- 1Key Laboratory of Health Risk Factors for Seafood of Zhejiang Province, College of Food Science and Pharmacy, Zhejiang Ocean University, Zhoushan, China
- 2National and Provincial Joint Laboratory of Exploration and Utilization of Marine Aquatic Genetic Resources, National Engineering Research Center of Marine Facilities Aquaculture, School of Marine Science and Technology, Zhejiang Ocean University, Zhoushan, China
For full use of the by-products during Siberian sturgeon (Acipenser baerii) processing, gelatin was extracted from the cartilages using the hot water method, and its physico-chemical properties and protective function on ultraviolet-A injured human skin fibroblasts (HFSBs) were measured. Using single-factor and orthogonal experiments, the conditions for extracting gelatin from Siberian sturgeon cartilage were optimized as extraction time of 7 h, pH 9, material-to-liquid ratio (g/ml) of 1:5, and temperature of 45°C. The prepared gelatin (TCG) with a yield of 28.8 ± 1.53% had Gly (307 residues/1,000 residues) as the major amino acid and contained a lower amount (214 residues/1,000 residues) of imino acids than that (227 residues/1,000 residues) of pigskin gelatin (PSG). Sodium dodecyl sulfate–polyacrylamide gel electrophoresis (SDS-PAGE), ultraviolet spectrum, and infrared spectroscopy analysis indicated that TCG had the main spectral characteristics of fish gelatin and contained α chains (α1 and α2 chains) and β chain of type I collagen, but its structural stability was lower than that of PSG due to its low content of imino acids, which induced the smaller molecular bands observed in the SDS-PAGE pattern. TCG exhibited lower water content, gel strength, emulsion stability index, foam capacity, foam stability, and water-holding capacity but higher ash content, transmittance, emulsion activity index, and fat-binding capacity (P < 0.05). Moreover, TCG could significantly protect HFSBs against ultraviolet-A injury by enhancing the activity of superoxide dismutase, catalase, and glutathione peroxidase to scavenge excess reactive oxygen species and decrease the content of malondialdehyde. Therefore, gelatin from Siberian sturgeon cartilages could act as promising candidates when applied in health-promoting products against ultraviolet-A injury.
Introduction
Gelatin with a molecular weight (MW) of 80–250 kDa is produced from partially hydrolyzed collagens from animal bones, skins, and connective tissues (Yang et al., 2019; Uddin et al., 2021; Cen et al., 2022). Taking into consideration its diverse and excellent properties, gelatin serves as a multifunctional ingredient applied in a variety of fields, especially food, cosmetics, photography, and pharmaceutical products (Sinthusamran et al., 2018; Al-Nimry et al., 2021; Valcarcel et al., 2021). The global market volume of gelatin is expected to exceed 650 kt, which is about US$ 4 billion, by 2024, and about 80 and 15% of the edible gelatin generated from pig skins and cattle hide splits in Europe (Gelatin Manufacturers of Europe (GME), 2016; Grand View Research, 2016). Confronting the issue is that gelatins generated from mammalian skins and bones have drawn the wide concern of customers because of the increasing infectious diseases and dietary restriction in Islam, Judaism, and Hinduism (Qiu et al., 2019; Uddin et al., 2021; Cen et al., 2022). Therefore, research interests are focused on searching and identifying substitute gelatins from alternative resources (Lv et al., 2019; Nurilmala et al., 2020).
According to the State of World Fisheries and Aquaculture 2020, marine capture fishery production was about 84.4 million tons (FAO, 2020). However, only approximately 50% of these catches are applied for human consumption, and vast aquatic product processing by-products, including bone, swimming bladder, viscera, head, frame, skin, and scales, were generated in the manufacturing process of those commercial fish (Ali et al., 2021; Coppola et al., 2021; Raju et al., 2021; Wang et al., 2022). Those by-products create burdensome disposal problems and will bring serious environmental pollution problems if they are subjected to unreasonable treatment (Ahmed et al., 2019; Yuan et al., 2021; Nisar et al., 2022; Wang et al., 2022). Therefore, researchers persistently search for active ingredients from these by-products, and several ingredients, such as collagen/gelatin, bioactive peptides, chitin/chitosan, chondroitin sulfate, and unsaturated fatty acids, have establish prepared methods for producing high-value marketable products (Zhao et al., 2018a; Zhao et al., 2018; Zhang et al., 2019; Nag M et al., 2022). Among these active ingredients, gelatin can serve as a substitute for mammalian gelatin due to its high nutrition, excellent moisture retention, and good biocompatibility properties (Al-Nimry et al., 2021; Cen et al., 2022). Additionally, the application of fish gelatin and its derivatives in medicine and cosmetics attracted great interest (Lv et al., 2019; Qiu et al., 2019; Al-Nimry et al., 2021). Gelatins and its hydrolysates from Pacific cod (Gadus macrocephalus) showed a significantly protective effect on ultraviolet-A (UVA) damaged cells and skins through increasing the activity of antioxidases, including superoxide dismutase (SOD), catalase (CAT), and glutathione peroxidase (GSH-Px) (Sun et al., 2013; Chen and Hou, 2016; Chen et al., 2016b). Gelatins from tilapia scale showed a significantly enhanced efficacy on cell adhesion, growth, and wound healing and a protective function on H2O2-damaged HaCaT cells (Huang et al., 2018). Therefore, fish gelatins have a potential application value in wound healing and skin photoaging.
Sturgeon, belonging to the family Acipenseridae, is the common name for 27 cartilaginous fish, and its farmed production in China was about 4.4 million tons, accounting for approximately 80% of world production (Gui et al., 2015; Luo et al., 2018). In the receiving process of sturgeon eggs, cartilage, which accounts for 10% of sturgeon’s weight, becomes a by-product. Therefore, active substances in sturgeon cartilage, such as chondroitin sulfate (Gui et al., 2015; Wang et al., 2020; Wu et al., 2022), collagen (Luo et al., 2018; Zhu et al., 2020; Lai et al., 2020), and antioxidant peptides (Yuan et al., 2021), were studied constantly for replacing shark cartilage used in health and functional products. In addition, cartilage collagens have been prepared from some cartilaginous fishes, such as Siberian sturgeon (Luo et al., 2018), scalloped hammerhead, red stingray, skate (Chi et al., 2013), silvertip shark (Jeevithan et al., 2014a; Jeevithan et al., 2014b), brownbanded bamboo shark, and blacktip shark (Kittiphattanabawon et al., 2010). Those collagens have shown potential to replace collagens from mammalian sources. Moreover, previous literatures indicated that gelatins, collagen hydrolysates, and peptides derived from cartilaginous fishes show significant biological activities, such as antioxidant (Li et al., 2013; Pan et al., 2016; Li et al., 2017; Tao et al., 2018), immunity enhancement (Bu et al., 2017), and anti-tumor (Pan et al., 2016). However, no report on the gelatin from sturgeon cartilage was found. Therefore, gelatin from the cartilage of Siberian sturgeon (Acipenser baerii) was prepared and characterized in this experiment. Furthermore, the protective function of cartilage gelatin on UVA-injured human skin fibroblasts (HSFBs) was evaluated.
Materials and Methods
Materials and Chemical Reagents
Cartilages of Siberian sturgeon (A. baerii) were kindly provided by Thousand Island Lake Sturgeon Technology Co., Ltd. (Hangzhou, China). Glutathione (GSH), Dulbecco’s modified Eagle’s medium (DMEM), pigskin gelatin (PSG), and high-MW protein markers were purchased from Shanghai Source Poly Biological Technology Co., Ltd. (China). HSFBs were purchased from Shanghai Cell Bank of Chinese Academy of Sciences. The cartilage collagen of Siberian sturgeon was prepared in our lab according to the reported method (Luo et al., 2018).
Preparation of Gelatin of Siberian Sturgeon Cartilage
Pretreatment of Cartilages
Cartilages of Siberian sturgeon were pretreated according to the reported process (Chi et al., 2013; Luo et al., 2018). Briefly, the cartilages were unfrozen, homogenized, treated with NaOH solution (0.1 M) to remove noncollagenous proteins, and decalcified using EDTA-2Na solution (0.5 M, pH 7.5).
Gelatin Extraction From Pretreated Cartilages
The pretreated cartilages were soaked in acetic acid solution (0.2 M) with a cartilage/solvent ratio of 1:10 (w/v) and have undergone limited hydrolysis with commercial pepsin at a dose of 2.0% (w/w) for 12 h at 4°C. Then, the resulting cartilages were cleaned using tap water, put in distilled water with continuous stirring, and extracted according to the designed conditions.
The extraction conditions of gelatin were firstly optimized by a single-factor experiment. Extraction time (2, 4, 6, 8, and 10 h), pH (6, 7, 8, 9, and 10), material-to-liquid ratio (1:2, 1:4, 1:6, 1:8, and 1:10, w/v), and temperature (30, 35, 40, 45, and 50°C) were chosen for the extraction of cartilage gelatin. According to the results, an orthogonal experiment [L9(34)] was designed to optimize the conditions of extraction time (5, 6, and 7 h), pH (7, 8, and 9), material-to-liquid ratio (1:5, 1:6, and 1:7), and temperature (40, 45, and 50°C) (Table 1).
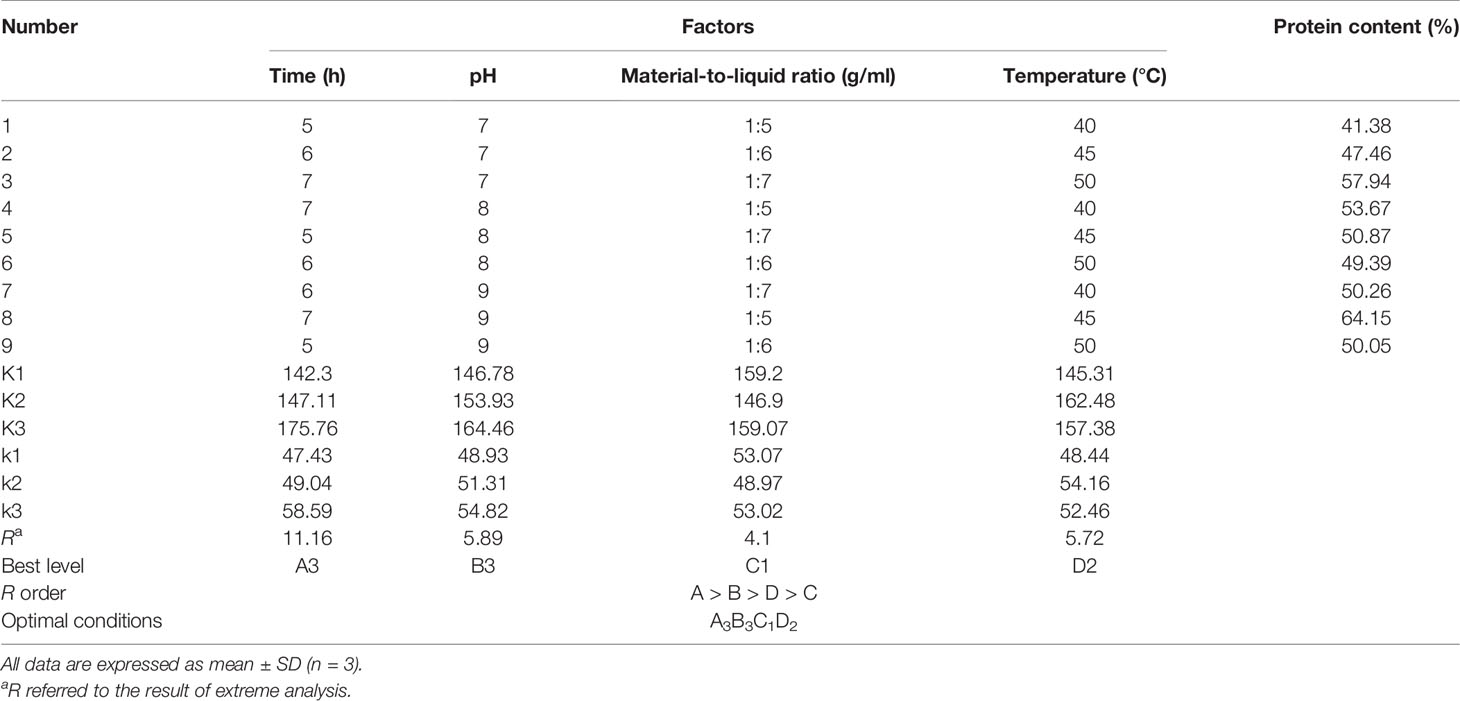
Table 1 Orthogonal array design matrix L9 (34) and experimental results for optimizing gelatin extraction conditions from Siberian sturgeon cartilage.
After the above-mentioned extraction process, the mixture solution was filtered using two layers of cheesecloth, and the resultingfiltrate was centrifuged at 4,000 g for 20 min at 4°C to wipe off unsolvable cartilage matter. The supernatant was collected and freeze-dried, and the gelatin prepared under optimal conditions was named as TCG. The yields of TCG were calculated based on the following equation: yield (%) = (weight of dried TCG/dry weight of used cartilages) × 100.
Characterization of TCG
Proximate Analysis
The moisture and ash contents of TCG and PSG were measured by the methods of AOAC (2003), using the method numbers 950.46B and 920.153 (AOAC. Official Methods of Analysis of AOAC International and Gaithersburg, 2003).
Determination of Amino Acid Composition
The amino acid composition of TCG and PSG was analyzed using the method reported by Qiu et al. (Qiu et al., 2019). The samples were hydrolyzed in 6 M HCl (110°C) for 24 h. The product was concentrated, and the residue was dissolved in 25 ml of citric acid buffer. The amino acid composition was analyzed using an automated amino acid analyzer of HITACHI 835-50 (Hitachi High-Technologies Corporation, Tokyo, Japan).
Sodium Dodecyl Sulfate–Polyacrylamide Gel Electrophoresis Pattern
The electrophoretic pattern of TCG was measured according to a previous method using 4% stacking gel and 7.5% separating gel (Yu et al., 2014; Li et al., 2018). The MWs of the TCG components were estimated using high-MW protein markers. PSG and collagen of Siberian sturgeon cartilage were used as standards.
Ultraviolet Absorption and Fourier Transform Infrared Spectra
The UV absorption spectra of TCG and PSG (0.5 mg/ml) from 190 to 400 nm were measured using a UV-1800 spectrophotometer (Mapada Instruments Co., Ltd., Shanghai, China). The FTIR spectra of TCG and PSG with wavenumber of 450–4,000 cm-1 were recorded in KBr disks with a Nicolet 6700 spectrophotometer (Thermo Fisher Scientific Inc., USA). TCG and PSG were separately mixed with KBr at a ratio of 1:100 (w/w) and squeezed into a transparent disk for further analysis.
Isoelectric Point Determination
The zeta potential was measured using a previous method (Kaewdang et al., 2014). Briefly, TCG samples (0.5 mg/ml) with pH ranging from 3 to 9 were stirred for 6 h, and the zeta potential of each gelatin sample was determined by a Zeta PALS analyzer (Brookhaven Instruments Co., Holtsville, NY, USA). The isoelectric point (pI) was calculated from a pH rendering a zeta potential of zero.
The percent transmittance of each TCG sample (1.0 mg/ml, pH 3–9) at 620 nm was measured using a UV-1800 spectrophotometer. The graph was made according to the relationship between pH and percent transmittance, in which the pH value corresponding to the lowest transmittance was the pI of gelatin.
Determination of Gel Strength
The gel strength of TCG and PSG was determined using the method of the British Standards Institution and was expressed as the maximum force (in grams) required for the plunger to press the gel by 4-mm depression at a rate of 0.5 mm/s (BSI (British Standards Institution), 1975).
Determination of Functional Properties
The emulsion activity index (EAI), foam capability (FC), foam stability (FS), and emulsion stability index (ESI) of TCG and PSG were determined according to the methods of Jellouli et al. (Jellouli et al., 2011). The fat-binding capacity (FBC) and water-holding capacity (WHC) of TCG and PSG were determined as per the reported procedures of Shyni et al. (Shyni et al., 2014).
Protective Function of TCG on UVA-Injured HSFBs
Cell Culture and Viability Determination
The HSFBs were cultured according to the method reported by Gęgotek et al. (Gęgotek et al., 2018) and Lin et al. (Lin et al., 2020). In brief, HSFBs (1.0 × 105 cells/well) were seeded into a 96-well plate containing 100 μl of culture media. After incubation for 24 h, 20 μl of TCG solution dissolved in the DMEM medium was added in the sample group, and this reached the final concentrations of 50, 100, 200, and 400 μg/ml, respectively. In addition, TCG was substituted by phosphate-buffered solution (PBS, pH 7.2) in the blank control group. After incubation for 24 h, 20 μl of MTT was added into the plate and OD490 nm was measured after 4 h. The cell viability was calculated by the formula:
Cell viability (%) = (ODsample/ODcontrol) × 100.
Protection of TCG on UVA-Injured HSFBs
HSFBs (1.0 × 105 cells/well) were seeded into a 96-well plate containing 100 μl of culture media. When the monolith of cells was covered with a 96-well plate, the culture media was discarded, and the HSFBs were washed three times with PBS buffer. Then, the resulting HSFBs were covered in PBS buffer and irradiated at a distance of 15 cm with 5, 10, 15, and 20 J/cm2 of UVA, respectively. After radiation, the HSFBs were washed three times with PBS buffer and cultured in a new culture medium for 24 h. The dose of UVA resulted in the HSFB viability of approximate 50% was chosen to establish the cell model (Lone et al., 2020).
The irradiated HSFBs were cultured in new culture media for 24 h. After aspirating the supernatant, 20 µl of TCG (with final concentrations of 50, 100, and 200 μg/ml, respectively) was loaded into the sample groups, respectively. After incubating for 24 h, the cell viability was determined. The blank and model groups used 20 μl PBS instead of TCG solution.
Determination of Reactive Oxide Species, Malondialdehyde, and Antioxidases
The levels of ROS in each group were measured by the previous method and presented as percent of blank control (Cai et al., 2019). The activity of antioxidases (SOD, GSH-Px, and CAT) and the content of MDA were measured using assay kits according to the protocols of Nanjing Jiancheng Bioengineering Institute Co., Ltd. (China), and the activity of antioxidases was indicated as U/mg prot.
Statistical Analysis
The data are expressed as mean ± standard deviation (SD, n = 3). An ANOVA test was used to analyze the differences between means of each group using SPSS 19.0 (Statistical Program for Social Sciences, SPSS Corporation, Chicago, IL, USA). Tukey or Duncan’ test was used to determine the significance between different groups (P < 0.05, P < 0.01, or P < 0.001).
Results and Discussion
Preparation of Gelatin From Siberian Sturgeon Cartilage
Optimization of Gelatin Extraction Conditions Using a Single-Factor Experiment
The extraction conditions play a key role in influencing the yield and physicochemical properties of gelatins (Pan et al., 2018; Kim et al., 2020; Fawale et al., 2021). Then, the effects of the extraction conditions, including time, pH, material-to-liquid ratio, and temperature, on the protein contents of the gelatin extract solution of Siberian sturgeon cartilage were optimized by a single-factor experiment (Figure 1). Figure 1A indicates that the protein content (52.10 ± 0.19%) of the gelatin extract solution prepared for 6 h reached the maximum, but it was not significantly higher than those of the gelatin extract solution prepared for 8 and 10 h (P < 0.05). Figure 1B illustrates that the protein content of the gelatin extract solution increased gradually when the pH value increased from 6 to 9 and approached the peak level (53.05 ± 0.27%) at pH 9. In addition, no significant difference in the protein content of the gelatin extract solution was observed at pH 7, 8, and 9 (P > 0.05). Figure 1C demonstrates that the protein content of the gelatin extract solution prepared at a material-to-liquid ratio of 1:6 was 57.71 ± 0.51%, which was significantly higher than those of the gelatin extract solution prepared at other material-to-liquid ratios (P < 0.05). Figure 1D indicates that the protein content of the gelatin extract solution was significantly influenced by the extraction temperature, and the protein content (59.22 ± 0.72%) of the gelatin extract solution prepared at 45°C was prominently higher than those of the gelatin extract solution prepared at other extraction temperatures (P < 0.05). Therefore, the range of extraction conditions of cartilage gelatin from the Siberian sturgeon was narrowed to 5–7 h, 7–9, 1:5–1:7, and 40–50°C for extraction time, pH, material-to-liquid ratio, and temperature, respectively.
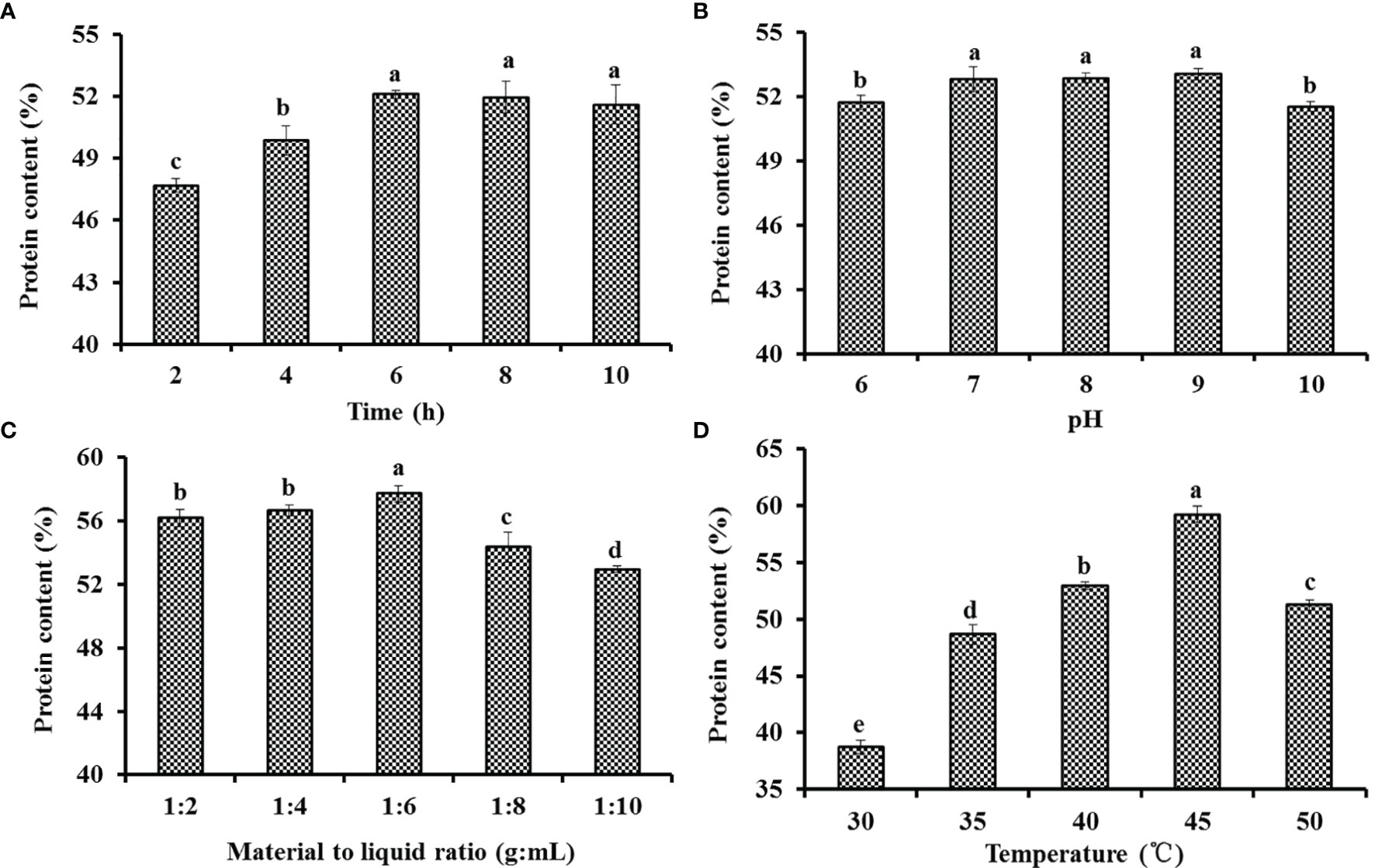
Figure 1 Effects of time (A), pH (B), material-to-liquid ratio (C), and temperature (D) on the protein contents of the gelatin extract solution of Siberian sturgeon cartilage. All data are expressed as mean ± SD (n = 3). Values with the same letters indicate no significant difference (P > 0.05).
Optimization of Gelatin Extraction Conditions Using Orthogonal Experiment
The results of the orthogonal experiment were analyzed and are shown in Table 1. Based on the K, k, and R values, the extraction conditions which affected the gelatin yield are shown in a descending order as follows: A > B > D > C. Therefore, time was proven to be the most key condition affecting the protein contents of the gelatin extract solution of Siberian sturgeon cartilage. According to the results of the orthogonal test, the optimum extraction conditions of gelatin from Siberian sturgeon cartilage were A3B3C1D2, that is to say, the optimal conditions were extraction time 7 h, pH 9, solid–liquid ratio (g/ml) 1:5, and temperature 45°C, respectively. Finally, the protein content of the gelatin extract solution reached 61.57 ± 1.11% under the optimized conditions by the confirmatory test.
Characterization of TCG
Basic Properties of TCG
As shown in Table 2, the yield of TCG was 28.8 ± 1.53% on dry weight basis. In our previous report, the yield of cartilage collagens (including acid-soluble and pepsin-soluble collagens) of Siberian sturgeon was 41.82% on dry weight basis (Luo et al., 2018), which indicated that part of the gelatin still remained in the raw material under the extraction process of this experiment.
The basic properties of TCG and PSG are shown in Table 2. The indexes of moisture, ash, and transparency of TCG were significantly better than those of the national standard of China (GB 6783-2013) Standardization Administration of China, (2013), which indicated that the TCG could be used as a substitute of PSG in food and medical industries. In addition, the water content of TCG was 6.13 ± 0.58%, which was significantly lower than that of PSG (P < 0.05). The finding indicated that TCG should have a longer storage life. The ash content of TCG (0.76 ± 0.05%) was significantly higher than that of PSG (P < 0.05), which might be that small amounts of minerals remained in the cartilage under the current process of EDTA-2Na.
The gel strength of TCG was 206.1 ± 4.7 g, which was same to that (206 g) of the gelatin skins of dog shark (Shyni et al., 2014), and significantly weaker than that of PSG (398.0 ± 5.2g) (P < 0.05). The finding was in line with the previous literatures that the gel strength of aquatic gelatin was weaker than those of mammalian gelatins (Boran and Regenstein, 2010; Fawale et al., 2021). In addition, the gel strength of gelatin is closely related to the content of imino acids, and the present finding suggested that the content of imino acids in TCG could be lower than that in PSG.
Amino Acid Composition of TCG
Amino acid composition was one of the most important factors that affect the properties of gelatins from marine resources (Yang et al., 2019; Qiu et al., 2019). As shown in Table 3, the amino acid composition patterns of TCG and PSG were similar. Firstly, Gly was the amino acid of TCG with a content of 307 residues/1,000 residues, which was because approximately 50-60% of α-chains were made up of a typical tripeptide repetition of Gly-X-Y (Chi et al., 2014; Tkaczewska et al., 2018). Secondly, TCG was rich in Pro, Glu, and Hyp in a descending order, and the data were in line with the previous literatures that those amino acids were the main compositions of gelatins (Regenstein and Zhou, 2007; Ahmad et al., 2018).
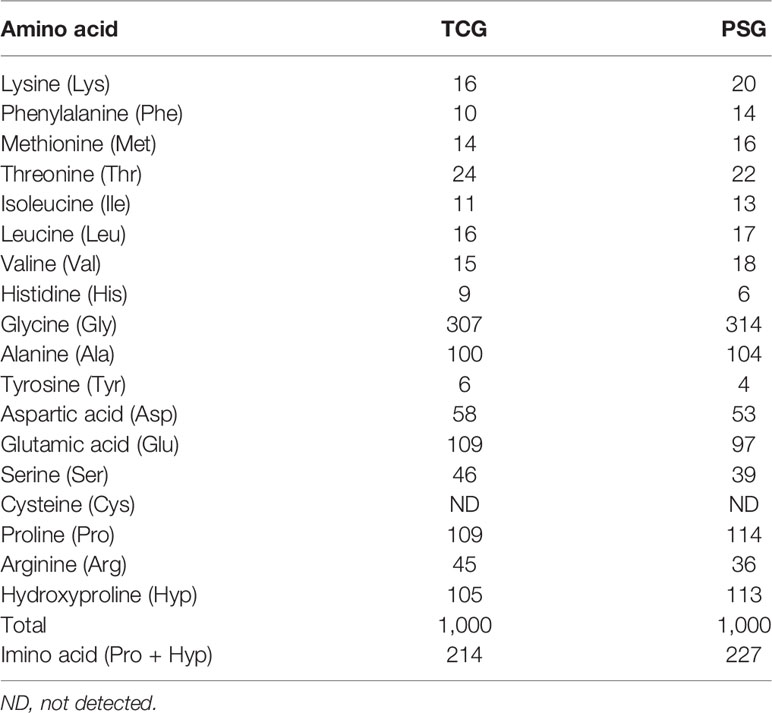
Table 3 Amino acid composition of cartilage gelatin (TCG) from Siberian sturgeon and pigskin gelatin (PSG) (residues/1,000 residues).
The triple-stranded collagen helix can be stabilized by the number of hydrogen bonds, which are formed through the hydroxyl group of Hyp (Chi et al., 2014; Tkaczewska et al., 2018). A high content of imino acids (Pro and Hyp) is helpful to intensify the thermostability of the triple helical structure of collagen/gelatin because their pyrrolidine rings can hold back the changes of the secondary configuration of polypeptide chains (Li et al., 2013a; Wang et al., 2013; Zhao et al., 2018a). Table 3 presents that the ratio of imino acids in TCG was 214 residues/1,000 residues, which was significantly less than that of PSG (227 residues/1,000 residues). Therefore, the helical structure of TCG could be easily destroyed than that of PSG due to the lower ratio of imino acids. In addition, the lower content of imino acids in TCG meant that its gel strength was weaker than that of PSG (Table 2).
SDS-PAGE Pattern of TCG
Molecular weight pattern additionally impacts the properties of collagen/gelatin (Jellouli et al., 2011; Kaewdang et al., 2014; Luo et al., 2018). Sodium dodecyl sulfate–polyacrylamide gel electrophoresis (SDS-PAGE) patterns of TCG, sturgeon cartilage collagen, and PSG were depicted in Figure 2. Two α chains (α1 and α2 chains) and one β chain were observed in the SDS-PAGE patterns of TCG, sturgeon cartilage collagen, and PSG, which proved that TCG and PSG kept the structure of type I collagen (Li et al., 2013a; Shyni et al., 2014; Yang et al., 2019). However, the lowest contents of α and β chains were found in TCG compared with those of sturgeon cartilage collagen and PSG. In addition, more molecular fragments below 100 kDa were found in TCG. This finding was mainly caused by two factors: firstly, cartilages of Siberian sturgeon were pretreated using pepsin at a dose of 2.0% (w/w) for 12 h, which induced the partial peptide bonds to be broken. Moreover, TCG contained less imino acids than PSG, so its structural stability was weaker than that of PSG and was easier to degrade.
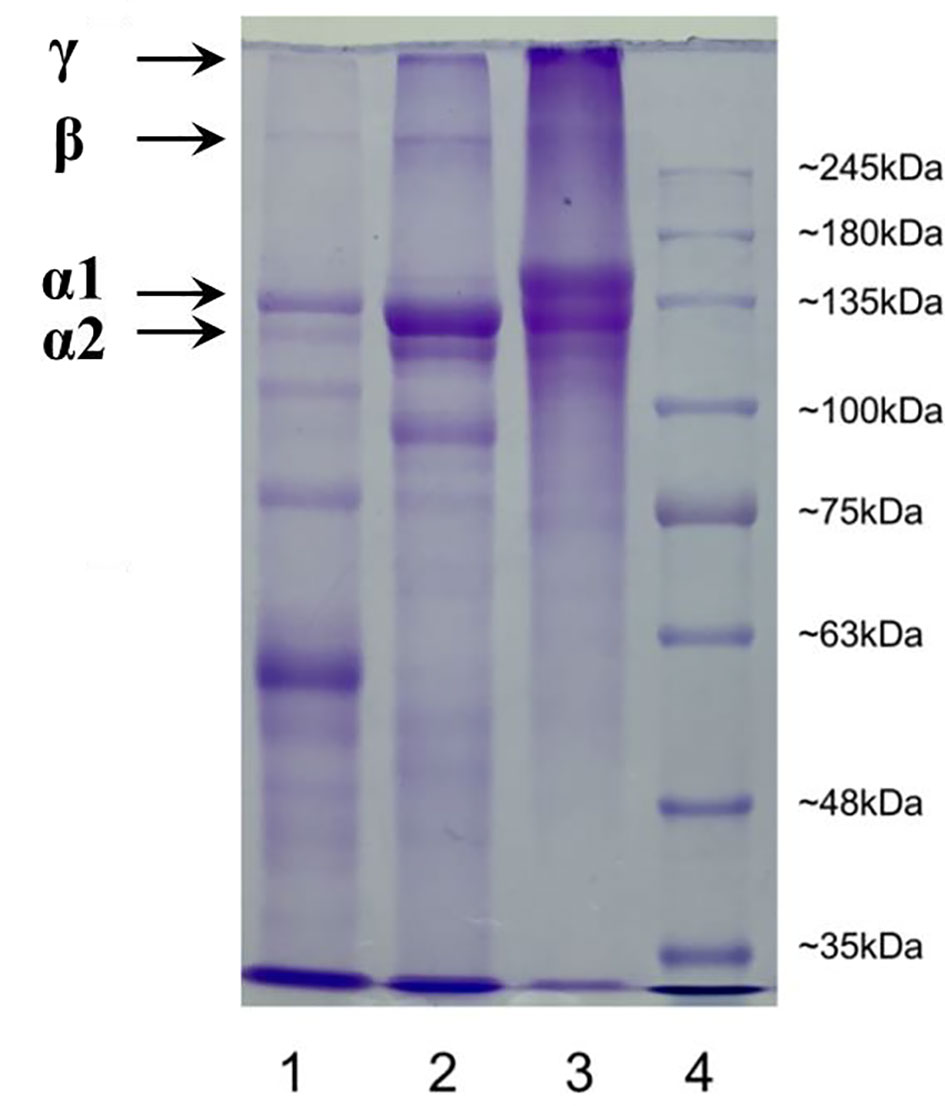
Figure 2 SDS-PAGE gel electrophoresis of cartilage gelatin from Siberian sturgeon and pigskin gelatin. Lane 1, sturgeon cartilage gelatin; lane 2, sturgeon cartilage collagen; lane 3, pigskin gelatin; 4, protein marker.
In addition, the results indicated that the present extraction method of TCG could significantly degrade the large molecules of α and β chains into small molecular peptides, which further reduce the strength of TCG (Bkhairia et al., 2016). The finding was agreement with the result in Table 1 that the gel strength of TCG was weaker than that of PSG.
UV and FTIR Spectra of TCG
The UV absorption spectra of TCG and PSG are shown in Figure 3. The results illustrated that the UV typical absorption peaks of TCG was approximately 229.0 nm, which was due to the existence of C=O, COOH, and CONH2 groups in gelatins (Qiu et al., 2019). In addition, the maximum wavelength of TCG was close to that (219 nm) of PSG (Figure 3B).
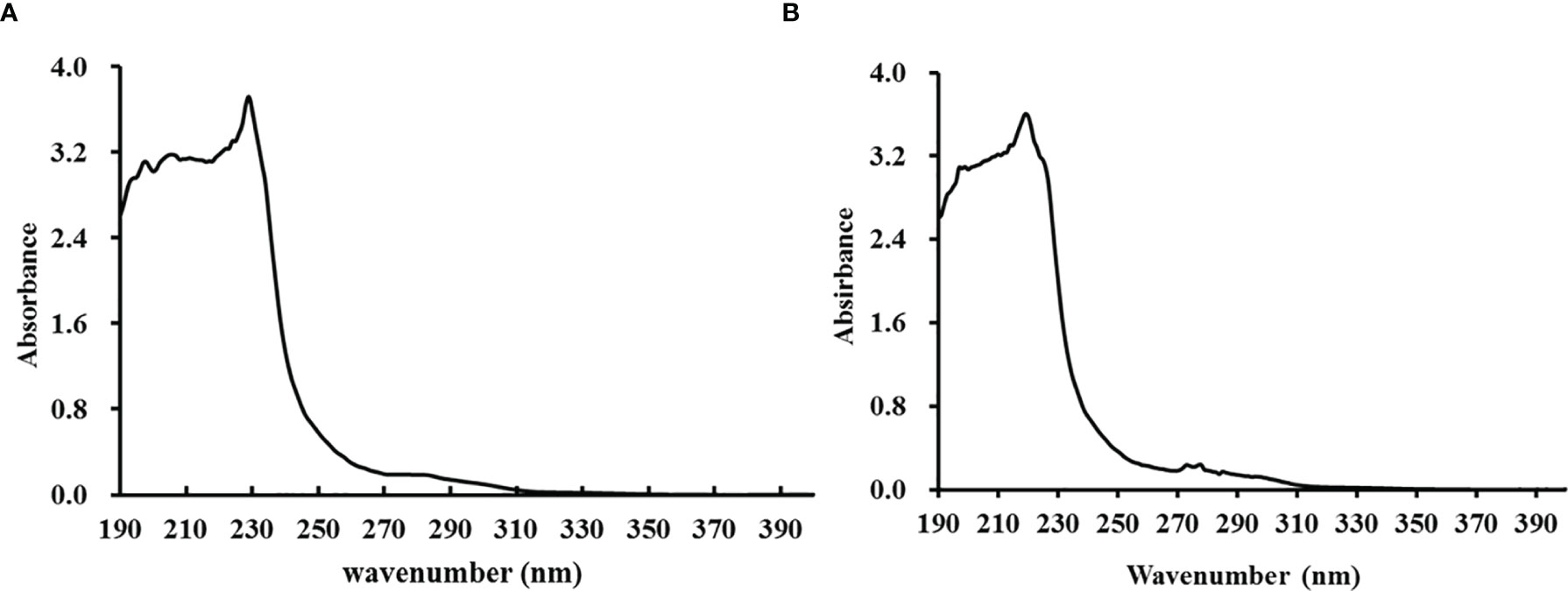
Figure 3 Ultraviolet absorption spectra of cartilage gelatin (A) from Siberian sturgeon and pigskin gelatin (B).
The FTIR spectrum of gelatin has five main regions: amide A (~3,300 cm-1), amide B (~3,100 cm-1), amide I (~1,660 cm-1), amide II (~1,570 cm-1), and amide III (~1,300 cm-1) (Sinthusamran et al., 2018; Ali et al., 2021). Figure 4 shows that the FTIR spectra of TCG and PSG were relatively similar and presented the characteristic vibration mode of gelatin, but the wavenumber position of the peak,was slightly different, indicating that their secondary structures were somewhat different (Cao et al., 2020). The amide A band is connected with the stretching vibration of the NH group. The stretching vibration height of the NH group is set at 3,400–3440 cm-1, but when the NH group joined in the hydrogen bond formation, the absorption peak of the stretching vibration shifts to a lower wavenumber. The amide A bands of TCG and PSG were 3,340 and 3,311 cm-1, respectively, proving that more NH groups joined in the formation of hydrogen bonds. It has been reported that amide A in gelatin appears at a shorter frequency, and less intramolecular hydrogen bond is broken (Zheng et al., 2017; Cao et al., 2020). Therefore, PSG retained more hydrogen bonds in molecules, while more hydrogen bonds were damaged in TCG. The amide B bands of TCG and PSG are 2,926 and 2,934 cm-1, respectively. The amide B band of TCG appeared at a lower wavenumber, which manifested the more interaction of NH3 groups among gelatin molecules (Yang et al., 2019). The amide I band is mainly related to the stretching vibration of the carbonyl group C=O (Stani et al., 2020). It reflects the secondary structure of peptides and proteins. Amide II is connected with NH bending and CN stretching vibrations. The wavenumb,ers of amide I and amide II of TCG (1,639 and 1,406 cm-1) were less than those of PSG (1,652 and 1,450 cm-1), which might be related to the decrease in molecular number. Amide III is involved in the tertiary helix structure of proteins. The amide III band of TCG (1,125 cm-1) was less than that of PSG (1,165 cm-1), indicating that the triple helix structure of TCG was partially destroyed in the preparation of gelatin.
Zeta Potential and Transparency of TCG
The zeta potential of TCG at different pH values is presented in Figure 5. At the acidic pH range (Sinthusamran et al., 2018; Yang et al., 2019; Cen et al., 2022), TCG had a positive charge, but it changed to the negative charge when the solution pH ranged from 5 to 9. According to the curve in Figure 5A, the net charge of TCG changed to zero at pH 4.53, which was regarded as the isoelectric point (pI) of TCG. The pI of TCG at an acidic pH was due to its high contents of Glu and Asp (101 and 60 residues/1,000 residues, respectively) (Table 3). In addition, the result that the transmittance was the lowest when the pH values of the gelatine solution was near 4.5 was confirmed (Figure 5B).
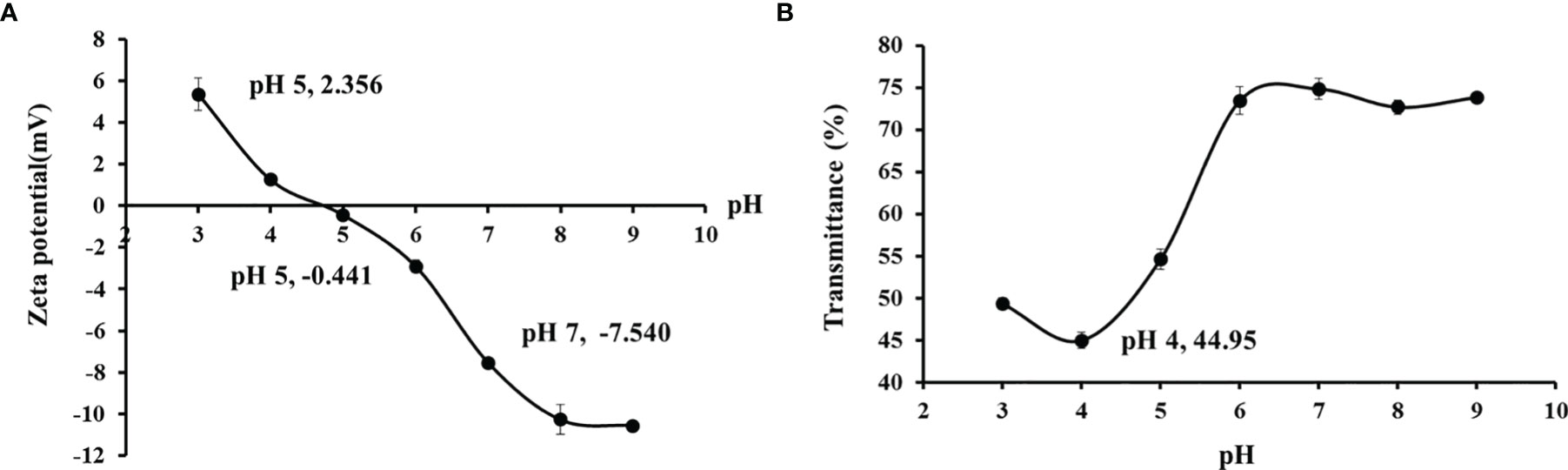
Figure 5 Zeta potential (A) and transparency (B) of cartilage gelatin from Siberian sturgeon. All data are expressed as mean ± SD (n = 3).
Emulsifying Property, Foam Capacity, and Foam Stability of TCG
The EAI of TCG was 45.39 ± 1.67 m2/g, which was lower than that (32.26 ± 1.58 m2/g) of PSG (P < 0.05) at 1.0 g/100 ml (Table 4). In the dispersing phase, proteins with high solubility can significantly improve their emulsifying efficiency because protein molecules can move rapidly to the surface of the fat droplets (Jellouli et al., 2011). The ESI of TCG was lower than that of PSG (P < 0.05), indicating that emulsions containing PSG were more stable than that of the TCG. The results agreed with the report that larger gelatin (MW = 120 kDa) could stabilize oil-in-water emulsions more effectively than low-molecular-weight gelatin (MW = 50 kDa) (Jellouli et al., 2011).
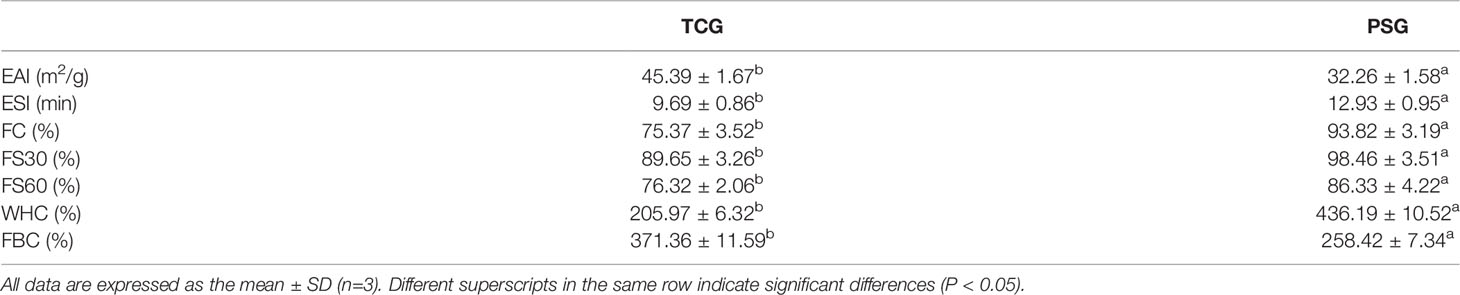
Table 4 Emulsion activity index (EAI), emulsion stability index (ESI), foam capacity (FC), foam stability (FS), fat-binding capacity (FBC), and water-holding capacity (WHC) of cartilage gelatin (TCG) and pigskin gelatin (PSG).
Gelatin is generated by the incomplete degradation of collagen, and the solubility of collagen hydrolysates was positively correlated with their molecular weights (Li et al., 2013; Chi et al., 2016). Therefore, SDS-PAGE patterns indicated that more molecular fragments below 100 kDa and lower contents of α and β chains were found in TCG compared with PSG (Figure 2), which caused the high EAI and low ESI of TCG.
As shown in Table 4, the FC of TCG (75.37 ± 3.52%) was significantly lower than that of PSG (93.82 ± 3.19%) (P < 0.05). The foaming characteristics of gelatins are positively correlated with the hydrophobicity of the unfolded proteins, which form the hydrophobic regions for the adsorption at the air–water interface (Mutilangi et al., 1996; Jellouli et al., 2011). The difference in FC between TCG and PSG should be due to the lower content (275 residues/1,000 residues) of hydrophobic amino acid (Ala, Val, Iso, Leu, Pro, Met, and Phe) of TCG than that (296 residues/1,000 residues) of PSG.
Similarly, the FS of TCG was lower than that of PSG at 30 and 60 min at the same concentration. The FS of the gelatin solution is generally positively related to the MWs of polypeptides (van der Ven et al., 2002). The molecular weight of TCG was lower than that of PSG, causing the films formed using TCG to have a weaker and smaller elasticity, thus inducing their unstable foam properties.
FBC is dependent on the content of the exposured hydrophobic residues inside the gelatin. Table 4 shows that the FBC of TCG was 205.97 ± 6.32%, which was lower than that (436.19 ± 10.52) of PSG. This result is due to the hydrophobic amino acid content of TCG (275 residues/1,000 residues) which was less than that of PSG (296 residues/1,000 residues) (Table 3). Conversely, the WHC of TCG (371.36 ± 11.59) was higher than that (258.42 ± 7.34) of PSG, which should be influenced by the content of hydrophilic amino acids between TCG (725 residues/1,000 residues) and PSG (704 residues/1,000 residues).
Protective Effect of TCG on UVA-Injured HSFBs
Effects of UVA Radiation and TCG Doses on the Viability of HSFBs
HSFBs were irradiated with different doses of UVA (5, 10, 15, 20, and 25 J/cm2) to establish the UVA-injured cell model. As shown in Figure 6A, the viability of HSFBs presented a significantly downward trend as the UVA doses increased from 5 to 25 J/cm2 and dropped to 50.93 ± 3.47% at the dose of 15 J/cm2. Lone et al. reported that the corresponding UVA radiation dose is the optimal choice for establishing a UVA-injured cell model when it induced the cell viability at about 50% (Lone et al., 2020). Therefore, the radiation dose of 15 J/cm2 was chosen to establish the UVA-injured HSFB model.
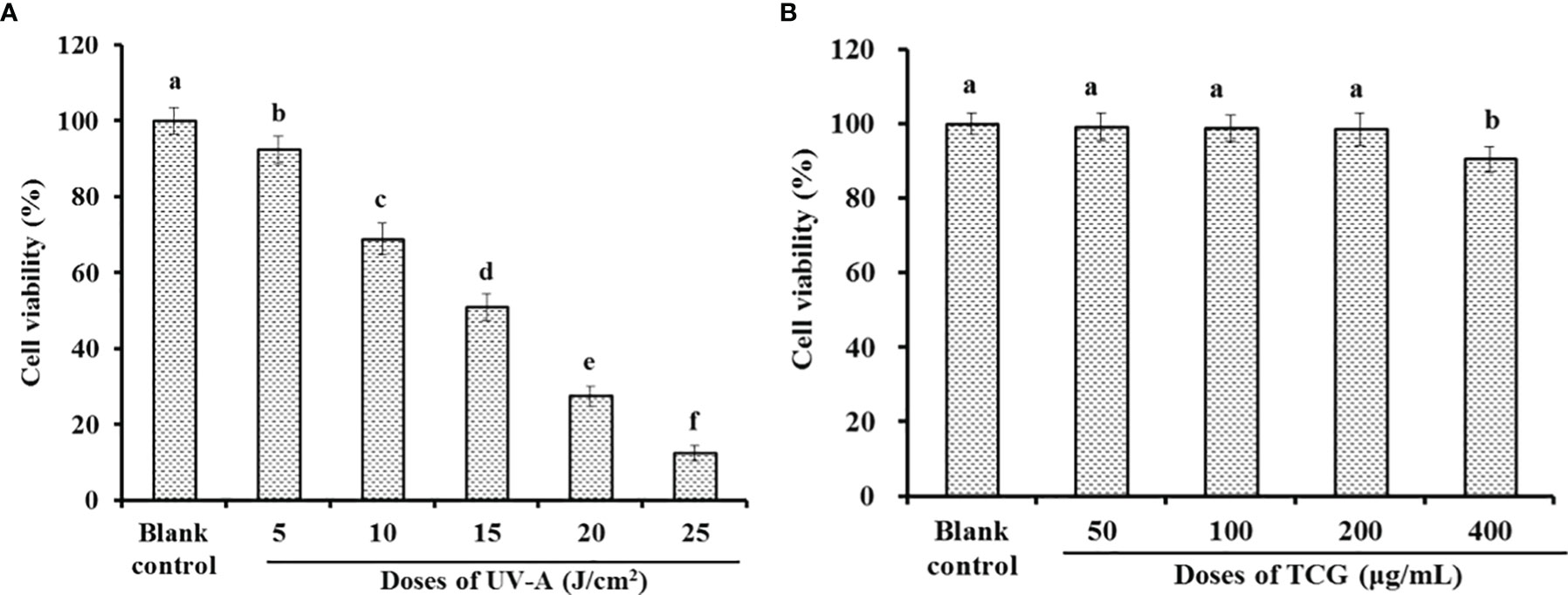
Figure 6 Effects of ultraviolet-A radiation (A) and cartilage gelatin (B) doses on the viability of human skin fibroblasts. All data are presented as the mean ± SD (n = 3). Values with the same letters indicate no significant difference (P > 0.05).
The effects of TCG doses (50, 100, 200, and 400 μg/ml) on the viability of HSFBs were studied by the MTT method, and the data are presented in Figure 6B. No significant difference was found between the blank control and TCG groups when the tested dose was not more than 200 μg/ml. Therefore, the TCG concentrations of 50, 100, and 200 μg/ml were selected for the cell protective experiment.
Effect of TCG on the Cell Viability, ROS Level, and MDA Content of UVA-Injured HSFBs
The protective effect of TCG on UVA-injured HSFBs was investigated, and the data are shown in Figure 7. The HSFB viability of the TCG groups was 58.63 ± 3.66, 64.79 ± 2.98, and 67.76 ± 3.49% at 50, 100, and 200 μg/ml, respectively, and the viability was significantly higher than that (50.93 ± 3.47%) of the model group (P < 0.05) and lower than that (84.34 ± 5.32%) of the positive control (P < 0.001) (Figure 7A). The ROS levels of the TCG groups were significantly decreased from 339.76 ± 23.67 to 293.82 ± 21.98, 259.76 ± 16.58, and 236.39 ± 10.55% of the blank control at 50, 100, and 200 μmol/L, respectively (P < 0.001) (Figure 7B). Similarly, TCG could significantly reduce the MDA contents of the UVA-injured HSFB model. Compared with the model group (6.57 ± 0.35 nmol/mg prot), the MDA contents of TCG were gradually reduced to 5.95 ± 0.31, 5.63 ± 0.28, and 5.38 ± 0.32 nmol/mg protein at 50, 100, and 200 μmol/L, respectively (P < 0.01) (Figure 7C). Furthermore, significant dose–effect relationships between TCG concentrations and cell viability, ROS level, and MDA content were observed, as shown in Figure 7.
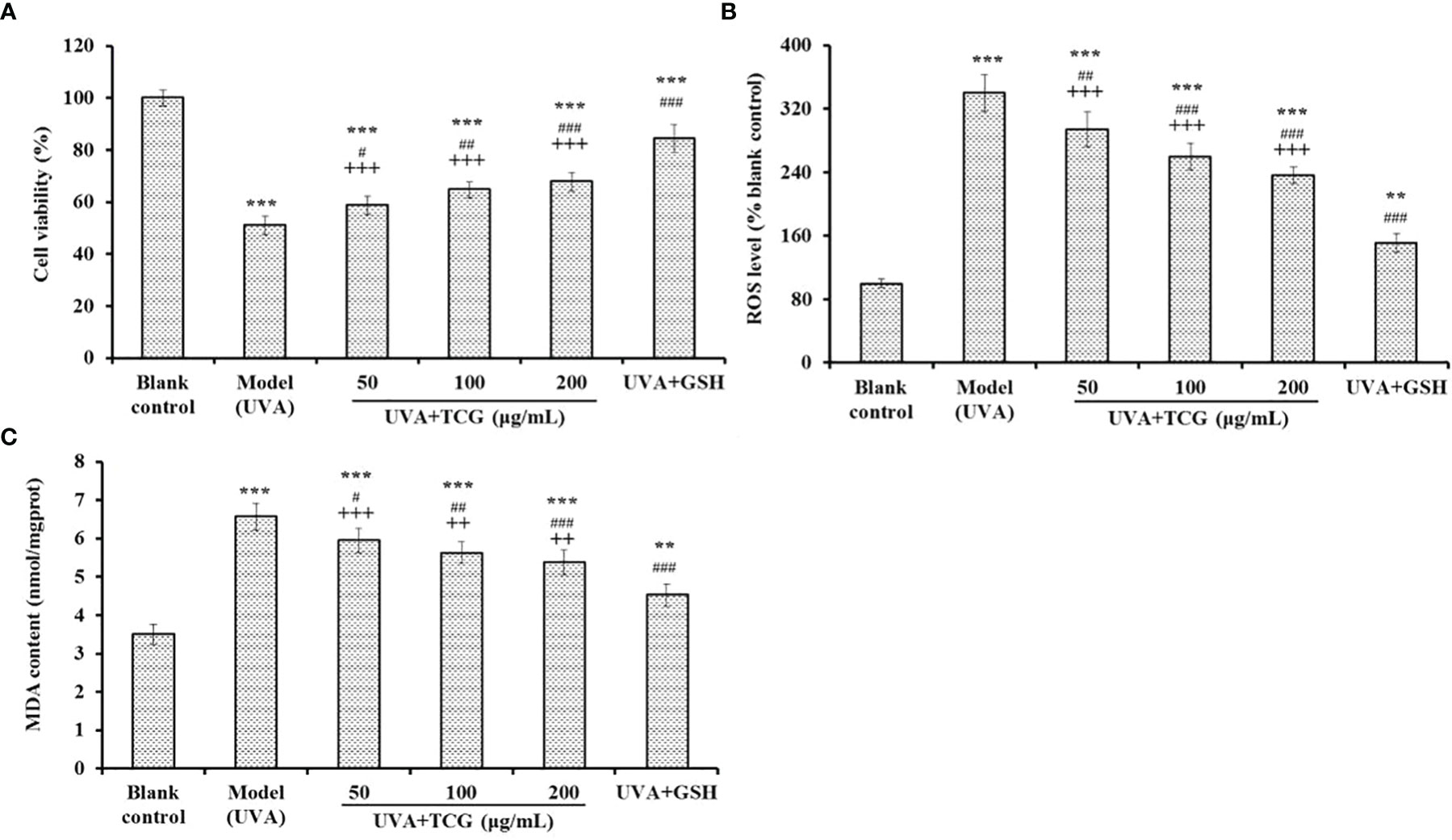
Figure 7 Effects of cartilage gelatin on cell viability (A), reactive oxygen species level (B), and malondialdehyde content (C) of UVA-injured human skin fibroblast model. Glutathione was used as the positive control. All data are presented as mean ± SD (n = 3). ***P < 0.001 and **P < 0.01 vs. blank group; ###P < 0.001, ##P < 0.01, and #P < 0.05 vs. model group; +++P < 0.001 and ++P < 0.01 vs. UVA + GSH group.
Effects of TCG on the SOD, GSH-Px, and CAT Activity in UVA-Injured HSFBs
As shown in Figure 8, the activity of antioxidases (SOD, GSH-Px, and CAT) of UVA-injured HSFBs incubated with TCG was concentration-dependently increased when the TCG concentrations were increased from 50 to 200 μg/ml. At 200 μg/ml, the SOD, GSH-Px, and CAT activity in the TCG groups was 162.29 ± 10.92, 34.17 ± 2.65, and 27.11 ± 1.83 U/mg protein, respectively, which was significantly higher than the activity of SOD (122.36 ± 7.45 U/mg prot), GSH-Px (23.19 ± 1.63 U/mg prot), and CAT (15.78 ± 1.46 U/mg prot) in the model group (P < 0.001). However, the activity of antioxidases in the TCG groups was significantly lower than that of the positive control (P < 0.01).
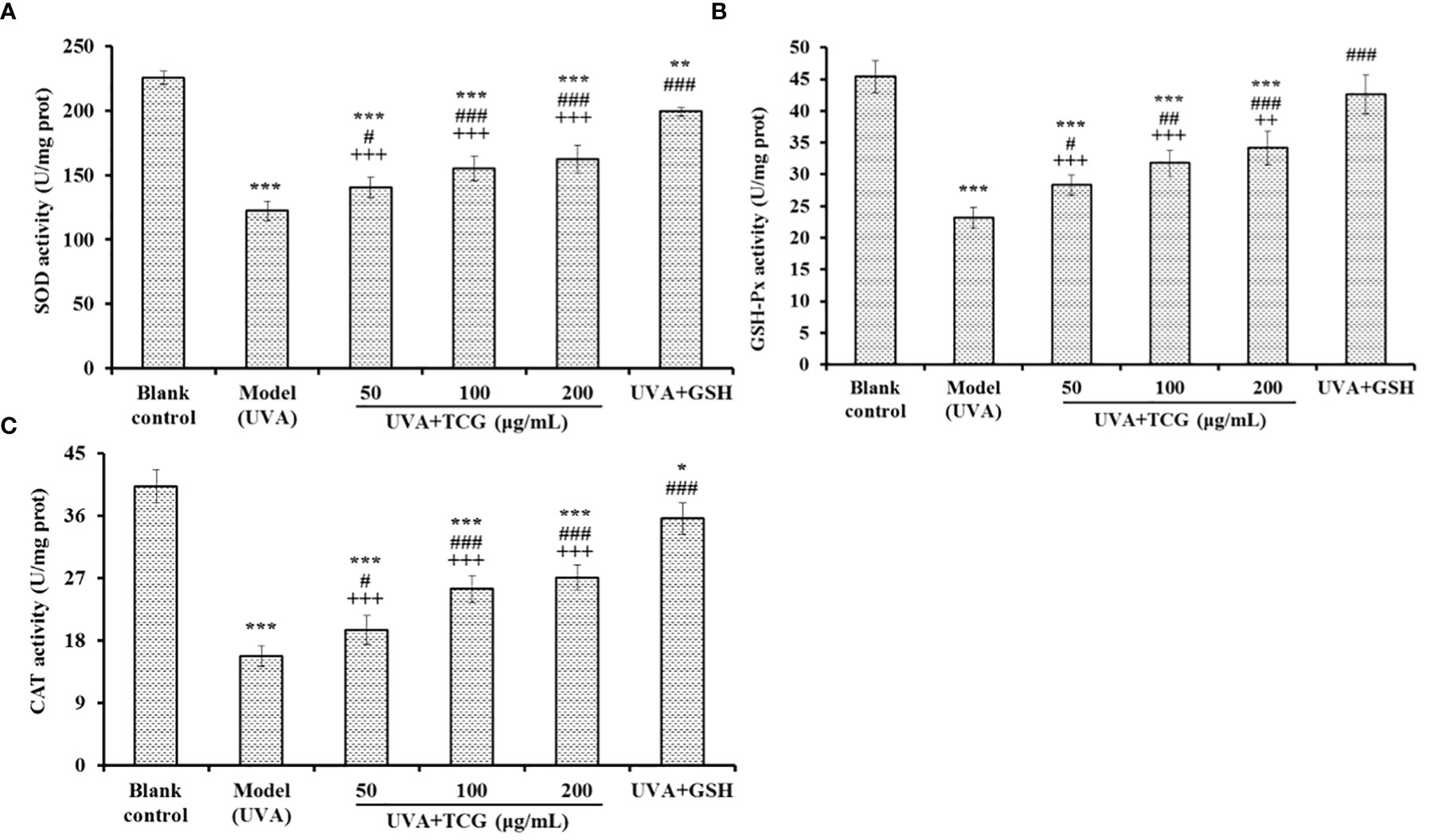
Figure 8 Effects of cartilage gelatin on superoxide dismutase (A), GSH-Px (B), and catalase (C) activity in a UVA-injured human skin fibroblasts model. Glutathione (GSH) was used as the positive control. All data are presented as mean ± SD (n = 3). ***P < 0.001, **P < 0.01, and *P < 0.05 vs. blank group; ###P < 0.001, ## P < 0.01, and #P < 0.05 vs. model group; +++P < 0.001 and ++P < 0.01 vs. UVA + GSH group.
Excess ROS generated from UVA exposure is linked to oxidative stress and damage to the skin, including loss of structural proteins, skin photoaging, DNA mutations, and even skin cancer (Ohba et al., 2016; Kuo et al., 2020). Therefore, excess ROS must be effectively eliminated by endogenous antioxidant defense systems, including antioxidants and antioxidant enzymes, to reduce the oxidative damage (Sila and Bougatef, 2016; Cai et al., 2019; Xiao et al., 2020; Wang et al., 2021). Presently, some bioactive natural products, such as crocetin (Ohba et al., 2016), caffeamide (Kuo et al., 2020), baicalin (Zhou et al., 2012), thymoquinone (Liang et al., 2021), and quercetin (Inal and Kahraman, 2000), show significant protective effects on cells and tissues by alleviating the oxidative and inflammatory responses induced by UVA. In addition, collagens, gelatins, and their derived peptides showed great potential in ameliorating skin photoaging induced by UVA (Pan et al., 2019; Zhang et al., 2020; Karnjanapratum and Benjakul, 2020; Li et al., 2022). The present results indicated that TCG could protect HSFBs against UVA injury by increasing the activity of antioxidant enzymes to scavenge excess ROS.
Conclusion
In the experiment, cartilage gelatin of Siberian sturgeon was prepared using the hot water method under optimized conditions using single-factor and orthogonal experiments. The TCG showed the main characteristics of gelatins, but its structure stability was lower than that of PSG because of the low content of imino acids. The TCG exhibited lower water content, gel strength, ESI, FC, FS, and WHC but higher ash content, transmittance, EAI, and FBC. More importantly, the TCG could significantly protect HSFBs against UVA injury by increasing the activity of antioxidant enzymes to scavenge excess ROS and decrease the content of MDA. The present results suggested that sturgeon by-products could be used to produce high-value-added functional products, and the prepared cartilage gelatin (TCG) might act as active ingredients applied in health-promoting products against UVA injury.
Data Availability Statement
The original contributions presented in the study are included in the article/supplementary material. Further inquiries can be directed to the corresponding authors.
Author Contributions
ZZ and Y-TQ: conceptualization, data curation, formal analysis, investigation, methodology, validation, and writing—original draft. Y-MW and C-FC: conceptualization, data curation, formal analysis, investigation, methodology, and validation. H-YL and BW: conceptualization, funding acquisition, resources, supervision, and writing—review and editing. All authors contributed to the article and approved the submitted version.
Funding
This work was funded by the National Natural Science Foundation of China (No. 82073764) and the Ten-Thousand Talents Plan of Zhejiang Province (No. 2019R52026).
Conflict of Interest
The authors declare that the research was conducted in the absence of any commercial or financial relationships that could be construed as a potential conflict of interest.
Publisher’s Note
All claims expressed in this article are solely those of the authors and do not necessarily represent those of their affiliated organizations, or those of the publisher, the editors and the reviewers. Any product that may be evaluated in this article, or claim that may be made by its manufacturer, is not guaranteed or endorsed by the publisher.
References
Ahmad T., Ismail A., Ahmad S. A., Khalil K. A., Awad E. A., Leoa T. K., et al. (2018). Characterization of Gelatin From Bovine Skin Extracted Using Ultrasound Subsequent to Bromelain Pretreatment. Food Hydrocolloid. 80, 264–273. doi: 10.1016/j.foodhyd.2018.01.036
Ahmed R., Haq M., Chun B. S. (2019). Characterization of Marine Derived Collagen Extracted From the by-Products of Bigeye Tuna (Thunnus Obesus). Int. J. Biol. Macromol. 135, 668–676. doi: 10.1016/j.ijbiomac.2019.05.213
Ali A., Wei S., Liu Z., Fan X., Sun Q., Xia Q., et al. (2021). Non-Thermal Processing Technologies for the Recovery of Bioactive Compounds From Marine by-Products. LWT 147, 111549. doi: 10.1016/j.lwt.2021.111549
Al-Nimry S., Dayah A. A., Hasan I., Daghmash R. (2021). Cosmetic, Biomedical and Pharmaceutical Applications of Fish Gelatin/Hydrolysates. Mar. Drugs 19 (3), 145. doi: 10.3390/md19030145
AOAC. Official Methods of Analysis of AOAC International, Gaithersburg M. D. (2003). Association of the Official Analytical Chemists (AOAC) International. 17th ed (Rockville: AOAC).
Bkhairia I., Mhamdi S., Jridi M., Nasri M. (2016). New Acidic Proteases From Liza Aurata Viscera: Characterization and Application in Gelatin Production. Int. J. Biol. Macromol. 92, 533–542. doi: 10.1016/j.ijbiomac.2016.07.063
Boran G., Regenstein J. M. (2010). Fish Gelatin. Adv. Food Nutr. Res. 60, 119–143. doi: 10.1016/S1043-4526(10)60005-8
BSI (British Standards Institution) (1975). Methods for Sampling and Testing Gelatin (Physical and Chemical Methods) (London: BSI).
Bu Y., Elango J., Zhang J., Bao B., Guo R., Palaniyandi K., et al. (2017). Immunological Effects of Collagen and Collagen Peptide From Blue Shark Cartilage on 6T-CEM Cells. Process. Biochem. 57, 219–227. doi: 10.1016/j.procbio.2017.04.008
Cai S. Y., Wang Y. M., Zhao Y. Q., Chi C. F., Wang B. (2019). Cytoprotective Effect of Antioxidant Pentapeptides From the Protein Hydrolysate of Swim Bladders of Miiuy Croaker (Miichthys Miiuy) Against H2O2-Mediated Human Umbilical Vein Endothelial Cell (HUVEC) Injury. Int. J. Mol. Sci. 20 (21), 5425. doi: 10.3390/ijms20215425
Cao S. M., Wang Y., Xing L. J., Zhang W. G., Zhou G. H. (2020). Structure and Physical Properties of Gelatin From Bovine Bone Collagen Influenced by Acid Pretreatment and Pepsin. Food Bioprod. Process. 121, 213–223. doi: 10.1016/j.fbp.2020.03.001
Cen S., Zhang L., Liu L., Lou Q., Wang C., Huang T. (2022). Phosphorylation Modification on Functional and Structural Properties of Fish Gelatin: The Effects of Phosphate Contents. Food Chem. 380, 132209. doi: 10.1016/j.foodchem.2022.132209
Chen T., Hou H. (2016). Protective Effect of Gelatin Polypeptides From Pacific Cod (Gadus Macrocephalus) Against UV Irradiation-Induced Damages by Inhibiting Inflammation and Improving Transforming Growth Factor-β/Smad Signaling Pathway. J. Photochem. Photobio. B. 162, 633–640. doi: 10.1016/j.jphotobiol.2016.07.038
Chen T., Hou H., Fan Y., Wang S., Chen Q., Si L., et al. (2016b). Protective Effect of Gelatin Peptides From Pacific Cod Skin Against Photoaging by Inhibiting the Expression of MMPs via MAPK Signaling Pathway. J. Photochem. Photobio. B. 165, 34–41. doi: 10.1016/j.jphotobiol.2016.10.015
Chi C., Hu F., Li Z., Wang B., Luo H. (2016). Influence of Different Hydrolysis Processes by Trypsin on the Physicochemical, Antioxidant, and Functional Properties of Collagen Hydrolysates From Sphyrna Lewini, Dasyatis Akjei, and Raja Porosa. J. Aquat. Food Prod. T. 25 (5/8), 616–632. doi: 10.1080/10498850.2014.898004
Chi C. F., Wang B., Li Z. R., Luo H. Y., Ding G. F. (2013). Characterization of Acid-Soluble Collagens From the Cartilages of Scalloped Hammerhead (Sphyrna Lewini), Red Stingray (Dasyatis Akajei), and Skate (Raja Porosa). Food Sci. Biotechnol. 22 (4), 909–916. doi: 10.1007/s10068-013-0163-0
Chi C. F., Wang B., Li Z. R., Luo H. Y., Ding G. F., Wu C. W. (2014). Characterization of Acid-Soluble Collagen From the Skin of Hammerhead Shark (Sphyrna Lewini). J. Food Biochem. 38 (2), 236–247. doi: 10.1111/jfbc.12042
Coppola D., Lauritano C., Palma Esposito F., Riccio G., Rizzo C., de Pascale D. (2021). Fish Waste: From Problem to Valuable Resource. Mar. Drugs 19 (2), 116. doi: 10.3390/md19020116
FAO (2020). The State of World Fisheries and Aquaculture 2020. (Rome: FAO Fisheries and Aquaculture Department).
Fawale O. S., Abuibaid A., Hamed F., Kittiphattanabawon P., Maqsood S. (2021). Molecular, Structural, and Rheological Characterization of Camel Skin Gelatin Extracted Using Different Pretreatment Conditions. Foods 10 (7), 1563. doi: 10.3390/foods10071563
Gęgotek A., Domingues P., Skrzydlewska E. (2018). Proteins Involved in the Antioxidant and Inflammatory Response in Rutin-Treated Human Skin Fibroblasts Exposed to UVA or UVB Irradiation. J. Dermatol. Sci. 90 (3), 241–252. doi: 10.1016/j.jdermsci.2018.02.002
Gelatin Manufacturers of Europe (GME) (2016) First-Class Raw Materials as a Matter of Cou-Rse. Available at: http://www.gelatine.org/en/about-gelatine/manufacturing/raw-materials.html.
Grand View Research (2016) Gelatin Market Size Expected to Reach $4.08 Billion by 2024. Available at: http://www.grandviewresearch.com/pressrelease/global-gelati-n-market.
Gui M., Song J., Zhang L., Wang S., Wu R., Ma C., et al. (2015). Chemical Characteristics and Antithrombotic Effect of Chondroitin Sulfates From Sturgeon Skull and Sturgeon Backbone. Carbohyd. Polym. 123, 454–460. doi: 10.1016/j.carbpol.2015.01.046
Huang C. Y., Wu T. C., Hong Y. H., Hsieh S. L., Guo H. R., Huang R. H. (2018). Enhancement of Cell Adhesion, Cell Growth, Wound Healing, and Oxidative Protection by Gelatins Extracted From Extrusion-Pretreated Tilapia (Oreochromis Sp.) Fish Scale. Molecules 23 (10), 2406. doi: 10.3390/molecules23102406
Inal M. E., Kahraman A. (2000). The Protective Effect of Flavonol Quercetin Against Ultraviolet a Induced Oxidative Stress in Rats. Toxicology 154 (1-3), 21–29. doi: 10.1016/S0300-483X(00)00268-7
Jeevithan E., Bao B., Bu Y., Zhou Y., Zhao Q., Wu W. (2014a). Type II Collagen and Gelatin From Silvertip Shark (Carcharhinus Albimarginatus) Cartilage: Isolation, Purification, Physicochemical and Antioxidant Properties. Mar. Drugs 12, 3852–3873. doi: 10.3390/md12073852
Jeevithan E., Wu W., Nanping W., Lan H., Bao B. (2014b). Isolation, Purification and Characterization of Pepsin Soluble Collagen Isolated From Silvertip Shark (Carcharhinus Albimarginatus) Skeletal and Head Bone. Process. Biochem. 49 (10), 1767–1777. doi: 10.1016/j.procbio.2014.06.011
Jellouli K., Balti R., Bougatef A., Hmidet N., Barkia A., Nasri M. (2011). Chemical Composition and Characteristics of Skin Gelatin From Grey Triggerfish (Balistes Capriscus). LWT-Food. Sci. Technol. 44 (9), 1965–1970. doi: 10.1016/j.lwt.2011.05.005
Kaewdang O., Benjakul S., Kaewmanee T., Kishimura H. (2014). Characteristics of Collagens From the Swim Bladders of Yellowfin Tuna (Thunnus Albacares). Food Chem. 155, 264–270. doi: 10.1016/j.foodchem.2014.01.076
Karnjanapratum S., Benjakul S. (2020). Asian Bullfrog (Rana Tigerina) Skin Gelatin Extracted by Ultrasound-Assisted Process: Characteristics and in-Vitro Cytotoxicity. Int. J. Biol. Macromol. 148, 391–400. doi: 10.1016/j.ijbiomac.2020.01.150
Kim T. K., Ham Y. K., Shin D. M., Kim H. W., Jang H. W., Kim Y. B., et al. (2020). Extraction of Crude Gelatin From Duck Skin: Effects of Heating Methods on Gelatin Yield. Poultry. Sci. 99 (1), 590–596. doi: 10.3382/ps/pez519
Kittiphattanabawon P., Benjakul S., Visessanguan W., Shahidi F. (2010). Isolation and Characterization of Collagen From the Cartilages of Brownbanded Bamboo Shark (Chiloscyllium Punctatum) and Blacktip Shark (Carcharhinus Limbatus). LWT. Food Sci. Technol. 43 (5), 792–800. doi: 10.1016/j.lwt.2010.01.006
Kuo Y. H., Chiang H. L., Wu P. Y., Chu Y., Chang Q. X., Wen K. C., et al. (2020). Protection Against Ultraviolet a-Induced Skin Apoptosis and Carcinogenesis Through the Oxidative Stress Reduction Effects of N-(4-Bromophenethyl) Caffeamide, a Propolis Derivative. Antioxidants. (Basel). 9 (4), 335. doi: 10.3390/antiox9040335
Lai C. S., Tu C. W., Kuo H. C., Sun P. P., Tsai M. L. (2020). Type II Collagen From Cartilage of Acipenser Baerii Promotes Wound Healing in Human Dermal Fibroblasts and in Mouse Skin. Mar. Drugs 18 (10), 511. doi: 10.3390/md18100511
Liang J., Lian L., Wang X., Li L. (2021). Thymoquinone, Extract From Nigella Sativa Seeds, Protects Human Skin Keratinocytes Against UVA-Irradiated Oxidative Stress, Inflammation and Mitochondrial Dysfunction. Mol. Immunol. 135, 21–27. doi: 10.1016/j.molimm.2021.03.015
Li X. R., Chi C. F., Li L., Wang B. (2017). Purification and Identification of Antioxidant Peptides From Protein Hydrolysate of Scalloped Hammerhead (Sphyrna Lewini) Cartilage. Mar. Drugs 15 (3), 61. doi: 10.3390/md15030061
Li C., Fu Y., Dai H., Wang Q., Gao R., Zhang Y. (2022). Recent Progress in Preventive Effect of Collagen Peptides on Photoaging Skin and Action Mechanism. Food Sci. Hum. Well. 11 (2), 218–229. doi: 10.1016/j.fshw.2021.11.003
Lin P., Hua N., Hsu Y. C., Kan K. W., Chen J. H., Lin Y. H., et al. (2020). Oral Collagen Drink for Antiaging: Antioxidation, Facilitation of the Increase of Collagen Synthesis, and Improvement of Protein Folding and DNA Repair in Human Skin Fibroblasts. Oxid. Med. Cell Longev. 2020, 8031795. doi: 10.1155/2020/8031795
Li Z., Wang B., Chi C., Gong Y., Luo H., Ding G. (2013). Influence of Average Molecular Weight on Antioxidant and Functional Properties of Cartilage Collagen Hydrolysates From Sphyrna Lewini, Dasyatis Akjei and Raja Porosa. Food Res. Int. 51 (1), 283–293. doi: 10.1016/j.foodres.2012.12.031
Li Z. R., Wang B., Chi C. F., Zhang Q. H., Gong Y. D., Tang J. J., et al. (2013a). Isolation and Characterization of Acid Soluble Collagens and Pepsin Soluble Collagens From the Skin and Bone of Spanish Mackerel (Scomberomorous Niphonius). Food Hydrocolloid. 31 (1), 103–113. doi: 10.1016/j.foodhyd.2012.10.001
Li L. Y., Zhao Y. Q., He Y., Chi C. F., Wang B. (2018). Physicochemical and Antioxidant Properties of Acid-and Pepsin-Soluble Collagens From the Scales of Miiuy Croaker (Miichthys Miiuy). Mar. Drugs 16 (10), 394. doi: 10.3390/md16100394
Lone A. N., Malik A. T., Naikoo H. S., Raghu R. S., Tasduq S. A. (2020). Trigonelline, a Naturally Occurring Alkaloidal Agent Protects Ultraviolet-B (UV-B) Irradiation Induced Apoptotic Cell Death in Human Skin Fibroblasts via Attenuation of Oxidative Stress, Restoration of Cellular Calcium Homeostasis and Prevention of Endoplasmic Reticulum (ER) Stress. J. Photoch. Photobio. B. 202, 111720. doi: 10.1016/j.jphotobiol.2019.111720
Luo Q. B., Chi C. F., Yang F., Zhao Y. Q., Wang B. (2018). Physicochemical Properties of Acid-and Pepsin-Soluble Collagens From the Cartilage of Siberian Sturgeon. Environ. Sci. pollut. R. 25 (31), 31427–31438. doi: 10.1007/s11356-018-3147-z
Lv L. C., Huang Q. Y., Ding W., Xiao X. H., Zhang H. Y., Xiong L. X. (2019). Fish Gelatin: The Novel Potential Applications. J. Funct. Foods. 63, 103581. doi: 10.1016/j.jff.2019.103581
Mutilangi W. A. M., Panyam D., Kilara A. (1996). Functional Properties of Hydrolysates From Proteolysis of Heat-Denatured Whey Protein Isolate. J. Food Sci. 61 (2), 270–275. doi: 10.1111/j.1365-2621.1996.tb14174.x
Nag M., Lahiri D., Dey A., Sarkar T., Pati S., Joshi S., et al. (2022). Seafood Discards: A Potent Source of Enzymes and Biomacromolecules With Nutritional and Nutraceutical Significance. Front. Nutr. 9, 879929. doi: 10.3389/fnut.2022.879929
Nisar U., Peng D., Mu Y., Sun Y. (2022). A Solution for Sustainable Utilization of Aquaculture Waste: A Comprehensive Review of Biofloc Technology and Aquamimicry. Front. Nutr. 8, 791738. doi: 10.3389/fnut.2021.791738
Nurilmala M., Hizbullah H. H., Karnia E., Kusumaningtyas E., Ochiai Y. (2020). Characterization and Antioxidant Activity of Collagen, Gelatin, and the Derived Peptides From Yellowfin Tuna (Thunnus Albacares) Skin. Mar. Drugs 18 (2), 98. doi: 10.3390/md18020098
Ohba T., Ishisaka M., Tsujii S., Tsuruma K., Shimazawa M., Kubo K., et al. (2016). Crocetin Protects Ultraviolet A-Induced Oxidative Stress and Cell Death in Skin In Vitro and In Vivo. Eur. J. Pharmacol. 789, 244–253. doi: 10.1016/j.ejphar.2016.07.036
Pan J., Li Q., Jia H., Xia L., Jin W., Shang M., et al. (2018). Physiochemical and Functional Properties of Tiger Puffer (Takifugu Rubripes) Skin Gelatin as Affected by Extraction Conditions. Int. J. Biol. Macromol. 109, 1045–1053. doi: 10.1016/j.ijbiomac.2017.11.080
Pan X. Y., Wang Y. M., Li L., Chi C. F., Wang B. (2019). Four Antioxidant Peptides From Protein Hydrolysate of Red Stingray (Dasyatis Akajei) Cartilages: Isolation, Identification, and In Vitro Activity Evaluation. Mar. Drugs 17 (5), 263. doi: 10.3390/md17050263
Pan X., Zhao Y. Q., Hu F. Y., Chi C. F., Wang B. (2016). Anticancer Activity of a Hexapeptide From Skate (Raja Porosa) Cartilage Protein Hydrolysate in HeLa Cells. Mar. Drugs 14 (8), 153. doi: 10.3390/md14080153
Pan X., Zhao Y. Q., Hu F. Y., Wang B. (2016). Preparation and Identification of Antioxidant Peptides From Protein Hydrolysate of Skate (Raja Porosa) Cartilage. J. Funct. Foods. 25, 220–230. doi: 10.1016/j.jff.2016.06.008
Qiu Y. T., Wang Y. M., Yang X. R., Zhao Y. Q., Chi C. F., Wang B. (2019). Gelatin and Antioxidant Peptides From Gelatin Hydrolysate of Skipjack Tuna (Katsuwonus Pelamis) Scales: Preparation, Identification and Activity Evaluation. Mar. Drugs 17 (10), 565. doi: 10.3390/md17100565
Raju N., Gulzar S., Buamard N., Ma L., Ying X., Zhang B., et al. (2021). Comparative Study of Astaxanthin, Cholesterol, Fatty Acid Profiles, and Quality Indices Between Shrimp Oil Extracted From Hepatopancreas and Cephalothorax. Front. Nutr. 8, 803664. doi: 10.3389/fnut.2021.803664
Regenstein J. M., Zhou P. (2007). “Collagen and Gelatin From Marine By-Products,” in Maximizing the Value of Marine by-Products. Ed. Shahidi F. (Cambridge: Woodhead Publishing Limited), 279–303.
Shyni K., Hema G. S., Ninan G., Mathew S., Joshy C. G., Lakshmanan P. T. (2014). Isolation and Characterization of Gelatin From the Skins of Skipjack Tuna (Katsuwonus Pelamis), Dog Shark (Scoliodon Sorrakowah), and Rohu (Labeo Rohita). Food Hydrocolloid. 39, 68–76. doi: 10.1016/j.foodhyd.2013.12.008
Sila A., Bougatef A. (2016). Antioxidant Peptides From Marine by-Products: Isolation, Identification and Application in Food Systems. A. review. J. Funct. Foods. 21, 10–26. doi: 10.1016/j.jff.2015.11.007
Sinthusamran S., Benjakul S., Hemar Y. (2018). Physical and Sensory Properties of Gelatin From Seabass (Lates Calcarifer) as Affected by Agar and κ-Carrageenan. J. Texture. Stud. 49 (1), 47–55. doi: 10.1111/jtxs.12280
Stani C., Vaccari L., Mitri E., Birarda G. (2020). FTIR Investigation of the Secondary Structure of Type I Collagen: New Insight Into the Amide III Band. Spectrochim Acta A. 229, 118006. doi: 10.1016/j.saa.2019.118006
Standardization Administration of China. (2013). National Food Industry Standardization Technical Committee of China. Food Additive - Gelatin (GB6783-2013) (Beijing: Standardization Administration of China).
Sun L., Zhang Y., Zhuang Y. (2013). Antiphotoaging Effect and Purification of an Antioxidant Peptide From Tilapia (Oreochromis Niloticus) Gelatin Peptides. J. Funct. Foods. 5 (1), 154–162. doi: 10.1016/j.jff.2012.09.006
Tao J., Zhao Y. Q., Chi C. F., Wang B. (2018). Bioactive Peptides From Cartilage Protein Hydrolysate of Spotless Smoothhound and Their Antioxidant Activity. Vitro. Mar. Drugs 16, 100. doi: 10.3390/md16040100
Tkaczewska J., Morawska M., Kulawik P., Zając M. (2018). Characterization of Carp (Cyprinus Carpio) Skin Gelatin Extracted Using Different Pretreatments Method. Food Hydrocolloid. 81, 169–179. doi: 10.1016/j.foodhyd.2018.02.048
Uddin S. M. K., Hossain M. A. M., Sagadevan S., Amin M. A., Johan M. R. (2021). Halal and Kosher Gelatin: Applications as Well as Detection Approaches With Challenges and Prospects. Food Biosci. 44, 101422. doi: 10.1016/j.fbio.2021.101422
Valcarcel J., Hermida-Merino C., Piñeiro M. M., Hermida-Merino D., Vázquez J. A. (2021). Extraction and Characterization of Gelatin From Skin by-Products of Seabream, Seabass and Rainbow Trout Reared in Aquaculture. Int. J. Mol. Sci. 22 (22), 12104. doi: 10.3390/ijms222212104
van der Ven C., Gruppen H., de Bont D. B. A., Voragen A. G. J. (2002). Correlations Between Biochemical Characteristics and Foam-Forming and-Stabilizing Ability of Whey and Casein Hydrolysates. J. Agr. Food Chem. 50 (10), 2938–2946. doi: 10.1021/jf011190f
Wang Y. M., Li X. Y., Wang J., He Y., Chi C. F., Wang B. (2022). Antioxidant Peptides From Protein Hydrolysate of Skipjack Tuna Milt: Purification, Identification, and Cytoprotection on H2O2 Damaged Human Umbilical Vein Endothelial Cells. Process. Biochem. 113, 258–269. doi: 10.1016/j.procbio.2022.01.008
Wang B., Wang Y. M., Chi C. F., Luo H. Y., Deng S. G., Ma J. Y. (2013). Isolation and Characterization of Collagen and Antioxidant Collagen Peptides From Scales of Croceine Croaker (Pseudosciaena Crocea). Mar. Drugs 11 (11), 4641–4661. doi: 10.3390/md11114641
Wang J., Wang Y. M., Li L. Y., Chi C. F., Wang B. (2022). Twelve Antioxidant Peptides From Protein Hydrolysate of Skipjack Tuna (Katsuwonus Pelamis) Roe Prepared by Flavourzyme: Purification, Sequence Identification, and Activity Evaluation. Front. Nutr. 8, 813780. doi: 10.3389/fnut.2021.813780
Wang Y. Z., Wang Y. M., Pan X., Chi C. F., Wang B. (2021). Antioxidant Mechanisms of the Oligopeptides (FWKVV and FMPLH) From Muscle Hydrolysate of Miiuy Croaker Against Oxidative Damage of HUVECs. Oxid. Med. Cell Longev. 6C), 1–15. doi: 10.1155/2021/9987844
Wang T., Zhang S., Ren S., Zhang X., Yang F., Chen Y., et al. (2020). Structural Characterization and Proliferation Activity of Chondroitin Sulfate From the Sturgeon, Acipenser Schrenckii. Int. J. Biol. Macromol. 164, 3005–3011. doi: 10.1016/j.ijbiomac.2020.08.110
Wu R., Li P., Wang Y., Su N., Xiao M., Li X., et al. (2022). Structural Analysis and Anti-Cancer Activity of Low-Molecular-Weight Chondroitin Sulfate From Hybrid Sturgeon Cartilage. Carbohyd. Polym. 275, 118700. doi: 10.1016/j.carbpol.2021.118700
Xiao L., Liao F., Fan Y., Miwa N. (2020). Enzyme-Digested Colla Corii Asini (E'jiao) Accelerates Wound Healing and Prevents Ultraviolet a-Induced Collagen Synthesis Decline and Wrinkle Formation in Three-Dimensional Skin Equivalents. Hum. Cell. 33 (4), 1056–1067. doi: 10.1007/s13577-020-00405-y
Yang X. R., Zhao Y. Q., Qiu Y. T., Chi C. F., Wang B. (2019). Preparation and Characterization of Gelatin and Antioxidant Peptides From Gelatin Hydrolysate of Skipjack Tuna (Katsuwonus Pelamis) Bone Stimulated by In Vitro Gastrointestinal Digestion. Mar. Drugs 17 (2), 78. doi: 10.3390/md17020078
Yuan L., Chu Q., Wu X., Yang B., Zhang W., Jin W., et al. (2021). Anti-Inflammatory and Antioxidant Activity of Peptides From Ethanol-Soluble Hydrolysates of Sturgeon (Acipenser Schrenckii) Cartilage. Front. Nutr. 8, 689648. doi: 10.3389/fnut.2021.689648
Yu D., Chi C. F., Wang B., Ding G. F., Li Z. R. (2014). Characterization of Acid-and Pepsin-Soluble Collagens From Spines and Skulls of Skipjack Tuna (Katsuwonus Pelamis). Chin. J. Nat. Medicines. 12 (9), 712–720. doi: 10.1016/S1875-5364(14)60110-2
Zhang L., Zhao G. X., Zhao Y. Q., Qiu Y. T., Chi C. F., Wang B. (2019). Identification and Active Evaluation of Antioxidant Peptides From Protein Hydrolysates of Skipjack Tuna (Katsuwonus Pelamis) Head. Antioxidants. (Basel). 8 (8), 318. doi: 10.3390/antiox8080318
Zhang Z., Zhu H., Zheng Y., Zhang L., Wang X., Luo Z., et al. (2020). The Effects and Mechanism of Collagen Peptide and Elastin Peptide on Skin Aging Induced by D-Galactose Combined With Ultraviolet Radiation. J. Photoch. Photobio. B. 210 (8), 111964. doi: 10.1016/j.jphotobiol.2020.111964
Zhao W. H., Chi C. F., Zhao Y. Q., Wang B. (2018a). Preparation, Physicochemical and Antioxidant Properties of Acid-and Pepsin-Soluble Collagens From the Swim Bladders of Miiuy Croaker (Miichthys Miiuy). Mar. Drugs 16 (5), 161. doi: 10.3390/md16050161
Zhao W. H., Luo Q. B., Pan X., Chi C. F., Sun K. L., Wang B. (2018). Preparation, Identification, and Activity Evaluation of Ten Antioxidant Peptides From Protein Hydrolysate of Swim Bladders of Miiuy Croaker (Miichthys Miiuy). J. Funct. Foods. 47, 503–511. doi: 10.1016/j.jff.2018.06.014
Zheng Y. Y., Ma Y., Luo Y. K., Li B. (2017). Effect of Extraction Methods and Transglutaminase Modification on Film Properties and Structure of Gelatin. Food Sci. 38 (19), 92–99. doi: 10.7506/spkx1002-6630-201719016
Zhou B. R., Yin H. B., Xu Y., Wu D., Zhang Z. H., Yin Z. Q., et al. (2012). Baicalin Protects Human Skin Fibroblasts From Ultraviolet a Radiation-Induced Oxidative Damage and Apoptosis. Free Radical Res. 6 (12), 1458–1471. doi: 10.3109/10715762.2012.726355
Keywords: Siberian sturgeon (Acipenser baerii), cartilage, gelatin, cytoprotection, ultraviolet-A injury
Citation: Zhang Z, Wang Y-M, Qiu Y-T, Chi C-F, Luo H-Y and Wang B (2022) Gelatin From Cartilage of Siberian Sturgeon (Acipenser baerii): Preparation, Characterization, and Protective Function on Ultraviolet-A-Injured Human Skin Fibroblasts. Front. Mar. Sci. 9:925407. doi: 10.3389/fmars.2022.925407
Received: 21 April 2022; Accepted: 18 May 2022;
Published: 14 June 2022.
Edited by:
Haibin Tong, Wenzhou University, ChinaReviewed by:
Zhongshan Zhang, Huzhou University, ChinaShan He, Ningbo University, China
Pinglin Li, Ocean University of China, China
Copyright © 2022 Zhang, Wang, Qiu, Chi, Luo and Wang. This is an open-access article distributed under the terms of the Creative Commons Attribution License (CC BY). The use, distribution or reproduction in other forums is permitted, provided the original author(s) and the copyright owner(s) are credited and that the original publication in this journal is cited, in accordance with accepted academic practice. No use, distribution or reproduction is permitted which does not comply with these terms.
*Correspondence: Bin Wang, d2FuZ2JpbjQxNTlAaG90bWFpbC5jb20=; Hong-Yu Luo, bGlzYTg5MTlAMTYzLmNvbQ==