- 1Biology Department, Woods Hole Oceanographic Institution, Woods Hole, MA, United States
- 2Sound Ocean Science, Calgary, AB, Canada
The ocean’s soundscape is fundamental to marine ecosystems, not only as a source of sensory information critical to many ecological processes but also as an indicator of biodiversity and habitat health. Yet, little is known about how ecoacoustic activity in marine habitats is altered by environmental changes such as temperature. The sounds produced by dense colonies of snapping shrimp dominate temperate and tropical coastal soundscapes worldwide and are a major driver broadband sound pressure level (SPL) patterns. Field recordings of soundscape patterns from the range limit of a snapping shrimp distribution showed that rates of snap production and associated SPL were closely positively correlated to water temperature. Snap rates changed by 15-60% per °C change in regional temperature, accompanied by fluctuations in SPL between 1-2 dB per °C. To test if this relationship was due to a direct effect of temperature, we measured snap rates in controlled experiments using two snapping shrimp species dominant in the Western Atlantic Ocean and Gulf of Mexico (Alpheus heterochaelis and A. angulosus). Snap rates were measured for shrimp held at different temperatures (across 10-30 °C range, with upper limit 2°C above current summer mean temperatures) and under different social groupings. Temperature had a significant effect on shrimp snap rates for all social contexts tested (individuals, pairs, and groups). For individuals and shrimp groups, snap production more than doubled between mid-range (20°C) and high (30°C) temperature treatments. Given that snapping shrimp sounds dominate the soundscapes of diverse habitats, including coral reefs, rocky bottoms, seagrass, and oyster beds, the strong influence of temperature on their activity will potentially alter soundscape patterns broadly. Increases in ambient sound levels driven by elevated water temperatures has ecological implications for signal detection, communication, and navigation in key coastal ecosystems for a wide range of organisms, including humans.
Introduction
The underwater acoustic environment, known as the soundscape, is fundamental to the survival and reproduction of a wide variety of marine taxa, from microscopic invertebrate larvae to the largest mammals on Earth (Au and Hastings, 2008; Cotter, 2008; Duarte et al., 2021). Soundscapes reflect and influence a range of key life history processes including communication, reproduction, navigation, and predation (Hildebrand, 2009; Pijanowski et al., 2011). The mosaic of acoustic signals that create a soundscape reflects the biological, geophysical, and anthropogenic features of a given location, and thus transmits rich sensory information to organisms about the composition and distribution of the local habitat, community, and resources. As in terrestrial ecosystems, these acoustic cues and signals are critical to myriad ecological processes in the marine environment and changes to that acoustic environment threatens many vital activities.
Substantial changes in natural soundscapes have occurred due to increases in human use (Slabbekoorn et al., 2010; Butler et al., 2016; Buxton et al., 2017; Duarte et al., 2021), and a recent body of research has focused on anthropogenic noise and its detrimental impacts on organism physiology and behavior (e.g., Luczkovich and Sprague, 2008; de Soto et al., 2013; Hawkins and Popper, 2017; Jones et al., 2020). Increasing attention is also being given to soundscape measurement and analysis as an approach to monitor the impacts of human disturbance and climate change on biodiversity (Gage and Axel, 2014; Krause and Farina, 2016; Lamont et al., 2022). Far less is known about climate-driven changes to the soundscape and the biological drivers of those potential alterations, particularly for acoustically-rich ocean habitats. Both human-produced noise pollution and environmentally-dependent animal acoustic output shifts could induce cascading impacts on ecosystems by altering the navigation, reproduction, and trophic dynamics of species and communities (Sueur et al., 2019; Duarte et al., 2021).
Changes in climate-driven environmental variables in marine systems (e.g., temperature, pH, dissolved oxygen) are likely to affect ambient soundscape patterns by altering the output of dominant sound producers, however, these relationships and their broader impacts on ocean soundscapes are largely unknown. Climate has been observed to drive the sound production of terrestrial and marine animals by regulating their physiology, biochemistry, and phenology (Sueur et al., 2019). Many studies have shown positive relationships between temperature and call rates in terrestrial ectotherms, such as insects and frogs [reviewed in (Gerhardt and Huber 2002)]. In marine environments, the influence of temperature on acoustic communication has been examined for a number of vocalizing fish (Connaughton et al. 2000), but these studies have focused on thermal dependency of call characteristics and acoustic ecology of the species [e.g., toadfish fundamental frequency varies with temperature (Bass and McKibben 2003)], and not within the context of soundscapes or population-level sound production. Future climate change scenarios are also expected to impact physical sound propagation properties, with implications for endangered marine mammal communication (Affatati et al., 2022).
Snapping shrimp, also referred to as pistol shrimp, produce one of the most pervasive of all biological underwater sounds (Johnson et al. 1947). Individually, these small crustaceans (mm to cm in length) generate a unique high intensity acoustic signal via the collapse of a cavitation bubble during rapid closure of a specialized claw (Versluis et al., 2000). Snapping shrimp typically live in dense aggregations hidden within the substrate of many coastal ecosystems (e.g., coral reef, sponges, rubble, oyster beds, rocky shores), together producing a continuous audible crackling sound (Johnson et al. 1947; Mathews, 2007). Snapping shrimp acoustic activity is often the major determinant of ambient sound levels in coastal seas (Everest et al, 1948; Johnson et al, 1947; Lillis et al, 2014; Kaplan et al, 2015), and variation in snapping shrimp acoustic output has been found to account for much spatiotemporal soundscape variation, both in sound levels and frequency content, within temperate and tropical benthic marine ecosystems (Radford et al. 2008; Radford et al. 2010; Lillis et al. 2014; Staaterman et al. 2014; Butler et al. 2017; Lee et al., 2021). This spatiotemporal variability of snapping shrimp sound production likely influence a range of acoustically-mediated animal activities, including navigation and habitat selection by settlement-stage fish and invertebrate larvae (Simpson et al., 2008; Lillis et al, 2018; Lillis et al, 2013), and perhaps even functions as an auditory cue for migrating cetaceans to avoid rocky shorelines (Allen, 2013). Snapping shrimp sound is also known to interfere with other important acoustic signals, an underwater communication problem for humans and marine organisms alike (Au and Banks, 1998; Chitre et al. 2006; Hildebrand, 2009). Indeed, early descriptions of snapping shrimp sounds stemmed from investigation by the US Navy as their persistent sounds can impair sonars and signal detection, but also may be leveraged for target imaging (Buckingham et al. 1996; Au and Penner 1981). Despite these examples of their importance to marine ecosystems and human endeavor in the sea, snapping shrimp acoustic ecology and environmental variables underlying their sound production patterns are not well studied.
Recent improvements in underwater sound recording ability and increased efforts to sample habitat soundscapes at high spatiotemporal resolution have generated datasets that reveal complex dynamics in snapping shrimp sound production (Lammers et al., 2008; Staaterman et al., 2014; Lillis and Mooney, 2016), and provide evidence that snapping-dominated soundscape patterns are related to temperature, as well as light, pH, currents, and dissolved oxygen (Bohnenstiehl et al., 2016; Lillis and Mooney, 2018; Rossi et al., 2016; Lee et al., 2021). Seasonal effects of temperature on snapping patterns in field recordings had previously been reported in temperate regions (Johnson, 1947), and more recent datasets from tropical regions similarly demonstrate correlations between water temperature and snapping over smaller time scales (days) and temperature changes (1-2°C) (Lillis and Mooney, 2016). However, the fundamental relationship between temperature and snapping shrimp acoustic activity has not been directly examined, nor have the effects of temperature on Alpheid shrimp acoustic behavior been tested.
Here, we investigated the relationship between snapping shrimp-dominated marine soundscapes and seawater temperature to better understand how warming trends and temperature anomalies may influence acoustic properties of coastal ecosystems. The aim of this study was to test the dependence of snapping shrimp-dominated marine soundscapes on water temperature to better understand how warming may influence acoustic properties in coastal habitats. First, we analyzed previous soundscape recordings collected at high temporal-resolution to closely examine in situ relationships between snap rates, sound pressure levels, and water temperature. Based on these observations, we tested the effects of water temperature on snapping patterns in a controlled laboratory setting for the dominant Alpheid species widespread in coastal and estuarine reef habitats of the Southeast United States and Gulf of Mexico (Alpheus heterochaelis and Alpheus angulosus) (Spence and Knowlton, 2008; Hughes et al, 2014). We compared the sound emission rates of shrimp under temperature treatments within their natural range (10-30°C) across different social conditions (i.e., isolated, in the presence of opposite sex shrimp, and for multiple shrimp groups) and different times of day, to gain insight into the interaction between temperature and snap context as a potential explanation for temporal variation in sound production by these animals.
Materials and methods
Soundscape field recordings and analysis
To investigate the relationship between observed snapping shrimp soundscape content and water temperature, we examined the snapping activity in three sets of ~8-week-long field recordings previously collected for an oyster reef soundscape study in West Bay Marine Reserve, Pamlico Sound, North Carolina, USA (34° 58.8517’ N 76° 21.3632’W) during 2012. The audio files, available in the BCO-DMO online data repository (Eggleston and Bohnenstiehl, 2015; Bohnenstiehl et al., 2016), were collected using a DSG acoustic recorder (Loggerhead Instruments) sampling for 1 minute every 15 minutes at 50 kHz, deployed at 3 m depth on the seafloor adjacent to oyster reef habitat. Deployments for analysis were selected from periods where Pamlico Bay water temperatures fluctuated by 5-15°C (Spring: 06 March–30 April; Early Summer: 12 May–6 July; Autumn: 21 September–27 November), and together included the full range of temperatures experienced at the site (~10-29°C). To quantify snapping activity, an automatic snap detection algorithm was applied (1.5-20 kHz band, 120 dB threshold), generating a snap rate (in detections per minute) for each file (Bohnenstiehl et al., 2016). This snap detection method, fully described in Bohnenstiehl et al. (2016) and further applied in Lillis & Mooney (2018), combines an amplitude threshold and correlation detector to enumerate signals that match the shape of snaps manually detected. The root-mean-square sound pressure level (SPL; 1.5-20 kHz band) was also calculated for each 1-minute sample. Water temperature data for the deployment periods were not available for the specific recording site in West Bay reserve, however, temperature records were obtained from the NOAA monitoring buoy in Hatteras, NC, the regional water quality monitoring station within Pamlico Sound closest to the recording site, where measurements are collected every 6 minutes (https://tidesandcurrents.noaa.gov/met.html?id=8654467). To enable comparisons between water temperature and snapping activity, hourly averages of acoustic data and temperatures were generated (n=4156). The strength of the relationships between the snap rate and water temperature was tested using a linear correlation function in Matlab (v.9.1, Mathworks, Cambridge, MA, USA), and regressions parameterized via a least-squares approximation.
Laboratory snapping shrimp experiments
A series of experiments using wild-caught snapping shrimp were conducted at the Woods Hole Oceanographic Institution (WHOI) Environmental Systems Laboratory (Woods Hole MA, USA) facility in September 2015, between June and November 2016, and in March 2017, to examine the effect of temperature on snapping shrimp sound production rates and to identify patterns under different social contexts (solitary, pairs, groups) in simulated natural conditions (summarized in Table 1). In the first experiment, using 24 shrimp (all A. heterochaelis), the effect of water temperature treatments of 10°C, 15°C, 20°C, and 25°C (n=6 per treatment) on individual spontaneous snap rates was tested. A second larger experiment was conducted with 60 individual shrimp (42 A. heterochaelis, 18 A. angulosus) and 54 pairs of shrimp (36 pairs of A. heterochaelis, 18 pairs of A. angulosus) testing the effect of water temperatures of 10°C, 20°C, and 30°C. Finally, an experiment was carried out to compare snap rates for three mesocosm groups of 10 shrimp each (5 male and 5 female A. heterochaelis each) exposed to 10°C, 20°C, and 30°C temperature treatments in different sequences (repeated measures design).
Snapping shrimp were collected by hand in coastal North Carolina, USA, during low tides at oyster bed areas in two locations close to Duke Marine Laboratory in Beaufort, within the North River Estuary and the shoreline of Pivers Island. In 2015, collections consisted of A. heterochaelis only, while 2016 collections included a small number of A. angulosus individuals. At WHOI seawater facilities, shrimp were housed individually in an enclosed outdoor laboratory (ambient light-cycle), where they each were provided a 1-L mesh-sided container with shells and gravel as shelter within a larger shaded flow-through seawater table maintained at 20°C outside of experimental periods and fed rations of dried shrimp pellets every third day.
Every trial measuring snap rates of individuals or pairs consisted of six or twelve replicate experimental units arranged within a 2.0 m x 1.0 m seawater table, randomly assigned to a temperature treatment at the onset of each trial (Figure 1). The 2-L monitoring chambers were placed on individual closed-cell neoprene foam mats and separated by sound-absorbing open-cell convoluted acoustic foam panels to avoid sound transfer between experimental units. Chambers contained gravel and shells as substrate, consistent with the containers in which shrimp were held prior to trials. Tank configuration changed slightly between Experiments 1 and 2 – where the initial experiment used flow-through seawater at each temperature, the latter experiment required the use of recirculating water baths to achieve stability for the desired highest water temperature treatment.
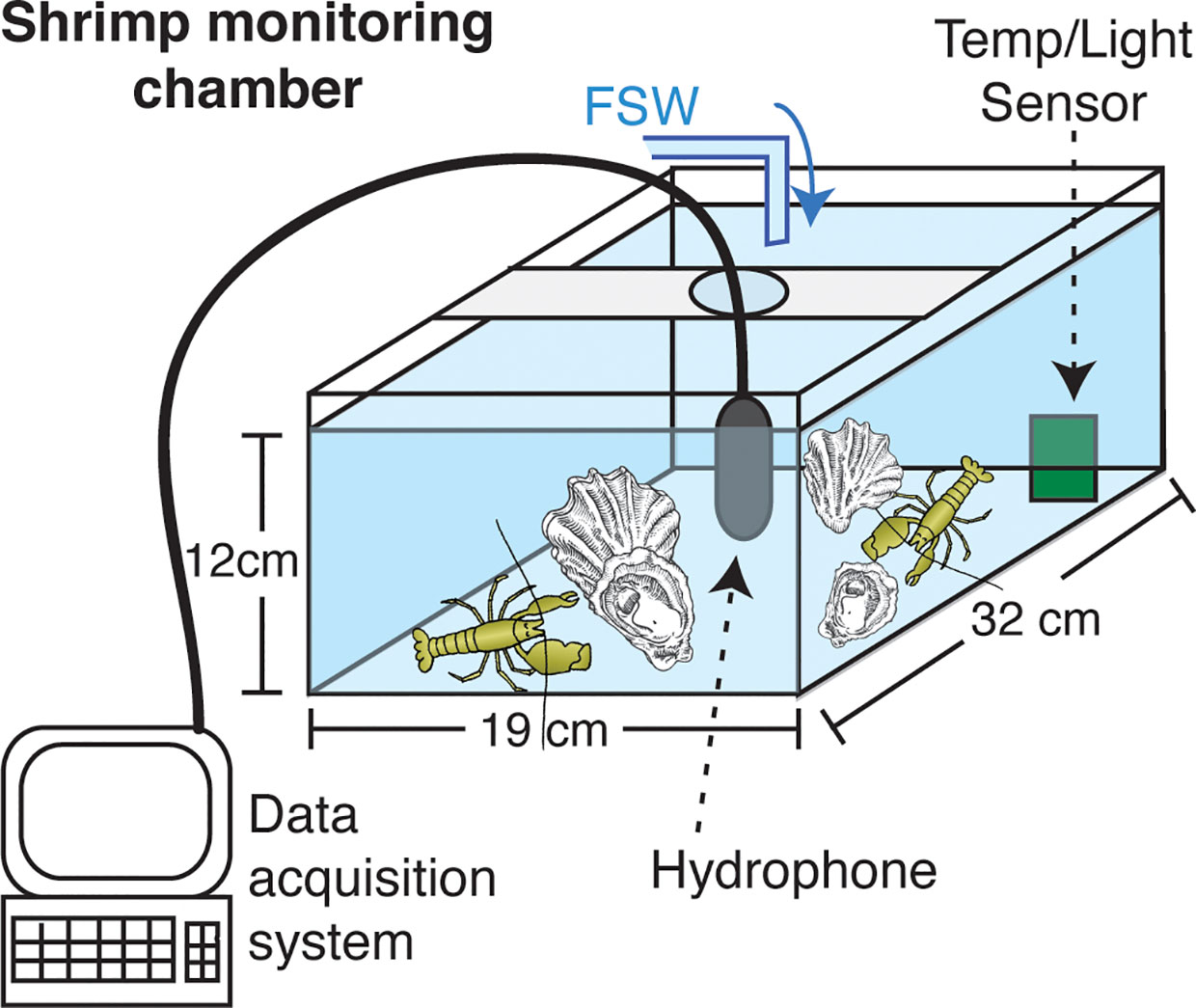
Figure 1 Experimental chamber set-up for acoustic monitoring of snapping shrimp under different temperature treatments. Schematic shows example of tank used in Experiments 1, which was slightly modified for Experiment 2 to use a recirculating water bath rather than flow-through to achieve desired temperatures. Experiment 3 was conducted with the same data acquisition and overall set-up but in larger seawater tables to accommodate 10 shrimp and sheltering habitat. Sample sizes, treatments, and groupings for each experiment appear in Table 1.
Acoustic measurements were made continuously during trials in all experimental tanks using HTI-96-min hydrophones (High-Tech Inc., Gulfport, MS, USA; sensitivity: -165 dB re:1V/µPa, flat frequency response: ~0.1–30 kHz) recording continuously at a 10 kHz sampling rate, acquiring data in 5-minute samples via a data acquisition device (16-bit, NI USB-6343, National Instruments, Austin, TX, USA) connected to a laptop running purpose-written Matlab acquisition code. Experimental tanks were also monitored with a HOBO pendant light and temperature sensor (Onset Computer Corporation, Bourne, MA, USA) logging at 1-minute intervals.
In each trial of Experiments 1 and 2, an individual or opposite-sex pair of shrimp was randomly selected and assigned to a single experimental chamber (Table 1; Figure 1). All chambers were initially held at 20°C, and a low flow of seawater at each temperature adjusted the tanks to 10°C and 30°C treatment levels over ~1 hour following introduction (20°C treatments were realized without manipulation). Trial start times occurred once all temperature treatment levels had been attained. The recording system was initiated after acclimation of shrimp and continued undisturbed for the length of the trial. While the 1-hour temperature change in Experiments 1 and 2 represents a more rapid rate of fluctuation than shrimp would experience in field conditions, preliminary observations of shrimp during temperature manipulation showed no acute thermal stress (e.g., movement from water input flow or mortality following temperature manipulation) and changes to snap rates were consistent throughout the trial period (i.e., snaps did not occur immediately in response to sudden temperature change).
In Experiment 3, three groups of 10 A. heterochaelis snapping shrimp (five female and five male) were randomly selected and placed in 75-L shallow mesocosm tanks (115 x 48 x 15 cm). Each tank contained sand and gravel substrate covered with larger cobble and oyster shells to provide plentiful sheltering material for shrimp and was supplied with flow-through seawater. Three sequential trials were carried out to expose each colony of shrimp to three temperature treatments (10, 20, 30°C). Each replicate tank was exposed to the three temperatures in a different sequence so that each temperature was represented in every trial. Prior to the first trial, each tank was randomly assigned one of the three temperature treatment orders, and a low flowing water input was started to slowly adjust the tank to the desired temperature treatment and to stabilize over 24 hours. Once treatment levels were reached, recording commenced for 48-hour trials, followed by 24-hour periods in which water temperature was slowly adjusted to the next temperature treatment and the shrimp group was provided ample dried shrimp pellets as food.
Data analysis
Following the completion of each snapping shrimp recording trial, files were digitized, and a threshold detector was applied using Matlab to detect and count snaps in all five-minute samples for each tank. Due to their specialized production mechanism (Versluis et al., 2000), shrimp snaps are highly stereotyped and typically saturate the signal in small tanks, making them easily distinguishable from other sounds. Other equivalently high amplitude impulsive sounds were rarely observed in any tank recordings. Following automatic detection, the waveforms of all snaps detected were visually examined and any false detections (e.g., caused by mechanical interference of shrimp or rare transient external noise) were removed from the datasets. The total number of snaps and time of snaps was then determined for each individual shrimp or shrimp pair for the duration of the trial, from which a total snap count was calculated. Because previous work observed strong diel cycles in snapping in field recordings that changed seasonally (Bohnenstiehl et al., 2016; Lillis and Mooney, 2016), experimental snap rates (snaps per hour) within different periods of the day (dawn, day, dusk, night) were also assessed for individual, pairs, and groups. For this analysis, dawn was defined as the period between the beginning of astronomical twilight and sunrise, day was the hours between sunrise and sunset, dusk between sunset and the end of astronomical twilight, and night between the end of dusk and beginning of dawn. All local twilight, sunrise, and sunset times were obtained from the U.S. Naval Observatory’s Astronomical Applications Department data services (http://aa.usno.navy.mil/data/docs).
The effect of temperature on individual and pair snap rates was compared with the fixed factors of pair type and time of day, using trial as a random effect (Sokal and Rohlf, 1995). Experiment 2 included the factor of species. Where significant interactions were detected, the effects of fixed factors were tested separately. All snap rate data that did not meet assumptions of normality were examined using a non-parametric Kruskal-Wallis test; when data showed heteroscedasticity, a Welch’s ANOVA was applied to test for differences (McDonald, 2014). To non-parametrically test the effect of time-of-day on individual and pair snap rates, repeated measures Friedman’s tests were used (McDonald, 2014). If significant effects of a given factor were found, differences between treatments/levels were determined using pairwise post-hoc tests with the Holm–Bonferroni method.
For Experiment 3 (mesocosm groups of 10 shrimp), a linear mixed effects model was used to test for the effect of temperature on snap rate, with trial (blocking factor) and group (repeated measure) as random effects (Sokal and Rohlf, 1995). Samples were further divided into the dawn, day, dusk, and night periods according to the methods described above for individual and pair trials, and snap rates (in snaps per hour) were calculated for each sample day.
Results
Field soundscape observations
There was a strong positive correlation between water temperature and snap rate for all three deployments (Figure 2; r=0.71-0.88), with variation in snap rates generally tracking closely with water temperature changes throughout the three deployments. Correspondingly, the recorded sound pressure levels were elevated at higher water temperatures (Figure 2, right panel). Examination of the time-series showed that snap rate fluctuations closely followed short-term (multi-day) water temperature variation of 1-2 degrees, with instances of two- to three-fold fluctuations in snap rates occurring when temperature increased and decreased within a 5-7 day period. Daily rhythms in snap rates did not follow trends of within-day temperature variation, however, as snapping activity is most closely related to crepuscular light cycles at the daily scale (Bohnenstiehl et al., 2016; Lillis and Mooney, 2018). Patterns in snap rates were most decoupled from water temperature in early Spring (Figure 2A, March) and late Fall (Figure 2C, November), when spikes in snap rates occurred without temperature increases.
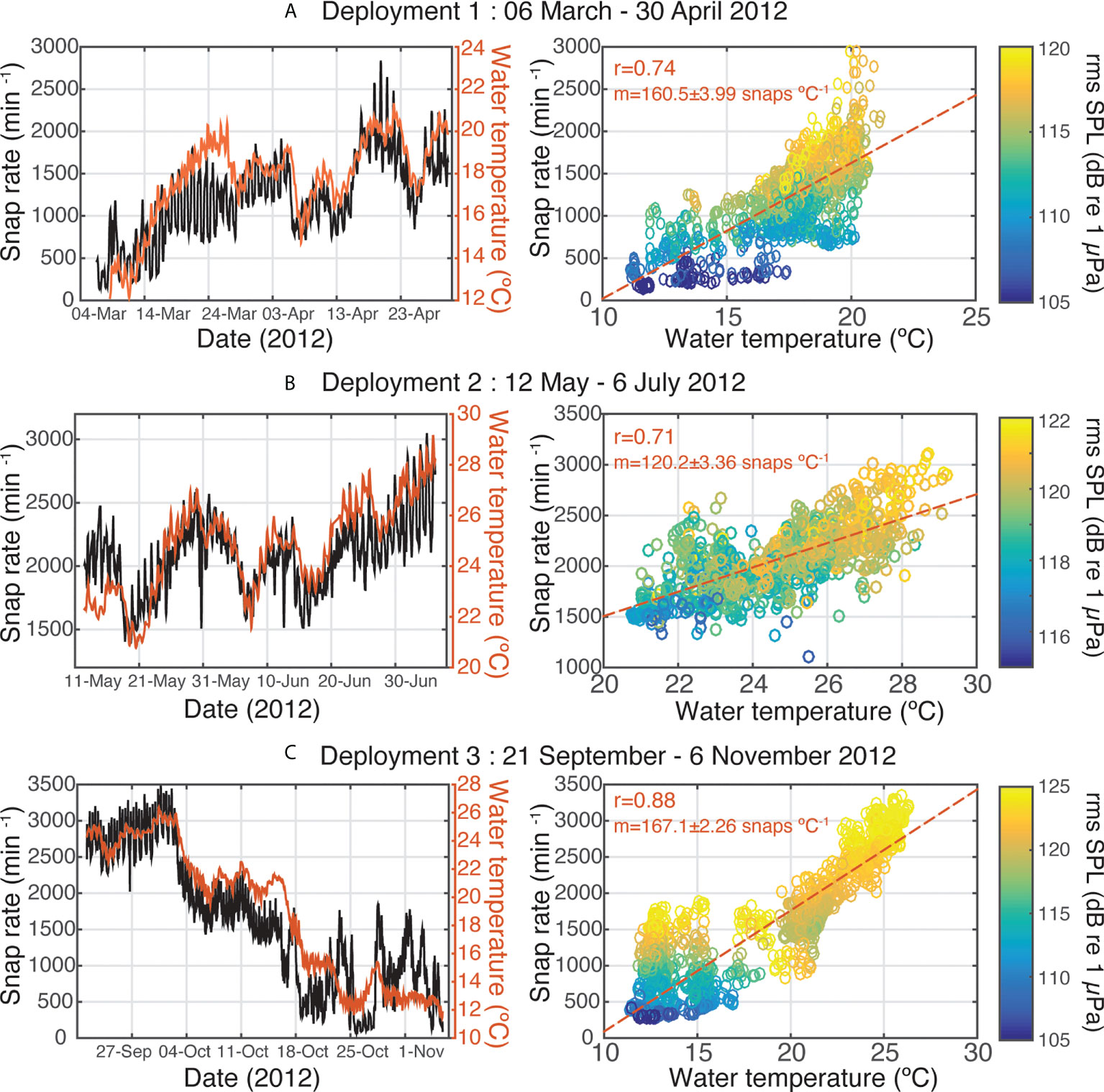
Figure 2 Time series and correlations of snap rates and water temperatures for 2012 deployment periods (A) 1, (B) 2, and (C) 3. Correlation plots show snap rate vs. water temperature with color indicating the measured root-mean-square sound pressure levels for each sample. Red lines are least squares fits to the data, and the correlation coefficients and slopes are displayed on each panel.
Laboratory experiments
Water temperature had a significant effect on snap rates for shrimp across all experiments (Figures 3, 4), for shrimp maintained in solitary, pair, and group configurations (Figure 4). Shrimp snap rates were consistently higher at higher temperatures (Figures 3, 4), but the strength of this pattern varied by social condition and diel period (Figures 4, 5).
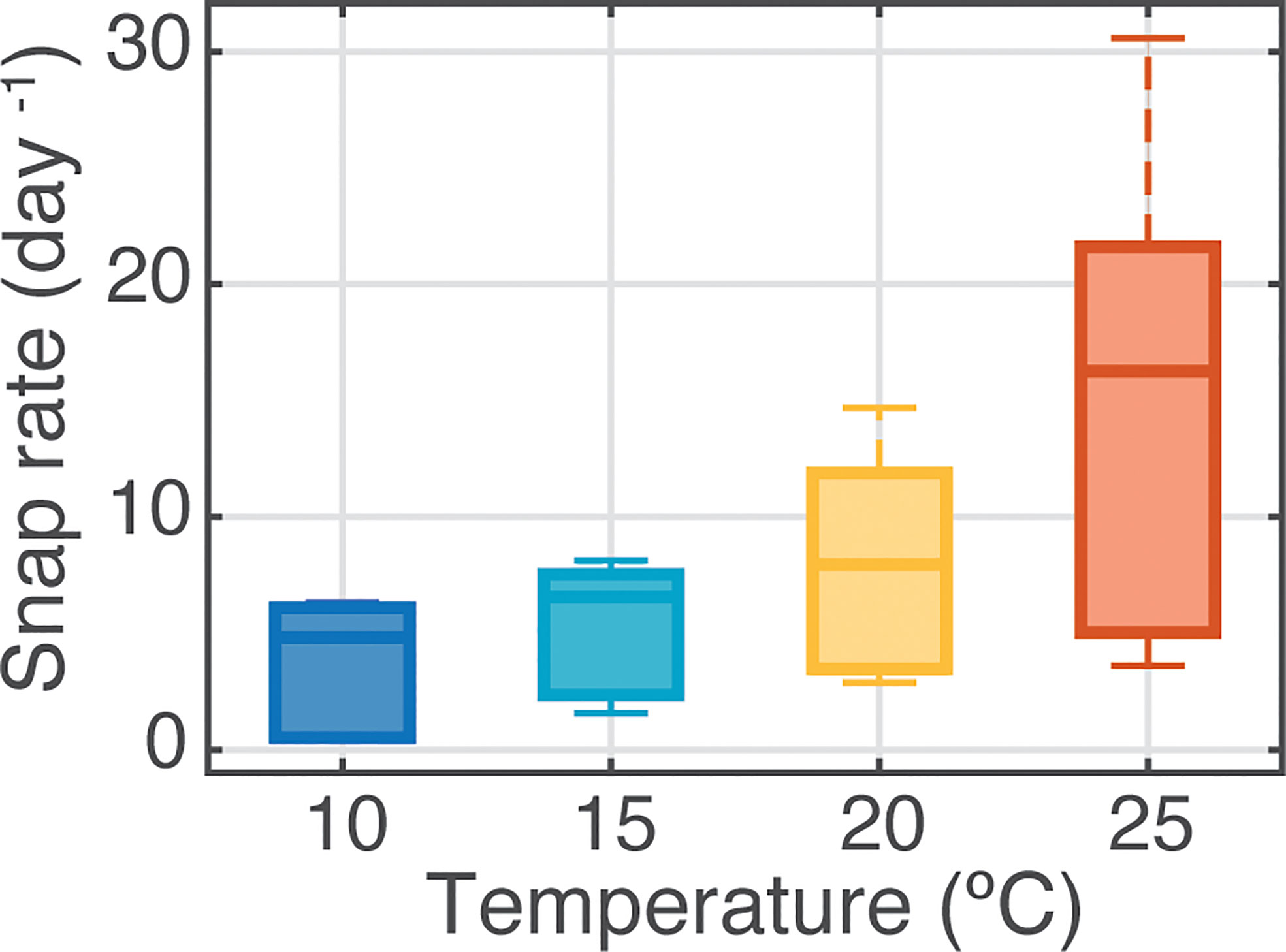
Figure 3 Effect of temperature on sound production by individual snapping shrimp Alpheus heterochaelis (n = 6 per treatment) in Experiment 1. Box plots show median values (solid horizontal lines), 50th percentile values (box outline), and 90th percentile values (whiskers) of snap rate (per day). Fitting a linear regression model of these data showed a significant effect of temperature (r2 = 0.32, F1,22 = 9.28, p<0.01).
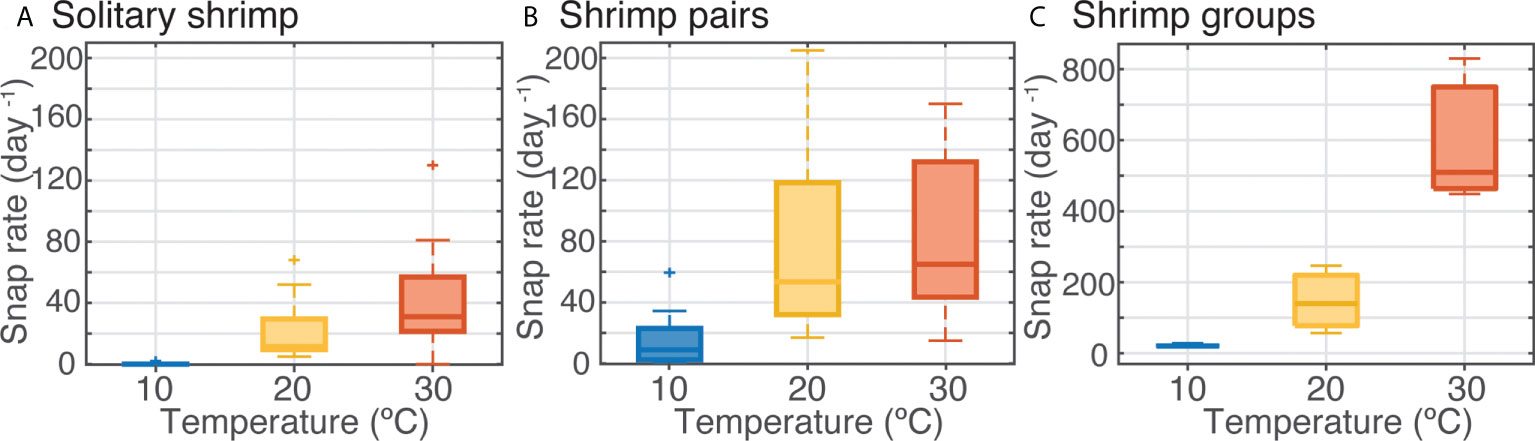
Figure 4 Effect of temperature on sound production by snapping shrimp in different social environments: (A) solitary, (B) pairs, and (C) groups of ten. Box plots show median values (solid horizontal lines), 50th percentile values (box outline), and 90th percentile values (whiskers) of snap rate (per day).
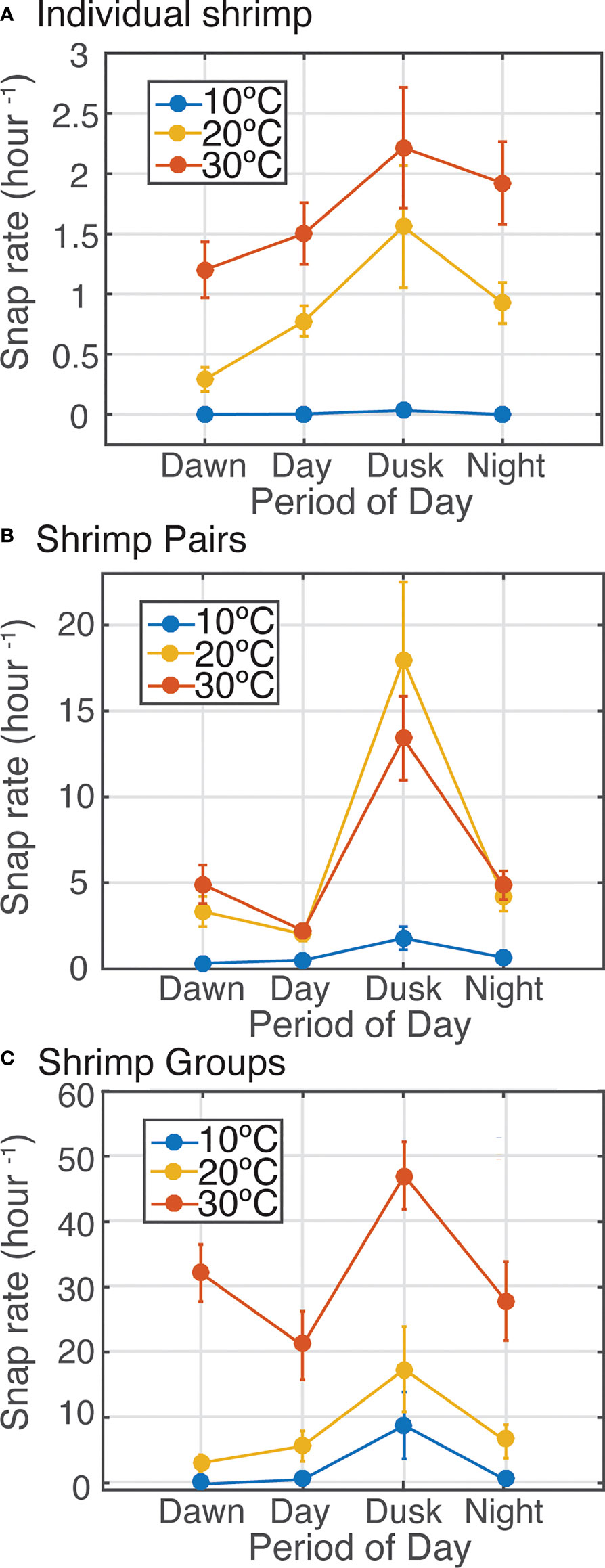
Figure 5 Effect of temperature on diel patterns in sound production by snapping shrimp measured in three experiments under different social contexts: (A) solitary, (B) pairs, and (C).
Experiment 1 showed that single shrimps maintained solitarily generated, on average, over three times more snaps per day at 25°C than at 10°C (Figure 3). Variability in snap emission rates among replicate shrimp was also highest within the elevated temperature treatments (Figure 3). In Experiment 2, in which the range of temperature was expanded to 30 °C and shrimp responses tested under different behavioral conditions, water temperature continued to show a substantial influence on snap rate for shrimp maintained both individually and in pairs (Figures 4A, B). Species was not found to be a significant factor for snap rate, in concordance with previous studies using these closely related species (Lillis et al., 2017), and therefore these data are presented as pooled results for the two species. Non-parametric Welch’s ANOVAs indicated a significant effect of temperature treatment on snap rates (solitary: F2,25 = 30.5, p<0.0001, n=20 per treatment; pairs: F2,26 = 23.4, p<.0001, n=18 per treatment). For shrimp held solitarily, snap rates were significantly lower at 10°C, with only one solitary shrimp producing a single snap detection compared to a median of 31 snaps day-1 for shrimp at 30°C (Figure 4A). While all pairs of shrimp did exhibit non-zero snap rates, at the 10 °C temperature treatment median snap rates were more than 7-fold lower than for pairs at 30°C (Figure 4B). Pair snap rate also showed high variability at both mid-range and high temperatures. In Experiment 3, where mixed-sex groups of 10 shrimp were held in mesocosms, median snap detections were 21, 140, and 510 snaps/day in the 10, 20, and 30°C treatments, respectively (Figure 4C). Log-transformed data for the groups of shrimp met the assumptions for an ANOVA, therefore, a linear mixed model (repeated measures) was applied to control for random effects of group and trial number (repeated measure and randomized block respectively). This test found that there was a statistically significant effect of temperature on group snap count (F1,7 = 96.8, p<.0001).
Examining snap production throughout the diel cycle revealed that shrimp acoustic output varied by time of day, but this pattern was modulated by temperature and behavioral context (Figure 5). Dawn and dusk periods are the time of day when snapping shrimp populations are observed to be most acoustically active in soundscape recordings (Radford et al., 2008; Radford et al.,2010; Lillis et al., 2017; Lillis and Mooney 2018). For all laboratory trials, within all treatments, snap rate was highest during dusk periods (Figure 5), except for individual shrimp, which did not snap under low temperatures (Figure 5A). It is evident that crepuscular snapping primarily drives the overall influence of temperature on snap rate, particularly when shrimp are held together as male-female pairs or mixed sex groups.
Discussion
The results of controlled laboratory experiments show that changes in water temperature strongly drive the sound production by snapping shrimp across different behavioral contexts. Combined with close examination of field measurements of snap rates, these investigations of shrimp snapping behavior in relation to water temperature indicate that warmer temperatures increase snap rates of snapping shrimp, most markedly in a mesocosm group setting that most closely approximate natural conditions. These findings support the hypothesis that correlations between snap rates and water temperature apparent in field data are primarily related to the effect of temperature on shrimp physiology and behavior, rather than a result of seasonality or changes in shrimp population size. Further, the field-based observations confirm that elevated snapping activity in response to warming results in substantial increases in environmental sound pressure levels (e.g., up to 15 dB, or 6-fold, increase over a three-week time scale).
Crustaceans are known to have sensitive thermoreception capabilities, with a thermosensitivity range of 0.2-2°C, and demonstrate behavioral modification in response to local temperature changes (Lagerspetz and Vainio, 2006). Many shallow water crustaceans have a broad temperature survival tolerance; however, being ectothermic animals their activities are strongly driven by water temperature (Withers, 1992). Warming can increase agitation and antagonistic encounters (Hoffman et al., 1975; Van Der Meeren, 1993; Souza et al., 2019), as well as feeding rate and bioturbative activity, up to thermal optima (Pearson et al., 2018; Pillay, 2019). Given that Alpheid snaps are primarily used in territorial or agonistic displays, as well as in feeding and colony communication (Duffy et al., 2002; Lillis et al., 2017), the increased snap rates at the highest temperatures observed in our experiments likely resulted from an increase in a combination of these behaviors dependent on the social context. In isolation, shrimp rarely snapped when held at the lowest temperature (10°C), whereas in paired and group settings snapping was observed at higher relative rates compared to the mid- and high-temperature treatments, suggesting that even at low temperatures that may metabolically restrict most activity, the intraspecific social function of snapping is important. This elevation in snapping, regardless of temperature, is seen especially at dusk, when snapping shrimp are known to peak in their sound production. Interestingly, when shrimp were held in opposite-sex pairs there was no difference between the snap rates under the mid- and high-temperature treatments, possibly a result of the close quarters they experienced relative to the mesocosm tanks, in which shrimp had more space to avoid interaction or confrontation. This implies that population density, social context, and resource availability could influence the relationship between temperature and snapping shrimp sound production and should be considered in future predictions of climate-mediated shifts in shrimp-dominated soundscapes.
The experiments described here were relatively short-term (days to weeks), and the long-term effects of elevated temperatures on shrimp acoustic activity, and consequently to the overall soundscape, need to be measured. It is worthy to note that shrimp in Experiments 1 and 2 were exposed to temperature changes more rapidly than are likely to occur in nature and trials to measure snap rates were short (24-48 h); experiments using mesocosm groups better represent naturalistic effects of temperature changes over days. Yet, it is possible that these animals may not be able to maintain higher snapping rates during longer heatwaves, or may adjust to longer-term climate patterns, and how seawater temperature increases may influence Alpheid population dynamics, and associated soundscape patterns introduces complexity to these predictions. In addition, other physical variables must be included in a predictive framework, since snap rates are known to be affected by pH (Rossi et al., 2016), as well as patterns in wind speed, light, dissolved oxygen (Jung et al., 2012; Bohnenstiehl et al., 2016) and current speed (Lee et al., 2021). Indeed, the drivers of snapping shrimp sound production patterns are likely to vary dependent on local ecological (e.g., species composition, life history) and physical (e.g., depth, temperature variation, light) conditions. Most investigations of snapping patterns in relation to physical variables are relatively short-term (e.g., Lee et al., 2021 examined snapping over 5 days in a 100-m deep, temperature-stable habitat) and do not enable predictions of how temperature may alter the sound production. Thus, further investigation is needed to understand the relative importance of physical drivers on soundscape variation in snapping-shrimp dominated seas. Interacting direct and indirect effects of climate changes and snapping shrimp habitat degradation (e.g., coral bleaching, sponge host die-off) on soundscape variation should also be considered (Butler et al., 2016; Butler et al., 2017), particularly since snapping sounds strongly influence commonly used passive acoustic monitoring metrics of habitat health (Bohnenstiehl et al., 2018; Elise et al., 2019) and have been suggested as indicators of “healthy” soundscapes or habitat restoration success (Butler et al., 2021; Lamont et al., 2022).
Snapping shrimp signals are biological sounds of particularly high intensity and broadband nature, extending from a few Hz to well beyond 250 kHz (Au and Banks, 1998), perhaps the broadest frequency range of any biological sound. Therefore, changes in the snap rate are particularly impactful on the baseline sound levels and frequency content of a habitat’s soundscape. It follows that climate change could have a profound impact on coastal marine soundscapes via snapping shrimp responses to warming, particularly in temperate and sub-tropical hotspots for climate-driven change in biological systems (Rius et al., 2014; Vergés et al., 2014; García Molinos et al., 2016). Coastal and estuarine benthic communities from tropical to temperate seas, across which established snapping shrimp populations are common, are predicted to experience further changes in temperature regime and extreme temperature events (Ummenhofer and Meehl, 2017). Lower temperatures have long been considered one of the limits to snapping shrimp geographic distributions (Knowlton, 1980), with a lower threshold at 10-11°C, though populations are known to occur in areas that drop below this, possibly due to favorable local conditions during critical life history phases (e.g., larval development, spawning) (Mathews, 2007). Based on the experimental evidence presented herein, not only is there the potential for substantially increased snap rates and corresponding broadband noise levels across the current geographical distribution of snapping shrimp, but also for introducing shrimp sound to higher latitude regions where it does not currently exist.
How temperature-induced alterations in soundscape biophony might affect other taxa or the communities which share habitats with snapping shrimp is an open question, but it is well-established that increasing human-produced noise can reduce communication space, mask biologically important signals, increase stress, and cause injuries, auditory and otherwise (e.g., Branstetter et al., 2013; de Soto et al., 2013; Jones et al., 2020; Mooney et al., 2020; Jones 2021). It remains to be explored whether the dominating contribution of snapping shrimp to soundscapes could induce similar deleterious effects when the sound intensity is increased; however, given that their broadband sounds form the background noise floor, patterns of snapping shrimp acoustic activity are relevant to all sound-receptive and sound-producing organisms living in these habitats. Indeed, at least in reef environments, snapping shrimp signals, typically centered between 2-7 kHz, may have played in role in the evolution of fish calls being confined to a relatively narrow range of low frequencies. Moreover, they contribute across all frequencies to create overall noisy conditions, known to influence fish call amplitude and rate, with fishes compensating to overcome background noise (Holt and Johnston, 2014). This suggests that a temperature-mediated change in snapping shrimp sound production will affect the acoustic communication space of organisms in their communities. While few studies have investigated community-scale acoustic interactions, current evidence underscores the broad ecoacoustic influence of snapping signals. For example, these sounds can impede fish acoustic communication (Thorson and Fine, 2002), influence larval settlement (Simpson et al., 2008), a key component to reef resiliency, and may play a role in coastal orientation and navigation of nearshore cetacean migrations (Allen, 2013). Certainly, given that snapping shrimp sounds are more pervasive than any other acoustic signal in any other ecosystem, and the potential for substantial soundscape alteration via climate change effects on snapping shrimp activity is now clear, there is a need to better understand their community-level impacts in coastal marine systems.
Researchers have recently been sounding the alarm regarding increasing anthropogenic noise in the sea and calling for it to be included in assessments of human impacts on marine ecosystems (Duarte et al., 2021), however, climate change is likely to have equally important not-so-silent impacts on soundscapes in the anthropocene through the modification of the behavior and physiology of dominant sound producers like snapping shrimp. The evidence presented here suggests that relationships between temperature and bioacoustic responses, and the potential cascading community-level impacts, should also be considered in predictions of future soundscapes. To our knowledge, these data are the first evidence supporting this concern for a climate-induced alteration of the marine soundscape via a temperature impact on a keystone acoustic taxon. Snapping shrimp present an intriguing case for investigation as a biological soundscape component whose acoustic output is widespread and appears to be strongly thermally regulated, yet has been relatively understudied with respect to its influence on its ecological community. More broadly, there is a growing understanding of the importance of soundscape cues in facilitating ecosystem-level processes and patterns in the sea; our findings highlight the need to consider the complex biotic and abiotic dependencies underpinning soundscape variation, especially from a global change perspective.
Data availability statement
The acoustic datasets analyzed for this study can be found in the Biological and Chemical Oceanography Data Management Office (BCO-DMO) repository as: Eggleston, David and Bohnenstiehl, DelWayne (2015) Raw files from DSG hydrophone collected from the West Bay Marine Reserve in Pamlico Sound, NC during 2011 (Larval settlement soundscapes project). Dataset version 2015-12-09. http://lod.bco-dmo.org/id/dataset/628710.
Author contributions
AL and TM conceived and designed the study. AL performed the experiments, analysis, and drafted the manuscript. TM contributed to the manuscript revision, read, and approved the submitted version. All authors contributed to the article and approved the submitted version.
Funding
Financial support was provided by NSF Biological Oceanography Grant #1536782, Woods Hole Oceanographic Institution’s Interdisciplinary Award and Postdoctoral Scholar Programs.
Acknowledgments
We thank the NCSU CMAST Summer Fellow program and Dr. David Eggleston, and especially Rachel Willis and Brenton Griffin, for their assistance with initial experimental animal collection. WHOI interns Apryle Panyi and Jessica Perelman were instrumental to experimental setup, shrimp husbandry, and assistance with data collection. Thanks also to Dr. Melissa Hughes for advice on snapping shrimp observation and husbandry, Dr. Richard Heard for species identification assistance, and Dr. Daniel Zitterbart for advice in the development of laboratory data acquisition code.
Conflict of interest
The authors declare that the research was conducted in the absence of any commercial or financial relationships that could be construed as a potential conflict of interest.
Publisher’s note
All claims expressed in this article are solely those of the authors and do not necessarily represent those of their affiliated organizations, or those of the publisher, the editors and the reviewers. Any product that may be evaluated in this article, or claim that may be made by its manufacturer, is not guaranteed or endorsed by the publisher.
References
Affatati A., Scaini C., Salon S. (2022). Ocean sound propagation in a changing climate: Global sound speed changes and identification of acoustic hotspots. Earths Future 10, e2021EF002099. doi: 10.1029/2021EF002099
Allen A. N. (2013). An investigation of the roles of geomagnetic and acoustic cues in whale navigation and orientation (Thesis) (The University is in Boston, Massachusetts, USA: Massachusetts Institute of Technology).
Au W. W. L., Banks K. (1998). The acoustics of the snapping shrimp synalpheus parneomeris in kaneohe bay. J. Acoust. Soc Am. 103, 41–47. doi: 10.1121/1.423234
Au W. W. L., Hastings M. C. (2008). Principles of marine bioacoustics (Springer, New York, NY: Springer Science).
Au W. W. L., Penner R. H. (1981). "Target detection in noise by echolocating Atlantic bottlenose dolphins", The Journal of the Acoustical Society of America 70, 687-693. doi: 10.1121/1.386931
Bass A. H., McKibben J. R. (2003). Neural mechanisms and behaviors for acoustic communication in teleost fish. Prog. Neurobiol. 69, 1–26. doi: 10.1016/S0301-0082(03)00004-2
Bohnenstiehl D. R., Lillis A., Eggleston D. B. (2016). The curious acoustic behavior of estuarine snapping shrimp: Temporal patterns of snapping shrimp sound in Sub-tidal oyster reef habitat. PloS One 11, e0143691. doi: 10.1371/journal.pone.0143691
Bohnenstiehl D. R., Lyon R. P., Caretti O. N., Ricci S. W., Eggleston D. B. (2018). Investigating the utility of ecoacoustic metrics in marine soundscapes. JEA 2, 1–1. doi: 10.22261/JEA.R1156L
Branstetter B. K., Trickey J. S., Bakhtiari K., Black A., Aihara H., Finneran J. J. (2013). Auditory masking patterns in bottlenose dolphins (Tursiops truncatus) with natural, anthropogenic, and synthesized noise. J. Acoust. Soc Am. 133, 1811–1818. doi: 10.1121/1.4789939
Buckingham M. J., Epifanio C. L., Readhead M. L. (1996). Passive imaging of targets with ambient noise: Experimental results. J. Acoust. Soc. Am. 100, 2736–2736. doi: 10.1121/1.416834
Butler J., Butler M. J. IV, Gaff H. (2017). Snap, crackle, and pop: Acoustic-based model estimation of snapping shrimp populations in healthy and degraded hard-bottom habitats. Ecol. Indic. 77, 377–385. doi: 10.1016/j.ecolind.2017.02.041
Butler J., Stanley J. A., Butler M. J. IV (2016). Underwater soundscapes in near-shore tropical habitats and the effects of environmental degradation and habitat restoration. J. Exp. Mar. Biol. Ecol. 479, 89–96. doi: 10.1016/j.jembe.2016.03.006
Butler J., Pagniello C. M.L. S., Jaffe J. S., Parnell P. E., Širović A. (2021). Diel and Seasonal Variability in Kelp Forest Soundscapes Off the Southern California Coast. Front. Mar. Sci. 8.
Buxton R. T., McKenna M. F., Mennitt D., Fristrup K., Crooks K., Angeloni L., et al. (2017). Noise pollution is pervasive in U.S. protected areas. Sci. 356, 531–533. doi: 10.1126/science.aah4783
Chitre M. A., Potter J. R., Ong S.-H. (2006). Optimal and near-optimal signal detection in snapping shrimp dominated ambient noise. Ocean. Eng. IEEE J. Of. 31, 497–503. doi: 10.1109/JOE.2006.875272
Connaughton M. A., Taylor M. H., Fine M. L. (2000). Effects of fish size and temperature on weakfish disturbance calls: Implications for the mechanism of sound generation. J. Exp. Biol. 203, 1503–1512.
Cotter A. (2008). “The “soundscape” of the sea, underwater navigation, and why we should be listening more,” in Advances in fisheries science: 50 years on from beverton and Holt. Eds. Payne A., Cotter J., Potter T. (Hoboken, USA: John Wiley and Sons), 451–471.
de Soto N. A., Delorme N., Atkins J., Howard S., Williams J., Johnson M. (2013). Anthropogenic noise causes body malformations and delays development in marine larvae. Sci. Rep. 3, 2831. doi: 10.1038/srep02831
Duarte C. M., Chapuis L., Collin S. P., Costa D. P., Devassy R. P., Eguiluz V. M., et al. (2021). The soundscape of the anthropocene ocean. Science 371, eaba4658. doi: 10.1126/science.aba4658
Duffy E. J., Morrison C. L., Macdonald K. S. (2002). Colony defense and behavioral differentiation in the eusocial shrimp synalpheus regalis. Behav. Ecol. Sociobiol. 51, 488–495. doi: 10.1007/s00265-002-0455-5
Eggleston D., Bohnenstiehl D. W. (2015). Raw files from DSG hydrophone collected from the West Bay Marine Reserve in Pamlico Sound, NC during 2011 (Larval settlement soundscapes project). Dataset version 2015-12-09. http://lod.bco-dmo.org/id/dataset/628710.
Elise S., Urbina-Barreto I., Pinel R., Mahamadaly V., Bureau S., Penin L., et al. (2019). Assessing key ecosystem functions through soundscapes: A new perspective from coral reefs. Ecol. Indic. 107, 105623. doi: 10.1016/j.ecolind.2019.105623
Everest F. A. (1948). Acoustical characteristics of noise produced by snapping shrimp. J. Acoust. Soc. Am. 20, 137. 10.1121/1.1906355
Gage S. H., Axel A. C. (2014). Visualization of temporal change in soundscape power of a Michigan lake habitat over a 4-year period. Ecol. Inform. Ecol. Acoustics 21, 100–109. doi: 10.1016/j.ecoinf.2013.11.004
García Molinos J., Halpern B. S., Schoeman D. S., Brown C. J., Kiessling W., Moore P. J., et al. (2016). Climate velocity and the future global redistribution of marine biodiversity. Nat. Clim. Change 6, 83–88. doi: 10.1038/nclimate2769
Gerhardt H. C., Huber F. (2002). Acoustic Communication in Insects and Anurans: Common Problems and Diverse Solutions. University of Chicago Press. 544 p.
Hawkins A. D., Popper A. N. (2017). A sound approach to assessing the impact of underwater noise on marine fishes and invertebrates. ICES J. Mar. Sci. 74, 635–651. doi: 10.1093/icesjms/fsw205
Hildebrand J. A. (2009). Anthropogenic and natural sources of ambient noise in the ocean. Mar. Ecol. Prog. Ser. 395, 5–20. doi: 10.3354/meps08353
Hoffman R. S., Dunham P. J., Kelly P. V. (1975). Effects of water temperature and housing conditions upon the aggressive behavior of the lobster homarus americanus. J. Fish. Board Can. 32(5), 713–717. doi: 10.1139/f75-092
Holt D. E., Johnston C. E. (2014). Evidence of the Lombard effect in fishes. Behav. Ecol. 25, 819–826. doi: 10.1093/beheco/aru028
Hughes M., Williamson T., Hollowell K., Vickery R. (2014). Sex and weapons: Contrasting sexual dimorphisms in weaponry and aggression in snapping shrimp. Ethology 120, 982–994. doi: 10.1111/eth.12270
Johnson M. W., Alton Everest F., Young R. W. (1947). The role of snapping shrimp (Crangon and Synalpheus) in the production of underwater noise in the sea. Biol. Bull. 93, 122–138.
Jones I. T., Stanley J. A., Mooney T. A. (2020). Impulsive pile driving noise elicits alarm responses in squid (Doryteuthis pealeii). Mar. pollut. Bull. 150, 110792. doi: 10.1016/j.marpolbul.2019.110792
Jones I. T., Peyla J. F., Clark H., Song Z., Stanley J. A., Mooney T. A. (2021). Changes in feeding behavior of longfin squid (Doryteuthis pealeii) during laboratory exposure to pile driving noise. Mar. Environ. Res. 165, 105250. doi: 10.1016/j.marenvres.2020.105250
Jung S.-K., Choi B. K., Kim B.-C., Kim B.-N., Kim S. H., Park Y., et al. (2012). Seawater temperature and wind speed dependences and diurnal variation of ambient noise at the snapping shrimp colony in shallow water of southern Sea of Korea. Jpn. J. Appl. Phys. 51, 7GG09. doi: 10.1143/JJAP.51.07GG09
Kaplan M. B., Mooney T. A., Partan J., Solow A. R. (2015). Coral reef species assemblages are associated with ambient soundscapes. Mar. Ecol. Prog. Ser. 533, 93–107. doi: 10.3354/meps11382
Knowlton N. (1980). Sexual selection and dimorphism in two demes of a symbiotic, pair-bonding snapping shrimp. Evolution 34, 161–173. doi: 10.1111/j.1558-5646.1980.tb04802.x
Krause B., Farina A. (2016). Using ecoacoustic methods to survey the impacts of climate change on biodiversity. Biol. Conserv. 195, 245–254. doi: 10.1016/j.biocon.2016.01.013
Lagerspetz K. Y. H., Vainio L. A. (2006). Thermal behaviour of crustaceans. Biol. Rev. 81, 237–258. doi: 10.1017/S1464793105006998
Lammers M. O., Brainard R. E., Au W. W. L., Mooney T. A., Wong K. B. (2008). An ecological acoustic recorder (EAR) for long-term monitoring of biological and anthropogenic sounds on coral reefs and other marine habitats. J. Acoust. Soc. Am. 123, 1720–1728. doi: 10.1121/1.2836780
Lamont T. A. C., Williams B., Chapuis L., Prasetya M. E., Seraphim M. J., Harding H. R., et al. (2022). The sound of recovery: Coral reef restoration success is detectable in the soundscape. J. Appl. Ecol. 59(3), 742–756. doi: 10.1111/1365-2664.14089
Lee D. H., Choi J. W., Shin S., Song H. C. (2021). Temporal variabilityin acoustic behavior of snapping shrimp in the East China Sea and its correlation with ocean environments. Front. Mar. Sci. 8, 779283. doi: 10.3389/fmars.2021.779283
Lillis A., Eggleston D. B., Bohnenstiehl D. R. (2013). Oyster larvae settle in response to habitat-associated underwater sounds. PLoS ONE 8, e79337. doi: 10.1371/journal.pone
Lillis A., Eggleston D. B., Bohnenstiehl D. R. (2014). Estuarine soundscapes: Distinct acoustic characteristics of oyster reefs compared to soft-bottom habitats. Mar. Ecol. Prog. Ser. 505, 1–17. doi: 10.3354/meps10805
Lillis A., Mooney T. A. (2016). Loudly heard, little seen, and rarely understood: Spatiotemporal variation and environmental drivers of sound production by snapping shrimp. Proc. Meet. Acoust. 27, 010017. doi: 10.1121/2.0000270
Lillis A., Mooney T. A. (2018). Snapping shrimp sound production patterns on Caribbean coral reefs: Relationships with celestial cycles and environmental variables. Coral Reefs. doi: 10.1007/s00338-018-1684-z
Lillis A., Perelman J. N., Panyi A., Aran Mooney T. (2017). Sound production patterns of big-clawed snapping shrimp (Alpheus spp.) are influenced by time-of-day and social context. J. Acoust. Soc Am. 142, 3311–3320. doi: 10.1121/1.5012751
Lillis A., Apprill A., Suca J. J., Becker C., Llopiz J. K., Mooney T. A. (2018). Soundscapes influence the settlement of the common Caribbean coral Porites astreoides irrespective of light conditions. R. Soc. Open Sci. 5, 181358. 10.1098/rsos.181358
Luczkovich J. J., Sprague M. W. (2008). Does boat noise affect spawning sound production of soniferous fish in shallow estuarine systems? Bioacoustics 17, 203–205. doi: 10.1080/09524622.2008.9753817
Mathews L. M. (2007). Evidence for restricted gene flow over small spatial scales in a marine snapping shrimp alpheus angulosus. Mar. Biol. 152, 645–655. doi: 10.1007/s00227-007-0718-9
McDonald J. H. (2014). Handbook of biological statistics. 3rd ed (Baltimore, Maryland: Sparky House Publishing).
Mooney T. A., Andersson M. H., Stanley J. (2020). Acoustic impacts of offshore wind energy on fishery resources: An evolving source and varied effects across a wind farm’s lifetime. Oceanography 33, 82–95. doi: 10.5670/oceanog.2020.408
Pearson R. M., Jinks K. I., Brown C. J., Schlacher T. A., Connolly R. M. (2018). Functional changes in reef systems in warmer seas: Asymmetrical effects of altered grazing by a widespread crustacean mesograzer. Sci. Total Environ. 644, 976–981. doi: 10.1016/j.scitotenv.2018.07.051
Pijanowski B. C., Villanueva-Rivera L. J., Dumyahn S. L., Farina A., Krause B. L., Napoletano B. M., et al. (2011). Soundscape ecology: The science of sound in the landscape. BioScience 61, 203–216. doi: 10.1525/bio.2011.61.3.6
Pillay D. (2019). Ecosystem engineering by thalassinidean crustaceans: Response variability, contextual dependencies and perspectives on future research. Diversity 11, 64. doi: 10.3390/d11040064
Radford C., Jeffs A., Tindle C., Montgomery J. (2008). Temporal patterns in ambient noise of biological origin from a shallow water temperate reef. Oecologia 156, 921–929.
Radford C.A., Stanley J.A., Tindle C.T., Montgomery J.C., Jeffs A.G. (2010). Localised coastal habitats have distinct underwater sound signatures. Mar. Ecol. Prog. Ser., 401, 21–29.
Rius M., Clusella-Trullas S., McQuaid C. D., Navarro R. A., Griffiths C. L., Matthee C. A., et al. (2014). Range expansions across ecoregions: interactions of climate change, physiology and genetic diversity. Glob. Ecol. Biogeogr. 23, 76–88. doi: 10.1111/geb.12105
Rossi T., Connell S. D., Nagelkerken I. (2016). Silent oceans: ocean acidification impoverishes natural soundscapes by altering sound production of the world’s noisiest marine invertebrate. Proc. R. Soc B Biol. Sci. 283, 20153046. doi: 10.1098/rspb.2015.3046
Simpson S. D., Meekan M. G., Jeffs A., Montgomery J. C., McCauley R. D. (2008). Settlement-stage coral reef fish prefer the higher-frequency invertebrate-generated audible component of reef noise. Anim. Behav. 75, 1861–1868. doi: 10.1016/j.anbehav.2007.11.004
Slabbekoorn H., Bouton N., van Opzeeland I., Coers A., ten Cate C., Popper A. N. (2010). A noisy spring: the impact of globally rising underwater sound levels on fish. Trends Ecol. Evol. 25, 419–427. doi: 10.1016/j.tree.2010.04.005
Sokal R. R., Rohlf F. J. (1995). Biometry: The principles and practices of statistics in biological research. 3rd ed (New York: WH Freeman & Co.).
Souza A. T., Ribas F. O., Moura J. F., Moreira C., Campos J., Ilarri M. I. (2019). Influence of temperature on intraspecific, unbalanced dyadic contests between crabs. PeerJ 7, e7845. doi: 10.7717/peerj.7845
Spence H. R., Knowlton R. E. (2008). Morphological and Developmental Differences in Three Species of the Snapping Shrimp Genus Alpheus (Crustacea, Decapoda). Southeast. Nat. 7, 207–218. doi: 10.1656/1528-7092
Staaterman E., Paris C. B., DeFerrari H. A., Mann D. A., Rice A. N., DAlessandro E. K. (2014). Celestial patterns in marine soundscapes. Mar. Ecol. Prog. Ser. 508, 17–32. doi: 10.3354/meps10911
Sueur J., Krause B., Farina A. (2019). Climate change is breaking earth’s beat. Trends Ecol. Evol. 34, 971–973. doi: 10.1016/j.tree.2019.07.014
Thorson R. F., Fine M. L. (2002). Acoustic competition in the gulf toadfish opsanus beta: Acoustic tagging. J. Acoust. Soc Am. 111, 2302–2307. doi: 10.1121/1.1466865
Ummenhofer C. C., Meehl G. A. (2017). Extreme weather and climate events with ecological relevance: A review. Philos. Trans. R. Soc Lond. B. Biol. Sci. 372, 20160135. doi: 10.1098/rstb.2016.0135
Van Der Meeren G. I. (1993). Initial response to physical and biological conditions in naive juvenile lobsters homarus gammarus l. Mar. Behav. Physiol. 24, 79–92. doi: 10.1080/10236249309378881
Vergés A., Steinberg P. D., Hay M. E., Poore A. G. B., Campbell A. H., Ballesteros E., et al. (2014). The tropicalization of temperate marine ecosystems: Climate-mediated changes in herbivory and community phase shifts. Proc. R. Soc B Biol. Sci. 281, 20140846. doi: 10.1098/rspb.2014.0846
Versluis M., Schmitz B., von der Heydt A., Lohse D. (2000). How snapping shrimp snap: Through cavitating bubbles. Science 289, 2114–2117. doi: 10.1126/science.289.5487.2114
Keywords: soundscape, underwater noise, acoustic ecology, acoustic monitoring, crustacean, ecoacoustics
Citation: Lillis A and Mooney TA (2022) Sounds of a changing sea: Temperature drives acoustic output by dominant biological sound-producers in shallow water habitats. Front. Mar. Sci. 9:960881. doi: 10.3389/fmars.2022.960881
Received: 03 June 2022; Accepted: 25 July 2022;
Published: 18 August 2022.
Edited by:
Thomas Wernberg, University of Western Australia, AustraliaReviewed by:
Mark W. Sprague, East Carolina University, United StatesFrancesco Caruso, Stazione Zoologica Anton Dohrn Napoli, Italy
Jee Woong Choi, Hanyang Universiy, South Korea
Copyright © 2022 Lillis and Mooney. This is an open-access article distributed under the terms of the Creative Commons Attribution License (CC BY). The use, distribution or reproduction in other forums is permitted, provided the original author(s) and the copyright owner(s) are credited and that the original publication in this journal is cited, in accordance with accepted academic practice. No use, distribution or reproduction is permitted which does not comply with these terms.
*Correspondence: Ashlee Lillis, YXNobGVlQHNvdW5kb2NlYW5zY2llbmNlLmNvbQ==; T. Aran Mooney, YW1vb25leUB3aG9pLmVkdQ==