York, NY, USA: Cambridge University Press), pp. 447–587. doi: 10.1017/9781009157964.007
Brasier M. J., Barnes D., Bax N., Brandt A., Christianson A. B., Constable A. J., et al. (2021). Responses of Southern Ocean Seafloor Habitats and Communities to Global and Local Drivers of Change. Front. Mar. Sci. 8, 622721. doi: 10.3389/fmars.2021.622721
Brooks C. M., Chown S. L., Douglass L. L., Raymond B. P., Shaw J. D., Sylvester Z. T., et al. (2020). Progress Towards a Representative Network of Southern Ocean Protected Areas. PLoS One 15 (4), e0231361. doi: 10.1371/journal.pone.0231361
Cabré A., Marinov I., Leung S. (2015). Consistent Global Responses of Marine Ecosystems to Future Climate Change Across the IPCC AR5 Earth System Models. Clim. Dyn. 45 (5), 1253–1280. doi: 10.1007/s00382-014-2374-3
Canadell J. G., Monteiro P. M., Costa M. H., da Cunha L. C., Cox P. M., Eliseev A., et al. (2021). “Global Carbon and Other Biogeochemical Cycles and Feedbacks,” in Climate Change 2021: The Physical Science Basis. Contribution of Working Group I to the Sixth Assessment Report of the Intergovernmental Panel on Climate Change. Eds. Masson-Delmotte V., Zhai P., Pirani A., Connors S. L., Péan C., Berger S., et al. (Cambridge University Press).
Carr M.-E., Friedrichs M. A. M., Schmeltz M., Noguchi Aita M., Antoine D., Arrigo K. R., et al. (2006). A Comparison of Global Estimates of Marine Primary Production From Ocean Color. Deep Sea Res. Part II Top. Stud. Oceanogr. 53 (5), 741–770. doi: 10.1016/j.dsr2.2006.01.028
Cavanagh R. D., Melbourne-Thomas J., Grant S. M., Barnes D. K. A., Hughes K. A., Halfter S., et al. (2021). Future Risk for Southern Ocean Ecosystem Services Under Climate Change. Front. Mar. Sci. 7. doi: 10.3389/fmars.2020.615214
Cavan E. L., Belcher A., Atkinson A., Hill S. L., Kawaguchi S., McCormack S., et al. (2019). The Importance of Antarctic Krill in Biogeochemical Cycles. Nat. Commun. 10 (1), 4742. doi: 10.1038/s41467-019-12668-7
CBD. (2019). Voluntary Guidelines for the Design and Effective Implementation of Ecosystem-Based Approaches to Climate Change Adaptation and Disaster Risk Reduction and Supplementary Information.Technical Series No. 93 (Montreal), 156 pages.
Crooks S., Windham-Myers L., Troxler T. G. (2018). “Defining Blue Carbon: The Emergence of a Climate Context for Coastal Carbon Dynamics,” in A Blue Carbon Primer: The State of Coastal Wetland Carbon Science, Pratice and Policy. Eds. Windham-Myers L., Crooks S., Troxler T. G. (Boca Raton, Florida: CRC Press).
Crossland C. J., Hatcher B. G., Smith S. V. (1991). Role of Coral Reefs in Global Ocean Production. Coral Reefs 10 (2), 55–64. doi: 10.1007/BF00571824
Davidson N. C., Finlayson C. M. (2019). Updating Global Coastal Wetland Areas Presented in Davidson and Finlayson, (2018). Mar. Freshwater Res. 70 (8), 1195–1200. doi: 10.1071/MF19010
DeConto R. M., Pollard D., Alley R. B., Velicogna I., Gasson E., Gomez N., et al. (2021). The Paris Climate Agreement and Future Sea-Level Rise From Antarctica. Nature 593 (7857), 83–89. doi: 10.1038/s41586-021-03427-0
DeVries T., Primeau F., Deutsch C. (2012). The Sequestration Efficiency of the Biological Pump. Geophys. Res. Lett. 39 (L13601), 1–5. doi: 10.1029/2012GL051963
DeVries T., Weber T. (2017). The Export and Fate of Organic Matter in the Ocean: New Constraints From Combining Satellite and Oceanographic Tracer Observations. Global Biogeochem. Cycles 31 (3), 535–555. doi: 10.1002/2016GB005551
Di Nitto D., Neukermans G., Koedam N., Defever H., Pattyn F., Kairo J. G., et al. (2014). Mangroves Facing Climate Change: Landward Migration Potential in Response to Projected Scenarios of Sea Level Rise. Biogeosci. 11 (3), 857–871. doi: 10.5194/bg-11-857-2014
Duarte C. M., Chiscano C. L. (1999). Seagrass Biomass and Production: A Reassessment. Aquat. Bot. 65 (1), 159–174. doi: 10.1016/S0304-3770(99)00038-8
Duarte C. M., Krause-Jensen D. (2017). Export From Seagrass Meadows Contributes to Marine Carbon Sequestration. Front. Mar. Sci. 4, 13. doi: 10.3389/fmars.2017.00013
Dunne J. P., Armstrong R. A., Gnanadesikan A., Sarmiento J. L. (2005). Empirical and Mechanistic Models for the Particle Export Ratio. Global Biogeochem. Cycles 19. doi: 10.1029/2004GB002390
Durfort A., Mariani G., Troussellier M., Tulloch V., Mouillot D. (2020). The Collapse and Recovery Potential of Carbon Sequestration by Baleen Whales in the Southern Ocean. Res. Square 2020–10–23, P. Version 1. doi: 10.21203/rs.3.rs-92037/v1
Ellison A. M., Felson A. J., Friess D. A. (2020). Mangrove Rehabilitation and Restoration as Experimental Adaptive Management. Front. Mar. Sci. 7 (327). doi: 10.3389/fmars.2020.00327
Emerson S. (2014). Annual Net Community Production and the Biological Carbon Flux in the Ocean. Glob. Biogeochem. Cycles 28 (1), 14–28. doi: 10.1002/2013GB004680
EPA (2022) Greenhouse Gas Equivalencies Calculator. Available at: https://www.epa.gov/energy/greenhouse-gas-equivalencies-calculator (Accessed March 8, 2022).
Fauchald P., Arneberg P., Debernard J. B., Lind S., Olsen E., Hausner V. H. (2021). Poleward Shifts in Marine Fisheries Under Arctic Warming. Environ. Res. Lett. 16 (7), 074057. doi: 10.1088/1748-9326/ac1010
Filbee-Dexter K., Wernberg T. (2020). Substantial Blue Carbon in Overlooked Australian Kelp Forests. Sci. Rep. 10 (1), 12341. doi: 10.1038/s41598-020-69258-7
Friedlingstein P., O'Sullivan M., Jones M. W., Andrew R. M., Hauck J., Olsen A., et al. (2020). Global Carbon Budget 2020. Earth Syst. Sci. Data 12 (4), 3269–3340. doi: 10.5194/essd-12-3269-2020
Friess D. A., Rogers K., Lovelock C. E., Krauss K. W., Hamilton S. E., Lee S. Y., et al. (2019). The State of the World's Mangrove Forests: Past, Present, and Future. Annu. Rev. Environ. Resour. 44 (1), 89–115. doi: 10.1146/annurev-environ-101718-033302
Gallo N. D., Victor D. G., Levin L. A. (2017). Ocean Commitments Under the Paris Agreement. Nat. Clim. Change 7 (11), 833–838. doi: 10.1038/nclimate3422
Gjerde K. M., Rulska-Domino A. (2012). Marine Protected Areas Beyond National Jurisdiction: Some Practical Perspectives for Moving Ahead. Int. J. Mar. Coast Law 27 (2), 351–373. doi: 10.1163/157180812X633636
Hamilton S. E., Casey D. (2016). Creation of a High Spatio-Temporal Resolution Global Database of Continuous Mangrove Forest Cover for the 21st Century (CGMFC-21). Global Ecol. Biogeogr. 25 (6), 729–738. doi: 10.1111/geb.12449
Hansell D. A., Carlson C. A., Repeta D. J., Schlitzer R. (2009). Dissolved Organic Matter in the Ocean. A Controversy Simulates New Insights. Oceanogr. 22 (4), 202–211. doi: 10.5670/oceanog.2009.109
Henson S. A., Sanders R., Madsen E., Morris P. J., Le Moigne F., Quartly G. D. (2011). A Reduced Estimate of the Strength of the Ocean's Biological Carbon Pump. Geophys. Res. Lett. 38 (4), L04606 1–5. doi: 10.1029/2011GL046735
Hernández-León S., Koppelmann R., Fraile-Nuez E., Bode A., Mompeán C., Irigoien X., et al. (2020). Large Deep-Sea Zooplankton Biomass Mirrors Primary Production in the Global Ocean. Nat. Commun. 11 (1), 6048. doi: 10.1038/s41467-020-19875-7
Hoegh-Guldberg O., Jacob D., Taylor M., Guillén Bolaños T., Bindi M., Brown S., et al. (2019a). The Human Imperative of Stabilizing Global Climate Change at 1.5°C. Science 365 (1263), 1–11. doi: 10.1126/science.aaw6974
Hoegh-Guldberg O., Lovelock C., Caldeira K., Howard J., Chopin T., Gaines S. (2019b). The Ocean as a Solution to Climate Change: Five Opportunities for Action (Washington, DC: World Resources Institute).
Howard J., Sutton-Grier A., Herr D., Kleypas J., Landis E., Mcleod E., et al. (2017). Clarifying the Role of Coastal and Marine Systems in Climate Mitigation. Front. Ecol. Environ. 15 (1), 42–50. doi: 10.1002/fee.1451
Hurd C. L., Law C. S., Bach L. T., Britton D., Hovenden M., Paine E., et al. (2022). Forensic Carbon Accounting: Assessing the Role of Seaweeds for Carbon Sequestration. J. Phycol. doi: 10.1111/jpy.13249.
Huxham M., Whitlock D., Githaiga M., Dencer-Brown A. (2018). Carbon in the Coastal Seascape: How Interactions Between Mangrove Forests, Seagrass Meadows and Tidal Marshes Influence Carbon Storage. Curr. For. Rep. 4 (2), 101–110. doi: 10.1007/s40725-018-0077-4
Ilyina T., Wolf-Gladrow D., Munhoven G., Heinze C. (2013). Assessing the Potential of Calcium-Based Artificial Ocean Alkalinization to Mitigate Rising Atmospheric CO2 and Ocean Acidification. Geophys. Res. Lett. 40 (22), 5909–5914. doi: 10.1002/2013GL057981
In S. Y., Schumacher K. (2021). Carbonwashing: A New Type of Carbon Data-Related ESG Greenwashing. Stanford (Sustainable Finance Initiative,Working Paper), 1–25. doi: 10.2139/ssrn.3901278
IOC-UNESCO. (2020). Global Ocean Science Report 2020–Charting Capacity for Ocean Sustainability. Ed. Isensee K. (Paris: UNESCO Publishing).
IPBES-IPCC (2021). Co-Sponsored Workshop Report on Biodiversity and Climate Change. IPBES and IPCC. doi: 10.5281/zenodo.4782538
IPCC. (2000). "Summary for Policymakers Land Use, Land-Use Change, and Forestry," in A Special Report of the IPCC (UK: Cambridge University Press), pp 375
IPCC. (2014). 2013 Supplement to the 2006 IPCC Guidelines for National Greenhouse Gas Inventories: Wetlands. Eds. Hiraishi T., Krug T., Tanabe K., Srivastava N., Baasansuren J., Fukuda M., Troxler T. G. (Switzerland: IPCC).
IPCC. (2018). Global Warming of 1.5°C. An IPCC Special Report on the Impacts of Global Warming of 1.5°C Above Pre-Industrial Levels and Related Global Greenhouse Gas Emission Pathways, in the Context of Strengthening the Global Response to the Threat of Climate Change, Sustainable Development, and Efforts to Eradicate Poverty. Eds. Masson-Delmotte V., Zhai P., Pörtner H. O., Roberts D., Skea J., Shukla P. R., et al (In Press).
IPCC. (2019a). Special Report on the Ocean and Cryosphere in a Changing Climate. Eds. Pörtner H.-O., Roberts D. C., Masson-Delmotte V., Zhai P., Tignor M., Poloczanska E., et al. (Cambridge, UK and New York, NY, USA: IPCC), pp. 3–35. doi: 10.1017/9781009157964.001
IPCC (2019b). “2019 Refinement to the 2006 IPCC Guidelines for National Greenhouse Gas Inventories: Wetlands,” in Volume 4: Agriculture, Forestry and Other Land Use. Eds. Buendia C., Tanabe K., Kranjc A., Baasansuren J., Fukuda M., Ngarize S., et al. (Switzerland: IPCC).
IPCC. (2021). “Climate Change 2021: The Physical Science Basis,” in Contribution of Working Group I to the Sixth Assessment Report of the Intergovernmental Panel on Climate Change. Eds. Masson-Delmotte V., Zhai P., Pirani A., Connors S. L., Péan C., Berger S., et al
IPCC (2022). “Climate Change 2022: Impacts, Adaptation, and Vulnerability,” in Contribution of Working Group II to the Sixth Assessment Report of the Intergovernmental Panel on Climate Change. Eds. Pörtner H.-O., Roberts D. C., Tignor M., Poloczanska E. S., Mintenbeck K., Alegría A., et al (Cambridge University Press).
IUCN. (2016). Nature-Based Solutions to Address Global Societal Challenges. Eds. Cohen-Shacham E., Walters G., Janzen C., Maginnis S. (Gland, Switzerland: IUCN). doi: 10.2305/IUCN.CH.2016.13.en
IUCN. (2020). Guidance for Using the IUCN Global Standard for Nature-Based Solutions. A User-Friendly Framework for the Verification, Design and Scaling Up of Nature-Based Solutions. First Edition (Gland, Switzerland: IUCN).
Jørgensen S. V., Hauschild M. Z. (2013). Need for Relevant Timescales When Crediting Temporary Carbon Storage. Int. J. Life Cycle Assess. 18 (4), 747–754. doi: 10.1007/s11367-012-0527-3
Jakovac C. C., Latawiec A. E., Lacerda E., Leite Lucas I., Korys K. A., Iribarrem A., et al. (2020). Costs and Carbon Benefits of Mangrove Conservation and Restoration: A Global Analysis. Ecol. Econ. 176, 106758. doi: 10.1016/j.ecolecon.2020.106758
Kawaguchi S., Ishida A., King R., Raymond B., Waller N., Constable A., et al. (2013). Risk Maps for Antarctic Krill Under Projected Southern Ocean Acidification. Nat. Clim. Change 3 (9), 843–847. doi: 10.1038/nclimate1937
Kharbush J. J., Close H. G., Van Mooy B. A. S., Arnosti C., Smittenberg R. H., Le Moigne F. A. C., et al. (2020). Particulate Organic Carbon Deconstructed: Molecular and Chemical Composition of Particulate Organic Carbon in the Ocean. Front. Mar. Sci. 7 (518). doi: 10.3389/fmars.2020.00518
Klein E. S., Hill S. L., Hinke J. T., Phillips T., Watters G. M. (2018). Impacts of Rising Sea Temperature on Krill Increase Risks for Predators in the Scotia Sea. PLoS One 13 (1), e0191011. doi: 10.1371/journal.pone.0191011
Krause-Jensen D., Duarte C. M. (2016). Substantial Role of Macroalgae in Marine Carbon Sequestration. Nat. Geosci 9 (10), 737–742. doi: 10.1038/ngeo2790
Krause-Jensen D., Lavery P., Serrano O., Marbà N., Masque P., Duarte C. M. (2018). Sequestration of Macroalgal Carbon: The Elephant in the Blue Carbon Room. Biol. Lett. 14 (6), 20180236. doi: 10.1098/rsbl.2018.0236
Krumhansl K. A., Scheibling R. E. (2012). Production and Fate of Kelp Detritus. Mar. Ecol. Prog. Ser. 467, 281–302. doi: 10.3354/meps09940
Laffoley D. (2020). Protecting and Effectively Managing Blue Carbon Ecosystems to Realise the Full Value to Society – a Sea of Opportunities. An Opinion Piece by Dan Laffoley for WWF-Uk (UK: Woking, Surrey), 42 pp.
Lavery T. J., Roudnew B., Gill P., Seymour J., Seuront L., Johnson G., et al. (2010). Iron Defecation by Sperm Whales Stimulates Carbon Export in the Southern Ocean. Proc. R. Soc B 277 (1699), 3527–3531. doi: 10.1098/rspb.2010.0863
Laws E. A., Falkowski P. G., Smith W. O. Jr., Ducklow H., McCarthy J. J. (2000). Temperature Effects on Export Production in the Open Ocean. Global Biogeochem. Cycles 14 (4), 1231–1246. doi: 10.1029/1999GB001229
Lecerf M., Herr D., Thomas T., Elverum C., Delrieu E., Picourt L. (2021). Coastal and Marine Ecosystems as Nature-Based Solutions in New or Updated Nationally Determined Contributions (Ocean & Climate Platform Conservation International, IUCN, GIZ, Rare, The Nature Conservancy and WWF).
Lee H. Z. L., Davies I. M., Baxter J. M., Diele K., Sanderson W. G. (2020). Missing the Full Story: First Estimates of Carbon Deposition Rates for the European Flat Oyster, Ostrea Edulis. Aquat. Conserv. Mar. Freshwater Ecosyst. 30 (11), 2076–2086. doi: 10.1002/aqc.3402
Lee S. Y., Hamilton S., Barbier E. B., Primavera J., Lewis R. R. (2019). Better Restoration Policies are Needed to Conserve Mangrove Ecosystems. Nat. Ecol. Evol. 3 (6), 870–872. doi: 10.1038/s41559-019-0861-y
Leung S. W., Weber T., Cram J. A., Deutsch C. (2021). Variable Particle Size Distributions Reduce the Sensitivity of Global Export Flux to Climate Change. Biogeosciences 18 (1), 229–250. doi: 10.5194/bg-18-229-2021
Lovelock C. E., Brown B. M. (2019). Land Tenure Considerations are Key to Successful Mangrove Restoration. Nat. Ecol. Evol. 3 (8), 1135–1135. doi: 10.1038/s41559-019-0942-y
Luisetti T., Ferrini S., Grilli G., Jickells T. D., Kennedy H., Kröger S., et al. (2020). Climate Action Requires New Accounting Guidance and Governance Frameworks to Manage Carbon in Shelf Seas. Nat. Commun. 11 (1), 4599. doi: 10.1038/s41467-020-18242-w
Macreadie P. I., Anton A., Raven J. A., Beaumont N., Connolly R. M., Friess D. A., et al. (2019). The Future of Blue Carbon Science. Nat. Commun. 10 (1), 3998. doi: 10.1038/s41467-019-11693-w
Macreadie P. I., Serrano O., Maher D. T., Duarte C. M., Beardall J. (2017). Addressing Calcium Carbonate Cycling in Blue Carbon Accounting. Limnol. Oceanogr. Lett. 2 (6), 195–201. doi: 10.1002/lol2.10052
Maldonado M. T., Surma S., Pakhomov E. A. (2016). Southern Ocean Biological Iron Cycling in the Pre-Whaling and Present Ecosystems. Philos. Trans. R. Soc London Ser. A 374 (2081), 20150292. doi: 10.1098/rsta.2015.0292
Mariani G., Cheung W. W. L., Lyet A., Sala E., Mayorga J., Velez L., et al. (2020). Let More Big Fish Sink: Fisheries Prevent Blue Carbon Sequestration—Half in Unprofitable Areas. Sci. Adv. 6 (44), eabb4848. doi: 10.1126/sciadv.abb4848
Martin J. H., Knauer G. A., Karl D. M., Broenkow W. W. (1987). VERTEX: Carbon Cycling in the Northeast Pacific. Deep Sea Res. Part A 34 (2), 267–285. doi: 10.1016/0198-0149(87)90086-0
März C., Freitas F. S., Faust J. C., Godbold J. A., Henley S. F., Tessin A. C., et al. (2022). Biogeochemical Consequences of a Changing Arctic Shelf Seafloor Ecosystem. Ambio. 51, 370–382. doi: 10.1007/s13280-021-01638-3
McKenzie L. J., Nordlund L. M., Jones B. L., Cullen-Unsworth L. C., Roelfsema C., Unsworth R. K. F. (2020). The Global Distribution of Seagrass Meadows. Environ. Res. Lett. 15 (7), 074041. doi: 10.1088/1748-9326/ab7d06
McKinley G. A., Fay A. R., Takahashi T., Metzl N. (2011). Convergence of Atmospheric and North Atlantic Carbon Dioxide Trends on Multidecadal Timescales. Nat. Geosci 4 (9), 606–610. doi: 10.1038/ngeo1193
McLeod E., Salm R. V. (2006). Managing Mangroves for Resilience to Climate Change (Gland, Switzerland: IUCN).
Meredith M., Sommerkorn M., Cassotta S., Derksen C., Ekaykin A., Hollowed A., et al. (2019). “Polar Regions,” in Special Report on the Ocean and Cryosphere in Changing Climate. Eds. Pörtner H.-O., Roberts D. C., Masson-Delmotte V., Zhai P., Tignor M., Poloczanska E., et al(Cambridge, UK and New York, NY, USA Cambridge University Press). pp. 203–320. doi: 10.1017/9781009157964.005
Mora C., Andrèfouët S., Costello M. J., Kranenburg C., Rollo A., Veron J., et al. (2006). Coral Reefs and the Global Network of Marine Protected Areas. Science 312 (5781), 1750–1751. doi: 10.1126/science.1125295
Nordlund L. M., Koch E. W., Barbier E. B., Creed J. C. (2016). Seagrass Ecosystem Services and Their Variability Across Genera and Geographical Regions. PLoS One 11 (10), e0163091. doi: 10.1371/journal.pone.0163091
Ortega A., Geraldi N. R., Alam I., Kamau A. A., Acinas S. G., Logares R., et al. (2019). Important Contribution of Macroalgae to Oceanic Carbon Sequestration. Nat. Geosci. 12, 748–754. doi: 10.1038/s41561-019-0421-8
Our Shared Seas. (2022). A Decade of Ocean Funding: Landscape Trends 2010–2020. Available at: https://oursharedseas.com/funding/ (Accessed March 8, 2022).
Peck L. S., Barnes D. K. A., Cook A. J., Fleming A. H., Clarke A. (2010). Negative Feedback in the Cold: Ice Retreat Produces New Carbon Sinks in Antarctica. Glob. Change Biol. 16 (9), 2614–2623. doi: 10.1111/j.1365-2486.2009.02071.x
Pendleton L., Donato D. C., Murray B. C., Crooks S., Jenkins W. A., Sifleet S., et al. (2012). Estimating Global “Blue Carbon” Emissions From Conversion and Degradation of Vegetated Coastal Ecosystems. PLoS One 7 (9), e43542. doi: 10.1371/journal.pone.0043542
Pershing A. J., Christensen L. B., Record N. R., Sherwood G. D., Stetson P. B. (2010). The Impact of Whaling on the Ocean Carbon Cycle: Why Bigger was Better. PLoS One 5 (8), e12444. doi: 10.1371/journal.pone.0012444
Pineda-Metz S. E. A., Gerdes D., Richter C. (2020). Benthic Fauna Declined on a Whitening Antarctic Continental Shelf. Nat. Commun. 11 (1), 2226. doi: 10.1038/s41467-020-16093-z
Pusceddu A., Bianchelli S., Martín J., Puig P., Palanques A., Masqué P., et al. (2014). Chronic and Intensive Bottom Trawling Impairs Deep-Sea Biodiversity and Ecosystem Functioning. PNAS 111 (24), 8861–8866. doi: 10.1073/pnas.1405454111
Queirós A. M., Stephens N., Widdicombe S., Tait K., McCoy S. J., Ingels J., et al. (2019). Connected Macroalgal-Sediment Systems: Blue Carbon and Food Webs in the Deep Coastal Ocean. Ecol. Monogr. 89, e01366. doi: 10.1002/ecm.1366
Roberts C. M., O’Leary B. C., McCauley D. J., Cury P. M., Duarte C. M., Lubchenco J., et al. (2017). Marine Reserves can Mitigate and Promote Adaptation to Climate Change. PNAS 114 (24), 6167–6175. doi: 10.1073/pnas.1701262114
Roshan S., DeVries T. (2017). Efficient Dissolved Organic Carbon Production and Export in the Oligotrophic Ocean. Nat. Commun. 8 (1), 2036. doi: 10.1038/s41467-017-02227-3
Saba G. K., Burd A. B., Dunne J. P., Hernández-León S., Martin A. H., Rose K. A., et al. (2021). Toward a Better Understanding of Fish-Based Contribution to Ocean Carbon Flux. Limnol. Oceanogr. Lett. 66 (5), 1639–1664. doi: 10.1002/lno.11709
Sala E., Mayorga J., Bradley D., Cabral R. B., Atwood T. B., Auber A., et al. (2021). Protecting the Global Ocean for Biodiversity, Food and Climate. Nature 592 (7854), 397–402. doi: 10.1038/s41586-021-03371-z
SBSTA. (2021). Informal Summary Report by the Chair of the Subsidiary Body for Scientific and Technological Advice (UNFCCC. Subsidiary Body for Scientific and Technological Advice (SBSTA)). Available at: https://unfccc.int/documents/279105.
Schlitzer R. (2002). Carbon Export Fluxes in the Southern Ocean: Results From Inverse Modeling and Comparison With Satellite-Based Estimates. Deep Sea Res. Part II Top. Stud. Oceanogr. 49 (9), 1623–1644. doi: 10.1016/S0967-0645(02)00004-8
Seddon N., Chausson A., Berry P., Girardin C. A. J., Smith A., Turner B. (2020). Understanding the Value and Limits of Nature-Based Solutions to Climate Change and Other Global Challenges. Philos. Trans. R. Soc London Ser. B 375 (1794), 20190120. doi: 10.1098/rstb.2019.0120
Seddon N., Smith A., Smith P., Key I., Chausson A., Girardin C., et al. (2021). Getting the Message Right on Nature-Based Solutions to Climate Change. Glob. Change Biol. 27 (8), 1518–1546. doi: 10.1111/gcb.15513
Siegel D. A., Buesseler K. O., Doney S. C., Sailley S. F., Behrenfeld M. J., Boyd P. W. (2014). Global Assessment of Ocean Carbon Export by Combining Satellite Observations and Food-Web Models. Glob. Biogeochem. Cycles 28 (3), 181–196. doi: 10.1002/2013GB004743
Siegel D. A., DeVries T., Doney S., Bell T. (2021). Assessing the Sequestration Time Scales of Some Ocean-Based Carbon Dioxide Reduction Strategies. Environ. Res. Lett 16, 104003. doi: 10.1088/1748-9326/ac0be0
Souster T. A., Barnes D. K. A., Hopkins J. (2020). Variation in Zoobenthic Blue Carbon in the Arctic's Barents Sea Shelf Sediments. Philos. Trans. R. Soc A 378 (2181), 20190362. doi: 10.1098/rsta.2019.0362
Su J., Friess D. A., Gasparatos A. (2021). A Meta-Analysis of the Ecological and Economic Outcomes of Mangrove Restoration. Nat. Commun. 12 (1), 5050. doi: 10.1038/s41467-021-25349-1
Taillardat P., Friess D. A., Lupascu M. (2018). Mangrove Blue Carbon Strategies for Climate Change Mitigation are Most Effective at the National Scale. Biol. Lett. 14 (10), 20180251. doi: 10.1098/rsbl.2018.0251
Tittensor D. P., Novaglio C., Harrison C. S., Heneghan R. F., Barrier N., Bianchi D., et al. (2021). Next-Generation Ensemble Projections Reveal Higher Climate Risks for Marine Ecosystems. Nat. Clim. Change 11, 973–981. doi: 10.1038/s41558-021-01173-9
Tulloch V. J. D., Plagányi É.E., Brown C., Richardson A. J., Matear R. (2019). Future Recovery of Baleen Whales is Imperiled by Climate Change. Glob. Change Biol. 25 (4), 1263–1281. doi: 10.1111/gcb.14573
Tzortziou M., Neale P. J., Megonigal J. P., Pow C. L., Butterworth M. (2011). Spatial Gradients in Dissolved Carbon Due to Tidal Marsh Outwelling Into a Chesapeake Bay Estuary. Mar. Ecol. Prog. Ser. 426, 41–56. doi: 10.3354/meps09017
Ullman R., Bilbao-Bastida V., Grimsditch G. (2013). Including Blue Carbon in Climate Market Mechanisms. Ocean Coast. Manage. 83, 15–18. doi: 10.1016/j.ocecoaman.2012.02.009
UNESCO. (2020). UNESCO Marine World Heritage: Custodians of the Globe’s Blue Carbon Assets (Paris, France: UNESCO).
van Kooten G. C., de Vries F. P. (2013). “Carbon Offsets,” in Encyclopedia of Energy, Natural Resource, and Environmental Economics. Ed. Shogren J. F. (Waltham: Elsevier), 6–8.
Visalli M. E., Best B. D., Cabral R. B., Cheung W. W. L., Clark N. A., Garilao C., et al. (2020). Data-Driven Approach for Highlighting Priority Areas for Protection in Marine Areas Beyond National Jurisdiction. Mar. Policy 122, 103927. doi: 10.1016/j.marpol.2020.103927
Watters G. M., Hinke J. T., Reiss C. S. (2020). Long-Term Observations From Antarctica Demonstrate That Mismatched Scales of Fisheries Management and Predator-Prey Interaction Lead to Erroneous Conclusions About Precaution. Sci. Rep. 10 (1), 2314. doi: 10.1038/s41598-020-59223-9
Zeng Y., Friess D. A., Sarira T. V., Siman K., Koh L. P. (2021). Global Potential and Limits of Mangrove Blue Carbon for Climate Change Mitigation. Curr. Biol. 31 (8), 1737–1743, e1733. doi: 10.1016/j.cub.2021.01.070
Keywords: blue carbon, climate change, coastal wetlands, mitigation, nature-based solution, carbon sequestration, additionality
Citation: Christianson AB, Cabré A, Bernal B, Baez SK, Leung S, Pérez-Porro A and Poloczanska E (2022) The Promise of Blue Carbon Climate Solutions: Where the Science Supports Ocean-Climate Policy. Front. Mar. Sci. 9:851448. doi: 10.3389/fmars.2022.851448
Received: 09 January 2022; Accepted: 30 March 2022;
Published: 29 April 2022.
Edited by:
Kum Fai Yuen, Nanyang Technological University, SingaporeReviewed by:
Chester John Sands, British Antarctic Survey (BAS), United KingdomNicholas D. Higgs, Cape Eleuthera Institute, Bahamas
Min Wu, Nanyang Technological University, Singapore
Copyright © 2022 Christianson, Cabré, Bernal, Baez, Leung, Pérez-Porro and Poloczanska. This is an open-access article distributed under the terms of the Creative Commons Attribution License (CC BY). The use, distribution or reproduction in other forums is permitted, provided the original author(s) and the copyright owner(s) are credited and that the original publication in this journal is cited, in accordance with accepted academic practice. No use, distribution or reproduction is permitted which does not comply with these terms.
*Correspondence: Anne B. Christianson, Q2hyaTE5NDJAdW1uLmVkdQ==