- 1Department of Biology, Marine Ecology Laboratory, University of Crete, Heraklion, Greece
- 2Hellenic Centre for Marine Research, Institute of Oceanography, Heraklion, Greece
- 3Hellenic Centre for Marine Research, Institute of Marine Biology, Biotechnology and Aquaculture, Heraklion, Greece
- 4Cyprus Marine and Maritime Institute, Larnaca, Cyprus
- 5Hellenic Centre for Marine Research, Institute of Marine Biological Resources and Inland Waters, Heraklion, Greece
Benthic–pelagic coupling studies have shown that the response of the benthic system to eutrophication is subject to complex nonlinear dynamics with specific thresholds beyond which abrupt changes in the response of the ecosystem occur and time lags between inputs and responses. The “HYPOXIA: Benthic–pelagic coupling and regime shifts” project aimed to investigate how nutrient input in the water column results in ecological processes of eutrophication, which may lead to significant, irreversible changes in the eastern Mediterranean marine ecosystems within a short period of time. The project included analysis of historical water and benthic data, field sampling, and mesocosm experiments. From the project results, it can be concluded that nutrient inputs are quickly capitalized by small phytoplankton species in the water column resulting in the bloom of specific species with high nutrient uptake capabilities. When Eutrophic Index values (calculated using nutrient and chlorophyll-a concentrations) cross the moderate-to-poor threshold, the precipitating organic matter can cause observable effects on the benthic system. Depending on eutrophication intensity and persistence, the effects can start from microbenthos, meiofauna, and macrofauna increase in abundance and biomass to significant changes in the community structure. The latter includes the proliferation of macrofaunal opportunistic species, an increase in deposit feeders, and the high risk of ecosystem quality degradation. However, contrary to other regions of the world, no water hypoxia or benthic dead zones were observed as chlorophyll-a and O2 concentrations showed a positive correlation. This is caused by the high photosynthetic activity of the phytoplankton and microphytobenthos, the increased bioturbation of macrofauna, and the increased abundance of sediment deposit-feeding species, which quickly consume the excess organic matter. Eastern Mediterranean coastal ecosystems show high resilience to the adverse effects of eutrophication, preventing hypoxia and azoic conditions when eutrophication is the only source of environmental disturbance.
Introduction
Traditionally, the research on eutrophication has focused mainly on phytoplankton, but it has now become obvious that eutrophication affects all components of coastal ecosystems, and it is therefore necessary to identify those impacts on both water and benthos (Duarte, 2009). Different regions around the globe show major differences regarding eutrophication effects on coastal ecosystems, with some regions being under significant pressure (i.e., Baltic Sea, the Northern gulf of Mexico, and regions in the North Atlantic) while others have localized problems (i.e., eastern Mediterranean or Australia) (Boesch, 2019). To address this issue, it is commonly accepted that management actions need to be taken to reduce nutrient loads and identify the status of the coastal ecosystems through environmental monitoring (Andersen et al., 2019).
After extensive effort in some regions facing periodic hypoxic events, a significant reduction of nutrient input has been recently reported, ranging from 25% (Chesapeake Bay) to 100% (Tampa Bay) (Duarte and Krause-Jensen, 2018). However, it is possible that even under an oligotrophication of a system, neither the chlorophyll-a (Chl-a) in the water column returns nor the benthos recovers fully to pre-conditions of hypoxia (Diaz and Rosenberg, 2008). Several studies have shown that the response of the ecosystem eutrophication is subject to complex nonlinear dynamics. According to Duarte (2009), causes for this nonlinearity are (a) occurrence of thresholds of nutrient inputs beyond which abrupt changes in the response of the ecosystem occur (e.g., hypoxia); (b) occurrence of time lags in the responses due to the accumulation and release of nutrients in the sediments or the long time spans involved in the recovery of some inherently slow-growing organisms, such as seagrasses; or (c) changes in the basis for comparison—shifting baselines (Duarte et al., 2009) altering the relationship between Chl-a and nutrient inputs over time.
In theory, high production of organic matter due to eutrophication in the water column causes sedimentation of particulate organic matter (POM) and increased oxygen demand, which gradually decreases the available dissolved oxygen (DO), therefore leading to benthic hypoxia. The first signs are a reduction of the layer with a positive redox potential (Eh), i.e., the depth of the Redox potential discontinuity (RPD) layer. Typically, the threshold for hypoxia used in the literature is 2 mg O2 L−1 (Diaz, 2001; Diaz and Rosenberg, 2008). However, Vaquer-Sunyer and Duarte (2008), analyzing experimental data in a large number of studies for various species, found that there are significant differences between taxonomic groups regarding their tolerance to minimal oxygen availability and concluded that the number and extent of coastal ecosystems affected by hypoxia have been underestimated.
Attempts to develop quantitative benthic–pelagic coupling models are available in the literature from Graf (1992) focusing on organic carbon (Vaquer-Sunyer and Duarte, 2008) relating primary production to the rate of oxygen consumption in the sediment, organic matter (Brady et al., 2013), and different biogeochemical variables (Ubertini et al., 2012), and recently from Zhang et al. (2021) who highlighted the importance of bioturbation to benthic–pelagic coupling models. However, the most challenging issue is the identification of a eutrophication key point beyond which significant changes in the status of the benthic system are expected. Such a point has only been mentioned in Comprehensive Studies for the Purposes of Article 6 of Directive 91/271 EEC (CSTT, 1997), which states that a Chl-a concentration in the water column greater than 10 Mg L−1, during the summer months, would be capable of causing anoxia conditions in the seabed. This value, although extensively republished, is essentially an expert judgement estimate that is not based on any published field or experimental data (Dimitriou et al., 2015).
In the water column, dissolved inorganic nutrient input (natural or anthropogenic) will likely result in a phytoplankton bloom (Bracken et al., 2015). Usually, micro-autotrophs will rapidly capitalize the available nutrients and increase their biomass, followed by species of slower metabolic dynamics (Lin et al., 2016). Furthermore, it is possible that different types of dissolved organic matter (DOM) may result in different bacterial communities (Teeling et al., 2012). Therefore, the response of different plankton communities to inorganic and/or organic nutrient addition may show significant variation (Santi et al., 2019).
Benthic macrofauna is possibly the most common biotic indicator characterizing the quality of marine ecosystems in environmental monitoring programs (Gray, 1981), because it integrates the effects that took place in time periods prior to the sampling event. The empirical model of hierarchical response to stress and the description of the succession pattern of macrofauna in gradients of organic enrichment (Pearson and Rosenberg, 1978) have been confirmed in hundreds of published papers worldwide. This general model was calibrated with values of total organic carbon (TOC) by Hyland et al. (2005) using a large global dataset. In recent years, in Europe, for the implementation of the Water Framework Directive and Marine Strategy Framework Directive, there is intense scientific interest for the study of eutrophication effects on benthos and the associated ecological status indicators in the eastern Mediterranean (ES) (Simboura and Argyrou, 2010; Pavlidou et al., 2015).
Except for macrofauna, which is now an established indicator of anthropogenic disturbance, other components of the benthic ecosystem have also been used to assess the effects of anthropogenic activities (including eutrophication) on ecosystem health, such as meiofauna (Schratzberger and Ingels, 2018) or microfauna (Aylagas et al., 2017). Furthermore, Apostolaki et al. (2007) have shown that in some habitats, the effects of disturbance on macrofauna may be limited, while at the same time (and at the same place), the impact on marine phanerogams, especially Posidonia oceanica, might be highly detrimental.
The “HYPOXIA: Benthic–pelagic coupling and regime shifts” project (2014–2016), funded by the Greek General Secretariat for Research and Technology (GSRT) in the framework of the Operational Program “Education and Lifelong Learning” of the National Strategic Reference Framework (NSRF)—ARISTEIA II (HYPOXIA project, No. 5381), aimed to investigate how nutrient input in the water column results in ecological processes of eutrophication, which may lead to significant, irreversible changes in the benthic marine ecosystems within a short period of time. Following the suggestion of Duarte (2009), the project investigated the response of various components of the ecosystem to eutrophication and the consequently induced organic enrichment of the benthos. The inclusion of different groups of organisms and biogeochemical processes, particularly at various spatial and temporal scales, served to further shed light on different aspects of the problem.
Description of the Project
The project included three independent approaches with different advantages and limitations: analysis of historical data, field sampling, and a mesocosm experiment (large enclosed experimental ecosystems). Within each approach, different components of the ecosystem were studied including plankton communities, microbial benthos, meiofaunal and macrofaunal communities, as well as environmental variables and ecological status indicators (Table 1).
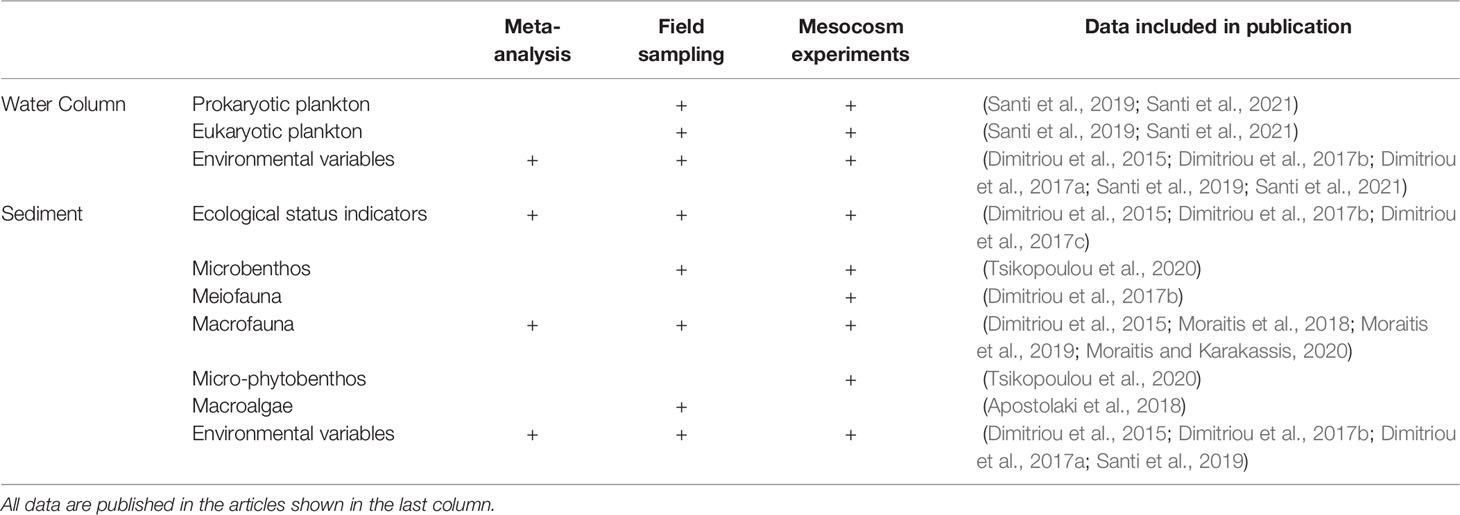
Table 1 Biotic and abiotic variables and communities studied in each approach of the HYPOXIA project.
The main objective of the analysis of historical data was to assemble a large dataset of stations, involving both water column and benthic data aiming to detect the response of the benthic system to a eutrophication gradient in the overlying water column. Overall, it included 126 sampling stations; 34 in the Ionian Sea, 68 in the Northern Aegean Sea, and 24 in the Southern Aegean or Cretan Sea (Figure 1). In order to reduce variability, specific inclusion criteria were set: Sediment and water had to be sampled simultaneously, with sampling taking place during spring or early summer, and more importantly, sites receiving allochthonous organic material (e.g., wastes from fish farms and sewage) were excluded from the analysis. The resulting stations were all sampled with a Niskin bottle in the water column and a 0.1 m2 grab or a 0.052 box corer in the sediment. Depth ranged from 5 m to 70 m (MD = 27 ± 15 m). Chl-a needed to be filtered in a 0.2-Mm filter and macrofauna needed to be identified to the species level or the lowest possible taxon.
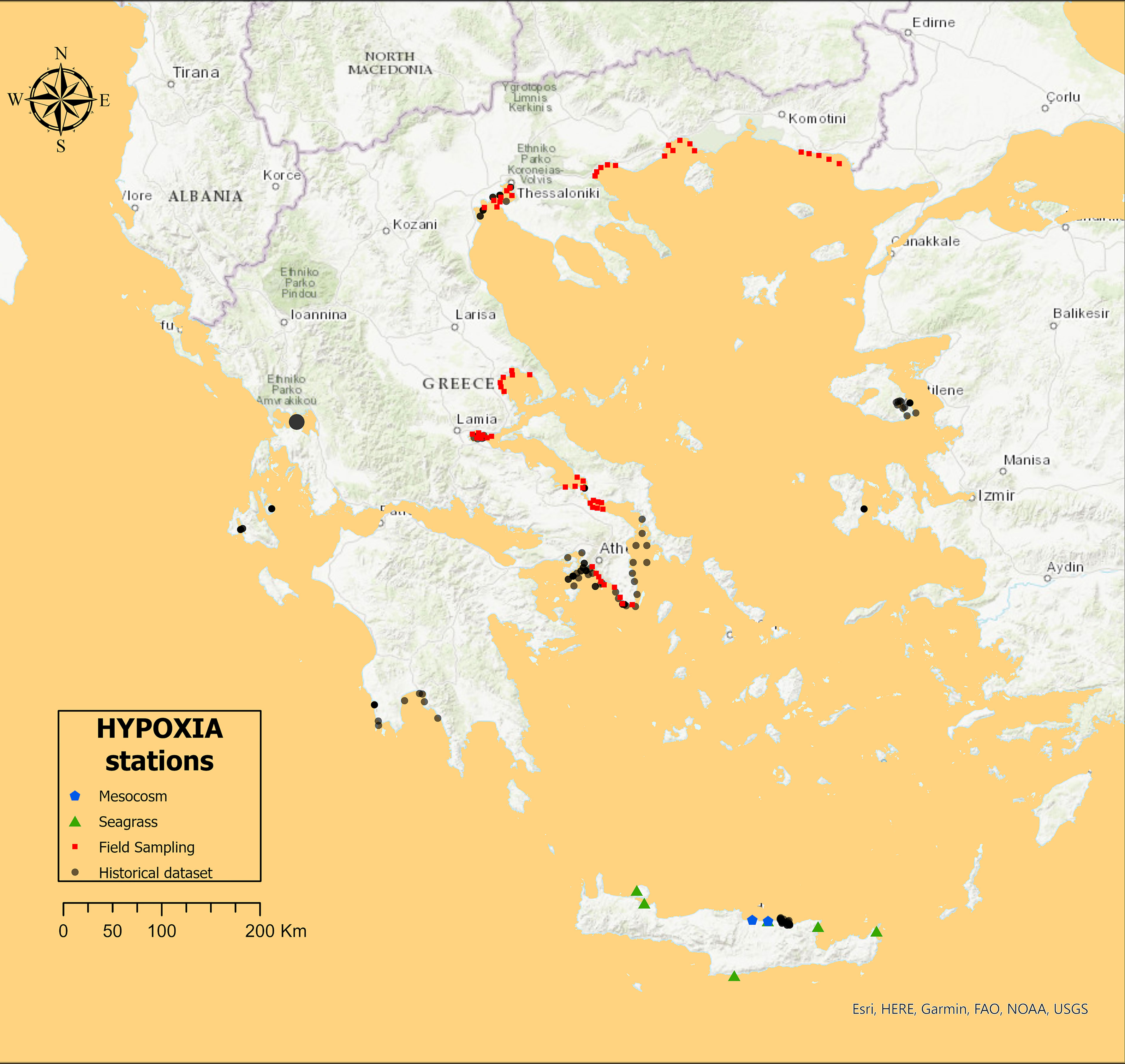
Figure 1 The sampling stations included in the HYPOXIA project, in the Aegean Sea and the Ionian Sea of the east Mediterranean Sea.
The field sampling campaign was carried out in areas with known high, medium, and low Chl-a concentration as classified using the Greek Chl-a scale (Simboura et al., 2015) and determined through remote sensing techniques. The objective was to sample different components of the marine ecosystem (benthic and planktonic) (Table 1), with the same sampling protocol (0.1 m2 grab and Niskin bottles), same bottom depth (20 m), and at the same season (late spring). Overall, it included 59 sampling stations from gulfs in the Aegean Sea (Figure 1). The design included sampling of the water column at the surface (2 m) and bottom (20 m) depths as well as of the sediment, studying both water and benthic communities and environmental variables at the same time. The field sampling and the consecutive laboratory analyses for common variables were performed using the same sampling equipment and analytical protocols as with the historical samples.
Finally, the main objective of the mesocosm experiment was to induce a perturbation beyond the usually high levels found normally in the Aegean to assess the effects of possible extreme nutrient additions. This was achieved through a sudden and extremely high nutrient enrichment in the water column and subsequently by investigating the response (i) of prokaryotic and eukaryotic plankton to nutrient enrichment, and (ii) of different benthic communities and environmental variables to the increased OM precipitation, over a 60-day experimental duration. The mesocosms used were developed specifically for benthic–pelagic coupling experiments and included an enclosed water column part (4 m deep, total volume 1.5 m3) and a sediment part in the bottom (0.3 m height, total volume 85 L). There were two levels of tion, codnutrient addienamed “low” (concentration after addition: 5 μM and 30 μM ) and “high” (concentration after addition: 10 μM and 60 μM ), as well as “control” mesocosms (no addition); both treatments and the control mesocosms were triplicated. The experiment took place in autumn 2014 (September–October), in the CRETACOSM mesocosm facilities of the Hellenic Center for Marine Research in Crete. The mesocosms were filled with seawater pumped from a coastal area in the vicinity of the CRETACOSM facilities and used to fill the mesocosms. The sediment was collected from the leisure boat area of the port of Heraklion (Figure 1), where annual values of water column Chl-a concentration ranged from 0.4 Mg L−1 in spring to 1.4 Mg L−1 during autumn. Sediment was collected using a box corer, gently placed into the containers and transported to the mesocosm facilities. Water samples were collected every 3 or 6 days and sediment corers were collected every 6 days using a corer sampler design especially for this study. All laboratory analyses (Table 1) used the same protocols with the field sampling. See Dimitriou et al. (2017b) for more details about the mesocosm design and sampling methodology, and see Dimitriou et al. (2017a) and Santi et al. (2019) for laboratory protocols.
Key Findings in Each Approach
Historical Data Analysis
Analysis of historical data from 126 coastal sites in the Aegean Sea and the Ionian Sea (Greece) revealed a negative correlation of the water column variables measuring eutrophication Chl-a scale (Simboura et al., 2012) and Eutrophic Index (EI) (Primpas et al., 2010) with the Eh of underlying marine sediments as well as the ES, as measured by means of benthic macrofauna. Macrofaunal opportunistic species abruptly increased in abundance (the stations being classified as poor or bad ES) after the threshold of moderate to poor ES was crossed in the water column, using Chl-a or EI as indicators. The most important finding was that no hypoxia was detected in any of these stations and that DO was positively correlated to water Chl-a concentration [i.e., highly eutrophic stations had high DO availability in the benthic boundary layer (BBL)]. Furthermore, despite the fact that Eh had a negative correlation to Chl-a, the lowest value recorded was −122 mV, a value suboxic but higher than Eh values recorded in studies focusing on organic enrichment with allochthonous OM from other sources such as aquaculture (Papageorgiou et al., 2010) or ports (Chatzinikolaou et al., 2018) in nearby regions.
Field Sampling
The results of the field sampling showed that despite the high Chl-a content in the water column (ranging from 0.06 to 2.04 Mg L−1) and from poor to good ES using the EI index, the DO at the BBL was in all cases above standard hypoxia levels and again positively correlated to the Chl-a concentration (Moraitis et al., 2018). Measured Chl-a was within the range recorded in the east Mediterranean in the context of monitoring programs. The plankton community was dominated by pico- and micro-planktonic groups with high nutrient uptake capability (Santi, 2019). The composition of the microbial plankton community was significantly different between the high and low Chl-a areas and the high Chl-a areas were characterized by low diversity (Santi, 2019).
In the sediment, the majority of the stations were classified by high sand content (mud content ranged from 5% to 20%). The change in trophic conditions was reflected on sediment Eh (−20 to +380 mV) with a negative correlation to Chl-a. However, the benthic macrofaunal community ES classified using the BQI_Family index (Dimitriou et al., 2012) was found good in the vast majority of the sampling stations, with ES indications for benthos changing to unacceptable conditions again, only after EI values of the overlying water crossed to poor indication (Moraitis et al., 2015). Eutrophication affected the benthic community by favoring the settlement of certain species: The abundance of the bivalve Corbula gibba, a tolerant to disturbance deposit feeder with high bioturbation potential, increased in all eutrophic areas. Another bivalve, Flexopecten hyalinus, a sensitive species endemic to the Mediterranean Sea, was found only in oligotrophic conditions related mainly to other environmental variables like increased salinity (Moraitis et al., 2018) and temperature (Moraitis et al., 2019), indicating the close relation between eutrophication and climate change. To further support this point, Moraitis and Karakassis (2020) suggested that when studying the structure of macrofaunal communities under a eutrophication gradient, the replacement component was more important than the richness component, indicating that species substitution (i.e., replacement) rather than richness difference is the main driver defining the macrobenthic community structure along the gradient, mirroring the pelagic environmental conditions at each region.
Contrary to macrobenthos, P. oceanica meadows, a highly sensitive and protected ecosystem in the Mediterranean, was affected by eutrophication (Apostolaki et al., 2018). In more detail, nutrient availability and eutrophication conditions in the water column was found to be closely related to the sulfide intrusion in the shoot of P. oceanica and other seagrasses. However, under the pressure of moderate eutrophication, there is detectable sulfide intrusion, in the shoots of the plant, despite the fact that the structure of the plant did not seem to be affected. Seagrasses can minimize sulfide intrusion, via reoxidation of sulfide in the rhizosphere or in the plant aerenchyma, or tolerate sulfides, via incorporation of sulfur in the plant tissue (Hasler-Sheetal and Holmer, 2015). Notably, in those stations, the BBL was well oxygenated, as well as the upper layer of the sediment, indicating high levels of sulfide reoxidation. Similarly to macrofauna, the plants can tolerate medium eutrophication; however, they were under pressure, making them vulnerable to degradation.
Mesocosms
Over the experimental duration, water temperature ranged from 24 to 19°C and light illuminance ranged from 1,000 lx to 4,000 lx at 1-m depth and from 2,500 to 15,000 lx at 3.5-m depth measured at noon (Dimitriou et al., 2017b). After the initial addition, both nutrient concentrations were several times higher than the usual coastal concentrations. In both treatments, nutrients were quickly consumed by autotrophic plankton causing several blooms and Chl-a maxima (approximately 8 Mg L−1, in both treatments). In the “low” treatment, nutrients were depleted after day 18. However, in the “high” treatment, concentrations of both nutrients did not fall below 0.5 MM until the end of the experiment (60 days). Chl-a and POC sedimentation rates were similar for both enriched treatments and maximized on day 24. However, after day 24, the rate was constantly higher in the high treatment, reaching a second maximum on day 24. DO concentration in the BBL at 4-m depth showed no water hypoxia during the whole experiment; DO was positively correlated to Chl-a. The EI showed constantly bad ES in the high treatment, whereas the low treatment started in bad ES, switched to poor (after day 21), and then to moderate (after day 30; (Dimitriou et al., 2017b).
Autotrophic nanoflagellates dominated the beginning of the first bloom, during the peak of nutrients (days 2–3), in both treatments. Later, during the second bloom (days 12–18), all autotrophic groups were present but pico- and nano-autotrophs dominated the community (Santi et al., 2019). Towards the end of the second bloom, the treatments clearly differentiated with micro-autotrophs (diatoms and dinoflagellates) increasing in the “low” treatment and nano-autotrophs prevailing in the Chl-a in the “high” treatment. A grazing effect on autotrophic nanoflagellates during the second bloom was hinted, and there was an important decrease of Synechococcus towards the end of the experiment (after day 21) in the “high” treatment (Santi et al., 2019).
In both enriched treatments, there was a transition from heterotrophic bacteria species of less-dynamic lifestyles, common in oligotrophic waters, like Pelagibacter (Teeling et al., 2016), to fast-growing species of the Bacteroidetes phylum, which are favored by phytoplankton-derived organic matter (Teeling et al., 2012). In addition, a Bacillariophyta-dominated community shifted to larger Dinoflagellata species and Haptophytes in both treatments. The high-nutrient treatment sustained for a prolonged time bacterial populations characterized by rapid metabolic rates, fast-growth capacities, and efficient nutrient uptake, such as Rhodobacteraceae and Flavobacteriaceae. The presence of Actinobacteria was also distinctive to the “high” treatment, possibly due to their ability to effectively escape grazing (Santi et al., 2019).
Sediment OM started to respond to the increased precipitation of OM after a time lag of 12 and 18 days in low and high treatments, respectively, reaching a maximum on day 24, while redox potential (Eh) started to decrease after a time lag of 30 days with sulfide production recorded 5 cm deep within the sediment. The pattern was similar in both enriched treatments; however, the effect was stronger in the high mesocosms where the sediment became temporarily hypoxic after 50 experimental days and recovered on day 60 (Dimitriou et al., 2017a).
Sediment heterotrophic bacteria quickly increased in abundance at the beginning of the experiment, responding to the initial high availability of OM, in both treatments (Tsikopoulou et al., 2020). However, in the high treatment, bacterial abundance decreased after the maximum value recorded, as a result of predation by bacteria eating benthic meiofaunal species. Benthic meiofauna abundance and diversity steadily increased over the experimental duration, with the high addition showing higher values (Dimitriou et al., 2017b). Specific polychaete macrofaunal species (species Paradoneis lyra, Chaetozone setosa, Leiochone leiopygos, Monticellina dorsobranchialis, and Notomastus latericeus) responded to the increased sedimentation rate in the high treatment forming a community significantly different for the low treatment in terms of abundance species composition and functional traits. Those species were characterized by fast reproduction cycles, small body sizes, sediment eating traits, and high bioturbation capabilities, as quantified by Queirós et al. (2013) using mobility and sediment reworking capability. By the end of the 60-day experiment, the macrofaunal community of the high-nutrient addition treatment had a bioturbation potential (measured by the bioturbation index; Queirós et al., 2013) three times higher than the control or the low treatment. The higher bioturbation potential of the high treatment not only contributed to the oxygenation of the sediment, keeping the Eh in oxic conditions, but also resulted in continuous resuspension of inorganic nutrients from the sediment to the water column (Dimitriou et al., 2017c). The resuspended nutrients continued to feed the water phytoplankton, resulting in several blooms. The microphytobenthic community in all mesocosms contained algal groups with high resistance to nutrient addition. In the high-nutrient addition treatment, microphytobenthic community also contributed in keeping the oxic conditions, as a significant increase in total abundance and photosynthetic activity was recorded consuming nutrients and producing O2 (Tsikopoulou et al., 2020).
Discussion
The primary objective of the HYPOXIA project, to detect hypoxic conditions due to eutrophication in the oligotrophic eastern Mediterranean, was not met. No hypoxia was detected in the field, nor was it possible to induce hypoxic conditions under the experimental manipulation, no matter how unusually high was the addition of nutrients. Usual eutrophic conditions induced Chl-a concentrations of 2–4 Mg L−1; the maximum values recorded in all cases did not exceed 8 Mg L−1, and have never exceeded the theoretical value of 10 Mg L−1, which could possibly induce hypoxia. The high water clarity found (common in Mediterranean waters) enabled high DO production from photosynthesis in both the water column and over the benthos, resulting in a positive correlation between DO and Chl-a. On the other hand, the project succeeded in providing critical values in nutrient concentration, which may result from observable changes in sediment geochemical variables to regime shifts in macrofaunal community structure. Such values could aid in designing interventions for reducing nutrients specific at the local level in east Mediterranean as highlighted by Duarte and Krause-Jensen (2018), since they can serve at measurable targets.
In phytoplankton, nutrient uptake is regulated by factors such as cell size and shape, nutrient uptake affinity, and rate of transport mechanisms of the phytoplankton community, especially when nutrients are in excess (Lin et al., 2016). All plankton samples analyzed from both the field and mesocosm studies showed high abundance of nano-, rather than micro-autotrophs; nano-autotrophs were the first to take advantage of the nutrient addition (Santi et al., 2019), while larger cell organisms such as diatoms were not found in the field or were observed much later in the succession in the mesocosm experiments. Small plankton species are known to be quickly grazed in the water column by heterotrophic nanoflagellates and micro-zooplankton in eutrophic gulfs or in aquaculture sites (Tsagaraki et al., 2013), therefore reducing sedimentation of OM to the sediment. Furthermore, the settled OM is highly bioavailable and may be quickly consumed not only by benthic bacteria (a fact that could increase oxygen demand) but also by meiofaunal and macrofaunal species, as concluded from the mesocosm experiments (Dimitriou et al., 2017b; Dimitriou et al., 2017c).
In the end, benthic macrofauna plays a very important role in regulating the benthic–pelagic coupling link through bioturbation. Settled OM and inorganic nutrients therein are constantly remineralized and released from the sediment to the water column providing up to 80% of the needed phytoplankton nutrient requirements (Zhang et al., 2021). This was clearly observed in the mesocosm experiment where nutrients were never entirely depleted from the water column, as they were constantly resuspended from the high bioturbation potential characterizing the benthic community. As a result, the water column was in a constant high productivity–high grazing–high OM sedimentation situation, further feeding benthic meiofauna and macrofauna, which are known to rely on OM sedimentation (Herman et al., 1999; Campanyà-Llovet et al., 2017).
Through bioturbation, the available OM is distributed within the sediment, along with oxygen contributing to macrofaunal subsistence and growth (Zhang et al., 2021). In the oligotrophic eastern Mediterranean, the surplus OM was quickly consumed in both the water column and sediment and transferred up the food web.
As the word “coupling” suggests, this link works both ways. While, as previously mentioned, the settling OM and nutrients are distributed, resuspended, and consumed by benthic fauna, the settling OM affects the benthic species composition by favoring specific biological characteristics and life strategies. Deposit feeding species, tolerant or indifferent to disturbance, are favored according to the AMBI grouping (Borja et al., 2000), forming communities characterized as either moderate, poor, or bad ES (Moraitis and Karakassis, 2020). Only when the settling OM exceeds the assimilative capacity of the benthic community (e.g., prolonged nutrient supply and maintenance of eutrophication conditions in the water column), as recorded in some stations in Dimitriou et al. (2015) and Pavlidou et al. (2019), will the Eh in the sediment then drop, favoring opportunistic species and eventually a shift towards bad ES. However, even then, no water hypoxia was recorded, preventing the total collapse of the system and dramatic regime shifts. This was recorded in the study of Dimitriou et al. (2015), who included a time series of 6 stations in the Thermaikos gulf, which were sampled before and after the start of operation of a sewage treatment plant; over a period of 5 years, ES changed from bad to moderate in the water column and from poor to good in the benthos. However, the benthic–pelagic coupling can be affected by the spatial scale of the study, the environmental characteristics, and the diversity partition that is being examined as several studies in the Eastern Mediterranean conclude (Moraitis et al., 2019; Moraitis and Karakassis, 2020).
All taxa studied throughout the project contributed in maintaining ecosystem health and good environmental status. Tett et al. (2013) suggested that ecosystem health is the result of a system’s resilience, which depends on organization and vigor, in other words, the system’s ability to maintain its structure (organization) and function (vigor) over time, in the face of external stress (resilience). The studied oligotrophic ecosystems had both these elements and, more specifically, (a) the organization in terms of co-adapted species and alternative trophic pathways (i.e., smaller plankton species, meiofauna-eating bacteria, deposit-feeding macrofauna, and species with high bioturbation abilities) and (b) the vigor (i.e., high primary production capability, small plankton with fast metabolism, and constant DO flux due to bioturbation), therefore forming a system with high resilience to eutrophication (external, possible anthropogenic pressure) (Figure 2).
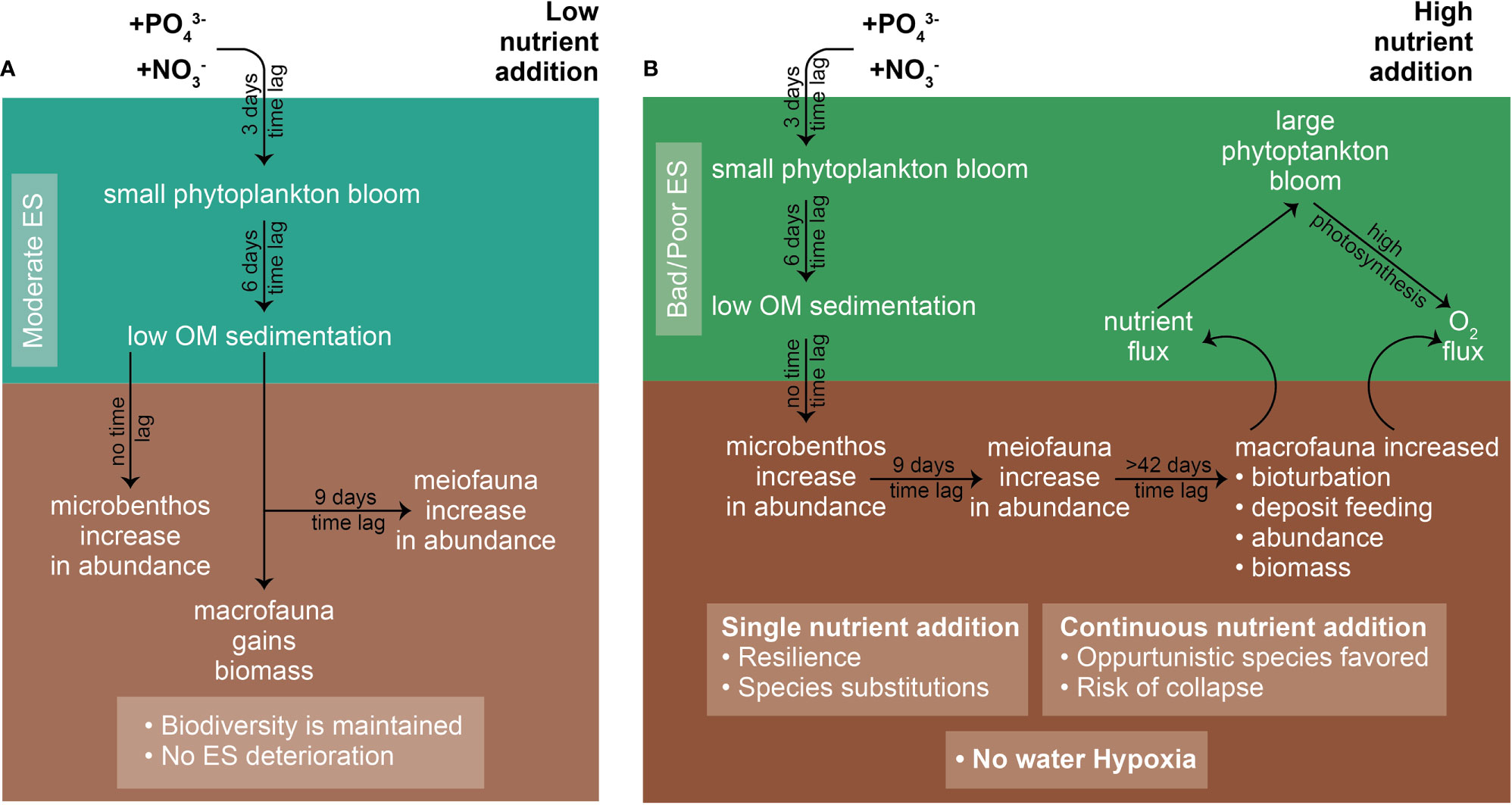
Figure 2 Schematic representation of the benthic–pelagic succession pattern under two different scenarios. (A) Low nutrient addition. (B) High nutrient addition (single or continuous).
As long as the threshold of poor to moderate ES in the water column is not crossed for a prolonged period of time (i.e., year round and not just during a spring or autumn bloom), the ES of the benthic community remains good. However, under constant nutrient supply and eutrophication pressure, and after a considerable time lag of at least 2 months after the initial nutrient addition, it is possible to overcome resilience and lead to a system collapse, suddenly and abruptly. This abrupt change after crossing the system’s resilience threshold was initially suggested by Elliott and Quintino (2007) and Tett et al. (2007) and called the “cliff metaphor”, which was later modified by Tett et al. (2013) and found to be in agreement with the data presented here. It should be noted that two key components of the oligotrophic ecosystems play a significant role in preventing benthic hypoxia and the resulting dead zones: the inability of the plankton system in the water column to reach Chl-a concentrations higher than 10 Mg L−1 and the high DO flux due to bioturbation, which keeps the BBL well oxygenated.
Author Contributions
PD: conceptualization, investigation, writing – original draft, visualization. IS, MM, IT, VP: investigation and writing – review and editing. IK: resources, methodology, writing – review and editing
Conflict of Interest
The authors declare that the research was conducted in the absence of any commercial or financial relationships that could be construed as a potential conflict of interest.
Publisher’s Note
All claims expressed in this article are solely those of the authors and do not necessarily represent those of their affiliated organizations, or those of the publisher, the editors and the reviewers. Any product that may be evaluated in this article, or claim that may be made by its manufacturer, is not guaranteed or endorsed by the publisher.
Funding
Thanks are due to the numerous colleagues involved in the field sampling campaign, the mesocosm experiment and the laboratory analyses. Helpful comments by two reviewers are gratefully acknowledged. The “HYPOXIA: Benthic-pelagic coupling and regime shifts” project (2014-2016), was funded by the Greek General Secretariat for Research and Technology (GSRT) in the framework of the Operational Program “Education and Lifelong Learning” of the National Strategic Reference Framework (NSRF) – ARISTEIA II (HYPOXIA project, No 5381).
References
Andersen J. H., Carstensen J., Holmer M., Krause-Jensen D., Richardson K. (2019). Editorial: Research and Management of Eutrophication in Coastal Ecosystems. Front. Mar. Sci. 6. doi: 10.3389/fmars.2019.00768
Apostolaki E. T., Holmer M., Santinelli V., Karakassis I. (2018). Species-Specific Response to Sulfide Intrusion in Native and Exotic Mediterranean Seagrasses Under Stress. Mar. Environ. Res. 134, 85–95. doi: 10.1016/j.marenvres.2017.12.006
Apostolaki E. T., Tsagaraki T., Tsapakis M., Karakassis I. (2007). Fish Farming Impact on Sediments and Macrofauna Associated With Seagrass Meadows in the Mediterranean. Estuar. Coast. Shelf. Sci. 75 (3), 408–416. doi: 10.1016/j.ecss.2007.05.024
Aylagas E., Borja MÁ., Tangherlini M., Dell'Anno A., Corinaldesi C., Michell C. T., et al. (2017). A Bacterial Community-Based Index to Assess the Ecological Status of Estuarine and Coastal Environments. Mar. Pollut. Bull. 114 (2), 679–688. doi: 10.1016/j.marpolbul.2016.10.050
Boesch D. F. (2019). Barriers and Bridges in Abating Coastal Eutrophication. Front. in Mar. Sci. 6. doi: 10.3389/fmars.2019.00123
Borja A., Franco J., Perez V. (2000). A Marine Biotic Index to Establish the Ecological Quality of Soft-Bottom Benthos Within European Estuarine and Coastal Environments. Mar. Pollut Bull. 40 (12), 1100–1114. doi: 10.1016/S0025-326X(00)00061-8
Bracken M. E. S., Hillebrand H., Borer E. T., Seabloom E. W., Cebrian J., Cleland E. E., et al. (2015). Signatures of Nutrient Limitation and Co-Limitation: Responses of Autotroph Internal Nutrient Concentrations to Nitrogen and Phosphorus Additions. Oikos 124 (2), 113–121. doi: 10.1111/oik.01215
Brady D. C., Testa J. M., Di Toro D. M., Boynton W. R., Kemp W. M. (2013). Sediment Flux Modeling: Calibration and Application for Coastal Systems. Estuar. Coast. Shelf. Sci. 117, 107–124. doi: 10.1016/j.ecss.2012.11.003
Campanyà-Llovet N., Snelgrove P. V. R., Parrish C. C. (2017). Rethinking the Importance of Food Quality in Marine Benthic Food Webs. Prog. Oceanog. 156, 240–251. doi: 10.1016/j.pocean.2017.07.006
Chatzinikolaou E., Mandalakis M., Damianidis P., Dailianis T., Gambineri S., Rossano C., et al. (2018). Spatio-Temporal Benthic Biodiversity Patterns and Pollution Pressure in Three Mediterranean Touristic Ports. Sci. Tot. Environ. 624, 648–660. doi: 10.1016/j.scitotenv.2017.12.111
CSTT (1997). “Comprehensive Studies for the Purposes of Article 6 of Directive 91/271 EEC). The Urban Waste Water Treatment Directive,” in Edinburgh: Scottish Environment Protection Agency (East Region). (Edinburgh: Scottish Environment Protection Agency (East Region)).
Diaz R. J. (2001). Overview of Hypoxia Around the World. J. Environ. Qual. 30 (2), 275–281. doi: 10.2134/jeq2001.302275x
Diaz R. J., Rosenberg R. (2008). Spreading Dead Zones and Consequences for Marine Ecosystems. science 321 (5891), 926–929. doi: 10.1126/science.1156401
Dimitriou P. D., Apostolaki E. T., Papageorgiou N., Reizopoulou S., Simboura N., Arvanitidis C., et al. (2012). Meta-Analysis of a Large Data Set With Water Framework Directive Indicators and Calibration of a Benthic Quality Index at the Family Level. Ecol. Indic. 20 (0), 101–107. doi: 10.1016/j.ecolind.2012.02.008
Dimitriou P. D., Papageorgiou N., Arvanitidis C., Assimakopoulou G., Pagou K., Papadopoulou K. N., et al. (2015). One Step Forward: Benthic Pelagic Coupling and Indicators for Environmental Status. PLoS One 10 (10), e0141071. doi: 10.1371/journal.pone.0141071
Dimitriou P. D., Papageorgiou N., Geropoulos A., Kalogeropoulou V., Moraitis M., Santi I., et al. (2017a). Benthic Pelagic Coupling in a Mesocosm Experiment: Delayed Sediment Responses and Regime Shifts. Sci. Tot. Environ. 605–606. doi: 10.1016/j.scitotenv.2017.06.239
Dimitriou P. D., Papageorgiou N., Geropoulos A., Kalogeropoulou V., Moraitis M., Santi I., et al. (2017b). A Novel Mesocosm Setup for Benthic-Pelagic Coupling Experiments. Limnol. Oceanog.: Methods 15 (4), 349–362. doi: 10.1002/lom3.10163
Dimitriou P. D., Papageorgiou N., Karakassis I. (2017c). Response of Benthic Macrofauna to Eutrophication in a Mesocosm Experiment: Ecosystem Resilience Prevents Hypoxic Conditions. Front. Mar. Sci. 4, 391. doi: 10.3389/fmars.2017.00391
Duarte C. M. (2009). Coastal Eutrophication Research: A New Awareness. Hydrobiologia 629 (1), 263–269. doi: 10.1007/s10750-009-9795-8
Duarte C. M., Conley D. J., Carstensen J., Sánchez-Camacho M. (2009). Return to Neverland: Shifting Baselines Affect Eutrophication Restoration Targets. Estuar. Coast. 32 (1), 29–36. doi: 10.1007/s12237-008-9111-2
Duarte C. M., Krause-Jensen D. (2018). Intervention Options to Accelerate Ecosystem Recovery From Coastal Eutrophication. Front. Mar. Sci. 5. doi: 10.3389/fmars.2018.00470
Elliott M., Quintino V. (2007). The Estuarine Quality Paradox, Environmental Homeostasis and the Difficulty of Detecting Anthropogenic Stress in Naturally Stressed Areas. Mar. Pollut. Bull. 54 (6), 640–645. doi: 10.1016/j.marpolbul.2007.02.003
Graf G. (1992). Benthic-Pelagic Coupling: A Benthic View. Oceanog. Mar. Biol.: Annu. Rev. 30 (B), 149–190.
Hasler-Sheetal H., Holmer M. (2015). Sulfide Intrusion and Detoxification in the Seagrass Zostera Marina. PLoS One 10 (6), e0129136. doi: 10.1371/journal.pone.0129136
Herman P. M. J., Middelburg J. J., Van De Koppel J., Heip C. H. R. (1999). “Ecology of Estuarine Macrobenthos,” in Advances in Ecological Research. Eds. Nedwell D. B., Raffaelli D. G. (Academic Press) 29, 195–240. doi: 10.1016/S0065-2504(08)60194-4
Hyland J., Balthis L., Karakassis I., Magni P., Petrov A., Shine J., et al. (2005). Organic Carbon Content of Sediments as an Indicator of Stress in the Marine Benthos. Mar. Ecol. Prog. Ser. 295, 91–103. doi: 10.3354/meps295091
Lin S., Litaker R. W., Sunda W. G. (2016). Phosphorus Physiological Ecology and Molecular Mechanisms in Marine Phytoplankton. J. Phycol. 52 (1), 10–36. doi: 10.1111/jpy.12365
Moraitis M. L., Dimitriou P. D., Geropoulos A., Tsikopoulou I., Karakassis I. (2015). “Monitoring and evaluating the ecological status of coastal bays in the Aegean Sea: matching water column and benthic indicators”, in: DEVOTES-EUROMARINE. Integrative Assessment of Marine Systems: The Ecosystem Approach in Practice). San Sebastian, Spain.
Moraitis M. L., Karakassis I. (2020). Assessing Large-Scale Macrobenthic Community Shifts in the Aegean Sea Using Novel Beta Diversity Modelling Methods. Ramifications on Environmental Assessment. Sci. Tot. Environ. 734, 139504. doi: 10.1016/j.scitotenv.2020.139504
Moraitis M. L., Tsikopoulou I., Geropoulos A., Dimitriou P. D., Papageorgiou N., Giannoulaki M., et al. (2018). Molluscan Indicator Species and Their Potential Use in Ecological Status Assessment Using Species Distribution Modeling. Mar. Environ. Res. 140,10–17. doi: 10.1016/j.marenvres.2018.05.020
Moraitis M. L., Valavanis V. D., Karakassis I. (2019). Modelling the Effects of Climate Change on the Distribution of Benthic Indicator Species in the Eastern Mediterranean Sea. Sci. Tot. Environ. 667, 16–24. doi: 10.1016/j.scitotenv.2019.02.338
Papageorgiou N., Kalantzi I., Karakassis I. (2010). Effects of Fish Farming on the Biological and Geochemical Properties of Muddy and Sandy Sediments in the Mediterranean Sea. Mar. Environ. Res. 69 (5), 326–336. doi: 10.1016/j.marenvres.2009.12.007
Pavlidou A., Simboura N., Pagou MM., Assimakopoulou G., Gerakaris V., Hatzianestis I., et al. (2019). Using a Holistic Ecosystem-Integrated Approach to Assess the Environmental Status of Saronikos Gulf, Eastern Mediterranean. Ecol. Indic. 96, 336–350. doi: 10.1016/j.ecolind.2018.09.007
Pavlidou A., Simboura N., Rousselaki E., Tsapakis M., Pagou K., Drakopoulou P., et al. (2015). Methods of Eutrophication Assessment in the Context of the Water Framework Directive: Examples From the Eastern Mediterranean Coastal Areas. Continent. Shelf. Res. 108, 156–168. doi: 10.1016/j.csr.2015.05.013
Pearson T. H., Rosenberg R. (1978). Macrobenthic Succession in Relation to Organic Enrichment and Pollution of the Marine Environment. Oceanog. Mar. Biol.: Annu. Rev. 16, 229–311.
Primpas I., Tsirtsis G., Karydis M., Kokkoris G. D. (2010). Principal Component Analysis: Development of a Multivariate Index for Assessing Eutrophication According to the European Water Framework Directive. Ecol. Indic. 10 (2), 178–183. doi: 10.1016/j.ecolind.2009.04.007
Queirós A. M., Birchenough S. N. R., Bremner J., Godbold J. A., Parker R. E., Romero-Ramirez A., et al. (2013). A Bioturbation Classification of European Marine Infaunal Invertebrates. Ecol. Evol. 3 (11), 3958–3985. doi: 10.1002/ece3.769
Santi I. (2019). Eukaryotic Microbial Plankton and Nutrient Supply in the Eastern Mediterranean Sea (PhD, University of Crete).
Santi I., Kasapidis P., Karakassis I., Pitta P. (2021). A Comparison of DNA Metabarcoding and Microscopy Methodologies for the Study of Aquatic Microbial Eukaryotes. Diversity 13 (5), 180. doi: 10.3390/d13050180
Santi I., Tsiola A., Dimitriou P. D., Fodelianakis S., Kasapidis P., Papageorgiou N., et al. (2019). Prokaryotic and Eukaryotic Microbial Community Responses to N and P Nutrient Addition in Oligotrophic Mediterranean Coastal Waters: Novel Insights From DNA Metabarcoding and Network Analysis. Mar. Environ. Res. 150, 104752. doi: 10.1016/j.marenvres.2019.104752
Schratzberger M., Ingels J. (2018). Meiofauna Matters: The Roles of Meiofauna in Benthic Ecosystems. J. Exp. Mar. Biol. Ecol. 502, 12–25. doi: 10.1016/j.jembe.2017.01.007
Simboura N., Argyrou M. (2010). An Insight Into the Performance of Benthic Classification Indices Tested in Eastern Mediterranean Coastal Waters. Mar. Pollut. Bull. 60 (5), 701–709. doi: 10.1016/j.marpolbul.2009.12.005
Simboura N., Tsapakis M., Pavlidou A., Assimakopoulou G., Pagou K., Kontoyiannis H., et al. (2015). Assessment of the Environmental Status in the Hellenic Coastal Waters (Eastern Mediterranean): From the Water Framework Directive to the Marine Strategy Framework Directive. Mediterranean. Mar. Sci. 16 (1), 46–64. doi: 10.12681/mms.960
Simboura N., Zenetos A., Pancucci-Papadopoulou M. A., Reizopoulou S., Streftaris N. (2012). Indicators for the Sea-Floor Integrity of the Hellenic Seas Under the European Marine Strategy Framework Directive: Establishing the Thresholds and Standards for Good Environmental Status. Med. Mar. Sci. 13 (1), 140–152. doi: 10.12681/mms.31
Teeling H., Fuchs B. M., Becher D., Klockow C., Gardebrecht A., Bennke C. M., et al (2012). Substrate-Controlled Succession of Marine Bacterioplankton Populations Induced by a Phytoplankton Bloom. Science 336, 608–611. doi: 10.1126/science.1218344
Teeling H., Fuchs B. M., Becher D., Klockow C., Gardebrecht A., Bennke C. M., et al. (2012). Substrate-Controlled Succession of Marine Bacterioplankton Populations Induced by a Phytoplankton Bloom. Science 336 (6081), 608–611. doi: 10.1126/science.1218344
Tett P., Gowen R., Mills D., Fernandes T., Gilpin L., Huxham M., et al. (2007). Defining and Detecting Undesirable Disturbance in the Context of Marine Eutrophication. Mar. Pollut. Bull. 55 (1), 282–297. doi: 10.1016/j.marpolbul.2006.08.028
Tett P., Gowen R. J., Painting S. J., Elliott M., Forster R., Mills D. K., et al. (2013). Framework for Understanding Marine Ecosystem Health. Mar. Ecol. Prog. Ser. 494, 1–27. doi: 10.3354/meps10539
Tsagaraki T. M., Pitta P., Frangoulis C., Petihakis G., Karakassis I. (2013). Plankton Response to Nutrient Enrichment is Maximized at Intermediate Distances From Fish Farms. Mar. Ecol. Prog. Ser. 493, 31–42. doi: 10.3354/meps10520
Tsikopoulou I., Santi I., Dimitriou P. D., Papageorgiou N., Pitta P., Karakassis I. (2020). Response of Microphytobenthos and Benthic Bacteria Viability to Eutrophication in a Benthic–Pelagic Coupling Mesocosm Experiment. Front. Mar. Sci. 7, 270. doi: 10.3389/fmars.2020.00270
Ubertini M., Lefebvre S., Gangnery A., Grangeré K., Le Gendre R., Orvain F. (2012). Spatial Variability of Benthic-Pelagic Coupling in an Estuary Ecosystem: Consequences for Microphytobenthos Resuspension Phenomenon. PLoS One 7 (8), e44155. doi: 10.1371/journal.pone.0044155
Vaquer-Sunyer R., Duarte C. M. (2008). Thresholds of Hypoxia for Marine Biodiversity. Proc. Natl. Acad. Sci. U. S. A. 105 (40), 15452–15457. doi: 10.1073/pnas.0803833105
Keywords: benthic–pelagic coupling, eutrophication, macrofauna, ecosystem health, ecosystem processes, phytoplankton
Citation: Dimitriou PD, Santi I, Moraitis ML, Tsikopoulou I, Pitta P and Karakassis I (2022) Benthic–Pelagic Coupling in the Oligotrophic Eastern Mediterranean: A Synthesis of the HYPOXIA Project Results. Front. Mar. Sci. 9:886335. doi: 10.3389/fmars.2022.886335
Received: 28 February 2022; Accepted: 20 April 2022;
Published: 19 May 2022.
Edited by:
Tamara Cibic, Istituto Nazionale di Oceanografia e di Geofisica Sperimentale, ItalyReviewed by:
Christian Henri Nozais, Université du Québec à Rimouski, CanadaVivianne Solis-Weiss, National Autonomous University of Mexico, Mexico
Copyright © 2022 Dimitriou, Santi, Moraitis, Tsikopoulou, Pitta and Karakassis. This is an open-access article distributed under the terms of the Creative Commons Attribution License (CC BY). The use, distribution or reproduction in other forums is permitted, provided the original author(s) and the copyright owner(s) are credited and that the original publication in this journal is cited, in accordance with accepted academic practice. No use, distribution or reproduction is permitted which does not comply with these terms.
*Correspondence: Panagiotis D. Dimitriou, cGRpbWl0cmlvdUB1b2MuZ3I=