Invading the Occupied Niche: How a Parasitic Copepod of Introduced Oysters Can Expel a Congener From Native Mussels
- 1Coastal Ecology Section, Wadden Sea Station Sylt, Alfred Wegener Institute, Helmholtz Centre for Polar and Marine Research, List/Sylt, Germany
- 2Sorbonne Université, CNRS, Station Biologique de Roscoff, Laboratoire Adaptation et Diversité en Milieu Marin, UMR 7144, CS90074, Roscoff Cedex, France
In species introductions, non-native species are often confronted with new niches occupied by more specialized natives, and for introduced parasites this conflict can be amplified because they also face novel hosts. Despite these obstacles, invasions of introduced parasites occur frequently, but the mechanisms that facilitate parasite invasion success are only rarely explored. Here, we investigated how the parasitic copepod Mytilicola orientalis, that recently spilled over from its principal host - the Pacific oyster Crassostrea gigas, managed to invade the niche of blue mussel Mytilus edulis intestines, which is densely occupied by its specialist congener, Mytilicola intestinalis. From field observations demonstrating invasion dynamics in nature, we designed a series of experiments addressing potential mechanisms facilitating a successful occupation of the new niche. As expected the specialist M. intestinalis can only infect mussel hosts, but displayed higher infection success there than M. orientalis in both principal host species combined. In the absence of direct competitive interactions M. orientalis compensated its lower infection success (1) by recurrent spill-over from its high-fitness reservoir oyster host, and (2) by active aggregation interference enhancing its own mating success while limiting that of M. intestinalis. The introduced parasite could thus avoid direct competition by changing its own epidemiology and indirectly decreasing the reproductive success of its competitor in the new host. Such mechanisms outside of direct competition have seldom been considered, but are crucial to understand invasion success, parasite host range and community assembly in the context of species introductions.
1 Introduction
Fuelled by global shipping and aquaculture activities marine ecosystems experienced an increasing number of species introductions in recent years (Bailey et al., 2020). When becoming invasive, introduced species can have a series of economic (Cuthbert et al., 2021) and foremost ecological consequences (Anton et al., 2019). In comparison, invasive parasites and their effect are investigated only rarely (Bailey et al., 2020). Recently several studies investigated the effect of invasive parasites and showed that parasites can have profound effects when introduced parasites cause disease (Bouwmeester et al., 2021) and affect invasion processes of free living species (Goedknegt et al., 2016a). Invasive parasites face a new environment that fights back – the host. Within the host they will often encounter established native parasites further intensifying selective pressures by competition exposing invaders to series of disadvantages. First of all, the newly introduced parasite will be rare, which alone can prevent establishment (Quigley et al., 2018). Furthermore, the introduced parasite will by definition be less adapted to the new host than the established parasite that has shared some coevolutionary history with the hosts (Feis et al., 2016; Blakeslee et al., 2020). Despite these unfavorable starting conditions, there are many examples of parasites that defy the odds and successfully invade occupied host niches (Goedknegt et al., 2016a). However, hardly anything is known about the mechanistic basis of species interactions and competition when introduced parasites meet native ones.
In general, host-parasite coupled to parasite-parasite coevolution could lead to the use of separate niches within the same host to avoid competition (Holmes, 1973). Consequently, research efforts have focused on competitive interactions to explain parasite community assembly and niche use within hosts (Mideo, 2009). In this context studies found superiority effects in simultaneous co-infections (Leung and Poulin, 2011), often finding that more generalistic species with a wider host range have a greater sensitivity to competition than specialists representing a cost of generalism (Dawson et al., 2000; Okabe et al., 2012). Similarly, priority effects in sequential infections revealed either antagonistic (Hoverman et al., 2013), facilitative (Halliday et al., 2020) but also no detectable interaction (Zilio and Koella, 2020) between prior and later infections. These different outcomes of competitive interaction among parasites can be attributed to their shared coevolutionary history, and therefore do not offer a direct comparison of the fitness landscapes parasites face when initially confronting each other. In recent biological invasions these conditions can be tested (Feis et al., 2016; Blakeslee et al., 2020). Introduced parasites can be forced to invade new niches (Frankel et al., 2015; Tepolt et al., 2020) that will often be occupied by native parasites (Goedknegt et al., 2016a) making them ideal systems to investigate the species interactions at the onset of competition.
Here, we utilize the recent invasion of the parasitic copepod Mytilicola orientalis leading to novel interactions with its congener Mytilicola intestinalis. M. orientalis was co-introduced with Pacific oysters Crassostrea gigas around the globe and also invaded the European Wadden Sea in the last decades (Feis et al., 2019), where it is displacing M. intestinalis in its specialized niche (Figure 1A). Spatially both parasites use the same niche and infest intestines of mollusks (Goedknegt et al., 2018b), where they attach with hooklike structures to the intestinal wall (Figueras et al., 1991; Bignell et al., 2008). Parasite attachment causes lesions and inflammations (Watermann et al., 2008) that invoke a costly immune response (Santarem et al., 1994) and can lead to reduced body condition (Goedknegt et al., 2018a). Relative to their intestinal habitat both parasites can reach considerable sizes (up to 12 mm (Goedknegt et al., 2018a)). Their large size and high infection prevalences and intensities spatially restrict niche availability, leading to competition between individuals (Feis et al., 2016). In the Wadden Sea, Mytilicola intestinalis is found exclusively in blue mussels Mytilus edulis (Elsner et al., 2011; Goedknegt, 2017), creating a narrow realized host range characteristic for specialist parasites. Mytilicola orientalis, on the other hand, has been globally distributed with aquaculture of Pacific oysters (Feis et al., 2019), from which it repeatedly spilled over into native Mytilus populations and rarely into other mollusks (Stock, 1993; Goedknegt et al., 2016b), thus displaying a wider host range than M. intestinalis. Both species reproduce sexually in the intestine of their hosts, and only parasites infecting a host that is already infected by another parasite of the opposite sex will get the chance to mate. Remaining the only infection in a host will inevitably lead to no reproduction, potentially creating allee effects that can decelerate population growth (Regoes et al., 2002; Berec et al., 2007). Therefore, aggregation within hosts is important for sexually reproducing parasites like Mytilicola and aggregation interference between competitors can influence population growth.
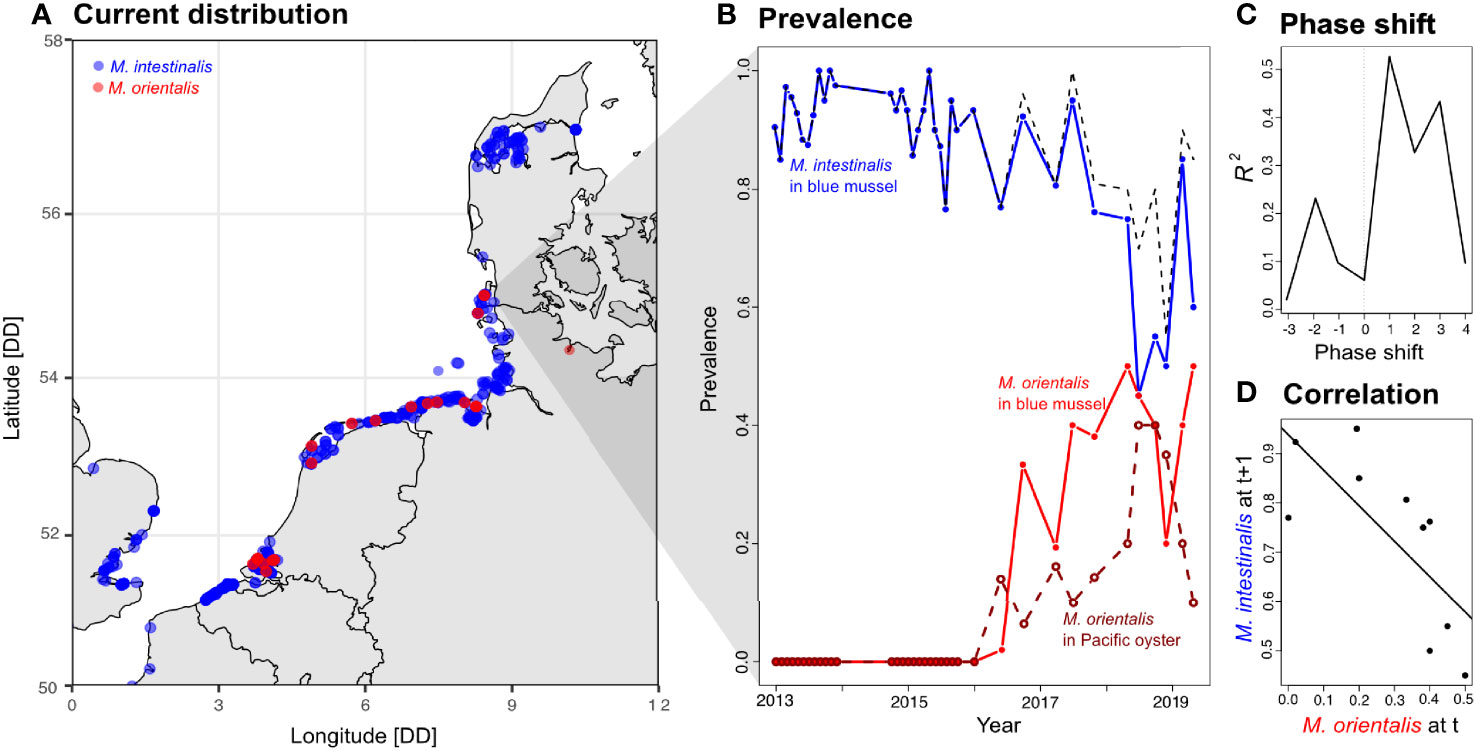
Figure 1 (A) Current distribution of Mytilicola intestinalis (blue) and M. orientalis (red) in the North Sea. Each dot represents an occurrence report and darker dots show multiple observations from the same site. (B) Prevalence of M. intestinalis in mussels (blue lines) and M. orientalis in mussels (red line) and oysters (dark red line, open circles) in Königshafen, Sylt, Germany covering the years 2013-2019. The black dashed line shows the overall prevalence of both species in mussel hosts. (C) Phase shift analysis including the years 2016 to 2019 when both parasite species were present. Observed prevalence data of M. intestinalis was shifted forward and backward along the time axis to calculate correlation coefficients between the prevalence curves of both parasites at time shifts ranging from -3 to +4 steps. The best correlation between prevalences was obtained when M. orientalis prevalence from time point t was correlated with M. intestinalis prevalence from time point t+1. (D) The resulting negative correlation (estimate = -0.718 ± 0.241, t = -2.985, p = 0.018) suggests that M. orientalis is driving M. intestinalis out of its niche in the mussel intestine.
In regions where both species already occur in sympatry (e.g. Texel in the southern Wadden Sea, the Netherlands) a decline of M. intestinalis from 60% in 1976 (Drinkwaard, 1993) to 13% in 2013 (Goedknegt, 2017) was observed coinciding with the arrival of M. orientalis. This might suggest that the generalist newcomer drove out the established specialist. However, it is uncertain if the generalist M. orientalis took over the specialist’s niche by direct competitive interactions, or whether abundance of M. intestinalis declined for other reasons that opened up its niche in the intestines of mussels.
Here, we now focus on the ongoing invasion of M. orientalis along the edge of its current distribution in the northern European Wadden Sea (Sylt, Germany, Figure 1A), where the recent arrival of M. orientalis in 2016 led to a delayed decline of M. intestinalis from very high (>90%) to considerably lower prevalences (<60%) over the time course of a few years (Figure 1B). Against this backdrop of a successful parasite invasion, we designed a series of experiments with the two ecologically relevant hosts M. edulis and C. gigas to reveal potential mechanisms that might give M. orientalis a competitive edge over M. intestinalis in the shared host, M. edulis. In detail, we assessed the competitive balance between the two species by testing superiority (i.e. competitive advantage in simultaneous infections) and priority effects (i.e. competitive advantage arising from the sequence of infections). To determine whether trade-offs in infectivity establish a cost of generalism, we investigated the infectivity of both parasites in both host species. We also asked whether recurrent spill-over of high fitness propagules could drive M. orientalis epidemiology by testing whether infectivity of M. orientalis was higher when originating from its reservoir hosts (Pacific oyster) or its newly acquired hosts (blue mussel). Finally, we tested if infection behavior in choice experiments is affected by the presence of previous con- and hetero-specific infections. Preference for previously infected individuals could then lead to aggregated distributions, which particularly important in initial stages of an invasion when the introduced species is still rare. Our experiments thus combine multiple mechanistic explanations that extend the scope for our understanding of how restricted and densely occupied niches can be invaded.
2 Materials and Methods
2.1 Monitoring the Ongoing Invasion of M. orientalis
To document the ongoing invasion of Mytilicola orientalis into the niche of M. intestinalis in the wild, we collected Pacific oysters Crassostrea gigas and blue mussels Mytilus edulis in the “oyssel reef” (a multilayered, mixed bed of mussels and oysters, sensu (Reise et al., 2017b)) in Königshafen, Sylt, Germany (55°02’17” N, 08°26’32” E) between 2013 and 2019. Mytilicola orientalis was absent from this location in 2008 (Elsner et al., 2011). For each time point, 20-30 individuals of both species were dissected under a stereomicroscope to count Mytilicola infections in the digestive tracts based on morphological species determination (Goedknegt et al., 2018b). We calculated prevalence (i.e. the proportion of hosts infected in a sample) and intensity (i.e. mean number of parasites in infected hosts within a sample) of both parasite species for each host species and time point.
To test the causal direction between the prevalences of both parasites, we performed a phase-shift analysis for the years between 2016 and 2019, when both parasites were present. Hereto, we shifted the observed prevalence data of M. intestinalis forward and backward along the time axis and calculated correlation coefficients between both prevalence curves at time shifts ranging from -3 to +4 sampling intervals. While this procedure cannot produce causality by itself, only past events can theoretically influence future observations. In other words, if the best correlations between the prevalence curves is observed at positive shift values (i.e. M. intestinalis prevalence is pushed into the future), current observations of M. orientalis correlate best with future observations of M. intestinalis suggesting that M. orientalis prevalence drives M. intestinalis prevalence. On the other hand, negative shift values giving the best correlation might rather suggest that M. intestinalis declines for unknown reasons and M. orientalis is taking advantage of the emptying niche in the intestine of mussels.
From the abundance data (i.e. the number of parasites found in each host) we also calculated parasite aggregation and the likelihood of finding a mate in an infected host. Aggregation was expressed as the variance-to-mean ratio calculated from the number of parasites found in each host for each sampling time point in all three host-parasite combinations (M. intestinalis in mussels, M. orientalis in mussels and M. orientalis in oysters). A variance-to-mean ratio of 1 indicates that data is distributed according to a Poisson distribution. Higher values indicate a higher degree of aggregation resulting from a clumped distribution with only few hosts carrying many parasites while many hosts show low infection intensities or no infection at all (Barbour and Pugliese, 2000). The likelihood of finding a mate, i.e. meeting a conspecific of the opposite sex, was calculated from binomial proportions of males and females assuming the simplest case of a 1:1 sex ratio based on the collected abundance data. In other words, if a parasite ends up in a host with only one other parasite, it has a 50% chance that the other specimen is of the opposite sex, whereas there is already a 96% chance to encounter the opposite sex when 5 parasites were already present.
2.2 Infection Experiments
2.2.1 General Infection Procedure
All experimental infections followed previously established protocols (Feis et al., 2016; Demann and Wegner, 2019). In short, uninfected mussel hosts (3.5-5 cm) from Sylt were obtained either by insecticide treatment (Blateau et al., 1992), only in simultaneous infections) or by sampling in populations with naturally low prevalence <10% (west shore breakers (Demann and Wegner, 2019)). For infection, we collected egg sacs from individual gravid female parasites, and we infected hosts in individual tanks by adding 24 copepodites. Copepodites not ingested by the host were counted after 24 h to calculate the exposure dose for each host. During the experiments mussels were kept individually in a flow-through system with algal food supply. We dissected hosts after 51-80 days and counted parasites in the intestine. Parasite species were identified by morphological characteristics or species-specific genetic polymorphisms (Goedknegt et al., 2018b), and infection rates were calculated as the proportion of successful infections from all ingested copepodites. We also included control groups in all experiments to check for background infections not originating from our controlled infections. Control mussels were treated the same way but were not exposed to copepodites. We only observed background infections in the preference experiment and removed those hosts as well as control hosts from infection rate analyses.
2.2.2 Simultaneous and Sequential Infections
To test the competitive balance between the two parasite species we assessed their infectivity in the shared host by conducting two co-infection experiments. Simultaneous infections were used to test superiority effects and sequential infections were used to establish priority effects (Figure 2). Both sets used a substitution design. In simultaneous infections we added 24 copepodites in the single species infections and mixes of 12 M. intestinalis and 12 M. orientalis copepodites for co-infections. This resulted in three experimental groups of 15 mussels for the simultaneous infections (n = 45). Infective copepodites of M. intestinalis were obtained from egg sacs collected in Oddewatt, Sylt, whereas we used egg sacs from Texel to obtain M. orientalis copepodites, as M. orientalis was not found on Sylt at the time both experiments were conducted (2014 and 2016, Figure 2). Infection rates were analyzed with binomial generalized linear mixed models (GLMM) using the R package lme4. The number of successful against failed infections from all ingested copepodites in each mussel was used as the binomial response. We recorded infection intensities for both parasites in co-infections separately and fitted “mussel ID” as a random intercept to account for the two infection intensities coming from the same host. We fitted “infection type” (single vs. co-infection) testing for differences in infection rates between single and co-infections, “parasite species” (M. intestinalis vs. M. orientalis) testing for differences in infection success between both parasite species and the interaction term describing whether differences between single and co-infections were dependent on the focal parasite species. Pairwise differences between treatment combinations were tested by Tukey-Posthoc test implemented in the multcomp package (Hothorn et al., 2008).
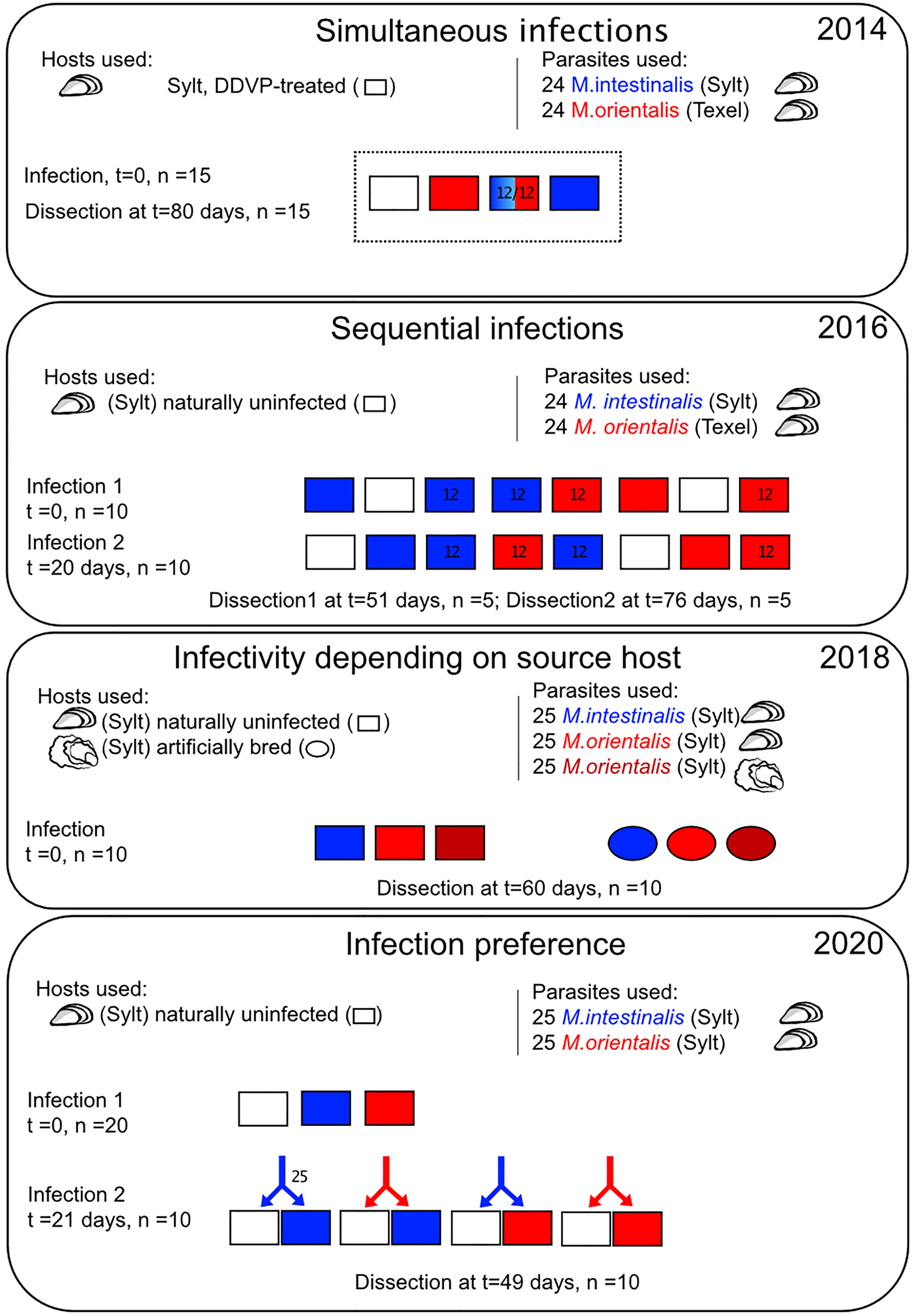
Figure 2 Schematic representation of the experimental infections. Each panel shows one experimental setup and the year in which the experiment was conducted. Boxes represent batches of experimental mussel and ovals batches of oyster hosts with blue coding for infections by M. intestinalis, red for infections by M. orientalis originating from mussels, and dark red for M. orientalis originating from oysters. Batch size is given by the n for each experiment. The geographic source of hosts and parasites is shown in brackets and source hosts are shown by pictograms.
For the sequential infections we used two rounds of infection separated by 20 days. To account for using different batches of copepodites between both infection rounds, we conducted single species infections with 24 copepodites of both parasites at both time points using a different set of hosts each time. The sequential infections consisted of mussels, which were either exposed twice (mixed infections) or once (single species infections) in all possible combinations resulting in 8 treatment groups of 10 mussels each (n = 80). We dissected the sequential infection experiment in two rounds of 5 individuals per treatment group to allow size differences between parasites to increase between first and second infections. Because there were no quantitative differences in infection rates between both time points, we analyzed the whole dataset together. We fitted a model similar to simultaneous infections for sequential infections. Next to the random factor of “mussel ID”, we fitted the fixed effects “parasite species” (M. intestinalis vs. M. orientalis), the modified three-level factor “infection type” (single infection in either the 1st or 2nd infection round vs. con-specific infection using the same species in both infection rounds vs. hetero-specific infection using the other parasite species in the 2nd round) and the additional factor “infection round” (1st infection round vs. 2nd infection round). These main effects tested for differences between the factor levels of each factor, while the three-way interaction between parasite species, infection type and infection round tested whether either parasite species reacted differently on the prior presence of the same or other parasite species.
2.2.3 Infectivity of Offspring Originating From Different Host Sources
This experiment was designed to test if M. orientalis is showing lower infection success in both of its host species consistent with a cost of generalism. Furthermore, we were also interested in whether mothers produce offspring of higher fitness/infectivity when they were isolated from oyster or mussel hosts, and whether the origin specifically influences infection rates in both host species. Therefore, we combined a source host treatment (M. intestinalis from mussels/M. orientalis from mussels/M. orientalis from oysters) with an infection host treatment (mussels/oysters) in a full factorial design, resulting in six treatment groups consisting of 10 hosts each (Figure 2).
Naturally uninfected mussels and Pacific oysters (1.5 year old lab bred broodstock) of shell lengths 4.0 – 4.6 cm were used as infection hosts. Mussels and oysters from the Oddewatt oysselreef also served as donors for M. intestinalis and M. orientalis copepodites. Infection rates were analyzed by binomial GLMs comparing the number of successful vs. failed infections as a function of “source host” (M. intestinalis vs. M. orientalis originating from mussels vs. M. orientalis originating from oysters) and “infection host” (mussel vs. oyster). The main effect “source host” tested if infection success depended on the host copepodites originated from, while the main effect “infection host” tested for differences between the host species used in the infection. The interaction between both terms would indicate that infection success was higher in specific combinations of source and infection hosts.
2.2.4 Parasite Infection Preference
This experiment aimed at testing whether Mytilicola species prefer to infect uninfected or previously infected hosts. Preference for hosts previously infected by conspecifics will lead to higher parasite aggregation within hosts, which can be equated to more opportunities to reproduce. We tested preference by offering a batch of 25 copepodites the choice of an experimentally infected and an uninfected host. To obtain infected hosts, we infected naturally uninfected mussels of shell lengths 2.8 – 4.2 cm with either 25 M. intestinalis or 25 M. orientalis copepodites. Each mussel from this group was then paired with a size-matched uninfected mussel and both were maintained in separate containers in the flow-through system until the actual preference infection three weeks later. The two matching hosts were then placed in a single container and were exposed to either 25 M. intestinalis or 25 M. orientalis copepodites. As the primary infections stretched out over several weeks, we always made sure that we used the same batch of copepodites for one preference infection for matching M. intestinalis and M. orientalis primary infections conducted at the same time (± 2 days). After 24 h, hosts were put back into their individual containers until the end of the experiment seven weeks after the primary infection (n = 45). Due to the paired design in each preference infection, we analyzed the data as the difference between infection rates of the host with primary infection minus the infection rate of the uninfected host. Positive values therefore reflect a preference for the infected host, while negative values show preference for the uninfected host. This preference score was then analyzed by a linear model, fitting the number of parasites from the first infection to control for dose dependency in the preference infection, the “parasite species” used for the second preference infection (M. intestinalis vs. M. orientalis) and a factor describing whether the “primary infection” used the same (con-specific) or the other parasite species (hetero-specific).
3 Results
3.1 Temporal Dynamics of Mytilicola Infections in the Field
During the period 2013 to 2016, M. intestinalis was the only Mytilicola species found in mussel intestines on the Oddewatt oysselreef (Figure 1B). With some seasonal fluctuations, M. intestinalis was found in high prevalence (between 77% and 100%) coupled to high mean infection intensities (between 4.2 and 10.4) reaching a maximal individual infection intensity of 42 parasites (Figure 1B). Multiple M. orientalis were found first in June 2016, when 2% of the mussels were infected. During the following years, M. orientalis increased in oysters as well as in mussels, while M. intestinalis prevalence steadily decreased and dipped below the prevalence of M. orientalis in mussels in 2018 (Figure 1B). Mean infection intensities of M. orientalis in mussels ranged between 1.5 and 3.1 and were somewhat lower than intensities in oyster, which ranged from 1.7 to 18.3 parasites per infected host. We found up to 46 M. orientalis specimen in a single oyster, but never found M. intestinalis in the 222 Pacific oysters we dissected. Our phase-shift analysis of prevalences in years where both parasite species were present (2016-2019) revealed that shifting M. intestinalis prevalences one sampling interval into the future (phase shift +1) gave the best correlation coefficient between both curves (Figure 1C). The significant negative correlation at this phase shift thus indicated that the prevalence of M. intestinalis was negatively affected by M. orientalis in mussels at the previous time point in field data (Figure 1D). This demonstrates the ongoing successful invasion of M. orientalis into the occupied niche of the mussel intestine and might indicate a competitive displacement of M. intestinalis.
The distribution of parasites found in each host also differed between the host-parasite combinations. Both parasites showed aggregated distributions with many uninfected and only few infected hosts with a high number of infections (Figure 3A). We nevertheless found significantly different degrees of aggregation (variance to mean ratio) between the different host-parasite combinations, with M. orientalis in oysters showing the highest degree of aggregation (Figure 3B). High aggregation values in M. intestinalis infecting mussels were only observed in the early years (2013-2016) with aggregation dropping substantially after M. orientalis invaded. The lower aggregation values in the later years also lead to a significantly decreased likelihood of encountering a mate in an infected host for M. intestinalis after M. orientalis invaded (Figure 3C). For M. orientalis, on the other hand, mating opportunities significantly increased in mussels and reached the highest values in oysters although this increase was only marginally significant (Figure 3C).
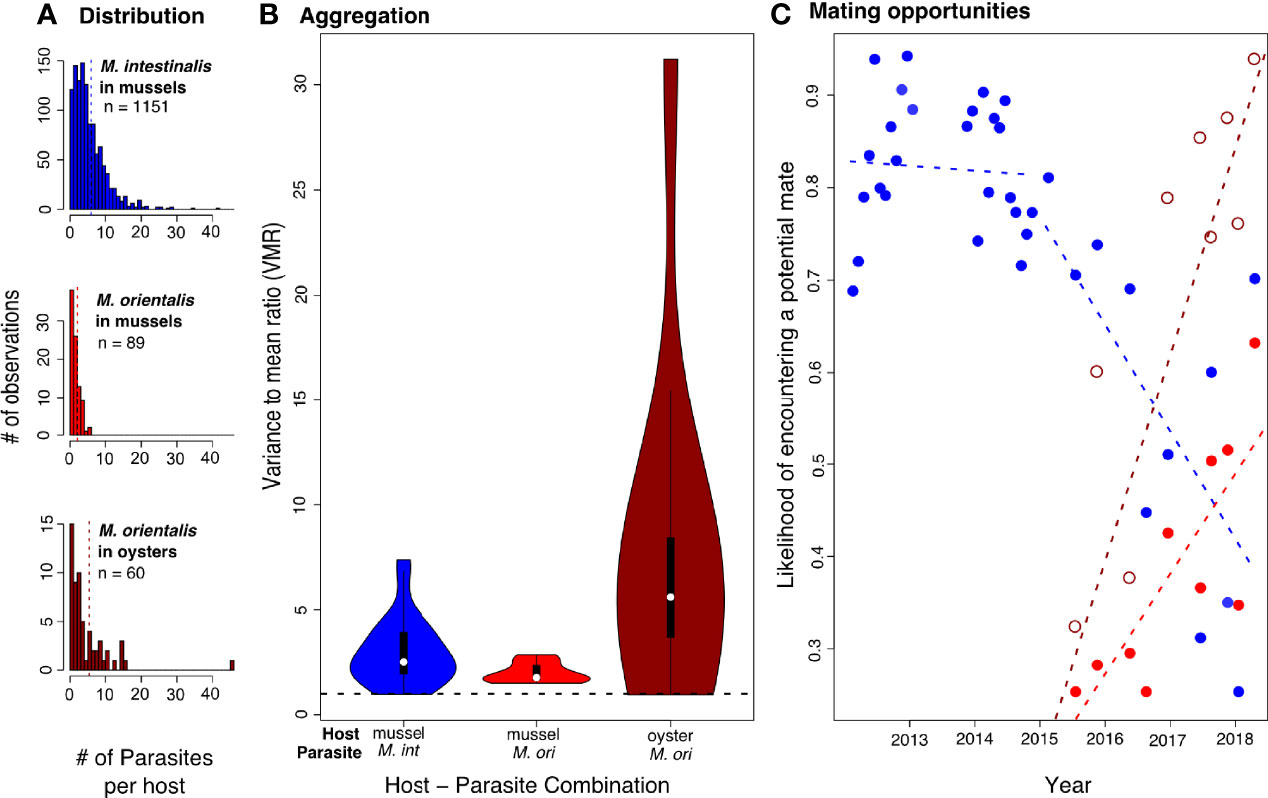
Figure 3 (A) Distribution of number of parasites per host for M. intestinalis (M. int, blue) and M. orientalis (M. ori, red, dark red) over all years of the field survey. Dashed vertical lines show the mean. (B) Aggregation of parasites expressed as the variance to mean ratio (VMR) for all years of the field survey. Variance and means were calculated from infection data of each parasite species found in all hosts dissected at each sampling time point. Violins show the range of the observed data with violin width representing the frequency distribution of values. Box and whiskers give the 50 and 95 percentile, and white dots within boxes show the median. Aggregation was significantly stronger for M. orientalis in oysters than for M. intestinalis in mussels (estimate = 4.570 ± 1.303, t = 3.507, p < 0.001) but did not differ between the parasite species when infecting mussels (estimate = -0.880 ± 1.303, t = -0.675, p = 0.502). (C) Likelihood of encountering a mate in an infected host on a given sampling event assuming the simplest case of a 1:1 sex ratio. In years without (2012-2016) Mytilicola orientalis mating opportunities for Mytilicola intestinalis (blue dots) showed no significant trend (R2 = 0.005, estimate = -0.000 ± 0.001, t = -0.34, p = 0.737). However, after the appearance of M. orientalis in 2016 a significant decline over the sampling period was observed (R2 = 0.421, estimate = -0.010 ± 0.004, t = -2.559, p = 0.031), while M. orientalis’ chances of encountering a potential mate in a given host increased in both hosts (mussel: R2 = 0.576, estimate = 0.008 ± 0.002, t = 3.497, p = 0.007; oyster: R2 = 0.257, estimate = 0.014 ± 0.007, t = 2.111, p = 0.064).
3.2 No Competitive Interaction in Simultaneous and Sequential Infections
In both simultaneous and sequential infection experiments, the specialist M. intestinalis had a higher infection rate than the generalist M. orientalis in the shared mussel host (Figure 4). During the simultaneous infection experiment eight mussels died (co-infection: 2, M. intestinalis: 2, M. orientalis: 4) and we observed a mean infection rate of 0.486 ± 0.054 for single species infections of M. intestinalis and 0.164 ± 0.047 for M. orientalis. No significant difference could be observed when comparing the single parasite infection rates to the parasite specific infection rates in the co-infection treatment of 0.506 ± 0.076 for M. intestinalis and 0.160 ± 0.043 for M. orientalis (Figure 4A and Table 1A), indicating that the presence of the other species did not influence infection success during the infection process. In sequential infections we observed a significant decline in infection rates between the first and second round of infections. This decline was mainly driven by low infection success of the second round of M. intestinalis infections, which was not observed in M. orientalis. In fact, pairwise comparisons revealed that the majority (7 out of 10) of significant differences were found between parasite species, while the only significant differences within parasite species were found between round 1 and round 2 infections with M. intestinalis. More importantly and resembling results obtained in simultaneous infections, we could not find significant differences between single infections and their corresponding infection rates in hetero-specific sequential infections (Figure 4B and Table 1B, M. intestinalis 1st infection round: single = 0.687 ± 0.049 vs. hetero-specific = 0.703 ± 0.079, 2nd infection: single = 0.348 ± 0.087 vs. hetero-specific = 0.404 ± 0.082, M. orientalis 1st infection: single = 0.423 ± 0.084 vs. hetero-specific = 0.215 ± 0.046, 2nd infection: single = 0.266 ± 0.072 vs. hetero-specific = 0.313 ± 0.099, all Tukey PostHoc comparisons p > 0.9). These results indicate that direct competition between M. intestinalis and M. orientalis did not affect infection success, neither by superiority in simultaneous infections, nor by priority in sequential infections where one species was given a head start over the other.
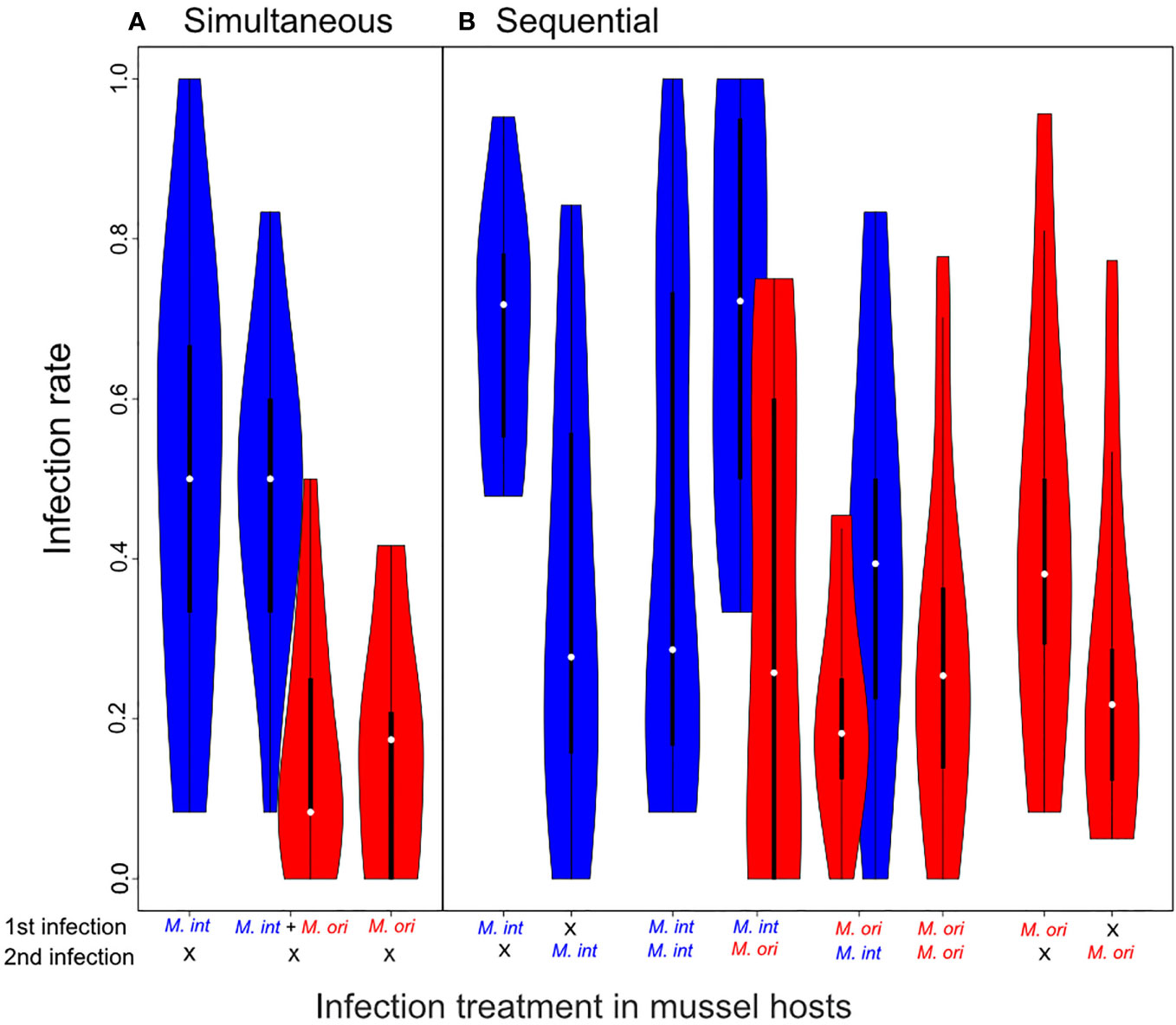
Figure 4 Infection rates of simultaneous infections and sequential infections. Violins show the range of the observed data with violin width representing the frequency distribution of values. Box and whiskers give the 50 and 95 percentile, and white dots within boxes show the median. (A) In simultaneous infections the only significant difference observed was the lower infection rates of Mytilicola orientalis (red) compared to Mytilicola intestinalis (blue), which was consistent between infection types (see Table 1A). Post-hoc tests between all groups showed the between-species comparisons were all significant (P < 0.001), whereas the within-species comparisons were not (p > 0.99). (B) Similarly, M. orientalis had significantly lower infection rates in sequential infections. Additionally, infection rates were on average lower in the second round of infection. This difference was however mainly driven by M. intestinalis infections, whereas there was no significant difference in M. orientalis infections (see Table 1B). Pairwise comparisons between single infections to the corresponding infection rates in hetero-specific infections showed no significant difference (all p -values > 0.9), indicating that between parasite interactions did not alter the outcome of infections. Infection rates resulting from the same species in both rounds were combined into one group.
3.3 M. orientalis From Oysters Have Higher Infectivity
Compared to M. intestinalis infection rates were significantly lower for M. orientalis when infecting it newly acquired host M. edulis (estimate = -2.680 ± 0.203, z = -13.192, p < 0.001) but also when infecting its reservoir host C. gigas (estimate = -2.558 ± 0.206, z = -12.409, p < 0.001). Even when combining infection rates for both hosts median infection rates of M. orientalis (0.082) was only 15% of the median infection rate of M. intestinalis (0.542), indicating that M. orientalis pays a cost of generalism (Figure 5). As expected from the absence of M. intestinalis infections in oysters in the field survey, we did not recover any successful infection of M. intestinalis in oyster hosts and can thus provide experimental confirmation that M. intestinalis is a specialist for blue mussels (Figure 5). For the host source dependence analysis, we therefore decided to drop this group from the analyses. Because we lacked the combination of M. intestinalis infection and oyster as a host, our full-factorial design collapsed and we decided to adopt an analysis strategy that first tested the infection treatment in all remaining mussel and oyster hosts. This confirmed our previous observations that M. orientalis had significantly lower infection success than M. intestinalis infections irrespective of their host origin (Figure 5; M. orientalis from mussel: estimate = -3.320 ± 0.244, z = -13.632, p < 0.001, M. orientalis from oyster: estimate = -2.152 ± 0.189, z = -11.419, p < 0.001). These non-overlapping estimates relative to M. intestinalis in mussels suggested that M. orientalis originating from oysters had higher infection success than M. orientalis from mussels. To formally test that we fitted a binomial GLM only containing M. orientalis infections with source (mussel vs. oyster) and infection hosts (mussel vs. oyster) as parameters. This confirmed that M. orientalis had significantly lower infection success when it originated from mussels than M. orientalis originating from oysters (Table 1C). This difference was consistent between infection hosts and did not depend on the combination of source and infection host (Table 1C), indicating that M. orientalis profits from reproducing in its principal oyster host, from where it can spill over offspring of relatively higher fitness into its newly acquired mussel host.
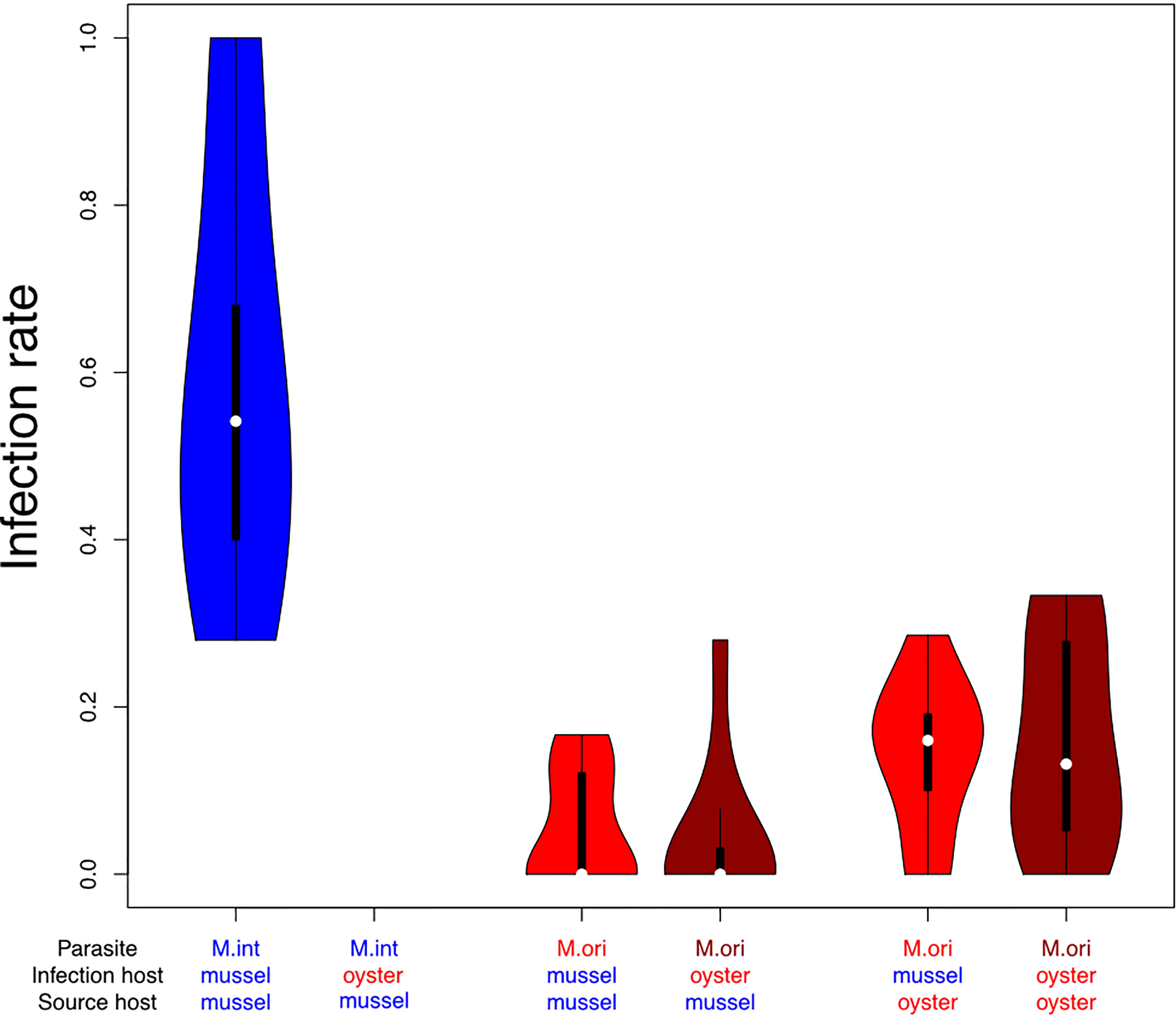
Figure 5 Infection rates of Mytilicola intestinalis (M. int, blue) and Mytilicola orientalis (M. ori, red, dark red) originating from mussel or oyster source hosts infecting mussels or oysters as infection hosts. Violins show the range of the observed data with violin width representing the frequency distribution of values. Box and whiskers give the 50 and 95 percentile, and white dots within boxes show the median. Infection rates of M. intestinalis were highest in mussels, whereas none of the experimental M. intestinalis infections were successful in oysters. Mytilicola orientalis had lower infection rates in both hosts combined than M. intestinalis in its only host. When originating from oysters M. orientalis showed a higher infectivity in both hosts, but they were still less successful than M. intestinalis in infecting mussels.
3.4 Both Parasites Differ in Their Aggregation Behavior
This experiment tested whether the observed aggregated distribution can be explained by M. orientalis preferentially infecting hosts with existing prior infections. While the primary infections showed the same trend of lower infection success of M. orientalis, we found significantly higher infection rates for M. orientalis than for M. intestinalis in the preference infection (Figure 6, F1,39 = 5.494, p = 0.024), which was independent of the primary infection (F1,39 = 0.347, p = 0.561) and the combination of primary and preference infection (F1,39 = 0.000, p = 0.991). The choice of host with and without primary infection, i.e. the infection preference, also differed between the two parasites irrespective of the overall infection rates. Here, M. orientalis significantly preferred hosts with primary infections over uninfected hosts in both con- and hetero-specific infections (Figure 6). In hetero-specific infections M. orientalis strongly preferred the infected host, whereas M. intestinalis seemed to avoid mussels with primary infections by M. orientalis (Figure 6). Consequently, we could detect a significant interaction effect between the parasite species used in the preference infection and whether the infected mussel was infected with a con- or a hetero-specific parasite (Figure 5). We found similar overall infection rates between hetero- and conspecific infections compatible with infection preference behavior rather than competitive exclusion after infection. Such active infection choice behavior leads to high aggregation in of M. orientalis, but also the decline in mating opportunities for M. intestinalis resulting from a lack of con-specific preference and avoidance of mussels with a primary M. orientalis infection (Figure 3C and Figure 6).
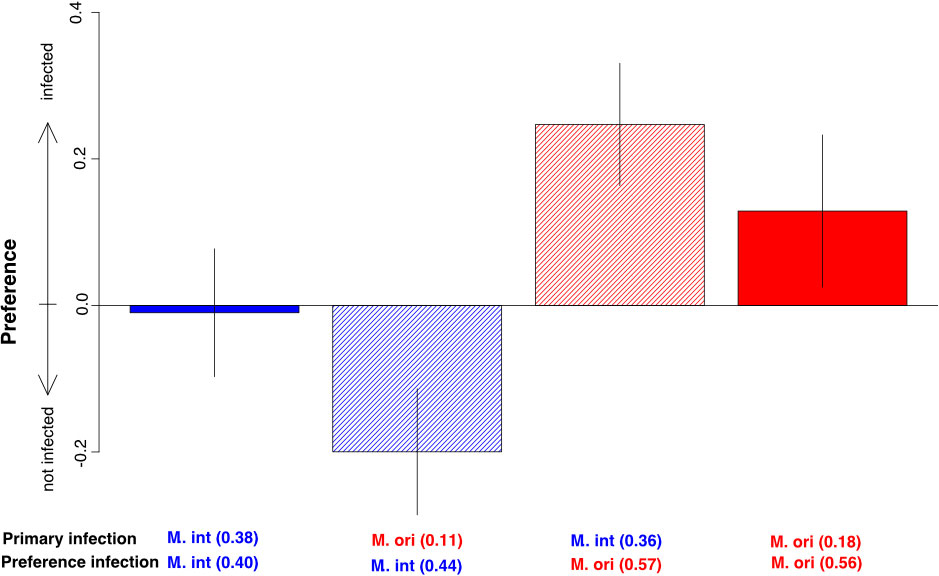
Figure 6 Preference of Mytilicola intestinalis (M. int, blue) and Mytilicola orientalis (M. ori, red) for hosts with or without a primary infection in host choice experiment. Preference is expressed as the difference of infection rate in hosts with primary infection minus the infection rate of the host without primary infection. Numbers in the x-axis labels show the overall infection rate of the respective parasite species in both infection rounds. Conspecific combinations of primary and preference infections have solid bars and hetero-specific combinations are hashed. The positive values for M. orientalis show a general preference for host with primary infections in both con- and hetero-specific preference infections (estimate = 0.539 ± 0.140, t = 3.850, p < 0.001). Hetero-specific showed significantly different infection preferences (estimate = -0.475 ± 0.207, t = -2.290, p = 0.028) indicating that M. intestinalis rather showed avoidance of mussels infected with M. orientalis and ambivalence towards mussels infected with M. intestinalis, while M. orientalis showed a strong preference for mussels previously infected with M. intestinalis.
4 Discussion
How generalist parasites can coexist with specialists in the same niche in the face of costs to generalism remains an unsolved question (Visher and Boots, 2020). Even more so, it is an enigma how a generalist can invade a niche that is densely occupied by an established specialist. Empirical systems to address these questions are rare, especially for non-microbial parasites. By focussing on the successful and ongoing invasion of the parasitic copepod Mytilicola orientalis into the niche of its specialist congener Mytilicola intestinalis, we could now identify mechanisms of establishment in the densely occupied intestine of blue mussels Mytilus edulis. Both parasites are morphologically and ecologically very similar, making competitive interactions likely (Fukami, 2015). Nevertheless, we have shown that in the absence of direct competition, indirect effects that alter the invader’s epidemiology and interfere with host choice of the established species can contribute to explaining the invasion success mirrored in the ongoing invasion observed in the field. A phase shift analysis on our epidemiological field data showed that the decreasing prevalence of M. intestinalis was best predicted by the preceding prevalence of M. orientalis, suggesting a causal implication of M. orientalis in the decline of M. intestinalis during the initial stages of its invasion. In situ observations of parasite interactions in the wild are rare in general (Telfer et al., 2008; Fenton et al., 2010; Hellard et al., 2015), but observations of one species displacing its established congener in a natural setting offer an excellent opportunity to study the onset of competition. Furthermore, other studies investigating competitive interactions between parasites use systems where interactions have evolved over evolutionary time (Lello et al., 2004) - sometimes even across speciation events (Dawson et al., 2000; Johnson et al., 2009). In the Mytilicola system used here, a biological invasion created novel competitors, and thus allowed us to catch a glimpse of the competitive landscapes during initial phases of such interactions.
4.1 Direct Competition Cannot Explain the Success of the Newcomer
Over all infection experiments the mussel specialist M. intestinalis was more successful at infecting its only host than M. orientalis at infecting both of its hosts. Such differences in infection success could be caused by closed encounter or compatibility filters, and observational field data can in many cases not distinguish between encounter and compatibility filters as long as one of the filters remains closed (Kuris et al., 2007). With our experimental design we could however ensure that we had an open encounter filter. Therefore, differential infection success between both parasite species must rely on different compatibilities or competition between both species. The infections testing direct competitive interactions during the infection process revealed no competitive interaction between both species (i.e. single-species infections showed the same infection rates than co-infections). We had to use allopatric sources of M. orientalis for the simultaneous and sequential infection experiments, which were carried out before M. orientalis invaded locally. Our first experiment using the identical source population for both parasite species (i.e. the host source experiment) as well as the primary infections in the preference experiment did however confirm these results with sympatric M. orientalis, suggesting differences between species rather than populations. Infection rates of M. orientalis were only higher than those of M. intestinalis in the preference infection of our last infection choice experiment. This difference partly arose from comparatively low infection rates of M. intestinalis. Together with the difficulty of finding gravid M. intestinalis females in 2020 (Theising & Wegner, personal observation), variation in infection rates might suggest a decline in population mean fitness of M. intestinalis. This effect could further contribute to the low abundance observed in the field. On the other hand, M. intestinalis was shown to adapt quickly to its local host population along separate evolutionary trajectories (Feis et al., 2016; Feis et al., 2018), indicating that there is substantial evolutionary potential, which might also be found in M. orientalis. Although co-infection can select for enhanced competitive abilities between parasite species (de Roode et al., 2005), it seems unlikely that M. orientalis shifted the infectiveness scale over a time period of a few generations. The low infection success of M. intestinalis in the second round of the sequential infection experiment and the high infection success of M. orientalis in the preference infection rather suggest that variation in infection success can be high in both species, and further experiments are needed to track the ongoing evolution of infectivity and competitive abilities in this system.
Overall, our results suggest that the decline of M. intestinalis did not occur because of direct competition between the two parasites in their shared mussel host. In fact, the only indication for some interference between both parasites was the lower infection rate of M. orientalis followed by M. intestinalis in sequential infections (Figure 3B). While the observed difference was not statistically significant, it may nevertheless indicate that prior M. orientalis infections might get aborted at a higher rate when followed by M. intestinalis infections. This would put M. orientalis at a competitive disadvantage, since any kind of competition is predicted to prevent the establishment of rare parasites (Quigley et al., 2018). Other studies also found such antagonistic priority effects in sequential infections (Hoverman et al., 2013), but also all other outcomes ranging from no interaction (Zilio and Koella, 2020) to superiority in simultaneous infections (Okabe et al., 2012) or facilitative interaction in sequential infections (Halliday et al., 2020) have been observed. The variety of these outcomes suggests that with extended contact over evolutionary time different co-evolutionary trajectories can be observed depending on the specific ecological conditions encountered during contact. In our case, the absence of direct competition between both parasite species surely helped the initial establishment of M. orientalis in its new mussel host.
4.2 Indirect Effects on Epidemiology and Host Choice Amplify Each Other
To fully explain M. orientalis’ invasion success into the occupied niche, alternative mechanisms are needed. In direct comparison M. orientalis already displayed higher resilience to changing environmental conditions (Brenner et al., 2019) indicating that this species has also evolved higher tolerance to abiotic conditions. However, tolerance to external environmental conditions mainly affects the free-living transmission stage, while within host environments can be considered as habitats that are buffered from abiotic fluctuations. Therefore, we focused our investigations on host-related factors and our first hypothesis was that the origin from the alternative hosts of M. orientalis parasite produces offspring of different quality. We could confirm this hypothesis, since infections originating from egg sacs collected from oyster hosts showed higher infection success than offspring from mussel hosts. This suggests that maturation and reproduction in the principal oyster host generates offspring of higher fitness that feeds back positively on the epidemiology in the mussel host. If a high proportion of mussel infections originate from oyster hosts, continuous spillover will provide a surplus in infective propagules able to infect the newly acquired host (Goedknegt et al., 2016b), thereby increasing overall transmission success. Indeed, only in the very first stages of the invasion M. orientalis prevalences were higher in oysters than in mussels (Figure 1B). Infection intensities, on the other hand, always remained higher in oysters, suggesting that an increasing number of mussels got infected from oyster reservoir hosts where high infection intensities produced a large number of infective offspring with higher transmission probability (Figure 5). Furthermore, dilution effects, i.e. the loss of infective stages by ending up in the wrong host species (Thieltges et al., 2008), are asymmetric between both Mytilicola species and their hosts (Goedknegt et al., 2019). The lower transmissibility of M. orientalis originating from mussels can be considered as a minor dilution for M. orientalis epidemiology. However, the incompatibility of M. intestinalis and oyster hosts indicates that M. intestinalis can lose many infective stages in oysters due to a dilution effect caused by a closed compatibility filter (Kuris et al., 2007). Dilution of M. intestinalis is further amplified by the modification of host preference depending on prior infections. Mytilicola intestinalis copepodites avoided mussels infected with M. orientalis, while M. orientalis copepodites preferentially infect mussels with prior infections (especially infection with M. intestinalis, Figure 6). This non-competitive priority effect generates a positive feedback where mussels with prior M. intestinalis infections are over-proportionally infected by M. orientalis originating from oyster reservoir hosts. Reservoir hosts have been shown to have strong effects on parasite epidemiology alone (Al-Shorbaji et al., 2016), and the growing numbers of feral oysters in the Waddensea (Reise et al., 2017a) will most certainly also lead to more M. orientalis infections in mussels. M. orientalis infections in mussels can then prevent M. intestinalis transmission potentially reducing M. intestinalis population growth. This behavior was also reflected in the field data where the overall infection prevalence was only additive when M. intestinalis was comparatively rare (i.e. late 2018 in Figure 1B). The combination of transmission stages with higher infectivity coming from oysters and the interference of M. intestinalis host finding by M. orientalis, makes oysters a diluter of M. intestinalis epidemiology and an amplifier of M. orientalis epidemiology at the same time. This modification of epidemiology is not accessible to M. instestinalis and can therefore largely contribute to invasion success of M. orientalis. Interestingly, the overall prevalence of both parasites combined in mussel hosts also dropped since M. orientalis invaded (Figure 1B). Since infection by both parasites leads to similar energetic costs and lowered body condition of the mussel host (Feis et al., 2016; Goedknegt et al., 2018a), mussels might have actually profited from the invasion of M. orientalis due to lower infection rates.
The population decline of M. intestinalis can then be further accelerated by Allee effects caused by lack of mating opportunities (Regoes et al., 2002; Berec et al., 2007). Aggregation in sexual parasites can be directly translated into potential mating opportunity, and low infection intensities will create Allee effects due to the lack of a suitable partner of opposite sex. In the context of mate limitation, the ability to detect and preferentially infect hosts with pre-existing infections can be adaptive and the observed aggregation patterns might suggest that M. orientalis can actively aggregate in hosts, whereas M. intestinalis suffered in terms of reproductive success due to the lack of aggregation ability. Even though the realized reproductive success can vary widely around the probability of encountering the opposite sex, this number sets a lower boundary that needs to be overcome. The active aggregation by preferential infection of hosts with prior infections observed for M. orientalis can circumvent such Allee effects, and is also needed for this species given its low prevalence in its principle oyster host. Mytilicola intestinalis, on the other hand, has experienced high prevalences (>90% during the reproductive season) making active choice for already infected hosts obsolete. With the onset of declining prevalences, mating opportunities then got increasingly rare, especially with the avoidance of hosts already infected with M. orientalis. Together, Allee effects will be amplified, and could thus further explain the accelerating decline of M. intestinalis.
4.3 Conclusion
The driving force behind the advance of M. orientalis at the detriment of M. intestinalis is not the direct competition between the species. Rather, recurrent spill-over (Goedknegt et al., 2016b) of high fitness propagules created positive effects on M. orientalis epidemiology, and the modification of hosts choice behavior can further accelerate the decline of M. intestinalis. Both aspects can act synergistically to open the gate for dominance of M. orientalis over M. intestinalis. These mechanisms can be considered as indirect effects that help a newly introduced parasite to overcome its low compatibility and take over the niche of the established specialist without direct competition. Indirect effects in parasite interactions have so far mainly focused on host immunity (Graham, 2008; Dunn et al., 2012; Demann and Wegner, 2019), but our data shows that other mechanisms can mediate indirect interactions outside of host immunity. Such mechanistically hidden indirect parasite interactions need to be addressed in a wider array of species to understand niche breadth evolution and invasion success.
Data Availability Statement
The datasets presented in this study can be found in the github repository: https://github.com/mathiaswegner-AWI/OccupiedNicheInvasion.git.
Author Contributions
Field data was collected by MF, LG, LR, FD, and KW, and analyses by KW. MF and KW designed and carried out the concurrent infection experiment. LG and KW designed and carried out the sequential experiment. LR and KW designed and carried out the parental effects experiment. FT and KW designed and carried out the aggregation experiment. MF, LG, LR, FT, and KW analyses the data from the experiments. MF and KW wrote the original draft of the manuscript, and all authors helped with editing. All authors contributed to the article and approved the submitted version.
Funding
This work was supported by the German Research Foundation (DFG) Special Program 1399 on host-parasite coevolution (grant number We4641/1-2 to KW).
Conflict of Interest
The authors declare that the research was conducted in the absence of any commercial or financial relationships that could be construed as a potential conflict of interest.
Publisher’s Note
All claims expressed in this article are solely those of the authors and do not necessarily represent those of their affiliated organizations, or those of the publisher, the editors and the reviewers. Any product that may be evaluated in this article, or claim that may be made by its manufacturer, is not guaranteed or endorsed by the publisher.
Acknowledgments
The authors would like to thank Anouk Goedknegt and David Thieltges for their help with mussel collections on Texel, Kaibil Escobar Wolf and Timm Kress for help with building the experimental setup, Sonja Anslinger and Eike Petersen for technical support in the lab, and Karsten Reise for helpful comments on the draft of this manuscript.
References
Al-Shorbaji F., Roche B., Gozlan R., Britton R., Andreou D. (2016). The Consequences of Reservoir Host Eradication on Disease Epidemiology in Animal Communities. Emerg. Microbes Infect. 5, 1–12. doi: 10.1038/emi.2016.46
Anton A., Geraldi N. R., Lovelock C. E., Apostolaki E. T., Bennett S., Cebrian J., et al. (2019). Global Ecological Impacts of Marine Exotic Species. Nat. Ecol. Evol. 3 (5), 787–800. doi: 10.1038/s41559-019-0851-0
Bailey S. A., Brown L., Campbell M. L., Canning-Clode J., Carlton J. T., Castro N., et al. (2020). Trends in the Detection of Aquatic Non-Indigenous Species Across Global Marine, Estuarine and Freshwater Ecosystems: A 50-Year Perspective. Diversity Distributions 26 (12), 1780–1797. doi: 10.1111/ddi.13167
Barbour A. D., Pugliese A. (2000). On the Variance-to-Mean Ratio in Models of Parasite Distributions. Adv. Appl. Probability 32 (3), 701–719. doi: 10.1239/aap/1013540240
Berec L., Angulo E., Courchamp F. (2007). Multiple Allee Effects and Population Management. Trends Ecol. Evol. 22 (4), 185–191. doi: 10.1016/j.tree.2006.12.002
Bignell J. P., Dodge M. J., Feist S. W., Lyons B., Martin P. D., Taylor N. G. H., et al. (2008). Mussel Histopathology: Effects of Season, Disease and Species. Aquat. Biol. 2, 1–15. doi: 10.3354/ab00031
Blakeslee A. M. H., Manousaki T., Vasileiadou K., Tepolt C. K. (2020). An Evolutionary Perspective on Marine Invasions. Evol. Appl. 13 (3), 479–485. doi: 10.1111/eva.12906
Blateau D., Lecoguic Y., Mialhe E., Grizel H. (1992). Mussel (Mytilus Edulis) Treatment Against the Red Copepod Mytilicola Intestinalis. Aquaculture 107 (2-3), 165–169. doi: 10.1016/0044-8486(92)90062-p
Bouwmeester M. M., Goedknegt M. A., Poulin R., Thieltges D. W. (2021). Collateral Diseases: Aquaculture Impacts on Wildlife Infections. J. Appl. Ecol. 58 (3), 453–464. doi: 10.1111/1365-2664.13775
Brenner M., Schulze J., Fischer J., Wegner K. M. (2019). First Record of the Parasitic Copepod (Mytilicola Orientalis Mor) in Blue Mussels (Mytilus Spp.) of the Baltic Sea. BioInvasions Records 8 (3), 623–632. doi: 10.3391/bir.2019.8.3.19
Cuthbert R. N., Pattison Z., Taylor N. G., Verbrugge L., Diagne C., Ahmed D. A., et al. (2021). Global Economic Costs of Aquatic Invasive Alien Species. Sci. Total Environ. 775, 145238. doi: 10.1016/j.scitotenv.2021.145238
Dawson L. H. J., Renaud F., Guegan J. F., de Meeus T. (2000). Experimental Evidence of Asymmetrical Competition Between Two Species of Parasitic Copepods. Proc. R. Soc. B Biol. Sci. 267 (1456), 1973–1978. doi: 10.1098/rspb.2000.1238
Demann F., Wegner K. M. (2019). Infection by Invasive Parasites Increases Susceptibility of Native Hosts to Secondary Infection via Modulation of Cellular Immunity. J. Anim. Ecol. 88, 427–438. doi: 10.1111/1365-2656.12939
de Roode J. C., Pansini R., Cheesman S. J., Helinski M. E. H., Huijben S., Wargo A. R., et al. (2005). Virulence and Competitive Ability in Genetically Diverse Malaria Infections. Proc. Natl. Acad. Sci. U. S. A. 102 (21), 7624–7628. doi: 10.1073/pnas.0500078102
Drinkwaard A. C. (1993). Het gevaar van de mosselparasiet voor de mosselkweek. Unpublished Report, commissioned by Productschap voor Vis en Visproducten, the Netherlands.
Dunn A. M., Torchin M. E., Hatcher M. J., Kotanen P. M., Blumenthal D. M., Byers J. E., et al. (2012). Indirect Effects of Parasites in Invasions. Funct. Ecol. 26 (6), 1262–1274. doi: 10.1111/j.1365-2435.2012.02041.x
Elsner N. O., Jacobsen S., Thieltges D. W., Reise K. (2011). Alien Parasitic Copepods in Mussels and Oysters of the Wadden Sea. Helgoland Marine Res. 65 (3), 299–307. doi: 10.1007/s10152-010-0223-2
Feis M. E., Goedknegt M. A., Arzul I., Chenuil A., Boon O. D., Gottschalck L., et al. (2019). Global Invasion Genetics of Two Parasitic Copepods Infecting Marine Bivalves. Sci. Rep. 9 (1), 12730. doi: 10.1038/s41598-019-48928-1
Feis M. E., Goedknegt M. A., Thieltges D. W., Buschbaum C., Wegner K. M. (2016). Biological Invasions and Host-Parasite Coevolution: Different Coevolutionary Trajectories Along Separate Parasite Invasion Fronts. Zoology 119, 366–374. doi: 10.1016/j.zool.2016.05.012
Feis M. E., John U., Lokmer A., Luttikhuizen P. C., Wegner K. M. (2018). Dual Transcriptomics Reveals Co-Evolutionary Mechanisms of Intestinal Parasite Infections in Blue Mussels Mytilus Edulis. Mol. Ecol. 27 (6), 1505–1519. doi: 10.1111/mec.14541
Fenton A., Viney M. E., Lello J. (2010). Detecting Interspecific Macroparasite Interactions From Ecological Data: Patterns and Process. Ecol. Lett. 13 (5), 606–615. doi: 10.1111/j.1461-0248.2010.01458.x
Figueras A. J., Jardon C. F., Caldas J. R. (1991). Diseases and Parasites of Rafted Mussels (Mytilus Galloprovincialis Lamark) - Prliminary Results. Aquaculture 99 (1-2), 17–33. doi: 10.1016/0044-8486(91)90285-f
Frankel V. M., Hendry A. P., Rolshausen G., Torchin M. E. (2015). Host Preference of an Introduced 'Generalist' Parasite for a Non-Native Host. Int. J. Parasitol. 45 (11), 703–709. doi: 10.1016/j.ijpara.2015.03.012
Fukami T. (2015). Historical Contingency in Community Assembly: Integrating Niches, Species Pools, and Priority Effects. Annu. Rev. Ecol. Evol. System. 46 (1), 1–23. doi: 10.1146/annurev-ecolsys-110411-160340
Goedknegt M. A., Bedolfe S., Drent J., van der Meer J., Thieltges D. W. (2018a). Impact of the Invasive Parasitic Copepod Mytilicola Orientalis on Native Blue Mussels Mytilus Edulis in the Western European Wadden Sea. Marine Biol. Res. 14 (5), 497–507. doi: 10.1080/17451000.2018.1442579
Goedknegt M. A., Feis M. E., Wegner K. M., Luttikhuizen P. C., Buschbaum C., Camphuysen K., et al. (2016a). Parasites and Marine Invasions: Ecological and Evolutionary Perspectives. J. Sea Res. 113, 11–27. doi: 10.1016/j.seares.2015.12.003
Goedknegt M. A. (2017). Pacific Oysters and Parasites: Species Invasions and their Impact on Parasite Host Interactions. Phd Thesis VU Amsterdam.
Goedknegt M. A., Nauta R., Markovic M., Buschbaum C., Folmer E. O., Luttikhuizen P. C., et al. (2019). How Invasive Oysters can Affect Parasite Infection Patterns in Native Mussels on a Large Spatial Scale. Oecologia. 90, 99–113. doi: 10.1007/s00442-019-04408-x
Goedknegt M. A., Schuster A.-K., Buschbaum C., Gergs R., Jung A. S., Luttikhuizen P. C., et al. (2016b). Spillover But No Spillback of Two Invasive Parasitic Copepods From Invasive Pacific Oysters (Crassostrea Gigas) to Native Bivalve Hosts. Biol. Invasions 19, 365–379. doi: 10.1007/s10530-016-1285-0
Goedknegt M. A., Thieltges D. W., van der Meer J., Wegner K. M., Luttikhuizen P. C. (2018b). Cryptic Invasion of a Parasitic Copepod: Compromised Identification When Morphologically Similar Invaders Co-Occur in Invaded Ecosystems. PloS One 13 (3), e0193354. doi: 10.1371/journal.pone.0193354
Graham A. L. (2008). Ecological Rules Governing Helminth-Microparasite Coinfection. Proc. Natl. Acad. Sci. U. S. A. 105 (2), 566–570. doi: 10.1073/pnas.0707221105
Halliday F. W., Penczykowski R. M., Barres B., Eck J. L., Numminen E., Laine A. L. (2020). Facilitative Priority Effects Drive Parasite Assembly Under Coinfection. Nat. Ecol. Evol. 4 (11), 1510–1521. doi: 10.1038/s41559-020-01289-9
Hellard E., Fouchet D., Vavre F., Pontier D. (2015). Parasite-Parasite Interactions in the Wild: How To Detect Them? Trends Parasitol. 31 (12), 640–652. doi: 10.1016/j.pt.2015.07.005
Holmes J. C. (1973). Site Selection by Parasitic Helminths - Interspecific Interactions, Site Segregation, and Their Importance to Development of Helminth Communities. Can. J. Zool. 51 (3), 333–347. doi: 10.1139/z73-047
Hothorn T., Bretz F., Westfall P. (2008). Simultaneous Inference in General Parametric Models. Biometrical J. 50 (3), 346–363. doi: 10.1002/bimj.200810425
Hoverman J. T., Hoye B. J., Johnson P. T. J. (2013). Does Timing Matter? How Priority Effects Influence the Outcome of Parasite Interactions Within Hosts. Oecologia 173 (4), 1471–1480. doi: 10.1007/s00442-013-2692-x
Johnson K. P., Malenke J. R., Clayton D. H. (2009). Competition Promotes the Evolution of Host Generalists in Obligate Parasites. Proc. Biol. Sci. 276 (1675), 3921–3926. doi: 10.1098/rspb.2009.1174
Kuris A. M., Goddard J. H., Torchin M. E., Murphy N., Gurney R., Lafferty K. D. (2007). An Experimental Evaluation of Host Specificity: The Role of Encounter and Compatibility Filters for a Rhizocephalan Parasite of Crabs. Int. J. Parasitol. 37 (5), 539–545. doi: 10.1016/j.ijpara.2006.12.003
Lello J., Boag B., Fenton A., Stevenson I. R., Hudson P. J. (2004). Competition and Mutualism Among the Gut Helminths of a Mammalian Host. Nature 428 (6985), 840–844. doi: 10.1038/nature02490
Leung T. L. F., Poulin R. (2011). Intra-Host Competition Between Co-Infecting Digeneans Within a Bivalve Second Intermediate Host: Dominance by Priority-Effect or Taking Advantage of Others? Int. J. Parasitol. 41 (3-4), 449–454. doi: 10.1016/j.ijpara.2010.11.004
Mideo N. (2009). Parasite Adaptations to Within-Host Competition. Trends Parasitol. 25 (6), 261–268. doi: 10.1016/j.pt.2009.03.001
Okabe K., Masuya H., Kanzaki N., Taki H. (2012). Regional Collapse of Symbiotic Specificity Between Lucanid Beetles and Canestriniid Mites. Naturwissenschaften 99, 959–965. doi: 10.1007/s00114-012-0979-0
Quigley B. J. Z., Brown S. P., Leggett H. C., Scanlan P. D., Buckling A. (2018). Within-Host Interference Competition can Prevent Invasion of Rare Parasites. Parasitology 145 (6), 770–774. doi: 10.1017/s003118201700052x
Regoes R. R., Ebert D., Bonhoeffer S. (2002). Dose-Dependent Infection Rates of Parasites Produce the Allee Effect in Epidemiology. Proc. Biol. Sci. 269 (1488), 271–279. doi: 10.1098/rspb.2001.1816
Reise K., Buschbaum C., Büttger H., Rick J., Wegner K. M. (2017a). Invasion Trajectory of Pacific Oysters in the Northern Wadden Sea. Marine Biol. 164 (4), 68. doi: 10.1007/s00227-017-3104-2
Reise K., Buschbaum C., Büttger H., Wegner K. M. (2017b). Invading Oysters and Native Mussels: From Hostile Takeover to Compatible Bedfellows. Ecosphere 8 (9), e01949. doi: 10.1002/ecs2.1949
Santarem M. M., Robledo J. A. F., Figueras A. (1994). Seasonal Changes in Hemocytes and Serum Defense Factors in the Blue Mussel Mytilus Galloprovincialis. Dis. Aquat. Organisms 18 (3), 217–222. doi: 10.3354/dao018217
Stock J. H. (1993). Copepoda (Crustacea) Associated With Commercial and Noncommercial Bivalvia in the East Scheldt, The Netherlands. Bijdragen Tot Dierkunde 63 (1), 61–64. doi: 10.1163/26660644-06301004
Telfer S., Birtles R., Bennett M., Lambin X., Paterson S., Begon M. (2008). Parasite Interactions in Natural Populations: Insights From Longitudinal Data. Parasitology 135 (7), 767–781. doi: 10.1017/s0031182008000395
Tepolt C. K., Darling J. A., Blakeslee A. M. H., Fowler A. E., Torchin M. E., Miller A. W., et al. (2020). Recent Introductions Reveal Differential Susceptibility to Parasitism Across an Evolutionary Mosaic. Evol. Appl. 13 (3), 545–558. doi: 10.1111/eva.12865
Thieltges D. W., Reise K., Prinz K., Jensen K. T. (2008). Invaders Interfere With Native Parasite–Host Interactions. Biol. Invasions 11 (6), 1421–1429. doi: 10.1007/s10530-008-9350-y
Visher E., Boots M. (2020). The Problem of Mediocre Generalists: Population Genetics and Eco Evolutionary Perspectives on Host Breadth Evolution in Pathogens. Proc Biol Sci 287 (1933), 20201230. doi: 10.1098/rspb.2020.1230
Watermann B., Thomsen A., Kolodzey H., Daehne B., Meemken M., Pijanowska U., et al. (2008). Histopathological Lesions of Molluscs in the Harbour of Norderney, Lower Saxony, North Sea (Germany). Helgoland Marine Res. 62 (2), 167–175. doi: 10.1007/s10152-008-0105-z
Keywords: biological invasion, epidemiology, host choice, macroparasite, mollusk, spill-over, Wadden Sea
Citation: Feis ME, Gottschalck L, Ruf LC, Theising F, Demann F and Wegner KM (2022) Invading the Occupied Niche: How a Parasitic Copepod of Introduced Oysters Can Expel a Congener From Native Mussels. Front. Mar. Sci. 9:915841. doi: 10.3389/fmars.2022.915841
Received: 08 April 2022; Accepted: 06 May 2022;
Published: 06 June 2022.
Edited by:
Lorenzo Mari, Politecnico di Milano, ItalyReviewed by:
Katie E. Costello, International Union for Conservation of Nature, United KingdomSarah Annalise Gignoux-Wolfsohn, Smithsonian Environmental Research Center (SI), United States
Copyright © 2022 Feis, Gottschalck, Ruf, Theising, Demann and Wegner. This is an open-access article distributed under the terms of the Creative Commons Attribution License (CC BY). The use, distribution or reproduction in other forums is permitted, provided the original author(s) and the copyright owner(s) are credited and that the original publication in this journal is cited, in accordance with accepted academic practice. No use, distribution or reproduction is permitted which does not comply with these terms.
*Correspondence: K. Mathias Wegner, mathias.wegner@awi.de