- 1Ruđer Bošković Institute, Center for Marine Research, Rovinj, Croatia
- 2Ruđer Bošković Institute, Department for Marine and Environmental Research, Zagreb, Croatia
- 3INA-INDUSTRIJA NAFTE, Plc., Central Testing Laboratory, Zagreb, Croatia
The microalgae of the genus Pseudochloris/Picochlorum are characterized by fast growth, and wide nutrient (type and concentration) and salinity tolerance, all contributing towards exploration of their use in high-density biomass production and wastewater bioremediation. In this study, removal of nitrogen and phosphorus nutrients from oil refinery wastewater was monitored during growth of the marine eukaryotic microalgae Pseudochloris wilhelmii, with emphasis on biochemical analyses of its biomass quality to evaluate suitability for biodiesel production. A series of growth experiments under various nutrient and light regimes were performed in a temperature range of 20-30°C to evaluate nutrient removal and biomass growth dependence on temperature. The highest removal rate of dissolved inorganic nitrogen reached under the given experimental conditions was 0.823 mmol/(gday) accompanied by the corresponding biomass productivity of 115.2 mg/(Lday). Depending on light and temperature, the final lipid concentration ranged 181.5 – 319.8 mg/L. Furthermore, increase in nutrient load decreased the maximum specific growth rate by 25%, and the maximum specific removal rate of the dissolved inorganic nitrogen by 19%, whereas the duration of bioremediation process was nearly doubled. In contrast, constant light exposure expedited the nitrogen removal, i.e. bioremediation process, by almost 40%, while supporting over three times higher biomass productivity and the highest maximum specific growth rate of 0.528 g/(gday). The conditions favoring the highest nitrogen removal and highest toxicity reduction in oil refinery wastewater are met at 24°C and 130 µmol phot/(m2s). The highest proportion of carbon-binding to the P. wilhelmii biomass was noticed under the same conditions, thus indicating them as the most favorable conditions for hydrocarbon removal as well as for CO2 sequestration. Pseudochloris wilhelmii therefore represents a promising candidate for oil refinery wastewater remediation and valuable biomass cogeneration on a large-scale.
1 Introduction
Remediation systems of oil refinery wastewaters (ORWW) are most commonly based on a combination of physicochemical and biological processes. The biological treatment has a foundation in bacteria’s ability to degrade dissolved and colloidal organic matter while transforming and using inorganic nitrogen and phosphorus as main mineral nutrients present in the ORWW. Since oil refinery wastewater is rich in ammonium and poor in bioavailable organic compounds, the ORWW treatment by heterotrophic bacteria consumes high quantities of added glycerol, phosphorus and oxygen, increasing the cost of the process. Recently, there has been a change of the paradigm in the oil refining industry from heterotrophic towards the autotrophic removal of contaminants in waste waters (WW) by microalgae, producing lucrative biomass useful in biofuel production (Maity et al., 2014). However, there are few algal strains resilient and able to grow in the presence of oil-related toxic compounds (Jiang et al., 2010). The microalgae of the genus Pseudochloris/Picochlorum are characterized by fast growth, and wide nutrient (type and concentration) and salinity tolerance (Von Alvensleben et al., 2013; Budiša et al., 2019; Concas et al., 2019). Picochlorum atomus showed that salinity had no effect on its growth performance, and turned out to be a useful agent for contamination control against some prokaryotes (Von Alvensleben et al., 2013). In contrast, Pseudochloris wilhelmii growth varies with salinity, with the fastest growth observed in the range from 7 psu1 to 21 psu (Budiša et al., 2019). Moreover, the marine picoeukaryotes belonging to Pseudochloris/Picochlorum genera have demonstrated high lipid content, up to 23% (De la Vega et al., 2011; Concas et al., 2019), a desired characteristics for biofuel production. In the study of Concas et al. (2019) the authors have demonstrated numerous advantages of using P. wilhelmii in the integrated cogeneration/WW treatment process. Mixing WW with seawater allows growth in the turbid and dark WW, increasing the penetration of light through the water column of the treatment plant reactor. Furthermore, the plants situated at the sea coast use seawater as a cooling medium offering re-use of the waste energy within the WW remediation process. Because of its complex chemical composition, with often high concentrations of toxic and growth inhibitory compounds, gas and oil refinery wastewater (ORWW) can represent a challenging medium for microalgae growth. Several studies tested the effect of ORWW dilution on the growth performance of different microalgae, noticing either a drop in biomass concentration with increase in the ORWW loading up to 50%, or increase in productivity due to the algae affinity towards increased salinity (Ammar et al., 2018; Rahman et al., 2020). The ORWW, being rich in nitrogen in the form of , offers a good medium for P. wilhelmii growth and lipid accumulation. Besides growth, the form of the present nitrogen ( and ) can impact the quality of the microalgae biomass, as well as the protein, lipid and carbohydrate content and their ratios (Ruiz et al., 2013). Nitrogen compounds contribute to the toxicity of the effluents, affect pH, and dictate the contamination by (cyano)bacteria, influencing the tolerance of cultivated microalgae. In addition to the high concentrations of ammonium, ORWW contains high concentrations of organic, and inorganic pollutants, such as petroleum hydrocarbons, mercaptans, polycyclic aromatic hydrocarbons (PAHs), benzene homologues, crude oil, heavy metals etc. (Jiang et al., 2010; Blažina et al., 2019).
Multiple studies suggest that some microalgae have high resilience to, and good remediation capacity of, organic pollutants. Calderón-Delgado et al. (2019) reported a decrease in phenols’ and total hydrocarbons’ concentrations in C. vulgaris exposed to 75% of ORWW and 100% of ORWW. Furthermore, marine diatoms were reported to successfully reduce concentrations of heavy metals and other elements harmful to the environment: Ba, Ca, Fe, Mn, Si, As, Sr, Al, Zn, Ni (Godfrey, 2012; Talebi et al., 2016; Al Ghouti et al., 2019), in spite the fact that some elements, such as Zn, damage the photosynthetic apparatus (Öncel et al., 2000). To overcome the problems of the growth inhibiting effects caused by toxic organic and inorganic pollutants, several authors have tested a series of ORWW loadings (e.g. 10 – 50%) for various species, such as Nannochloropsis oculata and Isochrysis galbana (Ammar et al., 2018).
Together with water-saving practices driven by economic drivers, exploitation of fast-growing, halotolerant microalgae strains, capable of removing contaminants of emerging concern from the WW, is of primary interest in bioremediation facilities. However, there is a gap in knowledge about the microalgae response towards multiple toxic effects and the way they affect biomass production and quality.
The primary goal of this study was to explore the capacity of P. wilhelmii for efficient removal from oil refinery WW and for the toxicity reduction of WW rich in contaminants of emerging concern in a microalgae based remediation concept. Further goal of our research was to evaluate potential for biofuel production of the microalgae biomass obtained under various conditions corresponding to the expected realistic industrial operation.
2 Materials and methods
2.1 Experimental design
Marine microalga Pseudochloris wilhelmii (SAG 55.87) was obtained from the Culture Collection of Algae at Gottingen University (SAG) and activated in the saltwater Blue-Green medium (BG-11). The inoculum culture was grown under the standard conditions (100 µmol photon/(m2s), 20°C; De la Vega et al., 2011) and harvested in the early exponential phase. For the experiments, appropriate volumes of the culture (depending on optical density measured at λ=720 nm i.e. OD720) were centrifuged for 10 min at 4900 rpm and the biomass pellets were inoculated into 2.6 L double-walled borosilicate glass photobioreactors (PBRs) with the initial biomass concentration set at 30 mg of dry weight (dw) L-1.
The light intensity, light cycle, temperature and pH (via CO2 flux) were controlled by the SCADA (Supervisory Control And Data Acquisition) system (Figure 1). Air or air/CO2 mixture (97:3 v/v) were injected at the bottom of the reactor through a glass tube. The pH of the culture was maintained at 8.2 during the experiments (regulated additionally by 1 M NaHCO3 when necessary). The reactors were illuminated with LED warm white light at illumination of 80 µmol photon/(m2s) and 130 µmol photon/(m2s). The growth medium in the PBRs was untreated oil refinery wastewater mixed with aged seawater in 1:1 (vol:vol) (both prefiltered on 0.2 µm, Millipore) in order to obtain optimum salinity of 19 psu for P. wilhelmii growth. Furthermore, the process of aging promotes decrease of labile dissolved organic carbon, and level of inorganic N and P below the detection limit of spectrophotometric determination, while preserving the presence of trace elements needed for microalgae growth. Medium was enriched by addition of KH2PO4 stock solution (100 µmol/L) to achieve initial nutrient ratio N:P=8.
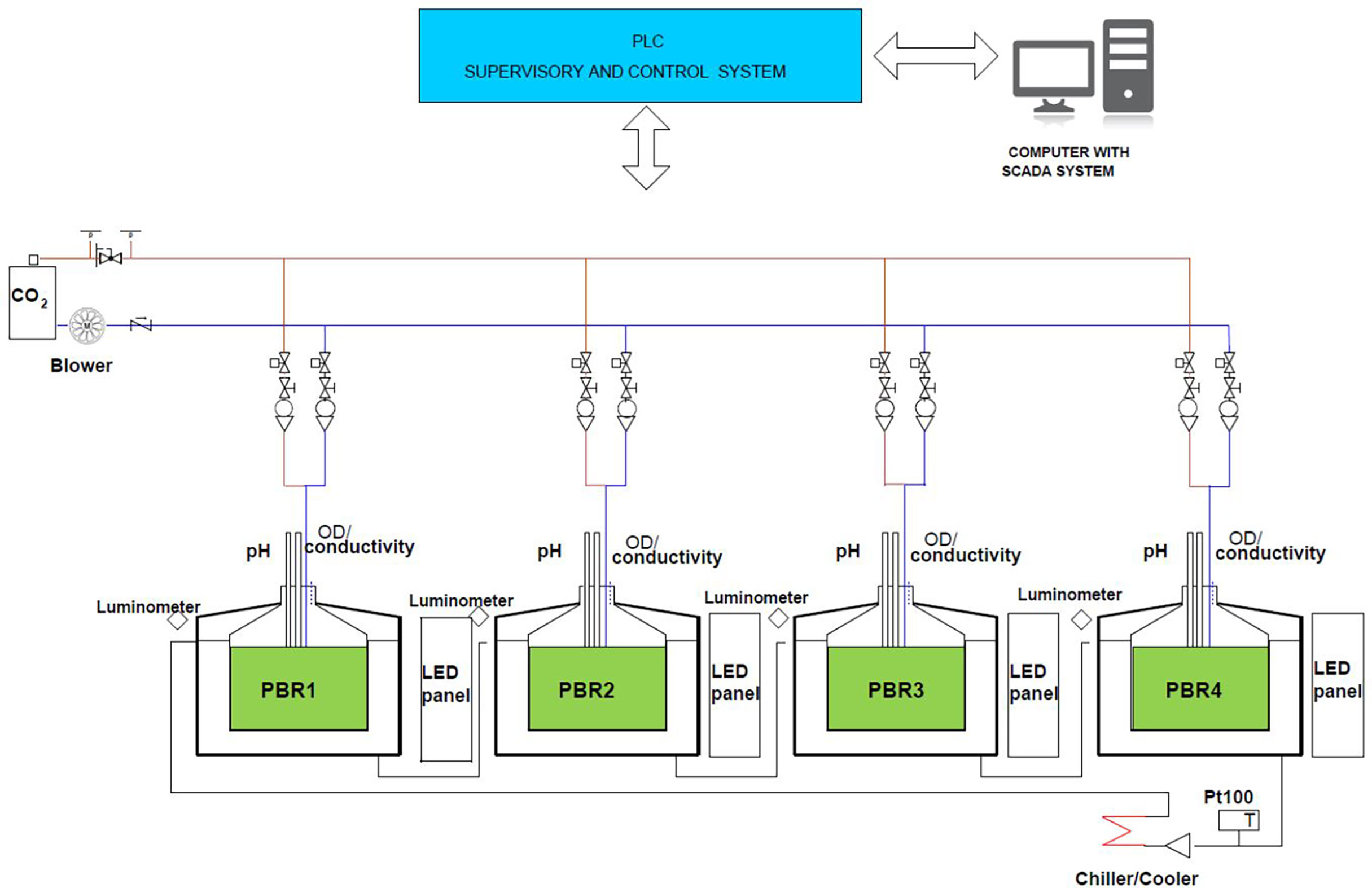
Figure 1 A scheme of 4 photobioreactors (PBR). Temperature, air/CO2 bubbling, pH, light intensity and diurnal cycle are controlled by SCADA system (Blažina et al., 2019).
2.1.1 Growth analysis and estimation of kinetic parameters
A series of growth experiments on biomass production and nutrient consumption rate was run at 20°C, 24°C, 26°C and 30°C to assess the effect of temperature (T) at 12:12 light/dark regime (standard regime) and 130 µmol phot/(m2s). Additionally, (i) growth experiment was conducted at 26°C to assess effects of increased (2x) nitrogen (N) - load (2.6 mmol/l) and prolonged (2x) light exposure period (24 h) on biomass production and dissolved inorganic nitrogen (DIN)-removal; and (ii) growth experiment was performed to test the effect of lower light intensity [80 µmol phot/(m2s)] at 24°C and 30°C (12:12 light/dark regime). The biomass concentration was estimated using optical density (OD720) as a proxy. All the measurements have been performed in triplicates, and the average values were used to present the results. The eventual outlier values among triplicates were omitted, after being detected by Modified z-score method (Iglewicz and Hoaglin, 1993)
The maximum growth rates were calculated from the obtained data using the exponential growth equation (Eq. 1) (Lim et al., 2012):
where X represents biomass concentration calculated from OD720 measurements and t represents time in days since the start of each batch. The maximum growth rate was determined from three consecutive readings that yielded the maximum slope on the biomass-time plot. Confidence intervals for biomass measurements were calculated from their triplicate measurements assuming normal population distributions of the means. Analogous procedure was applied for calculation of maximum specific nutrient DIN- removal (Mennaa et al., 2017).
2.2 Nutrient analysis
Aliquots (50 mL) were sampled for dissolved ammonia (), nitrite (), nitrate () and orthophosphate () in the PBRs. Samples were centrifuged (10 min, 5000 rpm) and the supernatant was immediately analyzed, following the procedures described in Parsons et al. (1984) and Ivančić and Degobbis (1984). When necessary, appropriate dilutions were used to fit the range of spectrophotometric determination (Shimadzu UV 1800).
2.3 Lipid content and GC-MS analysis
Aliquots of cultures (30-50 mL) were filtered on pre-combusted GF/F filters (Whatman) to determine the total lipid content and fatty acid methyl ester (FAME) profile. Filters were dried for 12 hours at 60°C and weighed again. Filters were mechanically disrupted using a tissue homogenizer and total lipids were extracted according to the modified Bligh and Dyer (1959) method in dichloromethane (DCM):methanol (MeOH) in 2:1 (vol/vol) ratio. The extraction procedure was repeated for three 30 min cycles in an ultrasonic water bath at 35°C (Aquasonic 750D). Extract was saponified, methylated and analyzed as described by Haberle et al. (2020). FAME were analyzed by gas–liquid chromatography (GLC) on a 6890N Network GC System equipped with a 5973 Network Mass Selective Detector with a capillary column (30m/0.25 mm/0.25 mm; cross linked 5% phenylmethylsiloxane) and ultra-high purity helium as the carrier gas. The oven temperatures were set to 70°C for 5 minutes, then ramped up to 205°C by 4°C min-1, held at 205°C for 4 min, then ramped up to 270°C by 4°C min-1. Column pressure was constant at 15 psi. Retention times, peak areas and mass spectra were recorded using the ChemStation Software. FAME were identified comparing the retention time with the standard mixtures of fatty acid methyl esters: FAME mix C18-C20, PUFA1, PUFA3 standards (Supelco, USA), C4-C24 FAME standard mix, and various individual pure standards (Sigma, Germany), previously analyzed by gas liquid chromatography-mass spectrometry (GC-MS).
2.4 C:H:N analysis
C:H:N of the algal pellet (obtained from the samples centrifuged for nutrient analysis; see the section 2.2) was determined using a “2400 CHN Elemental Analyzer” (Perkin Elmer, USA). Samples were dried in tin capsules for CHN analysis. The capsules were combusted in a reactor chamber of the CHN analyzer at a combustion temperature of about 990°C with supplemental oxygen boosts of 2s. The product gas mixture flew through a silica reduction tube packed with copper granules. At about 500°C, the remaining oxygen was bound and nitric/nitrous oxides were reduced. The gas stream included analytically important species CO2, H2O and N2. Eventually O2 or hydrohalogenides were absorbed at appropriate traps. High purity helium was used as carrier gas.
2.5 Wastewater toxic potential assessment-Microtox®
The toxic potential of the wastewater-based growth medium was assessed during growth of P. wilhelmii using the marine bioluminescence bacteria Aliivibrio fischeri in Microtox® in vitro testing system. Reduction of bacterial luminescence after exposure to serial dilutions of organic extract in growth medium was measured by commercially available freeze-dried A. fischeri in AZUR 500 luminometer according to the procedure described for seawater (Bihari et al., 2007) and modified for media samples. Briefly, 50 mL culture aliquots were centrifuged at 5000 rpm for 10 minutes. Supernatants of the samples were extracted with 5 ml dichloromethane, evaporated to dryness and dissolved in 50 μL dimethyl sulphoxide (DMSO). Initial testing concentration corresponding to 12.5 mL of growth media sample contained 12.5% WW. A series of eight 1:1 dilutions were incubated with bacteria and luminescence decline was measured after 15 minutes. EC50 values obtained by MicrotoxOmni software were expressed as percentages of WW equivalents present in growth medium with the corresponding 95% confidence intervals.
2.6 P. wilhelmii FAME-derived biodiesel properties
FAME profiles were used for calculation of the ratios between total unsaturated and saturated FAME (UNS/SAT), and the average degree of unsaturation was calculated by summing up all the products of mass fractions of each unsaturated fatty acid with their number of double bonds (Song et al., 2013). Biodiesel characteristics, described by kinematic viscosity (KV), specific gravity (SG), cloud point (CP), ketene number (CN), iodine value (IV) and higher heating value (HHV) were calculated by algorithms proposed in Hoekman et al. (2012) and compared to EN 14214 European Standards of European Committee for Standardization (2012).
3 Results and discussion
In this study, removal of nutrients from oil refinery wastewater (ORWW) was monitored along the growth curve of the marine eukaryotic microalgae Pseudochloris wilhelmii, with emphasis on biomass quality and its suitability for biodiesel production. Optimum temperature conditions for maximum growth were determined from growth experiments at 20°C, 24°C, 26°C and 30°C. The experiments were also used to analyze possibilities of utilizing the seawater used for cooling in the oil refining process as a dilution medium for microalgae growth. The growth curves presented in Figure 2 were used to determine the maximum growth rates using the batch growth rate equation (Eq. 1). Table 1 summarizes experimental conditions in PBRs and the observed basic growth and nutrient removal indices. Experimental temperatures capture both the expected optimal growth range, and expected in situ ORWW temperatures. The chosen light levels [HL = 130 µmol phot/(m2s), LL = 80 µmol phot/(m2s)] reflect the expected two representative levels of light intensity in turbid and dark ORWW. Standard environmental conditions included the diurnal photoperiod (12:12) to capture diurnal light exposure, initial inorganic nitrogen concentration of 1.3 mM and 19 psu salinity. The selected conditions represent average nutrient load of the untreated ORWW, when diluted with the cooling sea water in 1:1 (vol/vol) proportion. Two non-standard experiments with exceptional conditions were run at 26°C and the higher light intensity [130 µmol phot/(m2s)]:
1. 26HL+L, with a constant light exposure (24:0) to assess feasibility of additional artificial lights in reaching higher biomass production, and
2. 26HL+N, with an increased initial inorganic nitrogen concentration (2.6 mM) to mimic 1:1 dilution of the upper limit observed in ORWW, corresponding to a quite usual periodic occurrence in the oil refining practice.
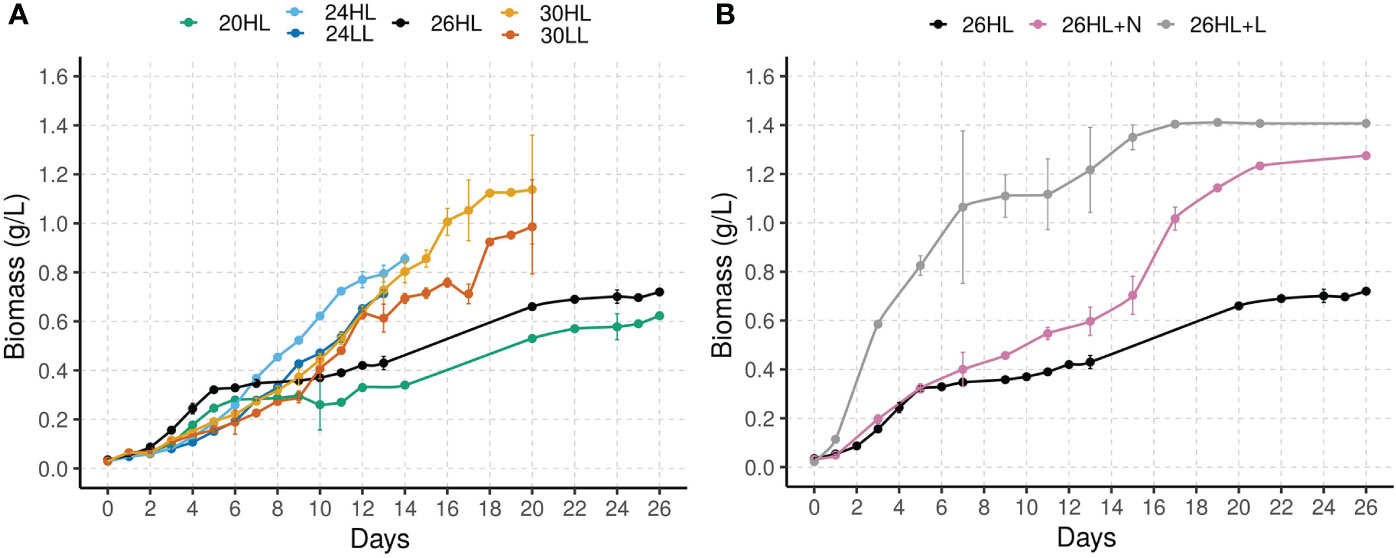
Figure 2 Biomass concentration of P. wilhelmii during batch cultivation under (A) 20°C, 24°C, 26°C and 30°C; c(DIN)= 1.3 mmol/L; light levels 130 µmol phot/(m2s) and 80 µmol phot/(m2s), and (B) at 26°C and 130 µmol phot/(m2s); constant light exposure (24:0) and c(DIN)=1.3 mmol/L; Diurnal light exposure (12:12) and c(DIN)=2.6 mmol/L.
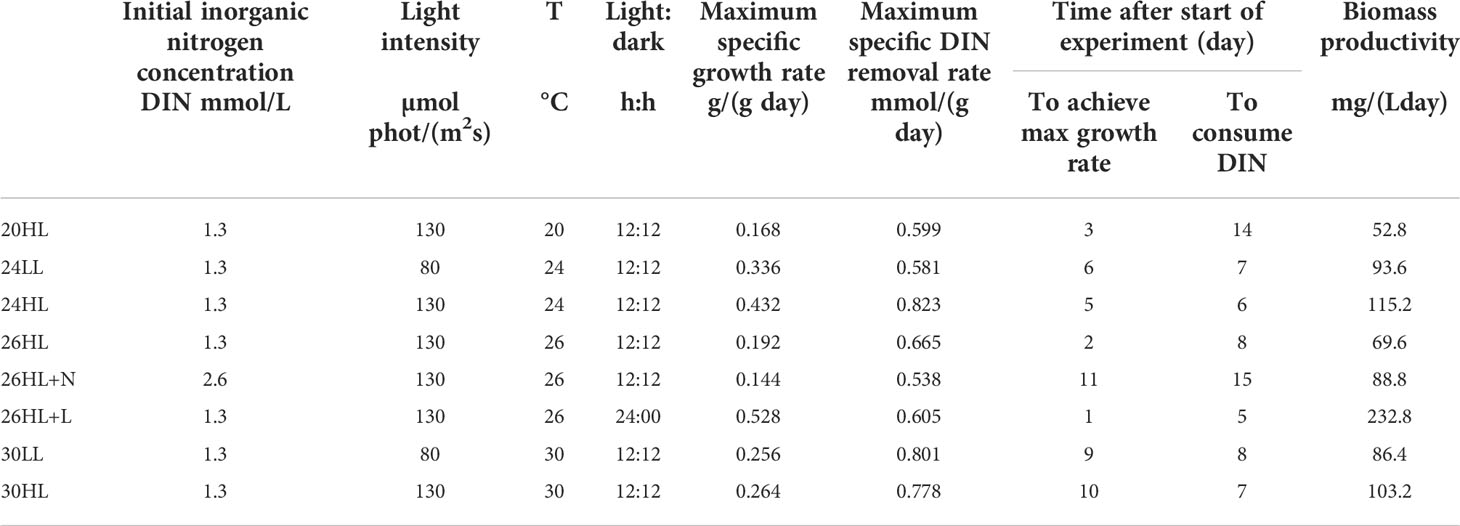
Table 1 Summary of controlled environmental variables and observed basic growth indices in experimental cultures, from the growth and DIN uptake batch-experiments by P. wilhelmii.
Biomass growth. Biomass increased under all experimental conditions (Table 1, Figure 2), despite the exposure to high, potentially toxic concentrations of , as well as other potentially toxic components of ORWW. Both exceptional conditions, the higher nutrient load (26HL+N) and constant light regime (26HL+L), had impact on biomass productivity and N-removal efficiency (Figure 2B). As expected, longer photosynthetic period due to prolonged exposure to light (Table 1, 26HL+L) increased the average rate of biomass synthesis, and ultimately led to the highest yield per day [232.8 mg/(Lday)]. The additional 12 hours of light resulted in the immense 234% increase in daily biomass production compared to the 69.6 mg/(Lday) obtained under the diurnal light regime (26HL). The strong difference is most likely due to the increased ratio of energy allocated to respiration, and the total energy captured by photosynthesis (Inomura et al., 2020). Faster biomass growth in the 26HL+L also increased nitrogen uptake, resulting in almost 40% faster total nitrogen removal from the system in only five days, compared to eight days in 26HL. Doubling the initial inorganic nitrogen concentration (26HL+N vs 26HL) increased final biomass by 77% (Figure 2B), despite only a moderate increase of 28% in the biomass production rate (Table 1), compared to 26HL.
Unsurprisingly, higher light resulted in higher growth rates at both tested temperatures (Figure 2A): 28.6% at 24°C (Table 1, 24HL vs 24LL), and 3.1% at 30°C (Table 1, 30HL vs 30LL). Results for the maximum specific growth rate echo those for the biomass productivity: environmental conditions in experiments 26HL+L and 24HL resulted in the highest algal growth potential, and the highest growth rate.
There are several studies in the literature presenting the productivity data obtained for marine and/or non-commercial strains (De La Vega et al., 2011; Ruiz et al., 2013; Von Alvensleben et al., 2013; Ledda et al., 2015). Most of them deal with municipal or piggery wastewater. However, there are a few studies investigating growth of non-commercial strains on highly toxic industrial waters, such as ORWW. Much higher lipid productivity and growth rates were observed for Picochlorum genus when grown in commercial growth media. However, some previous studies, find lower productivity then observed in our experiment. Von Alvensleben et al. (2013) reported significantly lower biomass productivities for Picochlorum atomus when grown under similar conditions in the artificial growth medium. The compilation of modeling and experimental results by Concas et al. (2019) predicted significantly lower biomass productivities, and maximum biomass obtained, when growing P. wilhelmii on municipal WW mixed with seawater in 1:1 proportion.
We only report the observed maximal growth rates – theoretically, since greatest possible specific growth rates are rarely achieved in experiments, higher growth rates should be possible. (Mennaa et al., 2017). The reported growth rates, therefore, help conservatively estimate maximum specific growth rates that can be used when optimizing industrial scale production in continuous-flow reactors.
Nitrogen removal. Despite the potential ( and other) toxicity of ORWW, inorganic nitrogen has been completely removed in all experiments (Table 1, Figures 3A, B). Standard initial concentration of dissolved inorganic nitrogen (1.3 mM) was removed in 5-8 days in experiments with favorable growth temperatures (24 - 30°C). Increasing light intensity increased nitrogen removal by about 15% for both 24°C and 30°C, thus reducing the number of days until removal: from 7 days (24LL) to 6 days (24HL) at 24°C, and 8 days (30LL) to 7 days (30HL) at 30°C. The experiment 24HL yielded the highest observed inorganic nitrogen removal rate of 0.823 µmolDIN/(mgday) among all diurnal light cycle experiments, accompanied by the corresponding biomass productivity of 115.2 mg/(Lday). These results indicate the optimum temperature for combination of fast bioremediation and biomass growth.
Increase in nutrient load decreases the maximum specific growth rate and the maximum specific DIN removal rate, almost doubling the time for bioremediation process (Table 1, 26HL+N vs 26HL, 8 vs 15 days) thus indicating that the removal efficiency could be largely independent of the initial inorganic nitrogen load. Doubling the nutrient load resulted in only 25% lower maximum specific growth rate and 28 % higher biomass productivity. In contrast, constant light exposure expedited the nitrogen removal, (i.e. bioremediation process) by almost 40%, while supporting over 3 times higher biomass productivity and the highest maximum specific growth rate of 0.528 g/(gday).
Influence of auxiliary water parameters. Results on biomass growth and nitrogen removal generally follow the expected physiological responses of phytoplankton cells to variations in culture conditions. However, analysis of the results under the same light and nutrient conditions, highlighted some inconsistencies. Although experiments performed at 20°C (20HL) and 26°C (26HL) reached maximum growth rates much sooner than experiments at 24°C (24HL) and 30°C (30HL) their overall growth dynamics and final biomass concentration were significantly lower. This resulted in the unexpected shape of the thermal performance curve, which predicts that growth at 26°C should be higher than at 24°C and 30°C (Figure 2B). Additionally, the experiments performed at 20°C (20HL) and 26°C (26HL) have a steady increase in biomass after day 10, while experiments at 24°C (24HL) and 30°C (30HL) do not. The difference in the growth dynamics and final biomass yield can be attributed to the different ORWW sampling dates, even though the ORWW used in experiments originated from the same process and same oil refining plant. Experiments 20HL and 26HL used samples collected in summer, while 24HL and 30HL used samples collected in spring (Table 2). While ORWW was controlled for parameters deemed important for algal growth (i.e. concentration and temperature – see methods for details), results suggest that other – auxiliary water parameters may affect algal growth. For example, auxiliary water parameters may influence phytoplankton growth by: i) providing additional nutrients in organic form that promote algal growth, and/or ii) diminishing growth by toxicity. We investigated this further by conducting CHN analysis and toxicity tests.
Intracellular C, N composition. CHN analysis results (Figure 3) track nitrogen incorporation into the microalgae, synthesis of cellular carbon, and the C/N ratio of the cell. Removal of inorganic nitrogen results in an increase in cellular nitrogen and carbon on both ORWW waters, but the dynamics of accumulation are different. On spring ORWW, the accumulation is unlimited (Figures 3A, C), while on summer water the entry into the stationary phase is visible after day 10 (Figures 3B, D). Unlimited accumulation of carbon is not surprising, since it can accumulate due to photosynthesis for more than 10 days after nitrogen depletion (Omta et al., 2017; Inomura et al., 2020), but the continuing increase in cellular nitrogen after removal of dissolved nitrogen from the medium is quite surprising.
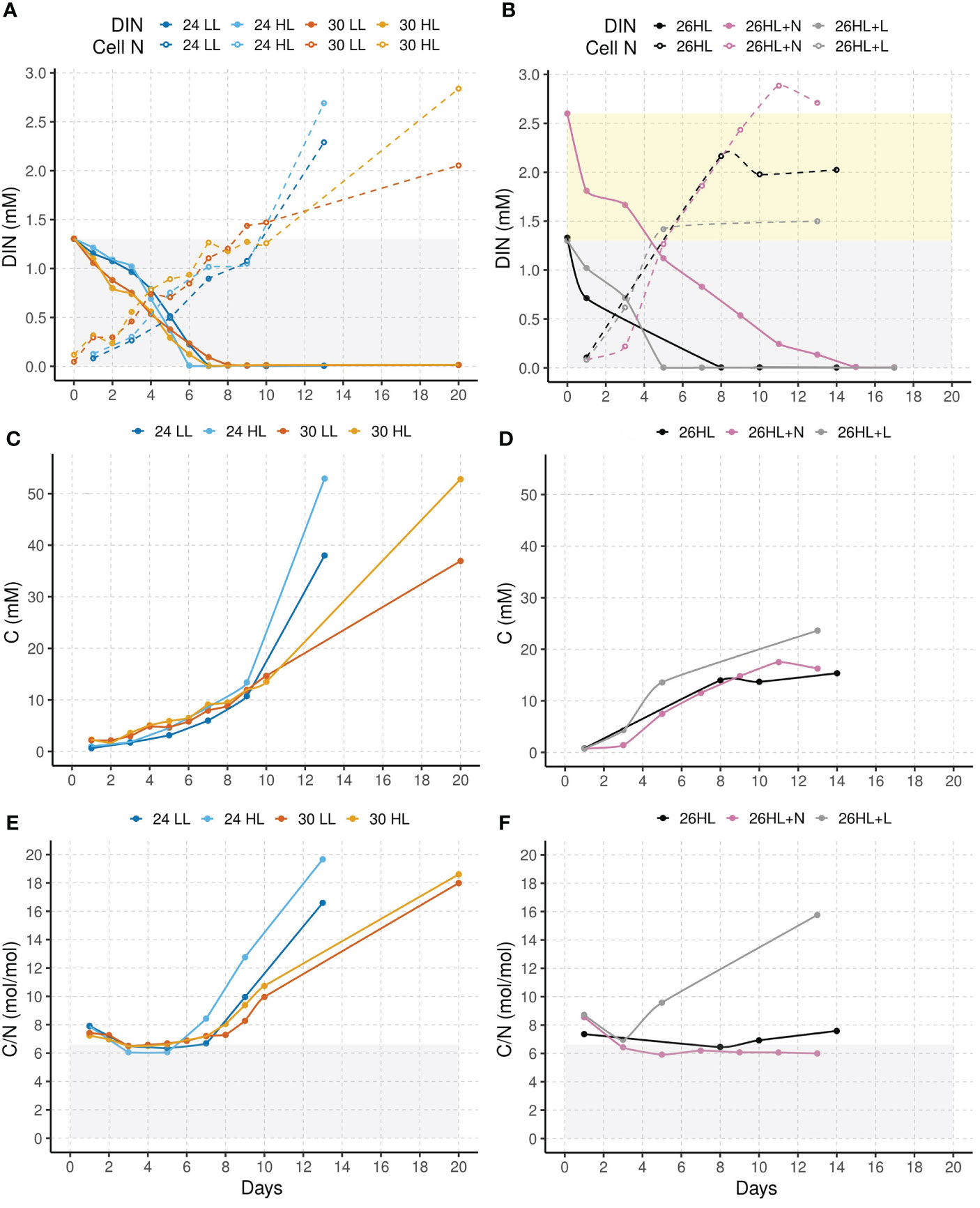
Figure 3 Nitrogen and carbon dynamics in batch-experiments by P. wilhelmii, grown in ORWW sampled in spring (left) and summer (right). Dissolved inorganic nitrogen and cellular N (A, B), cellular C (C, D), and cellular C/N ratio (E, F).
In both ORWW samples, observed cellular nitrogen exceeds the expected limit of maximum nitrogen incorporation defined by the initial inorganic nitrogen concentration (Figure 3A, B). Therefore, we hypothesize that both ORWW samples had some nitrogen of organic origin taken up by the cell. Since the increase in nitrogen lasts longer in spring than in summer ORWW samples, we conclude that the amount of organic nitrogen in spring ORWW sample was higher. This is consistent with higher COD measurements of the spring sample (Table 2). The uptake of organic nitrogen implies that P. wilhelmii could be using mixotrophic strategies. It was previously claimed that all the marine Nannochloris species, including our strain, are obligate photoautotrophs unable to metabolize organic carbon while using light as the only source of energy (Droop, 1974). However, recent findings by Pang et al. (2022) and Goswami et al. (2022) reported evidence of mixotrophy of novel Picochlorum sp. (Trebouxiophyceae strains). Therefore, our strain could be mixotrophic, too. The apparent ability of P. wilhelmii to remove organic nitrogen-containing contaminants from wastewater in addition to the proven ability to remove inorganic nitrogen is a significant feature for the use of this alga in the bioremediation of ORWW.
Intracellular C/N ratio in spring ORWW (Figure 3E) follows the expected pattern: the ratio stagnates around the Redfield ratio until inorganic nitrogen is removed from the water. Then, the ratio increases because photosynthesis continues to add carbon, while nitrogen levels remain constant (Liefer et al., 2019). Summer ORWW sample (Figure 3F), in contrast, does not yield such a pattern except for algae grown with a continuous light (26HL+L). The fact that longer light induces additional carbon sequestration, while shorter light does not, indicates that shorter light does not provide enough energy to achieve surplus carbon sequestration even though it does provide enough energy for growth. Intricacies of the underlying mechanism(s) warrant additional research.
Lipid composition. GC-MS analysis of the algae biomass, at both illumination intensities, and at temperatures 24°C and 30°C, revealed higher lipid accumulation compared to the healthy growing inoculum culture, ranging from 24.4 to 29.9% of lipid content in dry algal biomass (Table 3). Final lipid concentrations in the cultures, depending on light and temperature, ranged from 181.5 to 319.8 mg/L. The obtained results are comparable to those reported for Pseudoneochloris marina grown under various light and temperature conditions in based medium (Gonçalves et al., 2019). Higher FAME saturation and increase of short carbon chains in the molecular structure, were observed for cultures grown in ORWW.
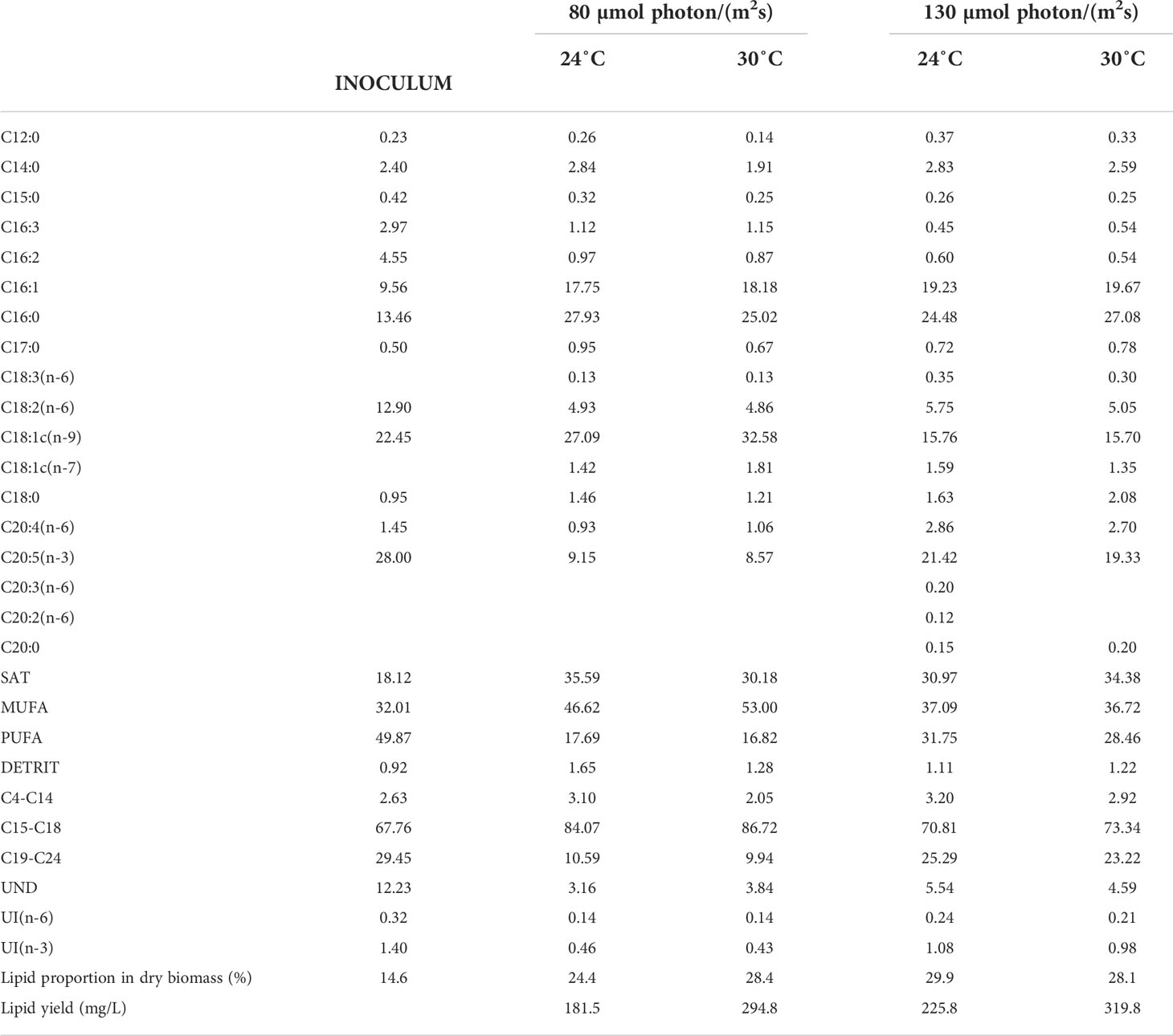
Table 3 Total lipid content, fatty acid profile and relevant FAME classes’ proportion of P. wilhelmii biomass grown under 24°C and 30°C; c(DIN) = 1.3 mmol/L; light levels 130 µmol phot/(m2s) and 80 µmol phot/(m2s), and diurnal (12:12) light cycle.
The unsaturation index (UND), one of the most important parameters determining biodiesel quality, demonstrated drastically lower values for P. wilhelmii grown in ORWW than in the commercial medium, ranging between 3.16–5.54 (compared to 12.23, Table 3). The highest proportion of saturated FAs of 35.59%, was found in the 24LL (Table 3, SAT), and the lowest polyunsaturated FA proportion of 16.82%, was observed in 30LL (Table 3, PUFA). According to the previous findings by Concas et al. (2019), P. wilhelmii (SAG 55.87) is characteristic of its long-chain FAME composition and high proportion of PUFA (> 20%). Herein we confirmed these findings. Furthermore, PUFA content in FAME of the healthy growing culture (inoculum) was 49.87%, with a particularly high proportion of 28% of eicosapentaenoic fatty acid (20:5n-3, EPA), known as essential (omega-3) fatty acid, important in human nutrition and health. Nevertheless, the highest proportions of C18:1, which is the most desirable FAME for biodiesel production, were found in the lipids obtained from the algae cultivated at 24°C (Table 3, C18:1c(n-9) + C18:1c(n-7); 28.5 and 34.4%). The causes of saturation during growth on toxic ORWW can be found in its composition (Table 2). The result is supported by the previously established findings that exposure to heavy metals, such as Cd, causes a decrease in the C18:3 levels by 12% and more, along with the increase in more saturated FA, such as C16:0, C18:1 and C18:2 (Chaffai and Cherif, 2020). Phenolic substances, PAHs, and other organics present in WW, are known to form free radicals capable of attacking multiple double bonds of the highly unsaturated microalgal fatty acids, and causing their peroxidation (Concas et al., 2019). The adaptation mechanisms maintaining membrane permeability and function of the photosynthetic apparatus, lead to the algae resilience over a wide range of toxicants’ concentrations, including PAHs and heavy metals (El Maghraby and Hassan, 2021). Thus the decrease in growth rate and lipid yield is to be expected.
Biodiesel production potential. The most pronounced changes in FA composition are shortening of the C-chain length and reduction in the number of double bonds (increase in saturation). These characteristics are important indicators of FAME quality, responsible for suitability of the biomass for biodiesel production (Budiša et al., 2019; Haberle et al., 2020). The main criteria used for biodiesel quality assessment is the average degree of unsaturation (ADU), developed by Islam et al. (2013). Biodiesel properties are estimated from the molecular structure of the FAME taking into account the acyl chain length, as well as quantity and position of unsaturated bonds (Table 4). A decrease in proportion of long-chain polyunsaturated fatty acids (C19-C22) compared to healthy growing culture (9.94 – 25.29%), resulted in ADU range 1.13 – 2.22.
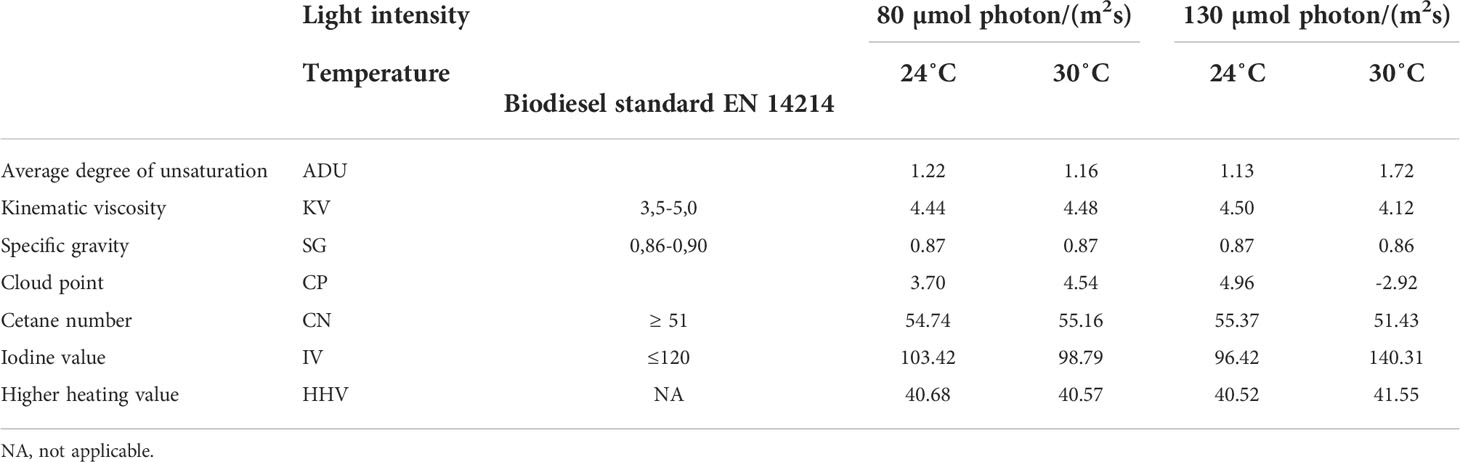
Table 4 Biodiesel properties calculated from the FAME profile of P. wilhelmii grown under 24°C and 30°C; c(DIN) = 1.3 mmol/L; light levels 130 µmol phot/(m2s) and 80 µmol phot/(m2s), and diurnal (12:12) light cycle.
The FAME obtained from the biomass grown in ORWW under all tested conditions demonstrated satisfactory kinematic viscosity and specific gravity. The values for cetane number (CN), one of the most important parameters indicating time delay in the ignition of fuel for diesel cycle engines, were also satisfactory at all light irradiances and 24°C and 30°C. Under 30HL the iodine value (IV 140.31, indicative of high degree of unsaturation, together with ADU), falls out of the regulatory limits (≤120). These traits classify cultivation of P. wilhelmii biomass at 30°C and illumination intensity 130 µmol phot/(m2s) as unfavorable for the production of biodiesel due to the higher polymerizing tendency as a consequence of the high number of double bonds. Our previous research demonstrated excessively high iodine values obtained for P. wilhelmii at lower temperatures (18°C) and unsatisfactory biodiesel parameters (Budiša et al., 2019). Therefore, the optimum conditions that would satisfy all criteria according to the EN 14214 standard are met at 24°C at both tested light irradiances in a range of 80 and 130 µmol phot/(m2s), and at 30°C at the lower tested light irradiance, 80 µmol phot/(m2s).
Toxicity reduction potential. High concentrations of ammonium, because of its natural dissociation into ammonia can be toxic and growth inhibiting to the microalgae in general (Bower and Bidwell, 1978). Our previous research has reported P. wilhelmii to have a high tolerance and even substantial affinity towards as a nutrient source (Budiša et al., 2019). However, the presence of highly toxic compounds in the ORWW certainly causes other cell damage, and cytotoxic and genotoxic effects that inhibit growth and slow down cell division.
We assessed the change in toxicity of ORWW due to the P. wilhelmii metabolic activity using Microtox® system, a well-known and effective tool in detecting effluent toxicity (Araujo et al., 2005). The results are presented in Table 5. Decrease in toxicity of the ORWW reached in a stationary phase, compared to the ORWW toxicity prior to inoculation, is expressed as percentage. The most efficient toxicity reduction of 76.5% was observed in experiment 24HL. Lower light irradiance [80 µmol phot/(m2s)] in experiments 24LL and 30LL also resulted in toxicity decrease, 59.6 and 67.6%, respectively. The least expressed reduction in toxicity was observed in experiment 30HL. Note that the major difference between 30°C and 24°C experiments was in the period of cultivation. Since the Microtox is a reflection of a general toxicity of medium, it cannot provide information about the origin of toxicity, i.e. the composition of the toxic substances causing the growth inhibition of A. fischeri. It is plausible that during days 14-20 the bacterial, as well as P. wilhelmii, secondary toxic metabolites accumulate in the medium shadowing the toxicity reduction effect (Costelli, 2014). Although there is no record of P. wilhelmii being able to synthesize toxic metabolites, some strains of the Picochlorum genus were reported to have been avoided by copepods as a food source (Ma et al., 2021), or to express cytotoxic activity against cancer cell line (Abolhasani et al., 2018). Since P. wilhelmii has been shown as promising strain for cultivation and isolation of valuable bioproducts, a deeper insight into the toxicity aspects is needed, particularly in a light of the adverse Microtox® results presented herein, as well as literature reports.
4 Conclusion
Pseudochloris wilhelmii showed high potential for oil refinery wastewater remediation and large-scale production of biomass suitable for biodiesel. Growth on ORWW resulted in substantial decrease of polyunsaturated FA proportions and increase in monounsaturated and saturated FA proportions, favoring final lipid quality for biodiesel production, in adherence to the EN 14214 standard. The conditions favoring the highest nitrogen removal and highest toxicity reduction in ORWW are met at 24°C and 130 µmol phot/(m2s) irradiance with the 12:12 light/dark regime. Under the same conditions the highest proportion of carbon-binding to the P. wilhelmii biomass is noticed indicating these conditions as the most favorable for hydrocarbon removal as well as for CO2 sequestration purposes. Generally, results suggest that P. wilhelmii might have mixotrophic capabilities in ORWW, and exhibits high potential for remediation of heavily contaminated wastewaters rich in organic and inorganic pollutants. However, the remediation capacity and biomass productivity strongly depend on auxiliary compounds present in wastewater, such as hydrocarbons, mercaptanes, and sulfides. Clearly, Pseudochloris wilhelmii is a promising candidate for ORWW remediation and valuable biomass cogeneration on a large-scale. Nevertheless, for an economically sustainable biodiesel production the biomass productivity and growth rate should be further improved, through physiological modeling, and molecular engineering.
Data availability statement
The raw data supporting the conclusions of this article will be made available by the authors, without undue reservation.
Author contributions
MB: Funding acquisition, Project administration, Supervision, Conceptualization, Data curation, Writing - original draft. TK: Conceptualization, Investigation, Data curation, Writing - review and editing. EH: Investigation, Formal analysis, Data curation, Writing - review and editing. SG: Formal analysis, Data curation. IH: Investigation, Formal analysis, Data curation. EP: Investigation. JK: Conceptualization, Methodology. LH: Resources, Investigation. MF: Conceptualization, Writing - review and editing. LZ: Investigation. All authors contributed to the article and approved the submitted version.
Funding
This work is fully supported by the Croatian Science Foundation under the project number PKP-06-2016-9081 (Assessment of Adriatic Algae Potential in Cogeneration Production of 3rd Generation Biofuel). The project is funded under the program of the Government of the Republic of Croatia to encourage research and development activities in the area of climate change.
Acknowledgments
The authors are thankful to Andrea Budiša, Darko Ferenčević and Jelena Godrijan for the technical assistance during the experimental part of the study. The support of the INA-INDUSTRIJA NAFTE, Plc., Croatian oil industry, for data provision, CHN analyses and supply of the wastewater is highly acknowledged.
Conflict of interest
Author LH is employed by INA-INDUSTRIJA NAFTE, Plc., Zagreb.
The remaining authors declare that the research was conducted in the absence of any commercial or financial relationships that could be construed as a potential conflict of interest.
Publisher’s note
All claims expressed in this article are solely those of the authors and do not necessarily represent those of their affiliated organizations, or those of the publisher, the editors and the reviewers. Any product that may be evaluated in this article, or claim that may be made by its manufacturer, is not guaranteed or endorsed by the publisher.
Footnotes
- ^ psu - practical salinity unit, corresponding to g of NaCl / kg water, dimensionless and equal to ‰
References
Abolhasani M. H., Safavi M., Goodarzi M. T., Kassaee S. M., Azin M. (2018). Identification and anti-cancer activity in 2D and 3D cell culture evaluation of an Iranian isolated marine microalgae Picochlorum sp. RCC486. DARU. J. Pharm. Sci. 26, 105–116. doi: 10.1007/s40199-018-0213-5
Al Ghouti M. A., Al-Kaabi M. A., Asfhaq M. Y., Da’na D. A. (2019). Produced water characteristics, treatment and reuse: A review. J. Water Process Eng. 28, 222–239. doi: 10.1016/j.jwpe.2019.02.001
Ammar S. H., Khadim K. J., Mohamed A. I. (2018). Cultivation of Nannochloropsis oculata and Isochrysis galbana microalgae in produced water for bioremediation and biomass production. Environ. Technol. Innov. 10, 132–142. doi: 10.1016/j.eti.2018.02.002
Araujo C. V. M., Nascimento R. B., Oliveira C. A., Strotmann U. J., da Silva E. M. (2005). The use of Microtox to assess toxicity removal of industrial effluents from the industrial district of Camaçari (BA, Brazil). Chemosphere 38, 1277–1281. doi: 10.1016/j.chemosphere.2004.10.036
Bihari N., Fafanđel M., Piškur V. (2007). Polycyclic aromatic hydrocarbons and ecotoxicological characterization of seawater, sediment, and mussel Mytilus galloprovincialis from the Gulf of Rijeka, the Adriatic Sea, Croatia. Arch. Environ. Contamin. Toxicol. 52, 379–387. doi: 10.1007/s00244-005-0259-5
Blažina M., Haberle I., Hrustić E., Budiša A., Petrić I., Konjević L., et al. (2019). Growth aspects and biochemical composition of Synechococcus sp. MK568070 cultured in oil refinery wastewater. J. Mar. Sci. Eng. 7 (6), 164. doi: 10.3390/jmse7060164
Bligh E. G., Dyer W. J. (1959). A rapid method for total lipid extraction and purification. Can. J. Biochem. Physiol. 37, 911–917. doi: 10.1139/y59-099
Bower C. E., Bidwell J. P. (1978). Ionization of ammonia in seawater: Effects of temperature, pH, and salinity. J. Fish. Res. Board Can. 35, 1012–1016. doi: 10.1139/f78-165
Budiša A., Haberle I., Konjević L., Blažina M., Djakovac T., Lukarić-Špalj B., et al. (2019). Marine microalgae Microchloropsis gaditana and Pseudochloris wilhelmii cultivated in oil refinery wastewater – a perspective on remediation and biodiesel production. Fresenius Environ. Bull. 28, 7888–7897.
Calderón-Delgado I. C., Mora-Solarte D. A., Velasco-Santamariía Y. M. (2019). Physiological and enzymatic responses of Chlorella vulgaris exposed to produced water and its potential for bioremediation. Environ. Monit. Assess. 139, 399. doi: 10.1007/s10661-019-7519-8
Chaffai R., Cherif A. (2020). The cadmium-induced changes in the polar and neutral lipid compositions suggest the involvement of triacylglycerol in the defense response in maize. Physiol. Mol. Biol. Plants 26 (1), 15–23. doi: 10.1007/s12298-019-00734-9
Concas A., Lutzu G. A., Pisu M., Cao G. (2019). On the feasibility of Pseudochloris wilhelmii cultivation in sea-wastewater mixtures: Modeling and experiments. J. Environ. Chem. Eng. 7, 103301. doi: 10.1016/j.jece.2019.103301
Costelli C. (2014). Genetic and phylogenetic characterization of microalgae strains in view of their exploitation for CO2 capture and biofuel production. PhD thesis (Cagliari, IT: Università degli Studi di Cagliari).
De la Vega M., Díaz E., Vila M., León R. (2011). Isolation of a new strain of Picochlorum sp. and characterization of its potential biotechnological applications. Biotechnol. Prog. 27, 1535–1543. doi: 10.1002/btpr.686
Droop M. R. (1974). Heterotrophy of carbon In: Stewart W. D. P., ed. Algal physiology and biochemistry. Berkeley, CA, USA: University of California Press, 530–559.
El Maghraby D. M., Hassan I. A. (2021). Photosynthetic and biochemical response of ulva lactuca to marine pollution by polyaromatic hydrocarbons (PAHs) collected from different regions in Alexandria city, Egypt. Egypt. J. Bot. 61 (2), 467–478. doi: 10.21608/ejbo.2021.37571.1531
Godfrey V. (2012). Production of biodiesel from oleaginous organisms using underutilized wastewaters. Master's Thesis (UT, USA: Utah State University, Logan).
Gonçalves C. F., Menegol T., Rech R. (2019). Biochemical composition of green microalgae Pseudoneochloris marina grown under different temperature and light conditions. Biocatal. Agric. Biotechnol. 18, 101032. doi: 10.1016/j.bcab.2019.101032
Goswami R. K., Mehariya S., Karthikeyan O. P., Verma P. (2022). Influence of carbon sources on biomass and biomolecule accumulation in Picochlorum sp. cultured under the mixotrophic condition. Int. J. Environ. Res. Public Health 19, 3674. doi: 10.3390/ijerph19063674
Haberle I., Hrustić E., Petrić I., Pritišanac E., Šilović T., Magić L., et al. (2020). Adriatic cyanobacteria potential for cogeneration biofuel production with oil refinery wastewater remediation. Algal Res. 50, 101978. doi: 10.1016/j.algal.2020.101978
Hoekman S. K., Broch A., Robbins C., Ceniceros E., Natarajan M. (2012). Review of biodiesel composition, properties, and specifications. Renew. Sustain. Energy Rev. 16, 143–169. doi: 10.1016/j.rser.2011.07.143
Iglewicz B., Hoaglin D. C. (1993). “The ASQC basic references in quality control: Statistical techniques,” in How to detect and handle outliers, vol. 16 . Ed. Mykytka E. F. (Milwaukee: ASQC Quality Press), 1–87.
Inomura K., Omta A. W., Talmy D., Bragg J., Deutsch C., Follows M. J. (2020). A mechanistic model of macromolecular allocation, elemental stoichiometry, and growth rate in phytoplankton. Front. Microbiol. 11, 86. doi: 10.3389/fmicb.2020.00086
Islam M. A., Magnusson M., Brown R. J., Ayoko G. A., Nabi M. N., Heimann K. (2013). Microalgal species selection for biodiesel production based on fuel properties derived from fatty acid profiles. Energies 6, 5676–5702. doi: 10.3390/en6115676
Ivančić I., Degobbis D. (1984). An optimal manual procedure for ammonia analysis in natural waters by the indophenol blue method. Water Res. 18, 1143–1147. doi: 10.1016/0043-1354(84)90230-6
Jiang Z., Huang Y., Xu X., Liao Y., Shou L., Liu J., et al. (2010). Advance in the toxic effects of petroleum water accommodated fraction on marine plankton. Acta Ecol. Sin. 30, 8–15. doi: 10.1016/j.chnaes.2009.12.002
Ledda C., Villegas G. I. R., Adani F., Fernández F. G. A., Grima E. M. (2015). Utilization of centrate from wastewater treatment for the outdoor production of Nannochloropsis gaditana biomass at pilot-scale. Algal Res. 12, 17–25. doi: 10.1016/j.algal.2015.08.002
Liefer J. D., Garg A., Fyfe M. H., Irwin A. J., Benner I., Brown C. M., et al. (2019). The macromolecular basis of phytoplankton C:N:P under nitrogen starvation. Front. Microbiol. 10, 763. doi: 10.3389/fmicb.2019.00763
Lim D. K. Y., Garg S., Timmins M., Zhang E. S., Thomas-Hall S. R., Schuhmann S., et al. (2012). Isolation and evaluation of oil-producing microalgae from subtropical coastal and brackish waters. PloS One 7, e40751. doi: 10.1371/journal.pone.0040751
Maity J. P., Hou C. P., Majumder D., Bundschuh J., Kulp T. R., Chen C. Y., et al. (2014). The production of biofuel and bioelectricity associated with wastewater treatment by green algae. Energy 78, 94–103. doi: 10.1016/j.energy.2014.06.023
Ma X., Jacoby C. A., Johnson K. B. (2021). Grazing by the copepod Parvocalanus crassirostris on Picochlorum sp. at harmful bloom densities and the role of particle size. Front. Mar. Sci. 8, 664154.
Mennaa F. Z., Arbib Z., Perales J. A. (2017). Urban wastewater photobiotreatment with microalgae in a continuously operated photobioreactor: growth, nutrient removal kinetics and biomass coagulation-flocculation. Environ. Technol., 40 1479–487X. doi: 10.1080/09593330.2017.1393011
Oüncel I., Keles Y., Uüstün A. S. (2000). Interactive effects of temperature and heavy metal stress on the growth and some biochemical compounds in wheat seedlings. Environ. pollut. 107, 315–320. doi: 10.1016/S0269-7491(99)00177-3
Omta A. W., Talmy D., Sher D., Finkel Z. V., Irwin A. J., Follows M. J. (2017). Extracting phytoplankton physiological traits from batch and chemostat culture data. Limnol. Oceanogr. Methods 15, 453–466. doi: 10.1002/lom3.10172
Pang M., Liu K., Liu H. (2022). Evidence for mixotrophy in pico-chlorophytes from a new Picochlorum (Trebouxiophyceae) strain. J. Phycol. 58, 80–91. doi: 10.1111/jpy.13218
Parsons T. R. (1984). A manual of chemical & biological methods for seawater analysis (New York: Pergamon Press).
Rahman A., Agrawal S., Nawaz T., Pan S., Selvaratnam T. (2020). Review of algae-based produced water treatment for biomass and biofuel production. Water 12, 2351. doi: 10.3390/w12092351
Ruiz J., Álvarez-Díaz P. D., Arbib Z., Garrido-Pérez C., Barragán J., Perales J. A. (2013). Performance of a flat panel reactor in the continuous culture of microalgae in urban wastewater: Prediction from a batch experiment. Biores. Technol. 127, 456–463. doi: 10.1016/j.biortech.2012.09.103
Song M. M., Pei H. Y., Hu W. R., Ma G. X. (2013). Evaluation of the potential of 10 microalgal strains for biodiesel production. Biores. Technol. 141, 245–251. doi: 10.1016/j.biortech.2013.02.024
Talebi A. F., Dastgheib S. M. M., Tirandaz H., Ghafari A., Alaie E., Tabatabaei M. (2016). Enhanced algal-based treatment of petroleum produced water and biodiesel production. RSC Adv. 6, 47001–47009. doi: 10.1039/C6RA06579A
Keywords: marine micro algae, Pseudochloris wilhelmii, wastewater, toxicity, phycoremediation, biodiesel
Citation: Blažina M, Fafanđel M, Geček S, Haberle I, Klanjšček J, Hrustić E, Husinec L, Žilić L, Pritišanac E and Klanjscek T (2022) Characterization of Pseudochloris wilhelmii potential for oil refinery wastewater remediation and valuable biomass cogeneration. Front. Mar. Sci. 9:983395. doi: 10.3389/fmars.2022.983395
Received: 01 July 2022; Accepted: 02 September 2022;
Published: 04 October 2022.
Edited by:
Maja Berden Zrimec, Algal Technology Centre, SloveniaReviewed by:
Tonmoy Ghosh, Indian Institute of Technology Indore, IndiaKit Wayne Chew, Xiamen University, Malaysia
Copyright © 2022 Blažina, Fafanđel, Geček, Haberle, Klanjšček, Hrustić, Husinec, Žilić, Pritišanac and Klanjscek. This is an open-access article distributed under the terms of the Creative Commons Attribution License (CC BY). The use, distribution or reproduction in other forums is permitted, provided the original author(s) and the copyright owner(s) are credited and that the original publication in this journal is cited, in accordance with accepted academic practice. No use, distribution or reproduction is permitted which does not comply with these terms.
*Correspondence: Maria Blažina, bWJsYXppbmFAaXJiLmhy
†These authors have contributed equally to this work