Effects of varying types and amounts of herbivory and nutrient enrichment on a tropicalizing seagrass meadow
- 1Dauphin Island Sea Lab, Dauphin Island, AL, United States
- 2Department of Marine Ecology, Center of Advanced Studies of Blanes, Blanes, Spain
- 3Institute of Environment, Coastlines and Oceans Division, and Department of Biological Sciences, Florida International University, Miami, FL, United States
- 4School of Marine and Environmental Sciences, University of South Alabama, Mobile, AL, United States
Climate change is impacting marine ecosystem community dynamics on a global scale. While many have assessed direct effects of climate change, indirect effects on marine ecosystems produced by biotic interactions remain poorly understood. For example, warming-induced range expansions and increased consumption rates of herbivores can lead to significant and unexpected changes in seagrass-dominated ecosystems. To better understand the threats tropicalization presents for the functioning of turtlegrass (Thalassia testudinum) meadows, we focused on the extensive turtlegrass beds of St. Joseph Bay, Florida in the northern Gulf of Mexico, a location with increasing numbers of tropically-associated green turtles. Our goals were to investigate experimentally how different grazing rates (natural and simulated),including high levels reflective of green turtle herbivory, coupled with nutrient supply, might alter turtlegrass structure and functioning in a higher latitude, subtropical turtlegrass meadow. We found that 4 months of varying levels of herbivory did not affect turtlegrass productivity, while 7 months of herbivory reduced percent cover, and 10 months reduced shoot density. Nutrient additions had few important effects. Ten months into the study, a massive recruitment of the herbivorous sea urchin (Lytechinus variegatus), whose densities reached 19 urchins/m2 completely overgrazed our study area and a large portion of the lush turtlegrass meadows of St. Joseph Bay. While local turtlegrass overgrazing had been previously noted at these urchin densities, a total loss of seagrass in such a large area has rarely ever been recorded. Overgrazing of the kind we observed, likely a result of both urchin and increasing green turtle grazing, can result in the loss of many key ecosystem services. As tropicalization continues, understanding how changes in biotic interactions, such as increased herbivory, affect higher latitude seagrass meadows will be necessary for their proper management and conservation.
Introduction
Climate change is altering the ecological functioning of a wide range of terrestrial and marine communities (Estes et al., 2011; Vergés et al., 2014a; Vergés et al., 2016; Wernberg et al., 2016). And it is well documented that climate-induced shifts in the relative abundances and geographic ranges of species can create transient and novel communities, with potentially unanticipated and dramatic effects on ecosystem structure (Blois et al., 2013; Vergés et al., 2019; Zarco-Perello et al., 2019). Some of the most instructive examples of such effects come from a growing number of studies of the ‘tropicalization’ of temperate kelp forests, in which warmer temperatures allow range expansions of tropical, mobile herbivores, resulting in greatly increased grazing on these temperate macroalgae that had previously been isolated from intense herbivory (Vergés et al., 2014a; Vergés et al., 2016; Wernberg et al., 2016).
When herbivory exerts strong top-down control on foundational species that in turn leads to large-scale changes in ecosystem properties, the consequences of poleward shifts in the ranges of tropical herbivores may easily outweigh the direct effects of warming on the ecological performance of basal species (Vergés et al., 2014b; Vergés et al., 2016; Wernberg et al., 2016). As is well-known, herbivory is particularly intense in marine environments, with nearly 70% of benthic production being consumed by grazers (Poore et al., 2012). As tropical herbivores expand their ranges with warming temperatures, they can and do impose powerful effects on native communities. The massive disappearance of kelp (Ecklonia spp.) and fucoid (Sargassum spp.) beds in southern Japan represents one of the best-documented cases. In total, several thousand hectares of temperate kelp habitat were initially converted to rocky barrens, and subsequently colonized by reef-building corals, producing a dramatic phase shift (Serisawa et al., 2004; Tanaka et al., 2012). These effects were largely attributed to a combination of direct negative thermal effects on kelp survival, and increased consumption of algal biomass by rabbitfish (Siganus spp.) and parrotfish (Calotomus japonicas). Similar trends have been documented in southeastern and southwestern Australia, where kelp decline and the appearance of algal turfs has followed increases in tropical herbivores and rates of algal grazing (Vergés et al., 2016; Wernberg et al., 2016; Zarco-Perello et al., 2019), as well as in the southern Mediterranean where rabbitfish immigrating from the Red Sea have become increasingly abundant and macroalgae less so (Vergés et al., 2014b).
In the Western Atlantic, seagrass meadows represent a foundational marine ecosystem that is undergoing tropicalization, although predictions of ecological changes in response to shifts in biotic interactions (Heck et al., 2015) have yet to be fully developed and tested. Seagrasses are ubiquitous in coastal waters where they provide key ecosystem services, including high rates of primary production, nutrient cycling, and habitat provisioning (Orth et al., 2006; Waycott et al., 2009) across the tropical and subtropical Western Atlantic. Turtlegrass (Thalassia testudinum) is a climax marine species that spans more than 30° of latitude and provides thousands of km2 of benthic habitat. This species is critical for structuring the benthic seascape and supporting a fisheries industry previously valued at nearly $3,500/ha1 year1 (Costanza et al., 1997). Apart from the conventional stressors that promote seagrass decline, such as poor water quality, meadows near the northern range boundary of turtlegrass in the northern Gulf of Mexico (nGOM) are currently under the looming influence of tropicalization. This area is warming and experiencing fewer freezes than in the past, and many tropically-associated species are moving northward along Gulf coastlines and becoming established (Osland et al., 2021).
Warming has also been linked to increases in tropical herbivores in the nGOM, notably in the parrotfish (Nicholsina usta), which consumes seagrass at nearly five times the rate of native sea urchin and fish grazers (Fodrie et al., 2010; Heck et al., 2015), along with other seagrass megaherbivores, especially juvenile green sea turtles (Chelonia mydas) (Avens et al., 2012; Williams et al., 2014; Rodriguez and Heck, 2020; Rodriguez and Heck, 2021). High green turtle abundances (resulting from successful conservation efforts) and their impressive rates of seagrass consumption have been responsible for large declines of turtlegrass beds in Bermuda and Mexico, as well as seagrass meadows in several Indian Ocean locations (Kelkar et al., 2013b; Kelkar et al., 2013a; Molina Hernández and van Tussenbroek, 2014; Fourqurean et al., 2019; Gangal et al., 2021).
Artificial clipping (Holzer and McGlathery, 2016) and natural grazing (Fourqurean et al., 2010; Molina Hernández and van Tussenbroek, 2014) experiments have documented impacts on turtlegrass structure and function; however, higher latitude seagrass meadows cope with lower irradiance (particularly in the winter) and, because of reduced amounts of solar energy for photosynthesis, might be expected to be less resilient to increased grazing than tropical turtlegrass meadows (Manuel et al., 2013). Reduced solar insolation can play a major role in influencing seagrass productivity, abundance, morphology, and depth distribution (Backman and Barilotti, 1976; Dennison, 1987), including for turtlegrass near the northern extent of its range in the Gulf of Mexico (Herzka and Dunton, 1997; Lee and Dunton, 1997; Major and Dunton, 2002). Herbivory and seasonality have been found to have synergistic effects on turtlegrass. For instance, in St. Joseph Bay, Florida (30°N, 85.5°W), Valentine and Heck (1991) found that sea urchin herbivory increased turtlegrass primary productivity during the summer months, but during fall and winter Lytechinus variegatus overgrazed localized turtlegrass patches. Similarly, in Perdido Bay, in northwest Florida, (Ibarra-Obando et al., 2004) found that while simulated green turtle herbivory itself produced few significant effects, simulated herbivory under low light conditions decreased turtlegrass productivity.
Prior work in Bermuda has demonstrated that nutrient-replete seagrass beds (which display high regrowth rates) may be more resilient to tropicalization-induced grazing via the rapid replacement of lost tissue (Holzer and McGlathery, 2016). Moreover, Christianen et al. (2012) documented strong interactions between turtle grazing and nutrient availability, whereby grazing increased productivity and tolerance to high nutrient loads. While such interactions between grazing and nutrient enrichment have been documented for terrestrial communities (Bakker et al., 2006), they have yet to be broadly tested with marine species, particularly where there is significant consumption of seagrass biomass (Heck and Valentine, 2006).
Here we describe the results of an experiment that was designed to evaluate the separate and interacting effects of 1) grazing (natural and simulated at two intensities – a full clip mimicking severe grazing and a half clip mimicking moderate grazing) and 2) nutrient enrichment on turtlegrass structure and functioning in a subtropical turtlegrass meadow experiencing increasing rates of green turtle herbivory.
Methods
Study site
Located in the northeastern Gulf of Mexico, St. Joseph Bay (30°N, 85.5°W) is a shallow semi-enclosed bay. Salinities range from 30 to 36 (Stewart and Gorsline, 1962), and temperatures from 8° to 30°C (Valentine and Heck, 1993), making this a warm temperate or subtropical bay, and one of few in the Gulf of Mexico with little freshwater input. St. Joseph Bay has a tidal range of approximately 0.47 m, very low current flow, and highly organic sediments (Stewart and Gorsline, 1962). Seagrass meadows cover about 2,900 ha and are comprised of turtlegrass (Thalassia testudinum), shoal grass (Halodule wrightii), and manatee grass (Syringodium filiforme), with T. testudinum being the dominant species (Yarbro and Carlson, 2016). Our experiment took place in the southern part of the bay, which contains several relatively deep channels and extensive, shallow (~ 0.5–1.5 m) lush T. testudinum beds (Figure 1). This location was chosen because the Bay’s primary seagrass herbivores have been known to reside and graze there (Lamont et al., 2015; Rodriguez and Heck, 2020).
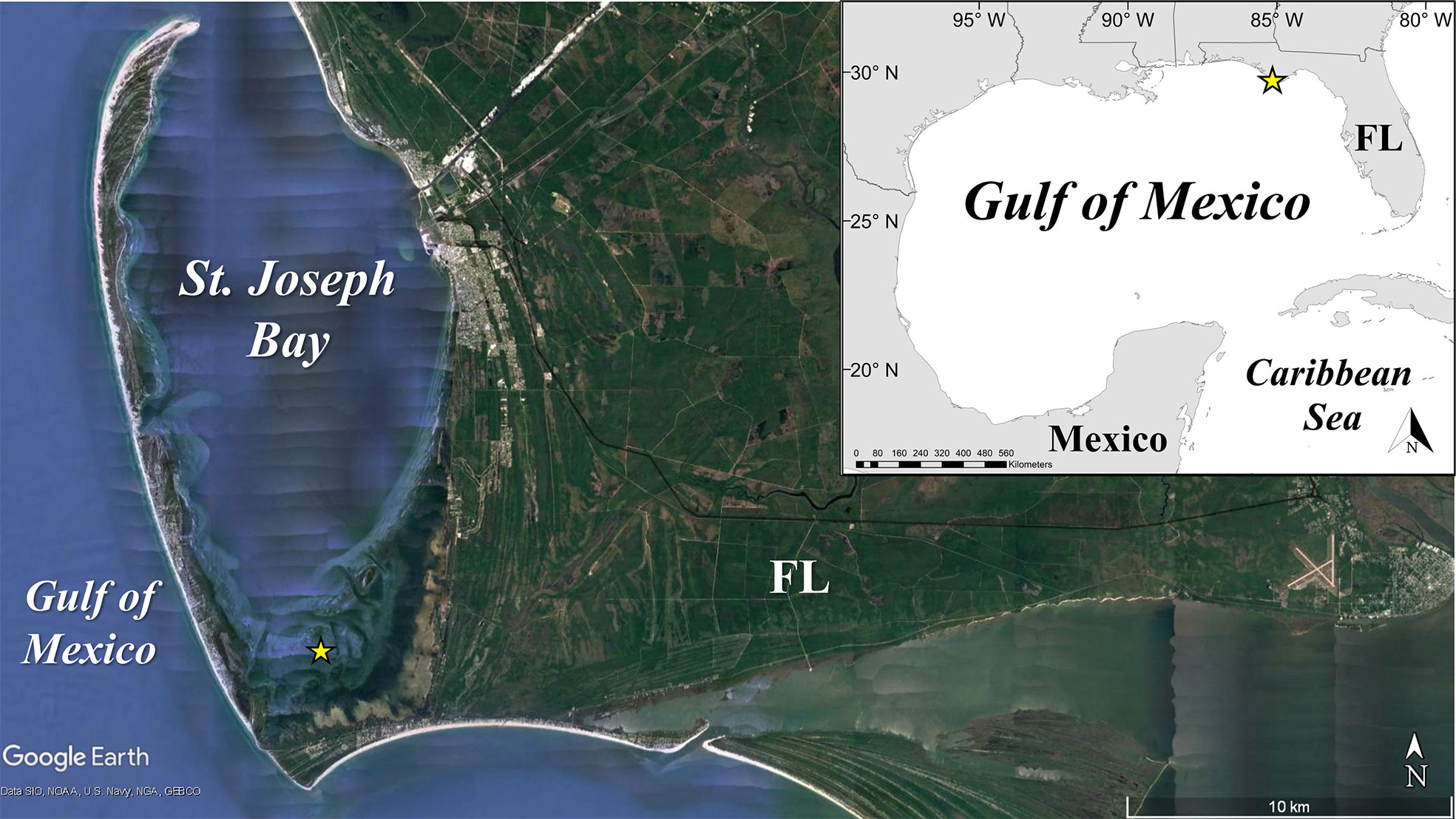
Figure 1 Study area in St. Joseph Bay, Florida in the northeastern Gulf of Mexico. Experimental array (yellow star) is located in the southern turtlegrass beds of St. Joseph Bay.
Herbivores in St. Joseph Bay
The current resident macroherbivores in the study area are L. variegatus and, more recently due to successful conservation efforts and warming temperatures, the green turtle (Chelonia mydas) (Rodriguez and Heck, 2020). Herbivorous fishes currently are relatively rare in St. Joseph Bay. Though pinfish (Lagodon rhomboides) are present, only 20 cm size classes and above are herbivorous, which only constitute 3% of the pinfish population in St. Joseph Bay (Prado and Heck, 2011). While emerald parrotfish, Nicholsina usta, primarily feed on seagrasses (Prado and Heck, 2011), and occur north and south of the Bay, they do not currently exist in St Joseph Bay (Fodrie et al., 2010). While the West Indian manatee (Trichechus manatus) has been sighted in St. Joseph Bay, there is yet no record of manatee herbivory. However, as waters continue to warm it is quite likely that tropicalization of the northern Gulf of Mexico will allow additional tropical herbivores, including parrotfish such as N. usta and the West Indian manatee, to expand their ranges and increase grazing intensity in the Bay.
Green turtles are present in St. Joseph Bay year-round (McMichael, 2005; Lamont and Iverson, 2018). Neritic juvenile green turtles are the life stage primarily found in St. Joseph Bay. Though loggerhead and Kemp’s ridley turtles are present, Foley et al. (2007) found that green turtles comprised > 96% of the turtles collected in St. Joseph Bay during a 2001 cold stunning event, which is the first published sighting of green turtles in St. Joseph Bay, though they likely existed here historically. Avens et al. (2012) saw similar proportions of green turtles during a 2010 cold stunning event (> 95%), and the most recent cold stunning in 2018 continued this trend, with > 95% of the ~1,250 turtles stunned being juvenile green turtles (M. Lamont, USGS, personal communication). After examining gastrointestinal (GI) tracts of cold-stunned turtles, Foley et al. (2007) confirmed that turtlegrass was a major diet component of the juvenile green turtles in St. Joseph Bay, and T. testudinum was present in the GI tract of all examined turtles. Williams et al. (2014) also found turtlegrass to be the major diet item in the juvenile C. mydas in St. Joseph Bay.
Lytechinus variegatus feed on green and decayed seagrass (Marco-Méndez et al., 2012), and their consumption rates vary seasonally (Rodriguez and Heck, 2021). As was observed in this and previous studies, these urchins can reach densities over 100/m2 as they forage in fronts (Camp et al., 1973; Rose et al., 1999; Peterson et al., 2002). L. variegatus and C. mydas exhibit different feeding strategies. L. variegatus occurs in constantly moving aggregations and feeds on aboveground seagrass biomass and detritus, taking small bites from leaf edges, and sometimes completely removing aboveground biomass (Valentine and Heck, 1991). C. mydas can exhibit different foraging strategies, but they generally completely crop aboveground biomass starting at the base of the shoot (Bjorndal, 1980). In St. Joseph Bay, at least some green turtles have been found to regraze plots ranging in size from < 0.1 to 10 m2, thereby maintaining a mosaic of grazed and ungrazed plots and moving between them, especially wherever structure is available (Rodriguez and Heck, 2020).
Field surveys
Invertebrate community surveys were performed by securing a 50 m transect tape with PVC poles and deploying a 1 m2 quadrat every 5 m along the transect tape. The transect tape was adjacent to the location of the caging experiment (see below) and the seagrass and sediment were carefully checked for cryptic organisms. Invertebrates visible to the naked eye were identified in the field to the lowest taxonomic level possible and abundances along quadrats were averaged by sampling date.
Thalassia testudinum tethering experiments were used to measure turtlegrass consumption rates and we used two sets of six replicate ropes (cf. Kirsch et al., 2002). The two deployments were approximately 30 m apart. Each replicate rope was attached at 0.25 m intervals to two wire stakes and had six clothespins, each of which secured a turtlegrass shoot. Photos of roughly similar-sized shoots were taken before and after deployment on a white surface with a ruler in the picture for scale. Before and after deployment images were analyzed using ImageJ to estimate changes in leaf area due to consumption by herbivores. Invertebrate surveys and tethering experiments were carried out at approximately monthly intervals for 1 year. The experiment ranged from April 2018 to May 2019, though only results through March 2019 will be presented due to the confounding effects of a massive urchin recruitment event that occurred at that time.
Caging experiment design
Experimental plots (0.25 m2) were established in a 5 x 10 grid layout with a minimum separation of 2 m. Each plot was bounded by four vertical PVC stakes driven into the sediment at the corners, along with one vertical PVC stake in the center for the attachment of slow-release fertilizer. Plots were randomly assigned to two broad categories, (1) those examining natural grazing x fertilization and (2) those examining simulated grazing x fertilization (Figure 2). Category 1 (natural grazing) was created by assigning plots to a variety of caging treatments that used aquaculture grade plastic mesh with 1 cm openings: full cages (no herbivore access which was prevented by mesh cages), partial cages (herbivore access possible with 1 side open but the top in place to control for shading), and uncaged plots (full herbivore access). These treatments were crossed with 2 levels of fertilization (unenriched and enriched) for a total of 6 treatments, each replicated five times (n=5).
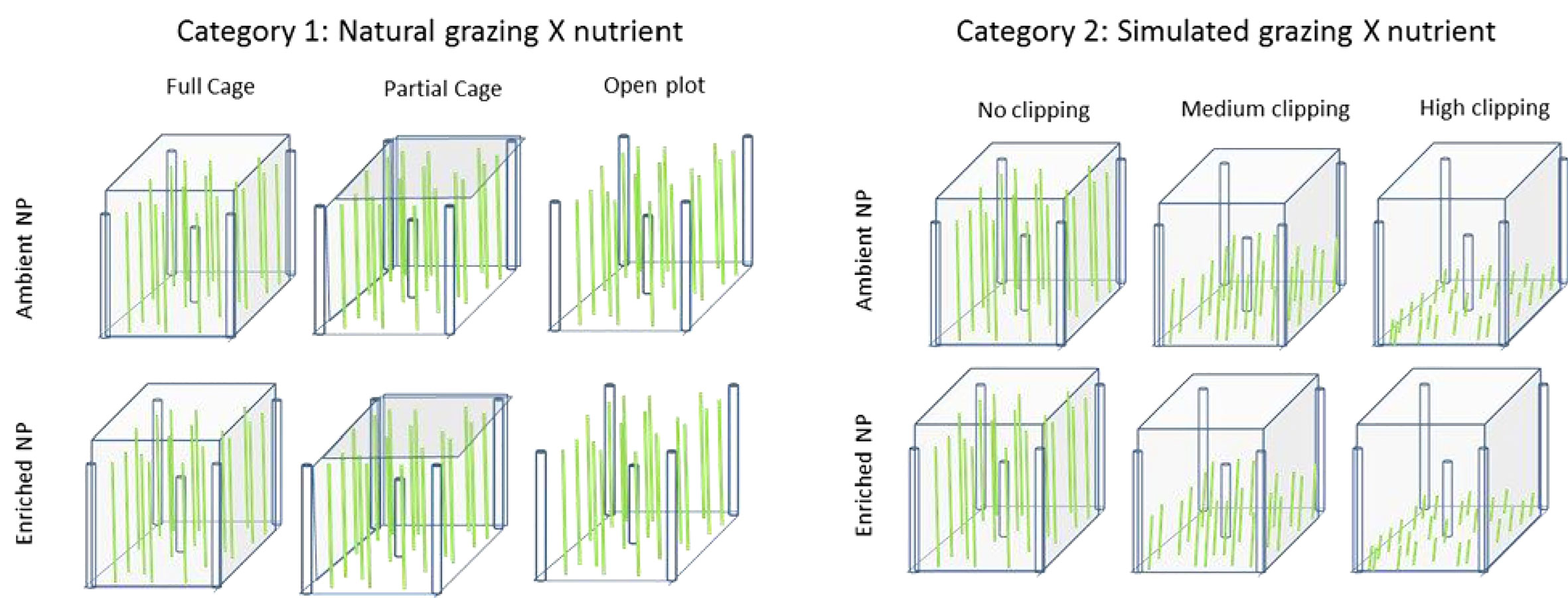
Figure 2 Graphical representation of treatments (n=5) established at our study site. Treatments included two broad categories: natural grazing (full cages, partial cages and uncaged plots) x fertilization; and simulated grazing (no clip, half clip and full clip) x fertilization.
Category 2 (simulated grazing) was created by completely enclosing plots in cages, within which artificial clipping and fertilization was imposed in a 2-way factorial design (3 clipping levels and 2 fertilization levels, n=5 for each treatment). Therefore, the site contained 10 treatments with 50 individual plots (the ‘full cage’ treatments of Category 1 and ‘no clipping’ treatments of Category 2 are the same and have been combined). All plots were initially trenched around the borders using dive knives to limit carbohydrate translocation into the plots, and cages were scrubbed with soft brushes to remove fouling at two-week intervals.
The effects of increased grazing due to tropicalization was simulated by artificially clipping turtlegrass shoots within full mesh exclosures, a technique previously demonstrated to effectively replicate macrograzer activity (Moran and Bjorndal, 2005; Christianen et al., 2012; Holzer and McGlathery, 2016). Three levels of clipping were implemented and maintained for the duration of the experiment: no clipping, half clipping, and full clipping. Half clipping was based upon empirical estimates of future seagrass consumption by smaller herbivores (Emerald parrotfish Nicholsina usta, Pinfish Lagodon rhomboides, and Planehead filefish Stephanolepis hispidus) within the northern Gulf of Mexico (Heck et al., 2015). For this level, at two-week intervals, an estimated 30% of the height of the turtlegrass canopy, as measured by a handheld ruler, was trimmed with stainless steel shears.
Full clipping replicated more intense grazing by larger megaherbivores (green turtles) and was based upon prior estimates of turtle grazing (Bjorndal, 1980). For this treatment, at two-week intervals, turtlegrass was clipped just above the leaf/sheath junction, or ~1–2 cm above the sediment surface (Moran and Bjorndal, 2005). In addition, a 10 cm trimmed border around the clipped plots was maintained to limit shading (Holzer and McGlathery, 2016). Clipped seagrass was removed from the plots.
Cage treatments will be referred to as: Control (open uncaged plots), Partial cages (cages with 1 side open), Full cage No clip (full caging treatment with no simulated herbivory), Full cage Half clip (full caging treatment with 30% of canopy clipped every 2 weeks), Full cage Full clip (full caging treatment with simulated clipping down to 1-2 cm above the sediment surface). Potential shading impacts were investigated by measuring photosynthetically active radiation (PAR) (Odyssey Integrating Light Sensor, New Zealand) within a Full cage as well as outside of the experimental array. PAR measurements were logged every 6 minutes.
Pilot testing of our proposed fertilization technique (cf. Campbell et al., 2018) produced loading rates of approximately 0.32 g N and 0.13 g P plot-1 day-1, similar to rates in prior studies documenting the effects of eutrophication in coastal habitats (Ferdie and Fourqurean, 2004). Enrichment plots received a 350 g fertilizer bag (filled with slow-release Osmocote fertilizer, NPK 14-14-14) attached to their center posts. These nutrient ratios were selected to ensure a balanced supply of elemental resources, and this technique also allowed consistent nutrient delivery across a wide range of temperatures. To ensure consistent nutrient delivery, all bags were replaced once every four weeks. Nutrient delivery at each plot was measured by recollecting used nutrient bags and calculating the mass of Osmocote lost over the four-week period.
While the experiment ran for 1 full year, in February 2019 (9 months into the experiment), L. variegatus started appearing in at densities up to 20/m2 at the experimental array. One month later (ten months into the experiment) urchin recruitment had destroyed the experimental array and overgrazed the adjacent seagrass. Urchins were removed from the cages every two weeks but were never fully eradicated from the full cages until the experiment’s conclusion on May 1st, 2019. Given the loss of the grazing treatments, we concluded that data gathered after March 2019 could not be used for comparisons among treatments because three months of extensive urchin grazing had confounded treatment effects.
Turtlegrass response variables
Turtlegrass growth was measured using a leaf marking technique (Zieman, 1974) on all shoots within a haphazardly placed 7.62 cm diameter PVC ring inside each plot. All shoots were pierced with a hypodermic needle at the shoot base near the leaf/sediment interface and harvested after 8 days of growth. In the laboratory, new growth of the harvested shoots was dried at 60°C for at least 48 hours and weighed. Area-specific productivity (g/m2 day1) was then calculated. Shoot density was estimated by enumerating shoots within replicate 7.62 cm PVC rings in each plot and reporting their average. Percent cover was visually estimated by the same observer at intervals of 5%.
Because our original intention was to present results after the conclusion of the experiment, the sampling schedule for the different response variables varied. Turtlegrass productivity was measured four months after the experiment began and turtlegrass shoot density was estimated at four, seven and ten months after beginning the experiment. Percent cover of turtlegrass was estimated at the start and at four and seven months after beginning the experiment. While percent cover is often related to shoot density, we have analyzed and reported both for completeness.
An unanticipated massive recruitment of the variegated sea urchin, a species known for episodic recruitment in Gulf of Mexico seagrass meadows (Camp et al., 1973; Rose et al., 1999; Peterson et al., 2002), and a common resident consumer of turtlegrass in the GOM and the Caribbean (Vadas and Fenchel, 1982; Valentine et al., 1997), occurred during our study. The results of this large increase in the abundance of seagrass-consuming urchins and their breaching of experimental exclosures was first noted in late February and brought a premature end to our experiment.
Data analysis
Turtlegrass primary production and shoot density were analyzed using 2-way analyses of variance (ANOVA) with the two main factors being Cage type and Nutrient enrichment (n=5). Shoot density was analyzed using 2-way ANOVAs of values that were estimated 4 and 10 months into the experiment. Turtlegrass percent cover was analyzed using a Before-After, Control-Impact (BACI) design because percent cover was estimated in all plots before the experiment began. In these analyses, control treatment values were subtracted from caged treatment values, then each cage treatment (Partial, Full cage No clip, Full cage Half clip, Full cage Full clip) was tested using a two-way ANOVA, with date collected and nutrient enrichment as the two factors. To test whether there was a significant reduction of light in caged areas, light availability measured as PAR at the seagrass canopy height was compared between a sensor placed within a full cage and a sensor placed outside of the experimental array using a t-test. For all t-tests and ANOVAs, normality was tested using a Shapiro-Wilk test, Levene’s test of equality was used to check data for homogeneity of variance, and post-hoc comparisons were made with a Tukey analysis after assumptions were met. All analyses were performed in RStudio with R language for statistical computing (R Core Team, 2019; R Studio Team, 2019).
Results
Field surveys
The macroinvertebrate community consisted mostly of the herbivorous sea urchin, Lytechinus variegatus, which was found on every sampling date (n = 18) and averaged 5.12 ± 1.11 urchins/m2 throughout the year (Figure 3). In February 2019, a large urchin recruitment event produced an urchin front that reached densities of nearly 20 individuals/m2 as they moved into our experimental array (Figure 4A). By the end of March, they were established within the cages (Figure 4B). By April, urchin densities started to decline as they moved out of the area (Figures 3, 4C, and 5). Among other macroinvertebrates observed, the rigid pen shell Atrina rigida was found on 16 of the 18 sampling dates, but only averaged 1.13 ± 0.05 individuals/m2. Throughout the year, the commercially important bay scallop (Argopectin irradians) averaged densities 1.175 ± 1.11 scallops/m2 with the highest density seen on September 17, 2018.
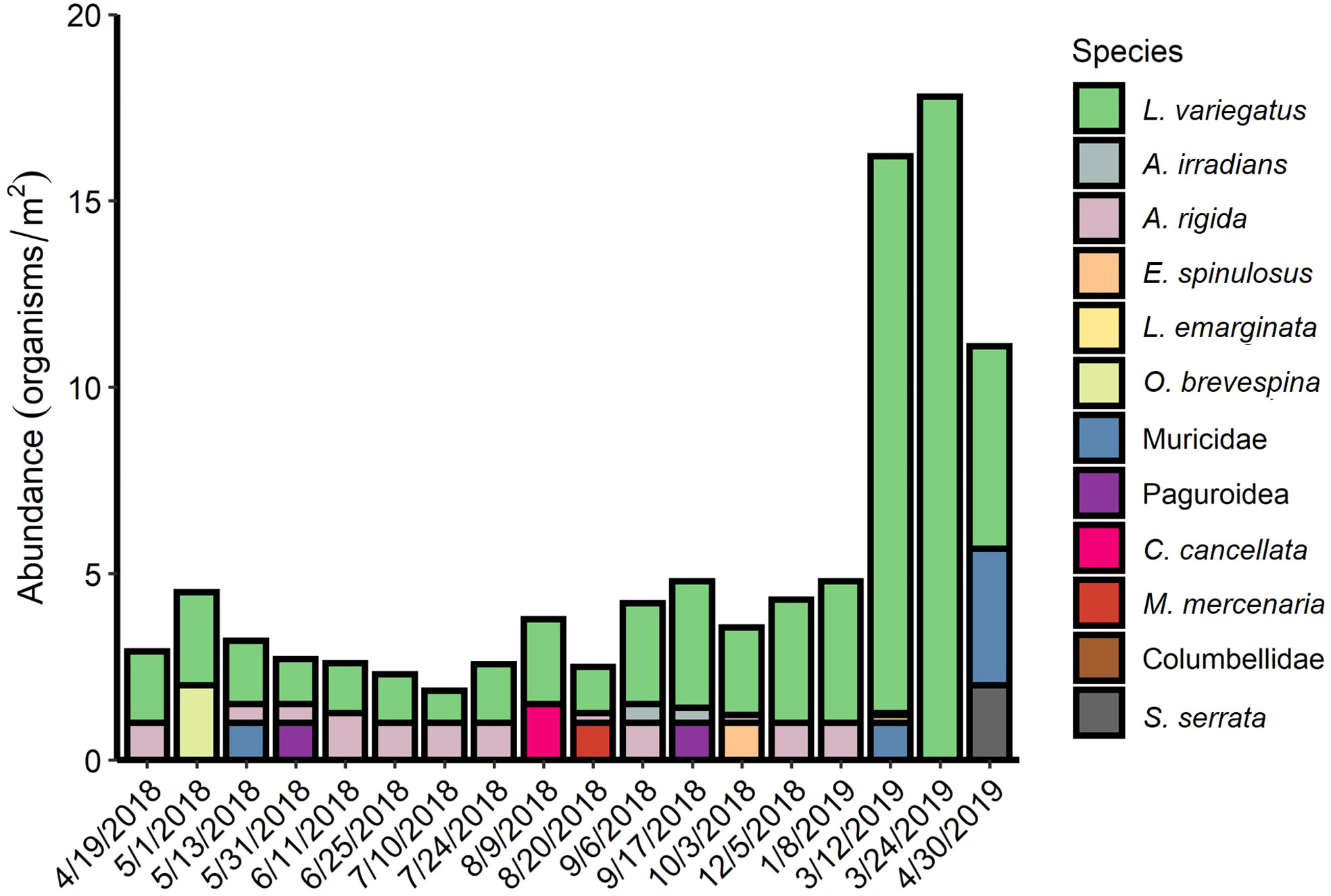
Figure 3 Macroinvertebrate survey results throughout the experimental period. The dates along the x-axis represent the dates surveys took place.
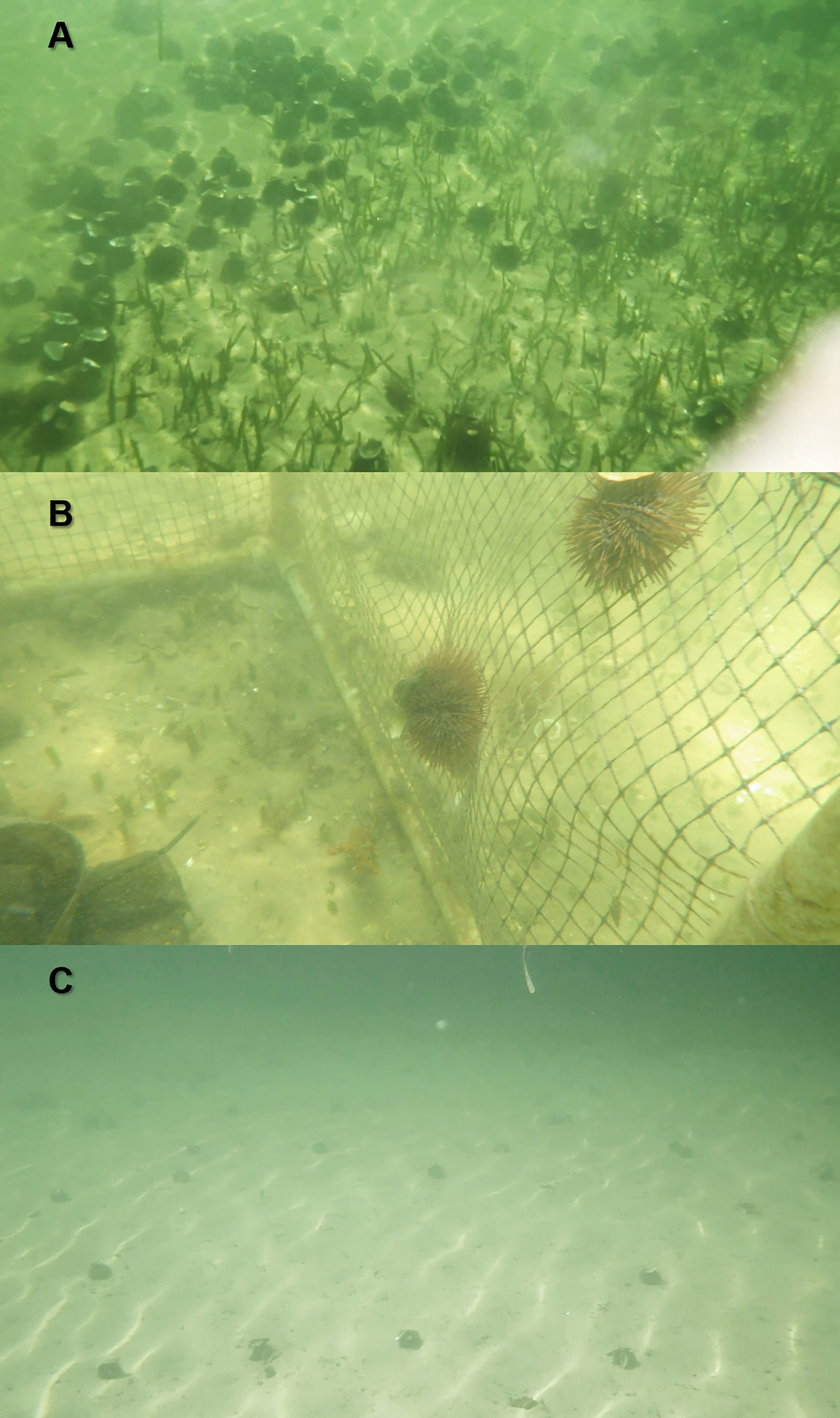
Figure 4 (A) Urchins (Lytechinus variegatus) after recruitment into the study area; (B) urchins breaching cages; and (C) study area after urchin overgrazing.
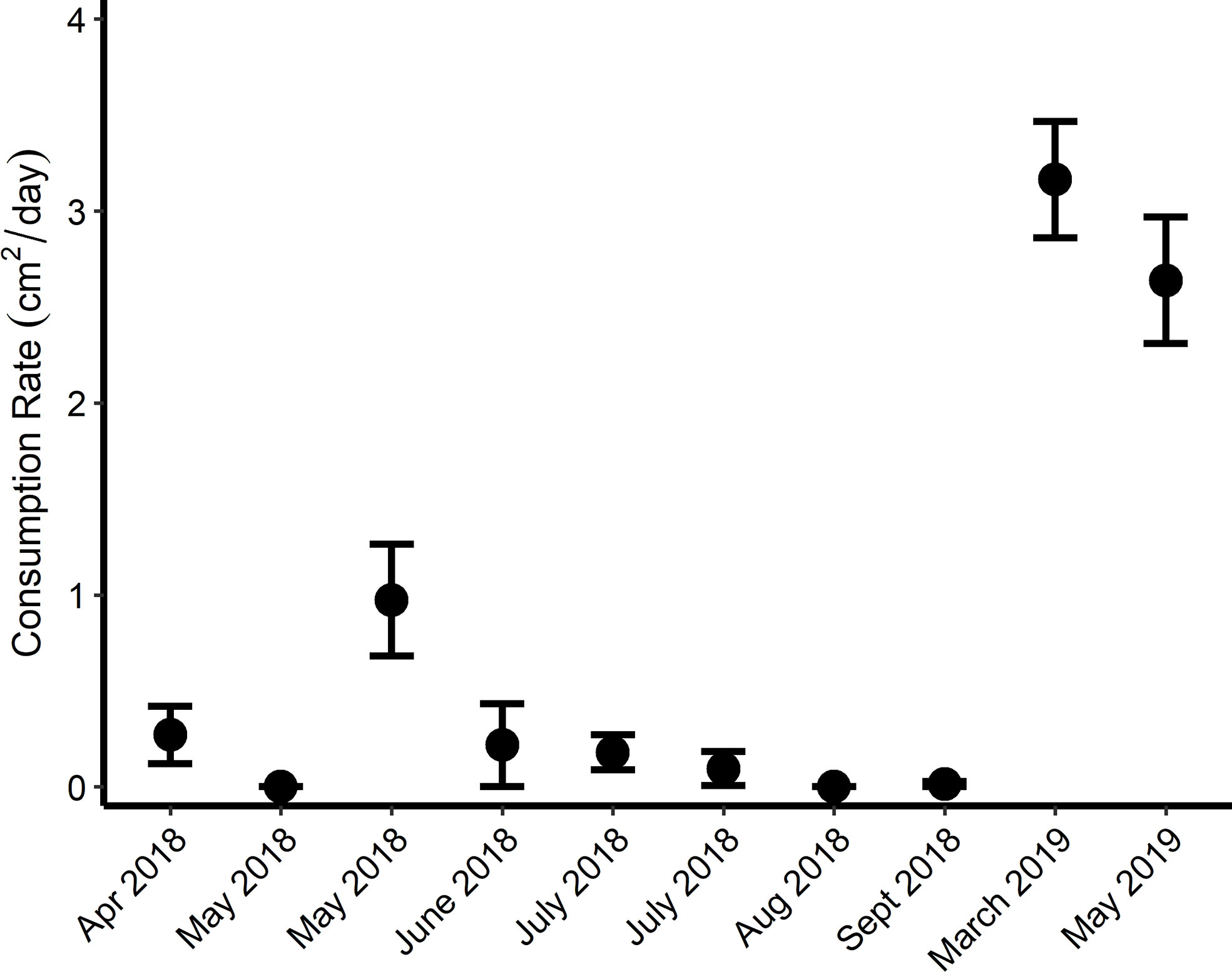
Figure 5 Consumption rates, cm2 day-1, (± 1 SE) of monthly turtlegrass (Thalassia testudinum) tethering experiments.
Turtlegrass consumption rate averaged 0.956 ± 0.089 cm2 day-1 throughout the 10 sampling periods (Figure 5). As expected, when L. variegatus density increased (beginning sometime in February but first recorded in March), so too did the average daily turtlegrass consumption rate, rising more than 10-fold to as much as 3 cm2 day-1 after the urchin recruitment event. Based on our inspection of grazing scars on the tethered shoots, we concluded that 141 of 661 leaves analyzed throughout the experiment showed evidence of grazing. Of these 141 leaves with evidence of grazing, 126 leaves had evidence of urchin grazing, 18 leaves had evidence of fish grazing, and 3 leaves had unidentifiable grazing scars.
Caging and simulated grazing experiment
Of 254 days during the experiment where both PAR loggers were deployed, there were no detectable differences in light levels between the sensor within the Full cage (46.40 ± 93.76 [SD] µmol photons m-2 s-1) and the sensor outside the experimental array (45.46 ± 99.93 [SD] µmol photons m-2 s-1) [t(98086) = 1.5311, p = 0.126].
In August 2018, 4 months into the experiment, there were no differences in T. testudinum primary production values between Cage types [F(4,38) = 0.497, p = 0.738], or nutrient-enriched plots [F(1,38) = 0.019, p = 0.891], and there was no significant interaction between the two factors [F(4,38) = 2.109, p = 0.099] (Table 1; Figure 6).
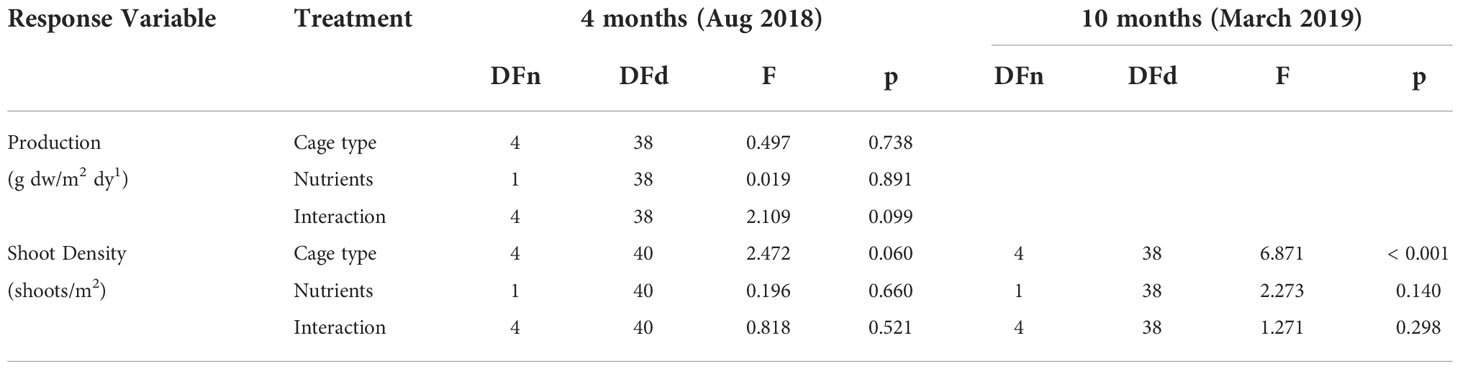
Table 1 2-way Analysis of Variance results on Thalassia testudinum response variables during a caging experiment testing Cage types and Nutrient enrichment as the Treatment variables.
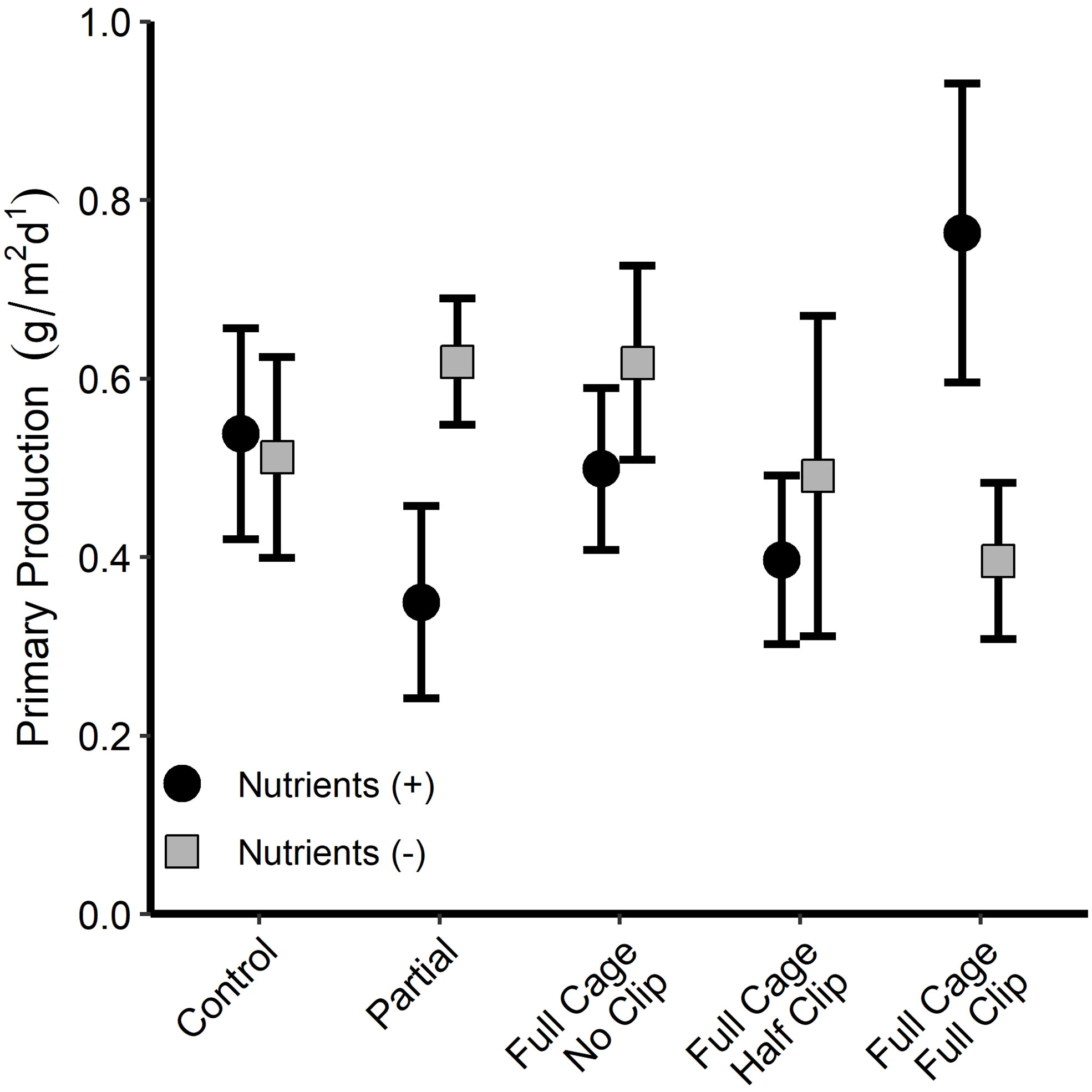
Figure 6 Mean Thalassia testudinum primary production values (± 1 SE) 4 months into the caging study. Full exclusion cage treatments also contained simulated grazing treatments. No clip = no clipping treatment. Half clip = top half of leaf clipping treatment. Full clip = fully clipped leaves (~1-2 cm above the sediment).
Four months into the experiment, there was a measurable difference in shoot densities between Cage types [F(4,40) = 2.472, p = 0.060], but no differences between nutrient treatments [F(140), = 0.196, p = 0.660], and no interactions between the two factors [F(4,40) = 0.818, p = 0.521]. The full simulated grazing treatment in full exclusion cages resulted in fewer turtlegrass shoots than in the uncaged, open control plots (Figure 7; p < 0.001). Ten months into the experiment, there was a large difference in shoot densities between Cage types [F(4,38) = 6.871, p < 0.001], but not between nutrient treatments [F(1,38) = 2.273, p = 0.140], and no interactive effects [F(4,38) = 1.271, p = 0.298]. Post-hoc comparisons revealed that the Control treatment had higher turtlegrass shoot densities than all the Full Cage treatments, regardless of clipping regime.
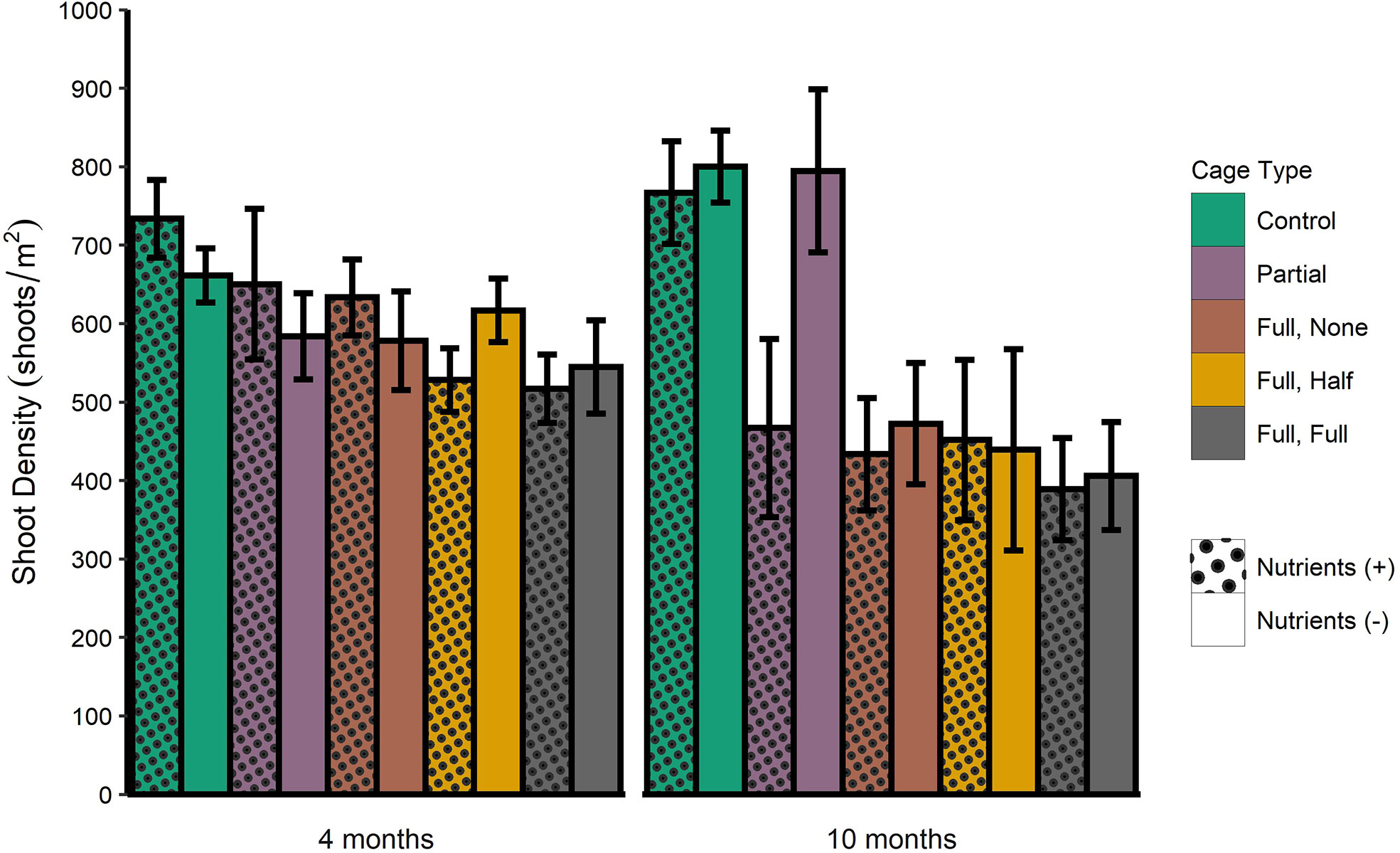
Figure 7 Thalassia testudinum shoot density means (± 1 SE) after 4 and 10 months of the caging experiment. Full exclusion cage treatments also contained simulated grazing treatments. Full, None = full cage, no clipping treatment. Full, Half = full cage, top half of leaf clipping treatment. Full, Full = full cage, fully clipped leaves (~1-2 cm above the sediment).
For percent cover (Table 2), in the partial cages (Figure 8A) there was a difference in partial cage-control treatments means between dates [F(2,24) = 9.371, p < 0.001], but no differences between nutrient treatments [F(1,24) = 2.247, p = 0.147], and no interactive effects between the factors [F(2,24) = 0.427, p = 0.657]. Post-hoc comparisons revealed that percent cover partial-control values 7 months into the experiment were lower than at the start of the experiment (p < 0.0001) and after 4 months into the experiment (p = 0.019), likely due to a structural effect where partial cages experienced greater herbivory due to green turtles being attracted to cage structure, which has been documented in this area before (Rodriguez and Heck, 2020), and was anecdotally noted within a few weeks of the experiment’s start. In the full cage treatment with no simulated grazing (Figure 8B), there was a difference in treatment-control means between dates [F(3,24) = 6.419, p = 0.006], but no differences between nutrient treatments [F(1,24) = 0.122, p = 0.730], and there were no interactive effects between the factors [F(3,24) = 0.527, p = 0.597]. Post-hoc comparisons revealed that 7 months of treatment resulted in less turtlegrass percent cover in the full cage no clipping-control values than recorded at the beginning of the experiment (p = 0.004). In the full cage treatment with the half leaf clipping treatment (Figure 8C), there was again a difference in treatment-control means between dates [F(2,23) = 31.185, p < 0.001], but no differences between nutrient treatments [F(1,23) = 0.662, p = 0.471], and no evidence of interactive effects between the factors [F(2,23) = 0.072, p = 0.930]. Post-hoc comparisons revealed that percent cover Full cage Half clip-control values 7 months into the experiment were lower than at the start of the experiment (p < 0.0001) and after 4 months into the experiment (p < 0.001).
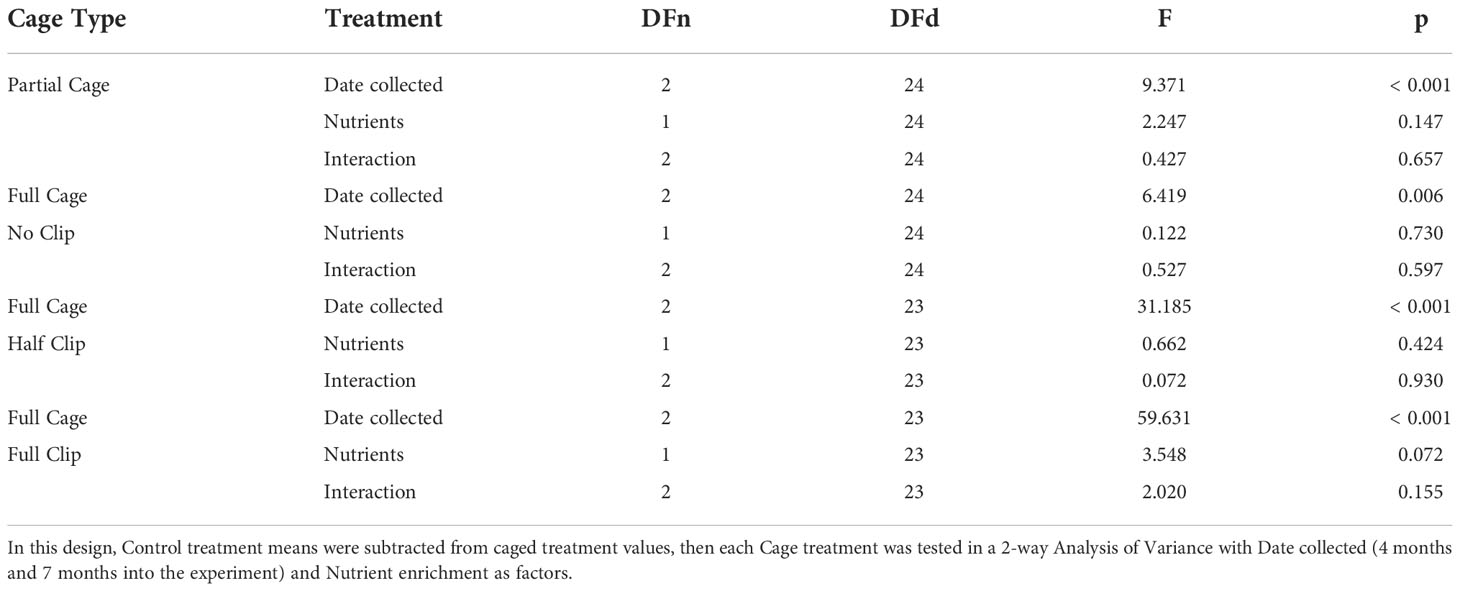
Table 2 Results of BACI (Before-After Control-Impact) design on Thalassia testudinum percent cover during a 7-month caging experiment.
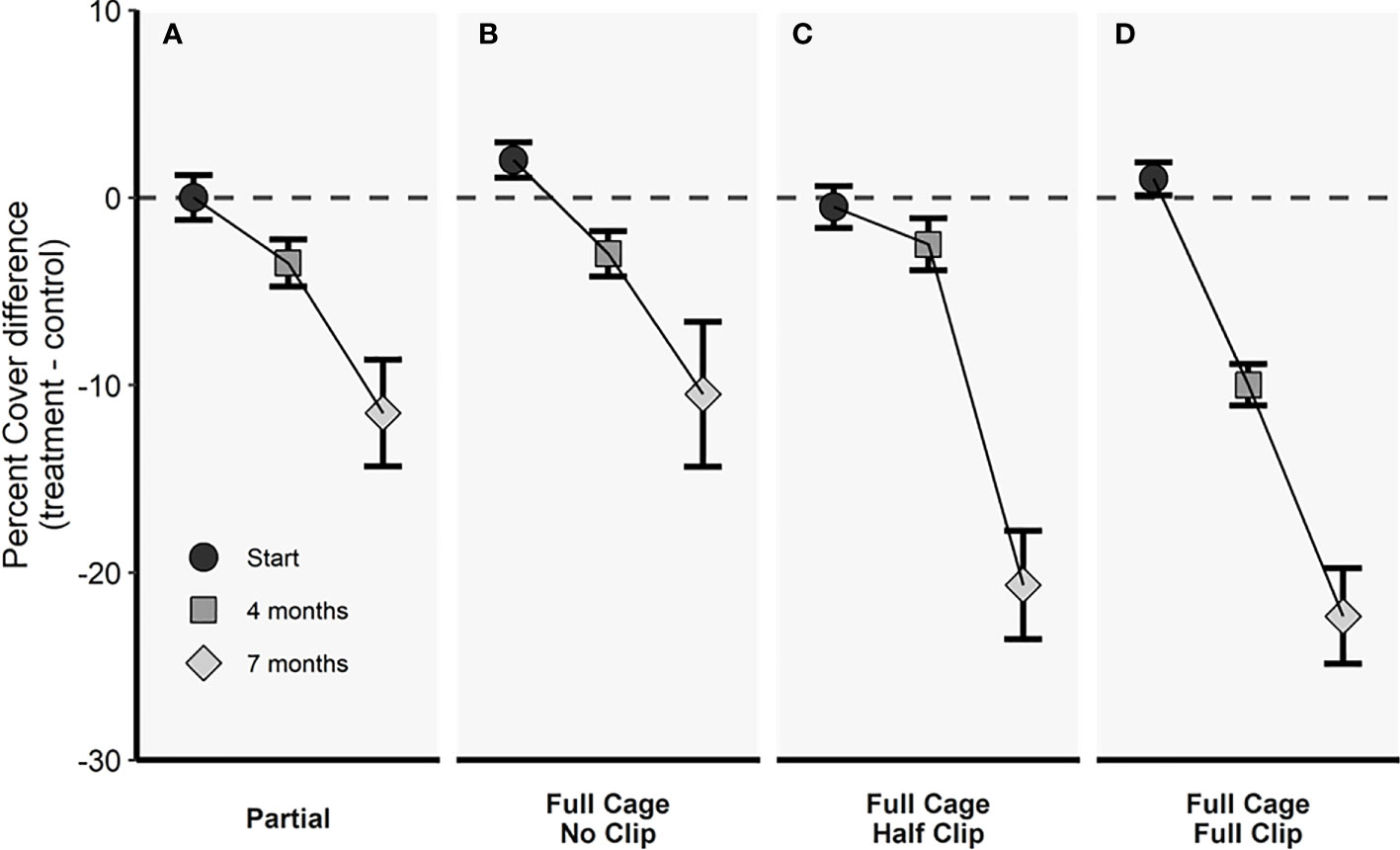
Figure 8 Thalassia testudinum percent cover treatment-control values (± 1 SE) for (A) Partial cages, (B) Full cages with no clipping treatment, (C) Full cages with half clipping treatment, and (D) Full cages with full clipping treatment throughout the caging study. The dotted line represents where there would be no difference between the treatment and control values.
In the full cage treatment with full simulated grazing (Figures 8D, 9), there was a difference in treatment-control means between dates [F(2,34) = 59.631, p < 0.001], and nutrient-enriched treatments had a higher percent cover treatment-control means (i.e., nutrient addition mitigated some simulated herbivory effects [F(1,23) = 3.548, p = 0.072]), but there were no interactive effects between the factors [F(223) = 2.020, p = 0.155]. Post-hoc comparisons revealed after 4 months treatment values were less than control values when compared to the beginning of the experiment (p < 0.001), and 7 months of treatment effects resulted in lower percent cover for the full cage full clipping-control values than at the beginning of the experiment (p < 0.001) and 4 months into the experiment (p < 0.001).
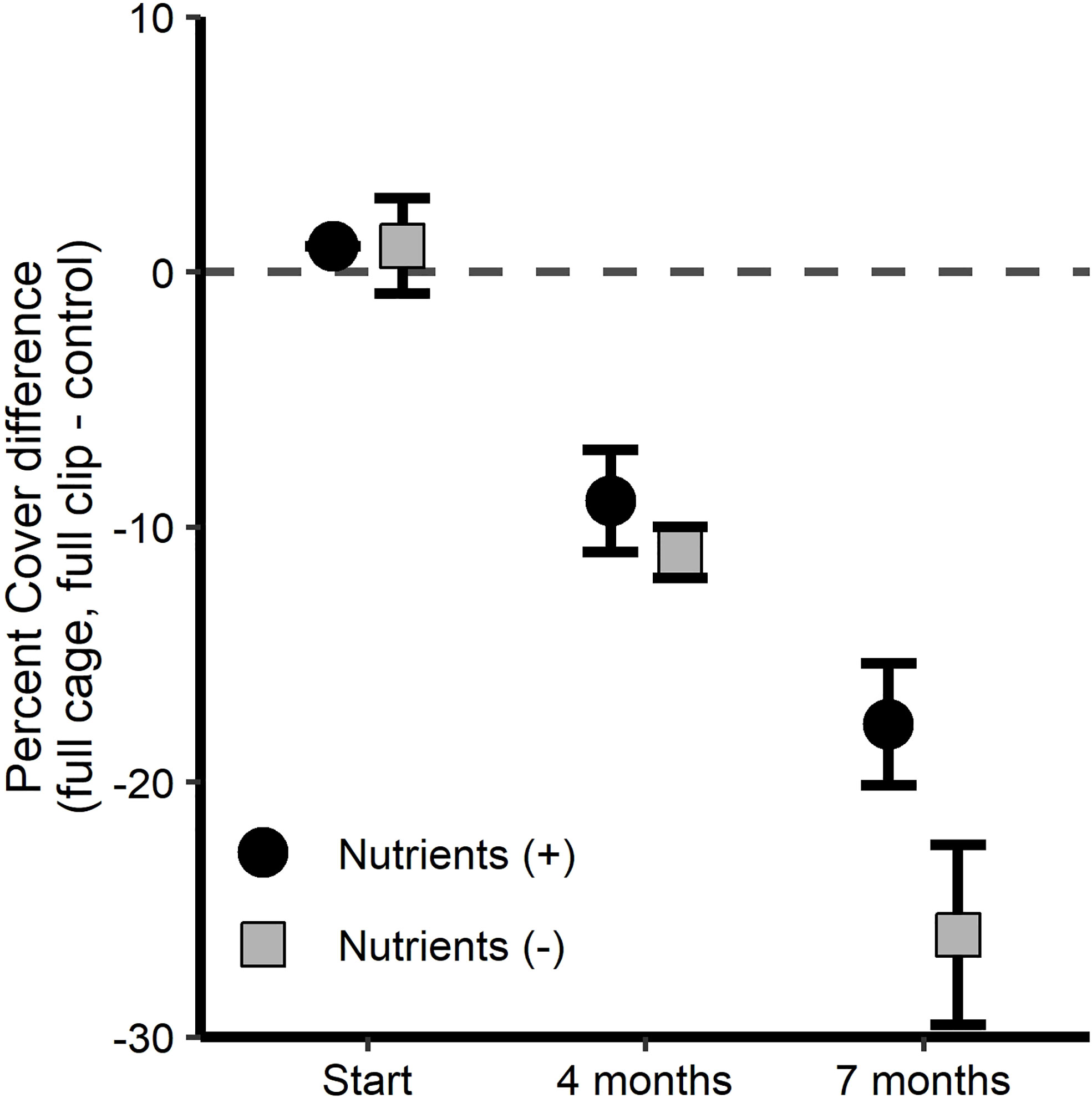
Figure 9 Thalassia testudinum percent cover treatment-control values (± 1 SE) for the full exclusion cage, full simulated grazing treatment with and without nutrient enrichment throughout the caging study.
Discussion
The macroinvertebrate community consisted mostly of the herbivorous variegated sea urchin, Lytechinus variegatus, which was found on every sampling date throughout the year. By April after most turtlegrass in the area had been consumed, urchin densities declined as they moved out of the area (Figures 3, 4C, and 5). As expected, when L. variegatus density increased (beginning sometime in February but first recorded in March), so too did the average daily turtlegrass consumption rate, which rose more than 10-fold after the urchin recruitment event. Thus, most of the consumption of the tethered T. testudinum shoots was done by urchins, with 86% of grazed leaves having evidence of urchin grazing. Fourteen percent of the grazed leaves had evidence of consumption by fish, but leaves showed no evidence of grazing by green turtles even though many green turtles were observed in the area and turtle grazing was apparent around the cage margins (Figure 10B).
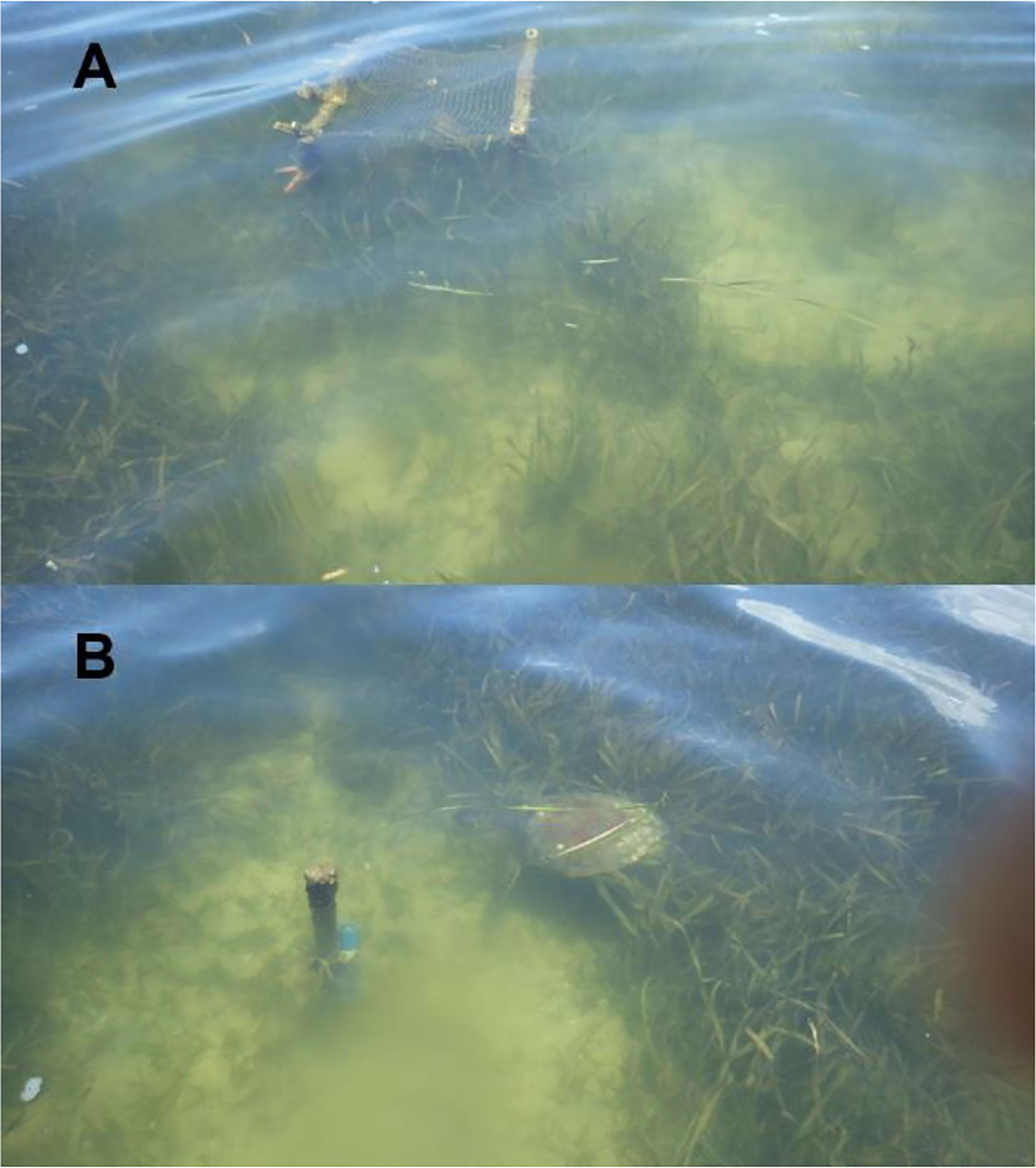
Figure 10 (A) green turtle (Chelonia mydas) grazed area adjacent to one of the cages; and (B) a juvenile green turtle entering a grazed patch near a temperature sensor just outside our experimental cage array.
Lytechinus variegatus densities at the experimental site were like previous studies in St. Joseph Bay. For example, Valentine and Heck (1991) reported L. variegatus densities in five sites to range between means of 12.5 and 24.6 individuals m−2 and up to 140 ind. m−2. Beddingfield and McClintock (2000) found L. variegatus density means (± 1 SD) to be 13.35 ± 7.5, 2.34 ± 3.4, and 0.46 ± 0.4 ind. m−2 in T. testudinum, and H. wrightii, and S. filiforme-dominated sites, respectively, and reported densities up to 35 m−2. Between 2017 and 2018 at two sites sampled after Hurricane Michael, Challener et al. (2019) reported mean densities (± 1 SD) between 2.90 ± 2.38 and 10.90 ± 7.02 ind. m−2. Rodriguez and Heck (2021) estimated L. variegatus mean densities across 24 sites around the bay in May 2016 and August 2019 and calculated means (± 1 SE) of 6.27 ± 0.09 ind. m−2 and 5.33 ± 0.08 ind. m−2, with an upper limit of 56 ind. m−2. While urchin means in most studies remain below the density suggested by Valentine and Heck (1991) required to overgraze seagrass meadows, high enough densities for overgrazing are common, though spatially heterogeneous, in St. Joseph Bay, and lead to local T. testudinum loss.
Before the massive urchin recruitment, nutrient enrichment failed to produce important detectable effects on turtlegrass in any of the treatments. Turtlegrass primary productivity, for example, showed no important differences between grazing treatments, nutrient enrichment levels, or interactions between these factors after four months (Figure 6). These results differed from those of Campbell et al. (2018), who tested the effects of nutrient enrichment and altered mesograzer and macrograzer herbivory in turtlegrass meadows at lower latitudes than the 300N St. Joseph Bay. Their study sites ranged from approximately 9-270N, with negative nutrient effects pronounced at the tropical locations (Campbell et al., 2018). However, the negative nutrient effects were caused by enhanced consumption of the nutrient-rich leaves by bucktooth parrotfish, a species known to preferentially consume N-rich seagrass tissue (Goecker et al., 2005; Prado and Heck, 2011). In the highest latitude site in the Indian River Lagoon (270N), where parrotfish were rare, nutrient effects were small and more similar to our results. Thus, the preference for N-rich leaves shown by vertebrates but not invertebrate grazers like urchins (Valentine and Heck, 2021), seems to be an important determinant of the effects of nutrient enrichment. And while the emerald parrotfish, N. usta, occur north and south of St. Joseph Bay, they do not currently exist there (Fodrie et al., 2010). Though fish grazing was observed in about 14% of grazed leaves, the area removed by fish grazing was small. However, as waters during winter continue to warm, it is likely that tropicalization of the northern Gulf of Mexico will allow tropical herbivores, including parrotfish such as N. usta and the West Indian manatee to expand their ranges and become established, thereby increasing grazing intensity in the Bay.
Turtlegrass shoot densities and percent cover also failed to show important nutrient effects or any interaction between nutrient enrichment and clipping, although simulated herbivory produced greatly reduced shoot densities and percent cover, especially in the full cage, full clip cages (Figures 7–9). While nutrient enrichment partially appeared to mitigate the effects of the full cage, full clip simulated grazing on the loss of turtlegrass (Figure 9), there is some evidence that grazers preferentially fed on nutrient-enriched open cage controls (Figure 7), which would negate this compensatory effect. We hypothesize that this preferential grazing was seen in the partial cages but not in the open control plots, because herbivores are attracted to structure. As observed previously (Rodriguez and Heck, 2020), turtle grazing within our experimental cage array was much greater than that observed in surrounding areas of St Joseph Bay, and it was concentrated near the cage structures. This means that studies of turtle grazing that use exclusion cages or other structures must be aware of the potential for enhanced grazing near structures, and is relevant to studies that establish control areas near cages because grazing in the vicinity of cages is likely to be much higher than in areas lacking structure. It would therefore be useful to review the results of prior studies to ensure that estimates of turtle consumption were not biased by their attraction to structures installed by researchers.
The nearly complete loss of turtlegrass from the entire study area after several months of urchin grazing (Figure 4C) is a dramatic result best explained by the combined effects of urchin and green turtle herbivory. Previously, Valentine and Heck (1991) have shown that densities of urchins greater than 20 individuals/m2 were sufficient to totally denude turtlegrass during spring when growth rates were relatively low compared to higher rates observed in the warmer, sunny months. Because urchin densities nearly reached average densities of 20 m-2 in spring 2019, this could have been sufficient to denude turtlegrass in our study area. However, although not quantified, green turtle grazing was also occurring at the study site (Rodriguez and Heck, 2020; Rodriguez and Heck, 2021; Figure 10). Even though we were able to keep turtles from interfering with the treatments, and turtles did not consume tethered seagrass leaves, our cage structures and water quality sampling instruments attracted turtles within days of deployment, and their grazing scars surrounding the cages and instruments were clearly observed throughout the experiment (see Figure 10A). Thus, it appears that the combination of turtle grazing and the massive urchin recruitment was sufficient to almost completely denude the entire study area. This was remarkable, especially since we chose the area for our experiment because of its lush, extensive stands of turtlegrass. Some observations shortly after the conclusion of the experiment indicated that there were a few scattered turtlegrass shoots remaining in the area, but it is unknown at this time whether turtlegrass will be able to recover to its former condition.
In conclusion, we found little evidence for nutrient effects but clear evidence of grazing effects, even before urchins recruited in such large numbers that they breached our cages (approximately 10 months into the experiment). It should be noted that because of the urchin recruitment event in early 2019, most results presented are from the warmer portion of the T. testudinum growing season in 2018. We have previously shown that T. testudinum primary production differs vastly seasonally and that, while T. testudinum exhibits some compensatory growth during summer, urchin grazing dramatically reduced productivity in the winter (Rodriguez and Heck, 2021). Because St. Joseph Bay is a relatively high latitude turtlegrass meadow, it is likely that reduced solar insolation during winter exacerbated grazing effects in this study, as has been noted in Bermuda, another high latitude location (Manuel et al., 2013). We also found that urchin densities of 20/m2, combined with green turtle grazing, were sufficient to almost completely denude several hundred hectares of very dense turtlegrass. Our findings validate previous studies (Valentine and Heck, 1991; Valentine et al., 1997; Beddingfield and McClintock, 1999; Rose et al., 1999; Valentine et al., 2000) and demonstrate that L. variegatus herbivory remains critically important in structuring seagrass meadows of St. Joseph Bay. Further, if green turtle densities (and possibly other tropical herbivores) continue to increase in St. Joseph Bay as expected, and urchin densities remain near levels we recorded in February and March of 2019, seagrass meadows in the Bay may soon be greatly reduced in size and stature (cf. Rodriguez and Heck, 2021), similar to what has been observed in Bermuda and Mexico after increases in green turtle abundance and grazing (Fourqurean et al., 2010; Molina Hernández and van Tussenbroek, 2014; Fourqurean et al., 2019). Therefore, top-down control of macroherbivores may eventually be necessary (Heithaus et al., 2014) to find the balance between herbivore- and seagrass-dominated systems that will maximize the ecosystem services provided by seagrasses (Scott et al., 2018).
Data availability statement
The datasets presented in this study can be found in online repositories. The names of the repository/repositories and accession number(s) can be found below: https://www.bco-dmo.org/project/750843.
Author contributions
Conceptualization and methodology: KH, JC. Formal analysis: AR. Investigation: AR, CM-M & KH. Resources: KH, JC. Data curation: AR & KH. Writing: AR, KH, & CM-M. Project administration: AR, JC. Funding acquisition: KH, JC. All authors have read and agreed to the published version of the manuscript. All authors contributed to the article and approved the submitted version.
Funding
This research was funded by the National Science Foundation Division of Ocean Sciences Grant Number 2019022 and NSF Dauphin Island Sea Lab Grant Number 1737144.
Acknowledgments
We thank current and past members of the Marine Ecology lab at the Dauphin Island Sea Lab (DISL) for their field and laboratoryassistance, in particular Samantha Linhardt. We would like to thank the Thalassia Experimental Network, in particular Olivia Rhoades andCalvin Munson. We also thank Presnell’s Bayside Marina and RV Resort in Port St. Joe, FL for allowing us to use their boat launch and boat slips. We acknowledge the National Science Foundation Biological Oceanography Program for funding this project.
Conflict of interest
The authors declare that the research was conducted in the absence of any commercial or financial relationships that could be construed as a potential conflict of interest.
Publisher’s note
All claims expressed in this article are solely those of the authors and do not necessarily represent those of their affiliated organizations, or those of the publisher, the editors and the reviewers. Any product that may be evaluated in this article, or claim that may be made by its manufacturer, is not guaranteed or endorsed by the publisher.
References
Avens L., Goshe L., Harms C., Anderson E., Goodman Hall A., Cluse W., et al. (2012). Population characteristics, age structure, and growth dynamics of neritic juvenile green turtles in the northeastern gulf of Mexico. Mar. Ecol. Prog. Ser. 458, 213–229. doi: 10.3354/meps09720
Backman T. W., Barilotti D. C. (1976). Irradiance reduction: Effects on standing crops of the eelgrass zostera marina in a coastal lagoon. Mar. Biol. 34, 33–40. doi: 10.1007/BF00390785
Bakker C., Van Bodegom P. M., Nelissen H. J. M., Ernst W. H. O., Aerts R. (2006). Plant responses to rising water tables and nutrient management in calcareous dune slacks. Plant Ecol. 185, 19–28. doi: 10.1007/s11258-005-9080-5
Beddingfield S. D., McClintock J. B. (1999). Food resource utilization in the Sea urchin lytechinus variegatus in contrasting shallow-water microhabits of saint Joseph bay, Florida. Gulf Mex. Sci. 17, 27–34. doi: 10.18785/goms.1701.03
Beddingfield S. D., McClintock J. B. (2000). Demographic characteristics of Lytechinus variegatus (Echinoidea: Echinodermata) from three habitats in a North Florida Bay, Gulf of Mexico. Mar. Ecol. 21, 17–40. doi: 10.1046/j.1439-0485.2000.00688.x
Bjorndal K. A. (1980). Nutrition and grazing behavior of the green turtle chelonia mydas. Mar. Biol. 56, 147–154. doi: 10.1007/BF00397131
Blois J. L., Zarnetske P. L., Fitzpatrick M. C., Finnegan S. (2013). Climate change and the past, present, and future of biotic interactions. Science 341, 499–504. doi: 10.1126/science.1237184
Campbell J. E., Altieri A. H., Johnston L. N., Kuempel C. D., Paperno R., Paul V. J., et al. (2018). Herbivore community determines the magnitude and mechanism of nutrient effects on subtropical and tropical seagrasses. J. Ecol. 106, 401–412. doi: 10.1111/1365-2745.12862
Camp D. K., Cobb S. P., van Breedveld J. F. (1973). Overgrazing of seagrasses by a regular urchin, “Lytechinus variegatus.” BioScience 23, 37–38. doi: 10.2307/1296366
Challener R., McClintock J. B., Czaja R., Pomory C. (2019). Rapid assessment of post-hurricane Michael impacts on a population of the Sea urchin lytechinus variegatus in seagrass beds of eagle harbor, port saint Joseph bay, Florida. Gulf Caribb. Res. 30, SC11–SC16. doi: 10.18785/gcr.3001.07
Christianen M. J. A., Govers L. L., Bouma T. J., Kiswara W., Roelofs J. G. M., Lamers L. P. M., et al. (2012). Marine megaherbivore grazing may increase seagrass tolerance to high nutrient loads. J. Ecol. 100, 546–560. doi: 10.1111/j.1365-2745.2011.01900.x
Costanza R., d’Arge R., de Groot R., Farber S., Grasso M., Hannon B., et al. (1997). The value of the world’s ecosystem services and natural capital. Ecol. Econ. 25, 3–15. doi: 10.1016/S0921-8009(98)00020-2
Dennison W. C. (1987). Effects of light on seagrass photosynthesis, growth and depth distribution. Aquat. Bot. 27, 15–26. doi: 10.1016/0304-3770(87)90083-0
Estes J. A., Terborgh J., Brashares J. S., Power M. E., Berger J., Bond W. J., et al. (2011). Trophic Downgrading of Planet Earth. Science 333, 301–306. doi: 10.1126/science.1205106
Ferdie M., Fourqurean J. W. (2004). Responses of seagrass communities to fertilization along a gradient of relative availability of nitrogen and phosphorus in a carbonate environment. Limnol. Oceanogr. 49, 2082–2094. doi: 10.4319/lo.2004.49.6.2082
Fodrie F., Heck K., Powers S., Graham W., Robinson K. (2010). Climate-related, decadal-scale assemblage changes of seagrass-associated fishes in the northern gulf of Mexico. Glob. Change Biol. 16, 48–59. doi: 10.1111/j.1365-2486.2009.01889.x
Foley A., Singel K., Dutton P., Summers T., Redlow A., Lessman J. (2007). Characteristics of a green turtle (Chelonia mydas) assemblage in northwestern Florida determined during a hypothermic stunning event. Gulf Mex. Sci. 25, 131–143. doi: 10.18785/goms.2502.04
Fourqurean J. W., Manuel S., Coates K., Kenworthy W., Smith S. (2010). Effects of excluding sea turtle herbivores from a seagrass bed: Overgrazing may have led to loss of seagrass meadows in Bermuda. Mar. Ecol. Prog. Ser. 419, 223–232. doi: 10.3354/meps08853
Fourqurean J. W., Manuel S. A., Coates K. A., Massey S. C., Kenworthy W. J. (2019). Decadal monitoring in Bermuda shows a widespread loss of seagrasses attributable to overgrazing by the green Sea turtle chelonia mydas. Estuaries Coasts 42, 1524–1540. doi: 10.1007/s12237-019-00587-1
Gangal M., Gafoor A.-B., D’Souza E., Kelkar N., Karkarey R., Marbà N., et al. (2021). Sequential overgrazing by green turtles causes archipelago-wide functional extinctions of seagrass meadows. Biol. Conserv. 260, 109195. doi: 10.1016/j.biocon.2021.109195
Goecker M., Heck K., Valentine J. (2005). Effects of nitrogen concentrations in turtlegrass thalassia testudinum on consumption by the bucktooth parrotfish sparisoma radians. Mar. Ecol. Prog. Ser. 286, 239–248. doi: 10.3354/meps286239
Heck K., Fodrie F., Madsen S., Baillie C., Byron D. (2015). Seagrass consumption by native and a tropically associated fish species: potential impacts of the tropicalization of the northern gulf of Mexico. Mar. Ecol. Prog. Ser. 520, 165–173. doi: 10.3354/meps11104
Heck K. L., Valentine J. F. (2006). Plant–herbivore interactions in seagrass meadows. J. Exp. Mar. Biol. Ecol. 330, 420–436. doi: 10.1016/j.jembe.2005.12.044
Heithaus M., Alcoverro T., Arthur R., Burkholder D., Coates K., Christianen M., et al. (2014). Seagrasses in the age of sea turtle conservation and shark overfishing. Front. Mar. Sci. 1. doi: 10.3389/fmars.2014.00028
Herzka S., Dunton K. (1997). Seasonal photosynthetic patterns of the seagrass thalassia testudinum in the western gulf of Mexico. Mar. Ecol. Prog. Ser. 152, 103–117. doi: 10.3354/meps152103
Holzer K. K., McGlathery K. J. (2016). Cultivation grazing response in seagrass may depend on phosphorus availability. Mar. Biol. 163, 88. doi: 10.1007/s00227-016-2855-5
Ibarra-Obando S. E., Heck K. L., Spitzer P. M. (2004). Effects of simultaneous changes in light, nutrients, and herbivory levels, on the structure and function of a subtropical turtlegrass meadow. J. Exp. Mar. Biol. Ecol. 301, 193–224. doi: 10.1016/j.jembe.2003.10.001
Kelkar N., Arthur R., Marba N., Alcoverro T. (2013a). Green turtle herbivory dominates the fate of seagrass primary production in the Lakshadweep islands (Indian ocean). Mar. Ecol. Prog. Ser. 485, 235–243. doi: 10.3354/meps10406
Kelkar N., Arthur R., Marbà N., Alcoverro T. (2013b). Greener pastures? high-density feeding aggregations of green turtles precipitate species shifts in seagrass meadows. J. Ecol. 101, 1158–1168. doi: 10.1111/1365-2745.12122
Kirsch K., Valentine J., Heck K. (2002). Parrotfish grazing on turtlegrass thalassia testudinum: evidence for the importance of seagrass consumption in food web dynamics of the Florida keys national marine sanctuary. Mar. Ecol. Prog. Ser. 227, 71–85. doi: 10.3354/meps227071
Lamont M., Fujisaki I., Stephens B., Hackett C. (2015). Home range and habitat use of juvenile green turtles (Chelonia mydas) in the northern gulf of Mexico. Anim. Biotelemetry 3, 1–12. doi: 10.1186/s40317-015-0089-9
Lamont M., Iverson A. (2018). Shared habitat use by juveniles of three sea turtle species. Mar. Ecol. Prog. Ser. 606, 187–200. doi: 10.3354/meps12748
Lee K.-S., Dunton K. H. (1997). Effect of in situ light reduction on the maintenance, growth and partitioning of carbon resources in thalassia testudinum banks ex könig. J. Exp. Mar. Biol. Ecol. 210, 53–73. doi: 10.1016/S0022-0981(96)02720-7
Major K. M., Dunton K. H. (2002). Variations in light-harvesting characteristics of the seagrass, thalassia testudinum: evidence for photoacclimation. J. Exp. Mar. Biol. Ecol. 275, 173–189. doi: 10.1016/S0022-0981(02)00212-5
Manuel S. A., Coates K. A., Kenworthy W. J., Fourqurean J. W. (2013). Tropical species at the northern limit of their range: Composition and distribution in bermuda’s benthic habitats in relation to depth and light availability. Mar. Environ. Res. 89, 63–75. doi: 10.1016/j.marenvres.2013.05.003
Marco-Méndez C., Prado P., Heck K., Cebrián J., Sánchez-Lizaso J. (2012). Epiphytes mediate the trophic role of sea urchins in thalassia testudinum seagrass beds. Mar. Ecol. Prog. Ser. 460, 91–100. doi: 10.3354/meps09781
McMichael E. (2005). Ecology of juvenile green turtles, chelonia mydas, at a temperate foraging area in the northeastern gulf of Mexico. (Florida, USA: University of Florida, Gainesville).
Molina Hernández A., van Tussenbroek B. (2014). Patch dynamics and species shifts in seagrass communities under moderate and high grazing pressure by green sea turtles. Mar. Ecol. Prog. Ser. 517, 143–157. doi: 10.3354/meps11068
Moran K. L., Bjorndal K. A. (2005). Simulated green turtle grazing affects structure and productivity of seagrass pastures. Mar. Ecol. Prog. Ser. 305, 235–247. doi: 10.3354/meps305235
Orth R., Carruthers T., Dennison W., Duarte C., Fourqurean J. W., Heck K., et al. (2006). A global crisis for seagrass ecosystems. BioScience 56, 987–996. doi: 10.1641/0006-3568(2006)56[987:AGCFSE]2.0.CO;2
Osland M. J., Stevens P. W., Lamont M. M., Brusca R. C., Hart K. M., Waddle J. H., et al. (2021). Tropicalization of temperate ecosystems in north America: The northward range expansion of tropical organisms in response to warming winter temperatures. Glob. Change Biol. 27, 3009–3034. doi: 10.1111/gcb.15563
Peterson B. J., Rose C. D., Rutten L. M., Fourqurean J. W. (2002). Disturbance and recovery following catastrophic grazing: Studies of a successional chronosequence in a seagrass bed. Oikos 97, 361–370. doi: 10.1034/j.1600-0706.2002.970306.x
Poore A. G. B., Campbell A. H., Coleman R. A., Edgar G. J., Jormalainen V., Reynolds P. L., et al. (2012). Global patterns in the impact of marine herbivores on benthic primary producers. Ecol. Lett. 15, 912–922. doi: 10.1111/j.1461-0248.2012.01804.x
Prado P., Heck K. (2011). Seagrass selection by omnivorous and herbivorous consumers: Determining factors. Mar. Ecol. Prog. Ser. 429, 45–55. doi: 10.3354/meps09076
R Core Team (2019) R: A language and environment for statistical computing. Available at: https://www.R-project.org/ (Accessed October 28, 2019).
Rodriguez A., Heck K. (2020). Green turtle herbivory and its effects on the warm, temperate seagrass meadows of st. Joseph bay, Florida (USA). Mar. Ecol. Prog. Ser 639, 37–51. doi: 10.3354/meps13285
Rodriguez A. R., Heck K. L. (2021). Approaching a tipping point? herbivore carrying capacity estimates in a rapidly changing, seagrass-dominated Florida bay. Estuaries Coasts 44, 522–534. doi: 10.1007/s12237-020-00866-2
Rose C. D., Sharp W. C., Kenworthy W. J., Hunt J. H., Lyons W. G., Prager E. J., et al. (1999). Overgrazing of a large seagrass bed by the sea urchin lytechinus variegatus in outer Florida bay. Mar. Ecol. Prog. Ser. 190, 211–222. doi: 10.3354/meps190211
Scott A. L., York P. H., Duncan C., Macreadie P. I., Connolly R. M., Ellis M. T., et al. (2018). The role of herbivory in structuring tropical seagrass ecosystem service delivery. Front. Plant Sci. 9. doi: 10.3389/fpls.2018.00127
Serisawa Y., Imoto Z., Ishikawa T., Ohno M. (2004). Decline of the ecklonia cava population associated with increased seawater temperatures in tosa bay, southern Japan. Fish. Sci. 70, 189–191. doi: 10.1111/j.0919-9268.2004.00788.x
Stewart R. A., Gorsline D. S. (1962). Recent sedimentary history of st. Joseph bay, Florida. Sedimentology 1, 256–286. doi: 10.1111/j.1365-3091.1962.tb01150.x
Tanaka K., Taino S., Haraguchi H., Prendergast G., Hiraoka M. (2012). Warming off southwestern Japan linked to distributional shifts of subtidal canopy-forming seaweeds. Ecol. Evol. 2, 2854–2865. doi: 10.1002/ece3.391
Vadas R. L., Fenchel T., Ogden J.C. (1982). Ecological studies on the sea urchin, lytechinus variegatus, and the algal-seagrass communities of the miskito cays, Nicaragua.Aquat. Bot. 14, 109–125. doi: 10.1016/0304-3770(82)90091-2
Valentine J. F., Heck K. L. (1991). The role of sea urchin grazing in regulating subtropical seagrass meadows: Evidence from field manipulations in the northern gulf of Mexico. J. Exp. Mar. Biol. Ecol. 154, 215–230. doi: 10.1016/0022-0981(91)90165-S
Valentine J. F., Heck K. L. (2021). Herbivory in seagrass meadows: An evolving paradigm. Estuaries Coasts 44, 491–505. doi: 10.1007/s12237-020-00849-3
Valentine J. F., Heck K., Busby J. Jr., Webb D. (1997). Experimental evidence that herbivory increases shoot density and productivity in a subtropical turtlegrass (Thalassia testudinum) meadow. Oecologia 112, 193–200. doi: 10.1007/s004420050300
Valentine J. F., Heck K. L., Kirsch K., Webb D. (2000). Role of sea urchin lytechinus variegatus grazing in regulating subtropical turtlegrass thalassia testudinum meadows in the Florida keys (USA). Mar. Ecol. Prog. Ser. 200, 213–228. doi: 10.3354/meps200213
Valentine J. F., Heck K. L. (1993). Mussels in seagrass meadows: Their influence on macroinvertebrate abundance and secondary production in the northern Gulf of Mexico. Mar. Ecol. Prog. Ser. 96, 63–74. doi: 10.3354/meps096063
Vergés A., McCosker E., Mayer-Pinto M., Coleman M. A., Wernberg T., Ainsworth T., et al. (2019). Tropicalisation of temperate reefs: Implications for ecosystem functions and management actions. Funct. Ecol. 33, 1000–1013. doi: 10.1111/1365-2435.13310
Vergés A., Doropoulos C., Malcolm H. A., Skye M., Garcia-Pizá M., Marzinelli E. M., et al. (2016). Long-term empirical evidence of ocean warming leading to tropicalization of fish communities, increased herbivory, and loss of kelp. Proc. Natl. Acad. Sci. 113, 13791–13796. doi: 10.1073/pnas.1610725113
Vergés A., Steinberg P. D., Hay M. E., Poore A. G. B., Campbell A. H., Ballesteros E., et al. (2014a). The tropicalization of temperate marine ecosystems: Climate-mediated changes in herbivory and community phase shifts. Proc. R. Soc B Biol. Sci. 281, 1–10. doi: 10.1098/rspb.2014.0846
Vergés A., Tomas F., Cebrian E., Ballesteros E., Kizilkaya Z., Dendrinos P., et al. (2014b). Tropical rabbitfish and the deforestation of a warming temperate sea. J. Ecol. 102, 1518–1527. doi: 10.1111/1365-2745.12324
Waycott M., Duarte C. M., Carruthers T. J., Orth R. J., Dennison W. C., Olyarnik S., et al. (2009). Accelerating loss of seagrasses across the globe threatens coastal ecosystems. Proc. Natl. Acad. Sci. 106, 12377–12381. doi: 10.1073/pnas.0905620106
Wernberg T., Bennett S., Babcock R. C., Bettignies T., Cure K., Depczynski M., et al. (2016). Climate-driven regime shift of a temperate marine ecosystem. Science 353, 169–172. doi: 10.1126/science.aad8745
Williams N., Bjorndal K. A., Lamont M., Carthy R. (2014). Winter diets of immature green turtles (Chelonia mydas) on a northern feeding ground: Integrating stomach contents and stable isotope analyses. Estuaries Coasts 37, 986–994. doi: 10.1007/s12237-013-9741-x
Yarbro L. A., Carlson P. R. (2016) Summary report for st. Joseph bay. st. Petersburg, Florida: Fish and wildlife research institute - FWCC. Available at: http://myfwc.com/media/4139007/st-joseph-bay.pdf (Accessed February 6, 2018).
Zarco-Perello S., Langlois T. J., Holmes T., Vanderklift M. A., Wernberg T. (2019). Overwintering tropical herbivores accelerate detritus production on temperate reefs. Proc. R. Soc B Biol. Sci. 286, 20192046. doi: 10.1098/rspb.2019.2046
Keywords: seagrass, sea urchin, green turtles, herbivory, grazing, Thalassia testudinum, Lytechinus variegatus
Citation: Rodriguez AR, Marco-Méndez C, Campbell J and Heck KL Jr (2022) Effects of varying types and amounts of herbivory and nutrient enrichment on a tropicalizing seagrass meadow. Front. Mar. Sci. 9:892219. doi: 10.3389/fmars.2022.892219
Received: 08 March 2022; Accepted: 06 September 2022;
Published: 20 December 2022.
Edited by:
Angel Borja, Technology Center Expert in Marine and Food Innovation (AZTI), SpainReviewed by:
Christian Todd Hayes, Waynesburg University, United StatesSavanna Barry, University of Florida, United States
Copyright © 2022 Rodriguez, Marco-Méndez, Campbell and Heck. This is an open-access article distributed under the terms of the Creative Commons Attribution License (CC BY). The use, distribution or reproduction in other forums is permitted, provided the original author(s) and the copyright owner(s) are credited and that the original publication in this journal is cited, in accordance with accepted academic practice. No use, distribution or reproduction is permitted which does not comply with these terms.
*Correspondence: Alexandra R. Rodriguez, arodriguez@disl.edu