Response of benthic foraminifera to environmental successions of cold seeps from Vestnesa Ridge, Svalbard: Implications for interpretations of paleo-seepage environments
- 1Centre of Arctic Gas Hydrate, Environment and Climate (CAGE), Department of Geosciences, UiT The Arctic University of Norway, Tromsø, Norway
- 2Fram Centre, Akvaplan-niva AS, Tromsø, Norway
- 3Department of Earth, Planetary, and Space Sciences, University of California Los Angeles, Los Angeles, CA, United States
- 4Department of Atmospheric and Oceanic Sciences, University of California Los Angeles, Los Angeles, CA, United States
- 5GEOMAR Helmholtz Centre for Ocean Research Kiel, Kiel, Germany
- 6Department of Paleoceanography, Institute of Oceanology of Polish Academy of Sciences, Sopot, Poland
This paper presents the results of a study on the response of living benthic foraminifera to progressing environmental successions in a cold-seep ecosystem. Sediment samples were collected from Vestnesa Ridge (79°N, Fram Strait) at ~1200 m water depth. The distribution of live (Rose Bengal-stained) foraminifera were analyzed in the upper sediment layers in relation to pore water biogeochemical data together with the distribution of sulfur-bacterial mats and Siboglinidae tubeworms. At methane cold seeps, the process of environmental succession is strongly connected to the duration and strength of methane seepage and the intensity of methane-related biological processes, e.g, aerobic and anaerobic oxidation of methane (MOx and AOM, respectively). The results show that the distribution patterns of benthic foraminifera change according to the progressing environmental succession. The benthic foraminifera seemed to thrive in sediments with a moderate activity of seepage, dominated by MOx, i.e, at an early stage of seepage or when seepage decreases at a late stage of the succession. Species composition of the foraminiferal fauna under these conditions was similar to the control sites (outside of pockmarks with no seepage); the dominant species being Melonis barleeanus and Cassidulina neoteretis. In sediments with strong seepage and high AOM activity, the hostile environmental conditions due to the presence of toxic sulfide caused a reduction in the foraminiferal population, and samples were almost barren of foraminifera. In environments of moderate methane seepage, the presence of chemosynthetic Siboglinidae tube worms potentially support communities of the epibenthic species Cibicidoides wuellerstorfi. Despite the very different environmental conditions, the foraminiferal assemblages were very similar (or nearly absent). Therefore, the foraminiferal faunas cannot be used as exclusive indicators of past strength of methane seepage in palaeoceanographic interpretations.
Introduction
Benthic foraminifera are unicellular organisms, commonly occurring in a variety of marine environments. Information about the distribution and abundance of foraminiferal species carry a valuable record of past oceanic conditions, thus they are commonly used as tools in paleoclimatic and –oceanographic reconstructions (Murray, 2006; Gooday and Jorissen, 2012). In recent years, cold-seep benthic foraminifera have gained increased attention due to their potential to record changes in past degrees of methane seepage (Torres et al., 2003; Millo et al., 2005; Bernhard et al., 2010; Martin et al., 2010; Consolaro et al., 2015; Schneider et al., 2017; Sztybor and Rasmussen, 2017). There is a link between the release of methane from geological reservoirs and climate change, e.g, during the Quaternary and the Paleocene periods (Wefer et al., 1994; Dickens et al., 1997; Smith et al., 2001). A large amount of natural gas is stored in Arctic marine sediments in the form of gas hydrates or free gas. It is important to understand the fate of methane in marine sediments to understand the potential impact of ongoing methane release on Arctic ecosystems. Methane seepage from subseafloor reservoirs is manifested by the presence of cold seep ecosystems, i.e, methane-dependent, chemosynthetic faunas at the seabed. This type of ecosystem provides a good analogue for past methane-rich environments and offers an opportunity to investigate possible effects of methane seepage on benthic foraminiferal communities. Although knowledge of the ecology and distribution patterns of cold-seep-associated benthic foraminiferal species remain one key to improve the interpretation of past records, the ecology and distribution patterns of living foraminiferal species in relation to different types of seep environments are still poorly studied.
It is commonly accepted that the distribution of benthic foraminifera is mainly determined by environmental factors, such as temperature, oxygen, food availability, and CO2 (e.g. Corliss et al., 1986; Jorissen et al., 1995; Thomas et al., 1995; Rasmussen and Thomsen, 2017). Due to the ephemeral nature of cold seeps, the above-mentioned environmental factors change over time, and so does the microhabitats in which the foraminifera live. Natural processes of changes in the faunal structure of an ecological community over time can result from the combined effects of changing environmental factors and biological interactions including among others commensalism and competition, and is referred to as environmental successions; Dayton and Hessler, 1972; Tanner et al., 1994; Chapman and Underwood, 1994). At cold seeps, the environmental successions are strongly connected to varying strengths of seepage of hydrocarbon fluids and methane-related biological processes such as aerobic and anaerobic oxidation of methane (MOx and AOM, respectively), which shape the biochemistry of the sediment. Seep community successions as modeled by Bergquist et al. (2003) and Bowden et al. (2013) suggests a general succession pattern beginning with a localized flux of methane-rich fluids which promotes growth of MOx bacterial communities at the sediment surface (Stage 1 of succession). Prolonged methane seepage causes expansion of sulfur-bacterial mats and increase in AOM activity (Stage 2). Moderate supply of sulfide between the patches of sulfur-bacterial mats can become inhabited by Vesicomyid clam populations (Stage 3). If seepage continue, colonization by Siboglinidae tubeworms starts. Over time, formation of authigenic carbonate rocks and pavements modifies methane flow and limit the extent of sulfide-rich sediments. As a result, Vesicomyid populations reduces in favor of the chemosynthetic Bathymodiolus mussels, which opposite to clams require hard substrates (Stage 4). As seepage declines, cold-water corals can colonize the authigenic carbonate rocks and eventually form large, long-lasting coral reefs in post-seepage conditions (Stage 5; Bergquist et al., 2003).
In this paper, we present the results of a study of the distribution patterns of living (Rose Bengal-stained) benthic foraminifera in the surface and near-surface sediments from a cold-seep ecosystem at Vestnesa Ridge together with the downcore distribution of pore water biogeochemical data.
Materials and methods
Study area
Vestnesa Ridge is a deep-sea ridge (>1000 m), located in the Fram Strait, west of Svalbard in the Arctic Ocean (79°N, 5–7°E; Figure 1). Vestnesa developed under the effect of contour currents (Eiken and Hinz, 1993; Hustoft et al., 2009) and it is estimated that periodic gas seepage has occurred since 2.7 Ma from the start of the Quaternary coinciding with glacial intensification at the western Svalbard margin (Plaza-Faverola et al., 2015). On the crest of Vestnesa Ridge a series of pockmarks occur (i.e, shallow seabed depressions where methane-rich fluids seep from the seabed), among which the two most active are informally referred to as ‘Lomvi’ and ‘Lunde’ (Bünz et al., 2012; Plaza-Faverola et al., 2015). In these pockmarks, TowCam video observations have revealed the presence of cold-seep related structures such as bacterial mats, macro- and megafaunas and methane-derived authigenic carbonate outcrops (Åström et al., 2016; Sztybor and Rasmussen, 2017; Åström et al., 2018; Melaniuk, 2021).
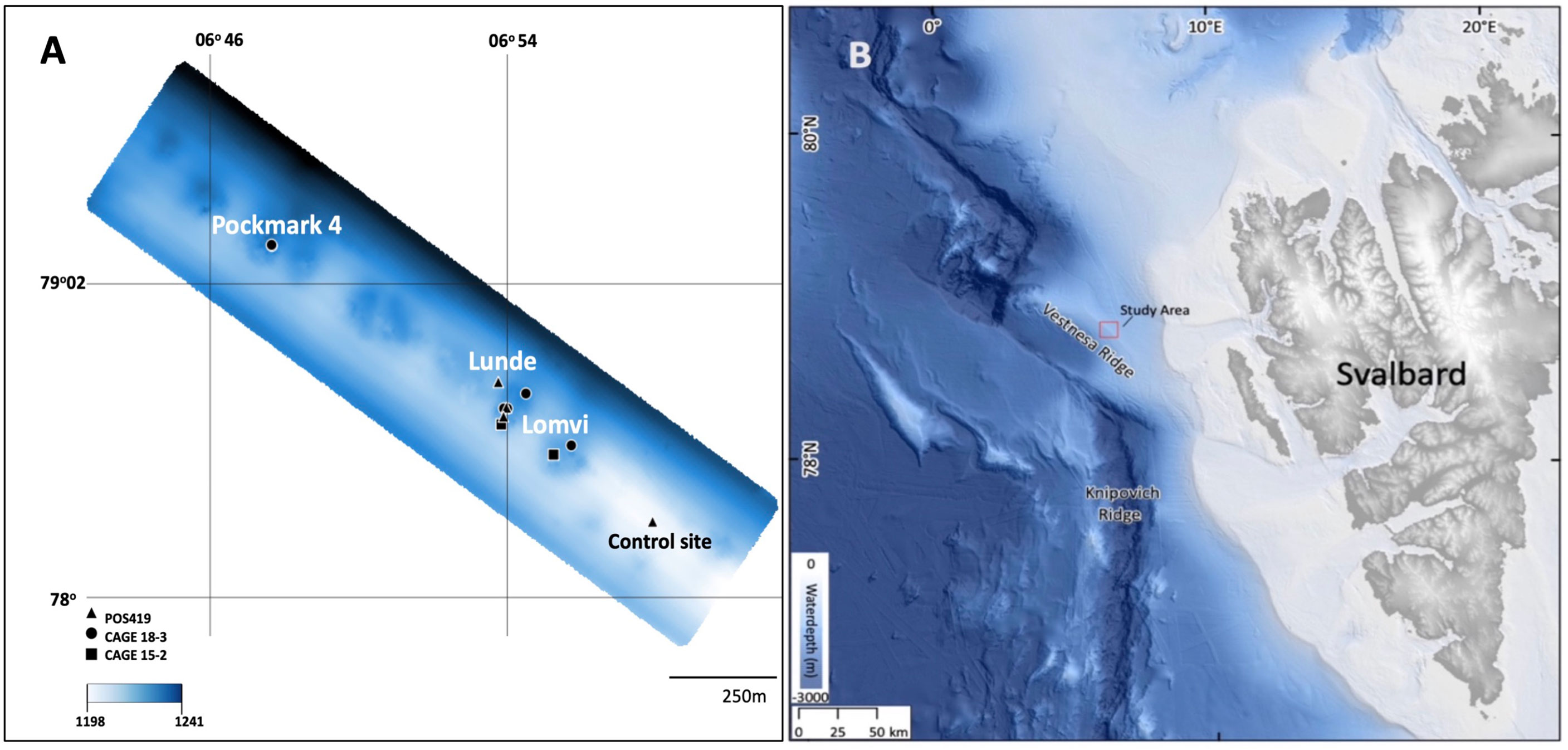
Figure 1 (A) Detail of Vestnesa Ridge (modified from Bünz et al., 2012). Triangles indicate POS419 multicorer locations, dots indicate CAGE 18-3 box core locations, and squares CAGE 15-2 multicorer locations (B) Svalbard margin in the Eastern Fram Strait (bathymetry from (Jakobsson et al., 2020).
Sediment sampling
Sediment samples for this study were collected during the POS419 expedition of RV Poseidon in August 2011, the CAGE 15-2 cruise in May 2015 (Melaniuk, 2021), and during the CAGE 18-3 cruise both with RV Helmer Hanssen to Vestnesa Ridge (Figure 1; Table 1). The two most active pockmarks were targeted. The sampling on POS419 and CAGE 15-2 cruises were done using a TV-guided multicorer to visually localize active methane seep sites within the pockmarks to select sampling spots. Porewater data for multicores MUC 10, MUC 11, and MUC 12 has been previously published (Melaniuk et al., 2022), and foraminiferal fauna data for multicores MC 893A and MC 886 in Melaniuk (2021). For this study we have added multicore MUC 8 (POS419) for additional analyses of porewater and foraminiferal faunas (Figure 1). All foraminiferal samples from POS419 (MUC 8, 10, 11, and -12, and the samples from the CAGE 18-3 cruise are new for this study.
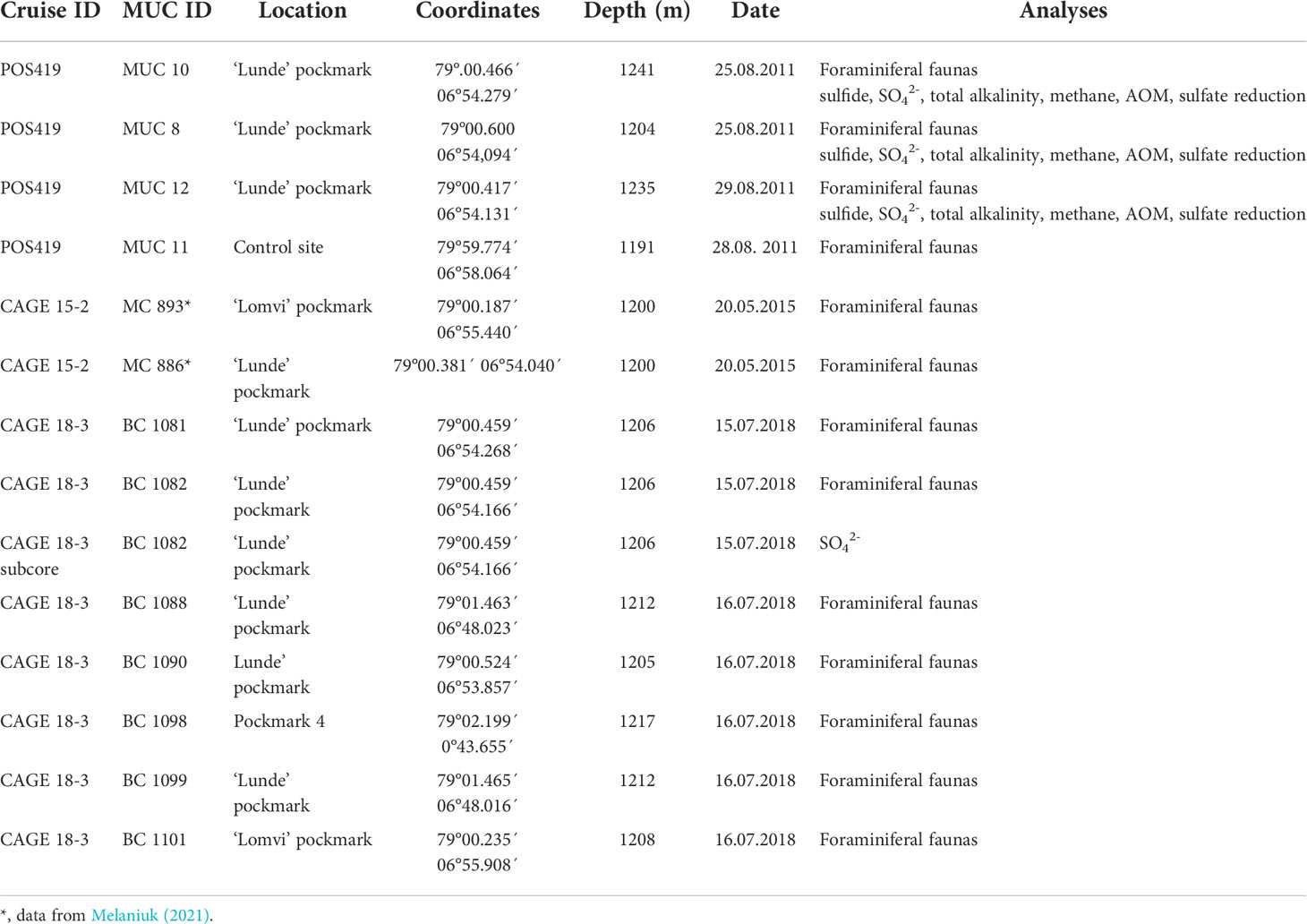
Table 1 Sampling locations, coordinates, water depth, date of sampling, environmental characteristics and analyses performed at sampling sites.
After recovery of the multicorer, selected cores were processed on board. Cores designated for benthic foraminiferal analyses were sampled in 1-cm thick slices. For this study, the top 1-cm thick slice of sediment was used for foraminiferal analyses. The sediment from POS419 was transferred into plastic containers and stained with a Rose Bengal-ethanol solution following the FOBIMO protocol (2 g L-1; Schönfeld et al., 2012). For details of sampling during the CAGE 15-2 cruise see Melaniuk (2021). During the CAGE 18-3 cruise, sediment samples were collected using a box corer (50 x 50 x 50 cm). The top 1 cm of the sediment was collected using a spatula and placed in plastic container with Rose Bengal-ethanol solution (2 g L-1; Schönfeld et al., 2007). For one station, a subcore was taken for geochemical analyses.
Rose Bengal was used in this study to make our results comparable with most other studies. All samples were kept onboard in a dark, cool (+4°C) room until further processing (for details see Melaniuk, 2021; Melaniuk et al., 2022).
Three cores collected from each station of the POS419 cruise were designated for the analyses of 1) sediment pore water chemistry, 2) sediment methane concentration, and 3) rates of microbial methane oxidation and sulfate reduction. All sediment sampling procedures were conducted at +4°C inside a cooled laboratory (for details see Melaniuk et al., 2022).
Foraminiferal analyses
Sediment samples from POS419 were sieved through a 100-µm sieve. The >100-µm residue of each sample was kept wet in distilled water and further examined under reflected-light microscopy. Rose Bengal-stained foraminifera representing both live and recently dead (but still containing cytoplasm) individuals were wet picked, counted and identified. Individuals that stained dark magenta and were fully filled with cytoplasm were considered ‘living’ foraminifera (i.e, live + recently dead individuals). Empty, unstained tests were omitted and not counted in this study. The numbers of living (Rose Bengal stained) foraminifera were compared using Student’s t-test (α = 0.05). The Shannon index S(H) of diversity of species, Evenness index which refers to how close in numbers each species in a sample is, and a Chao1 index for estimating the number of species in a sample (Table S1) were calculated for each sample. Rose Bengal-stained sediment samples collected during CAGE 15-2 and CAGE 18-3 were sieved through a 63-µm sieve (see details in Melaniuk, 2021).
Porewater analyses
Porewater data MUC 10 and 12 have been previously published (Melaniuk et al., 2022; see Supplementary Information). For this study, we have added the pore water data for MUC 8 following the same methods as in Melaniuk et al. (2022).
Porewater from MUC 8 was extracted at +4°C using a low‐pressure squeezer (argon at 1–5 bar) onboard the vessel and right after sampling. While squeezing, porewater was filtered through 0.2 μm cellulose acetate nuclepore filters and collected in argon‐flushed recipient vessels. The collected porewater samples were analyzed onboard for their content of dissolved total sulfides (in the following referred to as “sulfide”) (Cline, 1969). 50 μl of zinc acetate solution was added to a 1-ml sample of pore water. Subsequently, 10 μl of N,N‐dimethyl‐1,4‐phenylenediamine‐dihydrochloride color reagent solution and 10 μl of the FeCl3 catalyst were added and mixed. After 1 hour of reaction time, the absorbance was measured at 670 nm. Total alkalinity (TA) was determined by direct titration of 1 mL pore water with 0.02 M HCl using a mixture of methyl red and methylene blue as an indicator. Bubbling the titration vessel with argon gas was performed to strip CO2 and hydrogen sulfide. The analyses were calibrated using the IAPSO seawater standard, with a precision and detection limit of 0.05 mmol L−1. Porewater samples for sulfate (SO42−) analyses were stored in 2‐ml glass vials at +4°C and analyzed onshore. Sulfate was determined by ion chromatography (Metrohm, IC Compact 761). Analytical precision based on repeated analysis of IAPSO standards (dilution series) was <1%.
Methane measurements
Methane concentrations in MUC 8 were determined in 1 cm intervals down to a depth of 6 cm followed by 2 cm intervals down to 12 cm, 3 cm intervals down to 18 cm and 5 cm intervals deeper than 18 cm (Sommer et al., 2009). From each level, a 2 mL sub-sample was transferred into a septum-stoppered glass vial (21.8 ml) containing 6 ml of saturated solution of NaCl and 1.5 g of NaCl in excess. The volume of headspace was 13.76 ml. Within 24 h, the methane concentration in the headspace was determined using a Shimadzu GC 14A gas chromatograph fitted with a flame ionization detector and a 4 m × 1/8-inch Poraplot Q (mesh 50/80) packed column. Prior to the measurements the samples were equilibrated for 2 h on a shaking table. The precision to reproduce a methane standard of 9.98 ppm was 2%.
Microbial methane oxidation rates
On board, radioactive methane (14CH4 dissolved in water, injection volume 15 µl, activity ~5 kBq, specific activity 2.28 GBq mmol-1) was injected into three replicate mini cores at 1-cm intervals according to the whole-core injection method (Jørgensen, 1978). The mini cores were incubated at in-situ temperature for ~24 h in the dark. To stop bacterial activity, the sediment cores were sectioned into 1-cm intervals and transferred into 50-ml crimp glass vials filled with 25 ml of sodium hydroxide (2.5% w/w). After crimp-sealing, glass vials were shaken thoroughly to equilibrate the pore-water methane between the aqueous and gaseous phase. Control samples were first terminated before addition of tracer. In the home laboratory, methane oxidation rates and methane concentrations in the sample vials were determined according to Treude et al. (2020).
Microbial sulfate reduction rates
Sampling, injection, and incubation procedures were the same as for the methane oxidation samples. The injected radiotracer here was carrier-free 35SO42- (dissolved in water, injection volume 6 µl, activity 200 kBq, specific activity 37 TBq mmol-1). To stop bacterial activity after incubation, sediment cores were sectioned into 1-cm intervals and transferred into 50 ml plastic centrifuge vials filled with 20 ml zinc acetate (20% w/w) and frozen. Control sediment was first terminated before addition of tracer. In the home laboratory, sulfate reduction rates were determined according to the cold-chromium distillation method (Kallmeyer et al., 2004).
Results
Habitat characteristics
Based on visual and geochemical characteristics, the investigated samples have been assigned to individual stages of the environmental succession of the seep sites (Table 2).
Sediments of the Siboglinidae field from MUC 8 showed only modest signs of methane seepage activity. Sulfate showed only a small but steady decline from seawater concentration (28.8 mM) to 27.5 mM at 0 to 30 cm depth (Figure 2M). The methane concentration profile showed two different slopes: a modest increase in concentration from the surface reaching 15 µM at 16.5 cm, and a steeper increase below 16.5 cm reaching 88 µM at depth 30 cm (Figure 2M). The change in slope could possibly marks the lower end of sediment irrigation by Siboglinidae tubes. Sulfide was generally low (<10 µM) with sporadic peaks at the surface, at 9 cm, and at 16.5 cm (Figure 2R). No AOM data are available for MUC 8 and only one sulfate reduction replicate was obtained (Figure 2E, I). Sulfate reduction was low (< 2 nmol cm-3 d->3) throughout the core with no activity at the sediment-water interface and a distinct peak (max 1.6 nmol cm-3 d-3) at 6.5 cm and a second, smaller peak (0.56 nmol cm-3 d-3) at 14.5 cm. The fact that methane concentration steeply increased below 16.5 cm suggests that the second peak in sulfate reduction was related to methane-dependent AOM, while the shallower peak might be correlated to organoclastic sulfate reduction. The sulfate concentration in sediment in BC 1082 is close to the seawater concentration (28,8 mM; Figure 2P). No AOM data, methane, sulfate reduction rates, total alkalinity and sulfide are available for BC 1082 (Figure 2D, H, L, U).
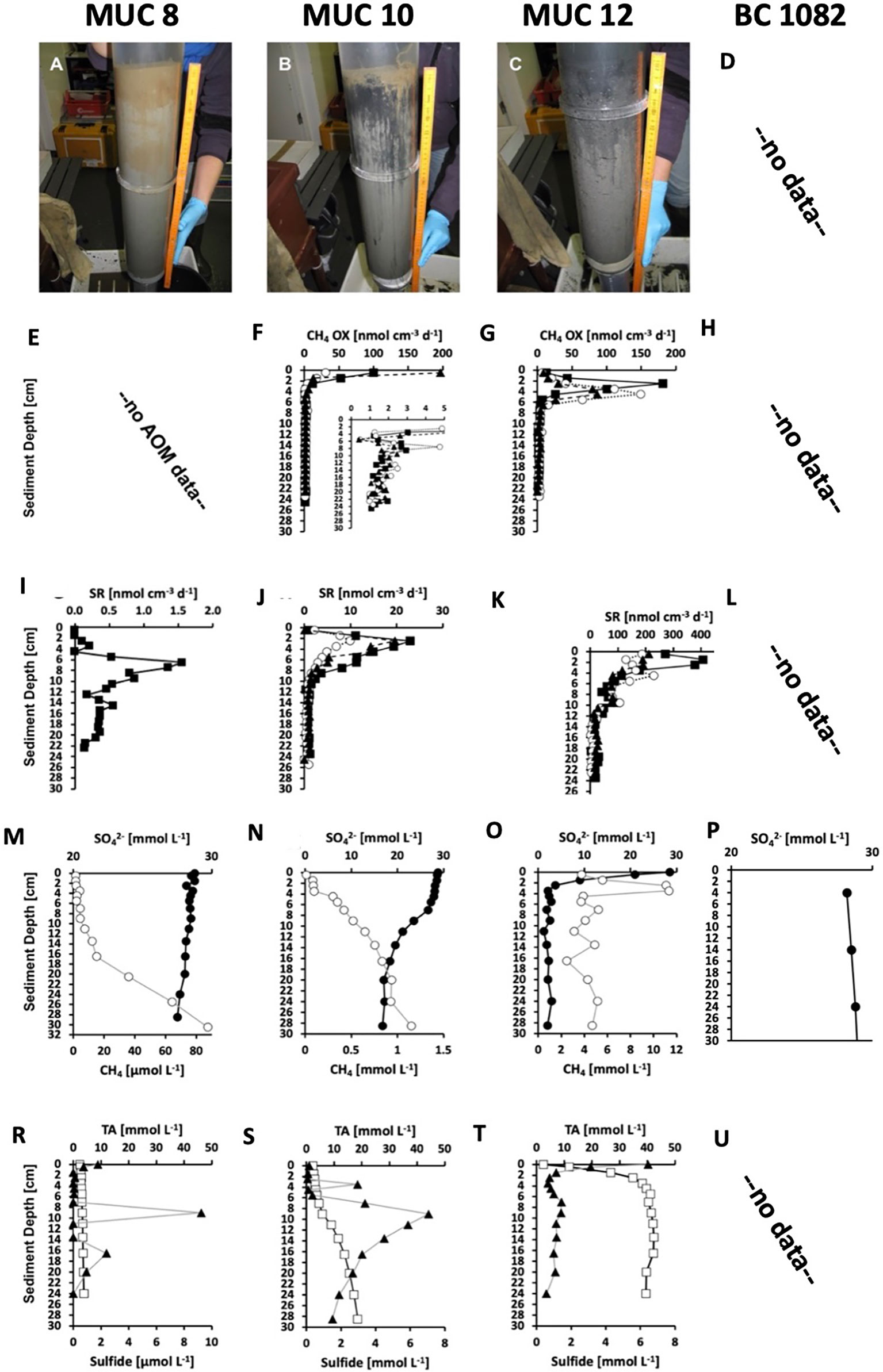
Figure 2 (A, B, C) Selected sediment core photos and biogeochemical parameters of MUC 8 (Stage 4, left panels, note the visible tubes at the surface), MUC 10 (Stage 4, middle panels, note the visible tubes at the surface), and MUC 12 (Stage 2, right panels, note the foamy sediment consistency caused by degassing). Note the different units for methane and sulfide in MUC 8. Data from MUC 10 and 12 were previously published in Melaniuk et al. (2022). P) Concentration of sulfate in sediment from BC 1082. Data courtesy T. Laier, Geological Survey of Denmark and Greenland (GEUS), Copenhagen, Denmark. (E, F, G) Methane oxidation rates (CH4 OX, symbols represent three replicates). (I, J, K, L) Sulfate reduction rates (SR, symbols represent three replicates). (M, N, O, P) Concentrations of pore-water sulfate (SO42−, solid circles) and sediment methane (CH4, open circles). Note the different x-axes for methane. (R, S, T, U) Concentrations of pore-water total alkalinity (TA, open squares), and sulfide (solid triangles).
Foraminiferal faunas
The results show variations in the density of foraminifera according to the succession of seep environments. The early Stage 1 of low methane release showed density of foraminifera from 7 to 25 individuals per 10 cm3 (Figure 3). In Stage 2 of strong seepage, the density decreased to 3 individuals per 10 cm3 (Table S1). The number of foraminifera increased in the Stage 3 of moderate seepage from 24 to 32 individuals per 10 cm3. At Stage 4, also of moderate seepage, the number of foraminifera ranged from 25 to 99 individuals per 10 cm3 of sediment. (Figure 3; Table S1). Student’s t-test (p = 0.09; α = 0.05) showed no statistical difference between the number of foraminifera at Stage 4 of the environmental succession and the control site of no seepage, and no significant differences between Stage 3 and Stage 4 (p = 0.12; α = 0.05) (both stages of moderate seepage). Further, student’s t-test showed statistical differences between number of foraminifera in Stages 1, 2, 3, and the control site of no seepage (Figure 3).
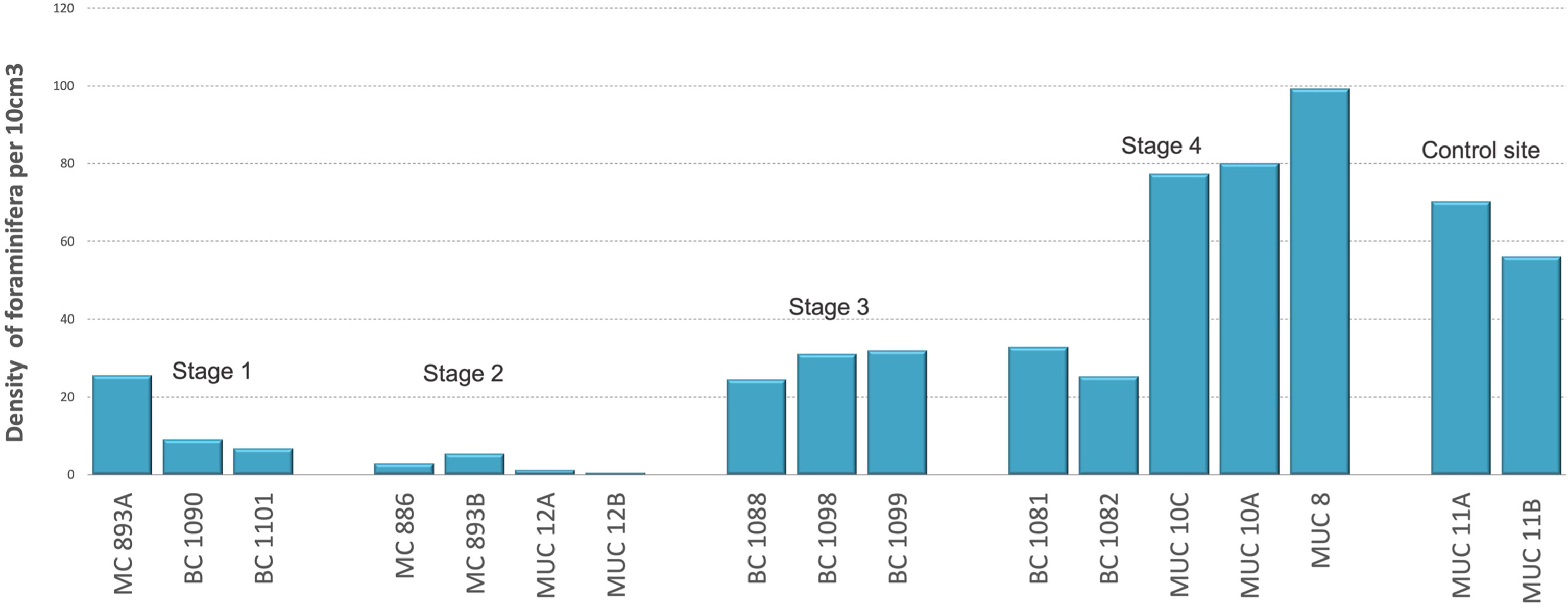
Figure 3 Number of Rose Bengal-stained foraminifera (calcareous and agglutinated) for each Stage (S) of environmental succession, per 10 cm3 of the sediment. Student’s t-tests: S1: S2 p = 0.01; S1: S3 p = 0.00; S1: 4 p = 0.03; S1: control site p = 0.00; S2: S3 p = 0.00; S2: S4 p = 0.00; S2: controls p = 0.00; S3: S4 p = 0.12; S3: control p = 0.01; S4: control site p = 0.09.
Rose bengal-stained agglutinated foraminifera contributed between 10 to 39% of the total foraminiferal fauna at Stage 1, between 0 to 69% at Stage 2, between 26 to 29% at Stage 3, and between 22 to 65% at Stage 4, and 54–55% of total foraminiferal assemblages at the control site (Figure 4). Student’s t-test showed no statistical differences between number of agglutinated foraminifera at Stage 1, Stage 2, Stage 3, and control site (p = 0.00, p = 0.00; p = 0.01; α = 0.05, respectively; Figure 4). There are no statistical differences between number of agglutinated foraminifera at Stage 1, Stage 3 (p = 0.05; α = 0.05) and between Stage 1 and Stage 4 (p = 0.05, α = 0.05; Figure 4). There were further significant differences in the number of agglutinated foraminifera at Stage 3 and Stage 4 (p = 0.16; α = 0.05), Stage 4 and the control site of no seepage (p = 0.94; α = 0.05).
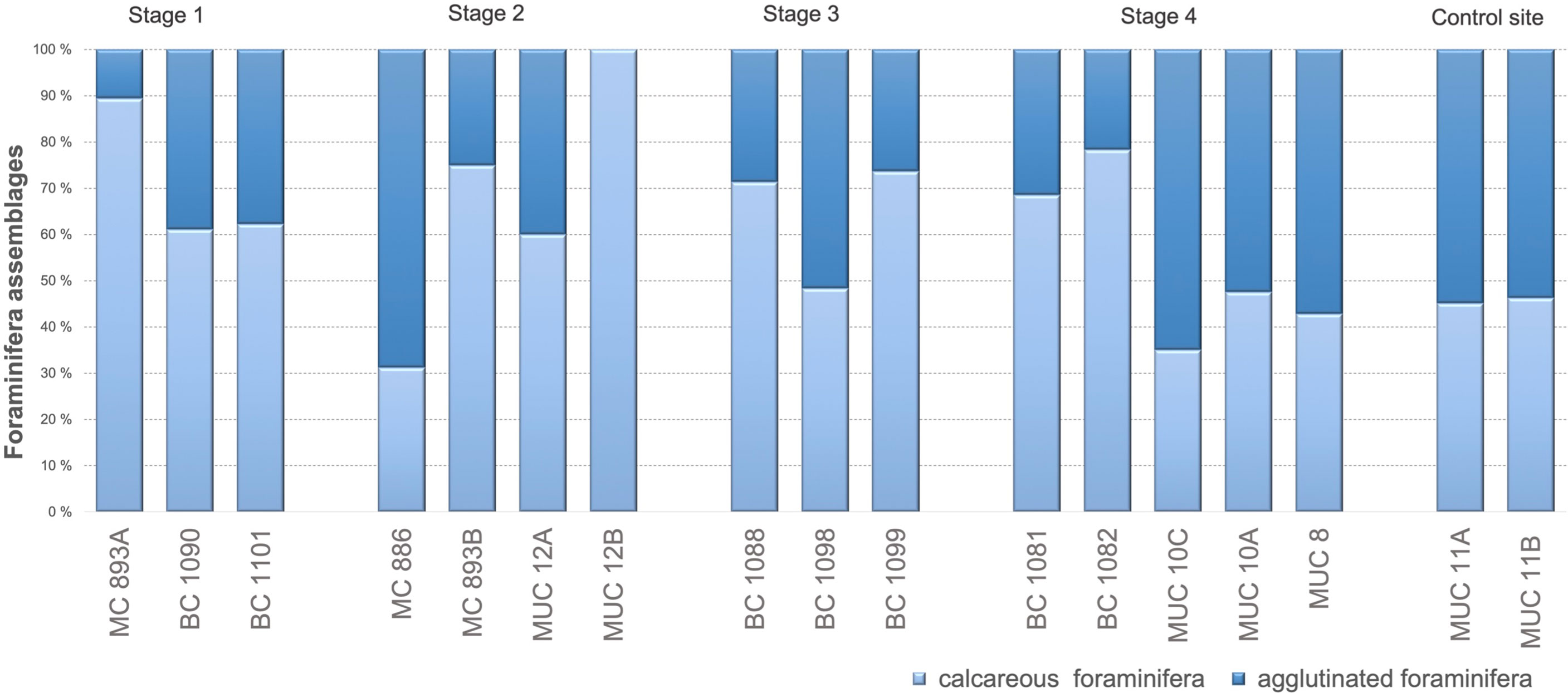
Figure 4 Percentage ratio between calcareous and agglutinated foraminifera for each Stage (S) of environmental succession; direct counting. S1: S2 p = 0.04; S1: S3 p = 0. 05; S1: S4 p = 0.05; S1: control site p = 0.00; S2: S3 p = 0.02; S2: S4 p = 0.06; S2: control site p = 0.00; S3: S4 p = 0.16; S3: control p = 0.01; S4: control site p = 0.94.
The Shannon index S(H) ranged from 1,65 to 2,42 at Stage 1, from 0,81 to 1,76 at Stage 2, from 2,11 to 2,52 at Stage 3, from 1,96 to 2,17 at Stage 4, and from 1,98 to 2,03 at the control site. The Evenness index ranged from 0,52 to 0,87 at Stage 1, from 0,78 to 1,13 at Stage 2, from 0,51 to 0,65 at Stage 3, from 0,51 to 0,63 at Stage 4, and from 0,56 to 0,63 at the control site.
In samples corresponding to Stage 1 of the environmental succession the most common species was Melonis barleeanus and Cassidulina neoteretis. Melonis barleeanus constituted to 12–53% of the total number of calcareous foraminifera, and C. neoteretis ranged from 18 to 38 (Figure 5). Stage 2 was dominated by M. barleeanus, which constituted between 50–76% of the calcareous species. At Stage 3 the most abundant species was C. neoteretis (13–35%), and M. barleeanus (12–51%) (Figure 5). In the surface sediment in core BC 1088 Bolivina pseudopunctata constituted up to 31% of the calcareous species. At Stage 4, C. neoteretis represented between 32 and 52% of the benthic foraminiferal fauna and M. barleeanus 5 to 35% (Figure 5). In samples from MUC 10A Cibicides wuellerstorfi reached 19% (Figure 5).
Discussion
Distribution patterns of living foraminiferal faunas
Samples collected at Vestnesa Ridge represent four potential stages of environmental successions of the seep ecosystems according to the model suggested by Bergquist et al. (2003) (Figure 6). The process depends on the intensity and duration of methane seepage and is manifested by changes in the distribution patterns of the benthic foraminiferal faunas. For example, appearance of filamentous sulfur-bacterial mats (e.g, Beggiatoa sp.) characterizes the Stages 1 and 2 of the succession, and symbiont-bearing animals (e.g, Siboglinidae, bivalves) characterize Stage 4, when seepage is slowing down, and sulfide conditions are stable (Bergquist et al., 2003; Levin, 2005). We observed that the distribution patterns and ecological successions of the benthic foraminiferal faunas are closely related to the properties and environmental succession of the different microhabitats and thus the species composition of the foraminiferal faunas changes according to the progressing environmental succession. The sampling location of MC 893, BC 1090, and BC 1101 represent Stage 1 of the environmental succession. The TowCam imaging survey during the sampling campaign of the CAGE 15-2 cruise showed that the sediment surface close to MC 893 was covered by patchily distributed white and grey filamentous sulfur-bacteria in areas where methane was leaking from the sediment (Yao et al., 2019; Dessandier et al., 2019; Melaniuk, 2021). The methanotrophic bacterial community at site MC 893 was not well developed yet, implying that the seepage by advective methane transport via mini-fractures at this location started relatively recently (Yao et al., 2019). These authors suggested that the seepage at site MC 983 most likely started a year prior to the sampling in 2015 (Yao et al., 2019). At Stage 1, Rose Bengal-stained foraminifera are present (Melaniuk, 2021), with the Shannon index between 1,65 to 2,42. Parallel to other foraminiferal faunas from cold-seeps, the fauna at Stage 1 is dominated by species adapted to low oxygen and high organic content; here M. barleeanus and C. neoteretis (Rathburn et al., 2000; Bernhard et al., 2001; Hill et al., 2003; Herguera et al., 2014; Etiope et al., 2014) (Figure 5). These two species are common on the mid-slope in the Nordic Seas (Sejrup et al., 1989; Mackensen et al., 1985; Wollenburg and Mackensen, 1998), and are usually present within non-seep marine environments, and previously reported at both seep- and non-seep sites at Vestnesa Ridge (Sztybor and Rasmussen, 2017; Dessandier et al., 2019; Melaniuk, 2021). Melonis barleeanus is an intermediate to deep infaunal species (i.e, a species which live within the sediment) associated with high-nutrient conditions and resistant to environmental stress due to organic matter degradation (Wollenburg and Mackensen, 1998; Alve et al., 2016). Methanotrophic-like bacteria have previously been found in close proximity to the apertural region of living M. barleeanus suggesting that methane-related bacteria can be a part of the diet of M. barleeanus (Bernhard and Panieri, 2018). Cassidulina neoteretis is a shallow infaunal species living in the sediment surface layer and presently one of the most common species at Arctic cold seeps such as at Vestnesa Ridge (Dessandier et al., 2019; Melaniuk, 2021), Storfjordrenna pingo sites (Melaniuk, 2021), and Ha°Ckon Mosby Mud Volcano (Wollenburg and Mackensen, 2009).
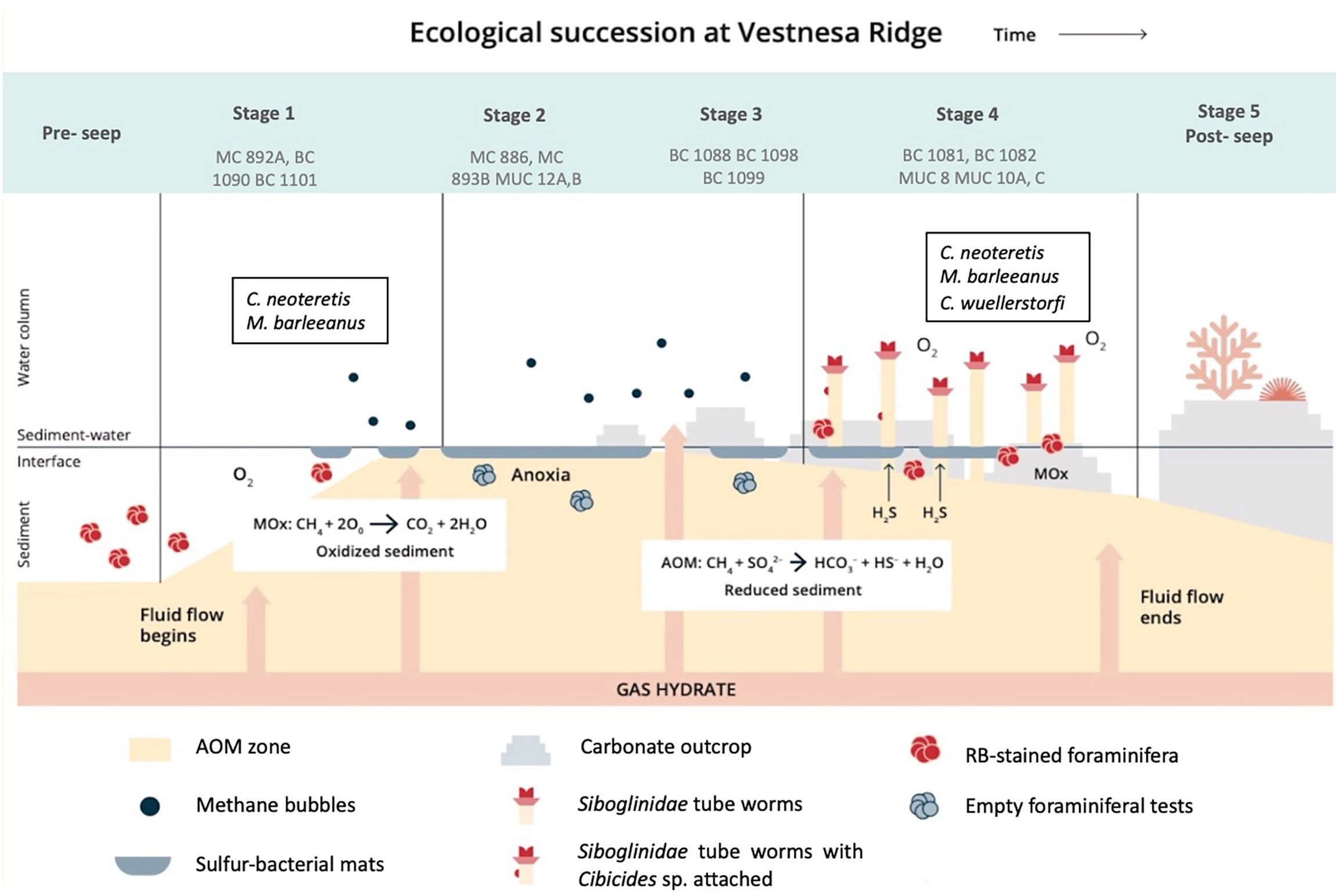
Figure 6 The proposed course of environmental and ecological successions at Vestnesa Ridge. Stage 5 has not (yet) been observed at Vestnesa.
Prolonged methane seepage causes oversaturation of methane in the sediment and lead to the development of a microbial AOM community. The environmental succession moves to Stage 2, represented by MUC 12A, B, MC 893B, and MC 886 in this study. The sediments become dark grey to black in color, release a strong smell of sulfide, are anoxic and reduced below the sediment surface (Figure 2C, T). The sediment of MUC 12 was densely covered by sulfur bacterial mats and dominated by AOM and sulfate reduction developing high concentrations of H2S just below the sediment surface (Figure 2G, K, O; Melaniuk et al., 2022). The geochemistry of site MC 886 was described earlier by Yao et al. (2019). The authors estimated that methane seepage at site MC 886 started earlier compared to the seepage at site MC 893 and that the methane transport was characterized by diffusion (Yao et al., 2019). We suggest that the distribution patterns of the benthic foraminifera at Stage 2 in the environmental succession in MC 886 mainly are controlled by the presence of sulfide released from the AOM consortium of archaea and sulfate reducers. Sulfide is considered highly toxic for aerobic marine organisms as it inhibits production of ATP (aadenosine triphosphate) by irreversibly binding to cytochrome c oxidase (Somero et al., 1989). Compared to Stage 1, food availability seems to play only a secondary role for the foraminiferal community. As a result of adverse environmental conditions, samples from MUC 12 were almost completely barren of benthic foraminifera. Except for a few Rose Bengal-stained specimens, there were no living benthic species found either in MUC 12 or MC 886 (Melaniuk, 2021) (Table S1). Compared to other Stages, Stage 2 is characterized by relatively high Evenness index (0,78–1,13) and the lowest Shannon index (0,81–1,76). It indicates a homogeneous species composition at Stage 2. Considering that Rose Bengal stains both live and dead cytoplasm, even a few months after the death of the foraminifera, especially in anoxic conditions (Jorissen et al., 1995; Bernhard et al., 2001), it is possible that the few RB-stained individuals were already dead at the time of sampling. There are evidence showing that some of the deep-sea benthic foraminiferal species posses organells such as kleptoplasts, which supports life in oxygen-depleted habitats (LeKieffre et al., 2017). Nevertheless, so far, no additional organelles and/or alternative, anaerobic metabolic pathways has been observed in any of the benthic foraminiferal species from Vestnesa Ridge (Bernhard and Panieri, 2018).
If methane seepage decreases and sulfidic conditions last long enough, they can support the development of chemosynthetic vesicomyid and solemyid clams (Niemann et al., 2006; Treude et al., 2007; Hansen et al., 2017; Hansen et al., 2020), which indicates Stage 3. There is evidence from fossils confirming the presence of chemosynthetic bivalves at Vestnesa Ridge (Ambrose et al., 2015; Hansen et al., 2017; Thomsen et al., 2019; Hansen et al., 2020). Still, clams, have not been observed in modern assemblages from Vestnesa Ridge or other Arctic seep sites (Rybakova et al., 2013; Hansen et al., 2017; Thomsen et al., 2019; Hansen et al., 2020; and references therein). So far, the only obligate chemosynthetic infaunal taxa found at Vestnesa Ridge are the Siboglinidae worms and Thyasiridae bivalves that are partially dependent on, or related to chemoautotrophy (Åström et al., 2018). As a result of a progressive decrease in methane flux, oxygen can penetrate the top layer of the sediment. In this study sediments from BC 1088, BC 1098, and BC 1099 correspond to Stage 3 of the environmental successions. The upper 1 to 2-cm layer of the oxygenated sediment provides an environment for a population of benthic foraminifera, and the underlying grey sulfide-rich sediment fuels aggregation of chemosynthetic Siboglinidae. As more oxygen is available, the number of foraminifera increased up to 20 individuals per 10 cm3. Stage 3 is characterized by the highest Shannon index (2,11–2,52) among all Stages of environmental succession and low Evenness index 0,51– 0,65) (Table S1). Similar to Stages 1 and 4, at Stage 3 the dominant species is C. neoteretis (13–36%) and M. barleeanus (12–51%). Additionally, in sediment from BC 1088 Bolivina pseudopunctata constitutes up to 31% of the calcareous species. Species of Bolivina sp. are common in areas with dysoxic/anoxic conditions, and are indicators of abundant food supply (Jorissen et al., 2007; Arreguín-Rodríguez and Alegret, 2016). They show ability to perform nitrate respiration (Glock et al., 2019), and thus are considered as indicators of methane-rich environments (Rathburn et al., 2000; Hill et al., 2003; Lobegeier and Sen Gupta, 2008; Wollenburg and Mackensen, 2009).
Stage 4 is represented by sampling sites MUC 8, MUC 10, BC 1081, BC 1082, and showed the typical bright-brown coloring of the top sediment layers, indicating oxygenated sediment from bio-irrigation by the tubeworms, which was further supported by biogeochemical profiles from the sites (Figures 2A, B) (Melaniuk et al., 2022). Deeper in the core, the sediment color changed to black and grey indicating reducing conditions (Figure 2B). The majority of methane oxidation occurred in the top 4 cm and was decoupled from sulfate reduction, suggesting that MOx was the dominant biological process (Figure 2F, J, S), while the lower parts of the core were subjected to AOM (Melaniuk et al., 2022). Thus, we assume that oxygen was present in the top layers of the core. Rose Bengal-stained foraminifera were present in MUC 8, MUC 10 (A and C), BC 1081, and BC 1082. Similar to Stage 1, the foraminiferal fauna at Stage 4 was dominated by M. barleeanus and C. neoteretis. Cassidulina neoteretis constitutes up to 51% of all foraminifera in MUC 10C, 31% of the foraminifera in MUC 8, 45% in BC 1081, and 32% in BC 1082. Similar to Stage 3, at Stage 4 the Shannon index is high from 1,96 to 2,17, and the Evenness index is low (0,51–0,63) compared to other Stages. Both indices suggest that environmental conditions are very hospitable for foraminifera.
At Stage 4 there were many specimens of the epibenthic species Cibicides wuellerstorfi (up to 19% of all calcareous foraminifera in MUC 10A), compared to any other site presented in this study, including the control site with no seepage of methane. Cibicides wuellerstorfi is an epifaunal species, which tend to attach itself to structures extending above the seafloor. It prefers low food supply brought by currents and a high oxygen concentration (Lutze and Thiel, 1989; Hald and Steinsund, 1996; Klitgaard-Kristensen et al., 2002; Wollenburg and Mackensen, 2009). Thus, it is not a species expected to be exclusively associated with methane-affected environments. Apparently, the Siboglinidae tubeworms aggregations elevated high above the sediment provide a surface for attachment of C. wuellerstorfi (Melaniuk et al., 2022).
It was previously suggested that agglutinated foraminifera are less abundant in sediments influenced by methane seepage than calcareous specimens (Dessandier et al., 2019; and references therein). Our study shows the sediment from the Siboglinidae field (Stage 3) is more densely populated by agglutinated specimens compared to the samples from sulfur-bacterial mats (MUC 12) (Table S1). In the upper 0–1 cm interval (MUC 8 and MUC 10A, B), living (RB-stained) agglutinated specimens constitute more than 50% of the foraminiferal fauna (Figure 4). The high number of agglutinated specimens may result from the elevated ambient pCO2 from CO2 produced during MOx. The elevated pCO2 could create acidification of the pore water, which can inhibit calcification, eventually causing dissolution of the calcareous species and give agglutinated species a competitive advantage (Figure 4; Table S1). The most abundant species among the agglutinated foraminifera are specimens which belong to the Reophax genus. They constitute up to 36% of the total benthic foraminiferal fauna at Stage 3 and up to 37% at the control site (Table S1).
Although our interpretation is based on a limited number of samples similar patterns of environmental successions of seep environments has been found at Ha°Ckon Mosby Mud Volcano (HMMV) located south of Svalbard at 72°N (Wollenburg and Mackensen, 2009). Sites from this area display strict horizontal and vertical distribution patterns according to microhabitat distributions i.e, the hummocky peripheral part with abundant Siboglinidae tube worms, a middle sulfidic zone with AOM and sulfur-bacterial mats, and the active center with visible gas bubbles that is sulfate-free and dominated by aerobic methane-oxidizing bacteria (Pimenov et al., 1999; Gebruk et al., 2003; Jerosch et al., 2007).
The middle sulfidic zone of the HMMV corresponds to Stage 2 of the environmental succession, corresponding to cores MUC 12A, B, MC 893B, and MC 886 in this study. In this zone fluid flow is relatively high and sulfate is still present in the sediment and available for AOM (Niemann et al., 2006). Similar to our MUC 12, the middle zone is covered by sulfur-bacterial mats and is practically barren of foraminifera. Only a few individuals of C. neoteretis have been found in dysoxic sediments in the upper 1-cm surface layer of this zone (Wollenburg and Mackensen, 2009). Sediments at the peripheral zone of the HMMV with low fluid flow and no AOM activity in the surface sediment (Niemann et al., 2006) corresponds to Stage 4 in our study. Sediment were oxidized down to 10 cm depth and inhabited by Siboglinidae tubeworms (Wollenburg and Mackensen, 2009). Similar to Stage 4 (MUC 10 and 8) presented in this paper, Rose Bengal-stained Fontbotia wuellerstorfi (i.e, C. wuellerstorfi) were abundant and quite a large proportion of the individuals were attached to the Siboglinidae tubes.
Stage 5 has not been observed in our study as we have targeted the most active pockmarks at Vestnesa Ridge. To find succession Stage 4, formerly active, but presently inactive pockmarks found at the deeper part of the ridge at 1300 m water depth (Bünz et al., 2012; Consolaro et al., 2015) should be sampled. In the study of Consolaro et al. (2018), the benthic foraminiferal faunas from an inactive pockmark near the core top (and not stained) from this area consists of almost equal proportions of C. neoteretis, and C. reniforme (each c. 20%) accompanied by lower percentages of M. barleeanus (15%) and C. wuellerstorfi (10%). Agglutinated specimens constitute only 7% of the fauna.
Ecological succession and its significance in palaeoceanographic interpretation
Based on the results of this work, it becomes apparent that none of these faunal characteristics can be used exclusively as indicators of strength of methane emission. A clear link between strength of methane seepage and distribution of modern benthic foraminifera has not been found to allow the use of fossil foraminiferal faunas for the reconstruction of past methane emission. It seems that foraminifera are indeed attracted to methane-oxidizing bacteria as a potential food source, as suggested earlier (e.g, Hill et al., 2003; Bernhard et al., 2010), but only when methane seepage is moderate or low as we show for MC 893A of succession Stage 1. Also, during high methane flux and intense AOM activity, oxygen is no longer available for foraminifera in addition to high levels of toxic H2S within the sediment, and hence the foraminiferal population is radically reduced, or samples are barren of foraminifera.
Furthermore, it was not possible to identify any particular species, or a group of species, that could potentially indicate presence of methane seepage and, there was no endemic species, and the observed species were similar to those from other non-seep locations within the Nordic Seas. The benthic foraminiferal fauna was dominated by species adapted to high organic carbon content and low oxygen conditions, and the key species were M. barleeanus, C. neoteretis, Reophax sp, and in one case B. pseudopunctata.
Nevertheless, some subtle differences between seep and non-seep sites do exist, for example at Stage 4 of environmental successions, presence of a chemosynthetic macrofauna such as Siboglinidae tubes generating secondary hard substrates arguably support Cibicides sp. communities. Increased numbers of Cibicidoides wuellerstorfi were observed both at site MUC 8 and 10 as well in previously studies of the Ha°Ckon Mosby Mud Volcano (Wollenburg and Mackensen, 2009). Additionally, compared to other sites samples collected from Siboglinidae tubeworm fields (Stage 4 of the environmental successions of seeps) were characterized by a high number of agglutinated foraminifera. This characteristic, however, can probably rarely be used as a methane seepage indicator. Many agglutinated foraminiferal species are often not preserved in fossil records due to their fragile structures (Gooday, 1994), while calcareous species may not preserve due to the elevated ambient pCO2 (a high ratio of carbon dissolution is common in deep-sea settings including non-seep sites within the Arctic) (Zamelczyk et al., 2013).
Conclusions
Our study shows that the distribution patterns and the species composition of the foraminiferal faunas change according to the progressing environmental succession model of seep environments. Overall, progressing environmental successions result in patchy distributions of living benthic foraminifera; from barren samples in areas with dense sulfur-bacterial mats in sediments affected by AOM and very strong seepage of methane (Stage 2) to high densities in sediment samples from Siboglinidae tubeworm fields with MOx as a dominant process and moderate seepage (Stage 4). The main characteristic of each stage of methane seep environments are as follows:
● At Stage 1 with scattered patches of sulfur-bacterial mats, the species composition of the benthic foraminiferal assemblages is similar to the non-seep locations in the Arctic and Nordic Seas with main dominant species Cassidulina neoteretis, and Melonis barleeanus being adapted to low oxygen and high organic content. The density of foraminifera is lower compared to other Stages of environmental succession.
● At Stage 2 with presence of dense sulfur-bacterial mats presence of hydrogen sulfide (H2S) produced by anaerobic methane oxidation (AOM) is the main limiting factor controlling foraminiferal populations at Vestnesa Ridge with foraminifera-barren or almost barren samples. This Stage is characterized by the lowest Shannon index and highest Evenness index.
● Stages 3, with the 1–2 cm top sediment layer oxygenated and the underlying sediment anoxic, the foraminiferal fauna is similar to Stage 1 and Stage 4. The Shannon index is high compared to the control side, but with a low Evenness index.
● At Stage 4 with moderate methane seepage, and with presence of numerous chemosynthetic Siboglinidae tubeworms, a rich foraminiferal community is found. Large numbers of C. neoteretis, M. barleeanus, and the agglutinated genus Reophax sp. were found and in high percentages. The presence of Siboglinidae tubes supports the epifaunal benthic foraminiferal species Cibicides wuellerstorfi by generating a secondary hard bottom. Similar to Stage 3, the The Shannon index is higher compared to the control side and the Evenness index is low compared to the other Stages.
Foraminifera from the same 1-cm thick interval in a sediment core may represent short-term changes in the foraminiferal population due to the different stages of environmental successions and/or a mix of several different degrees of strengths of methane seepage events. Overall, none of the faunal characteristics can be used exclusively as an indicator of methane emission, strength of methane release, and stages of methane emissions. This makes it difficult to use the foraminiferal fauna compositions as a single tool for reconstructions of past methane release and its intensity.
Data availability statement
The original contributions presented in the study are included in the article/Supplementary Material. Further inquiries can be directed to the corresponding author.
Author contributions
Author contributions to the manuscript was as follows: KM and TLR designed the study. KS, TT, and SS collected and processed samples. KM analyzed data and prepared the manuscript with input from all co-authors. TLR obtained funding for the study. All authors contributed to the article and approved the submitted version.
Funding
Funding of this project was provided by Tromsø Research Foundation (Tromsø Forskningsstiftelse, TFS) to the Paleo-CIRCUS project 2010–2014 and with further support from the Research Council of Norway through its Centers of Excellence funding scheme, grant number 223259 (KM, KS, TLR). Support was also provided through the Cluster of Excellence “The Future Ocean” funded by the German Research Foundation and through the Alexander von Humboldt Foundation (TT, SS).
Acknowledgments
We would like to thank the captain, crew, chief scientist (O. Pfannkuche) and scientific party of the RV Poseidon PO419 expedition for technical support and the crew and scientific staff from RV Helmer Hanssen in 2015 and 2018 for their help in sampling. M. Veloso, V. Bertics, and K. Kretschmer are thanked for subsampling of sediment. J. Hommer and B. Domeyer are thanked for geochemical analyses. We also acknowledge J. M. Bernhard for hosting and guiding K. Sztybor at WHOI.
Conflict of interest
The authors declare that the research was conducted in the absence of any commercial or financial relationships that could be construed as a potential conflict of interest.
Publisher’s note
All claims expressed in this article are solely those of the authors and do not necessarily represent those of their affiliated organizations, or those of the publisher, the editors and the reviewers. Any product that may be evaluated in this article, or claim that may be made by its manufacturer, is not guaranteed or endorsed by the publisher.
Supplementary material
The Supplementary Material for this article can be found online at: https://www.frontiersin.org/articles/10.3389/fmars.2022.999902/full#supplementary-material
References
Alve E., Sergei K., Schönfeld J., Dijkstra N., Golikova E., Hess S., et al. (2016). Foram-AMBI: A sensitivity index based on benthic foraminiferal faunas from north-East Atlantic and Arctic fjords, continental shelves and slopes. Mar. Micropaleontol 122, 1–12. doi: 10.1016/j.marmicro.2015.11.001
Ambrose W. G., Panieri G., Schneider A., Plaza-Faverola A., Carroll M. L., Åström E. K. L., et al. (2015). Bivalve shell horizons in seafloor pockmarks of the last glacial-interglacial transition: A thousand years of methane emissions in the a rctic O cean. Geochem Geophys Geosyst 16 (12), 4108–4129. doi: 10.1002/2015GC005980
Arreguín-Rodríguez G. J., Alegret L. (2016). Deep-sea benthic foraminiferal turnover across early Eocene hyperthermal events at northeast Atlantic DSDP site 550. Palaeogeogr Palaeoclimatol Palaeoecol. 451, 62–72. doi: 10.1016/j.palaeo.2016.03.010
Åström E. K. L., Carroll M. L., Ambrose W. G., Carroll J. (2016). Arctic Cold seeps in marine methane hydrate environments: Impacts on shelf macrobenthic community structure offshore Svalbard. Mar. Ecol. Prog. Ser. 552, 1–18. doi: 10.3354/meps11773
Åström E. K. L., Carroll M. L., Ambrose W. G., Sen A., Silyakova A., Carroll J. (2018). Methane cold seeps as biological oases in the high-Arctic deep sea. Limnology Oceanogr 63 (S1), S209–S231. doi: 10.1002/lno.10732
Bergquist D. C., Andras J. P., McNelis T., Howlett S., van Horn M. J., Fisher C. R. (2003). Succession in gulf of Mexico cold seep vestimentiferan aggregations: The importance of spatial variability. Mar. Ecol. 24 (1), 31–445. doi: 10.1046/j.1439-0485.2003.03800.x
Bernhard J. M., Buck K. R., Barry J. P. (2001). Monterey Bay cold-seep biota: Assemblages, abundance, and ultrastructure of living foraminifera. Deep Sea Res. Part I: Oceanographic Res. Papers 48 (10), 2233–22495. doi: 10.1016/S0967-0637(01)00017-6
Bernhard J. M., Martin J. B., Rathburn A. E. (2010). Combined carbonate carbon isotopic and cellular ultrastructural studies of individual benthic foraminifera: 2. toward an understanding of apparent disequilibrium in hydrocarbon seeps. Paleoceanography 25, PA4206. doi: 10.1029/2010PA001930
Bernhard J. M., Panieri G. (2018). Keystone Arctic paleoceanographic proxy association with putative methanotrophic bacteria. Sci. Rep. 8 (1), 10610. doi: 10.1038/s41598-018-28871-3
Bowden D. A., Rowden A. A., Thurber A. R., Baco A. R., Levin L. A., Smith C. R., et al. (2013). Cold seep epifaunal communities on the hikurangi margin, new Zealand: Composition, succession, and vulnerability to human activities. PloS One 8 (10), e76869. doi: 10.1371/journal.pone.0076869
Bünz S., Polyanov S., Vadakkepuliyambatta S., Consolaro C., Mienert J. (2012). Active gas venting through hydrate-bearing sediments on the vestnesa ridge, offshore W-Svalbard. Mar. Geol. 332–334. doi: 10.1016/j.margeo.2012.09.012
Chapman M. G., Underwood A. J. (1994). Dispersal of the intertidal snail, Nodilittorina pyramidalis, in response to the topographic complexity of the substratum. J. Exp. Mar. Biol. Ecol. 179 (2), 145–169. doi: 10.1016/0022-0981(94)90111-2
Cline J. D. (1969). Spectrophotometric determination of hydrogen sulfide in waters. Limnology Oceanogr 14 (3), 454–458. doi: 10.4319/lo.1969.14.3.0454
Consolaro C., Rasmussen T. L., Panieri G. (2018). Palaeoceanographic and environmental changes in the eastern fram strait during the last 14,000years based on benthic and planktonic foraminifera. Mar. Micropaleontol 139, 84–101. doi: 10.1016/j.marmicro.2017.11.001
Consolaro C., Rasmussen T. L., Panieri G., Mienert J., Bünz S., Sztybor K. (2015). Carbon isotope (δ13C) excursions suggest times of major methane release during the last 14 kyr in fram strait, the deep-water gateway to the Arctic. Clim. Past 11 (4), 669–685. doi: 10.5194/cp-11-669-2015
Corliss B. H., Martinson G., Keffer T. (1986). Late quaternary deep-ocean circulation. GSA Bull. 97 (9), 1106–11215. doi: 10.1130/0016-7606(1986)97<1106:LQDC>2.0.CO;2
Dayton P. K., Hessler R. R. (1972). Role of biological disturbance in maintaining diversity in the deep sea. Deep Sea Res. Oceanographic Abstracts. doi: 10.1016/0011-7471(72)90031-9
Dessandier P.-A., Borrelli C., Kalenitchenko D., Panieri G. (2019). Benthic foraminifera in Arctic methane hydrate bearing sediments. Front. Mar. Sci. 6, 765. doi: 10.3389/fmars.2019.00765
Dickens G. R., Castillo M. M., Walker J. C. G. (1997). A blast of gas in the latest Paleocene: Simulating first-order effects of massive dissociation of oceanic methane hydrate. Geology 25 (3), 259–2625. doi: 10.1130/0091-7613(1997)025<0259:ABOGIT>2.3.CO;2
Eiken O., Hinz K. (1993). Contourites in the fram strait. Sedimentary Geol 82 (1), 15–32. doi: 10.1016/0037-0738(93)90110-Q
Etiope G. P., Fattorini D., Regoli F., Vannoli P., Italiano F., Locritani M., et al. (2014). A thermogenic hydrocarbon seep in shallow Adriatic Sea (Italy): Gas origin, sediment contamination and benthic foraminifera. Mar. Petroleum Geol 57, 283–293. doi: 10.1016/j.marpetgeo.2014.06.006
Gebruk A. V., Krylova E. M., Lein A. Y., Vinogradov G. M., Anderson E., Pimenov N. V., et al. (2003). Methane seep community of the håkkon mosby mud volcano (the Norwegian sea): composition and trophic aspects. Sarsia 88, 394–403. doi: 10.1080/00364820310003190
Glock N., Roy A. S., Romero D., Wein T., Weissenbach J., Revsbech N. P., et al. (2019). Metabolic preference of nitrate over oxygen as an electron acceptor in foraminifera from the Peruvian oxygen minimum zone. Proc. Natl. Acad. Sci. U.S.A. 116, 2860–2865. doi: 10.1073/pnas.1813887116
Gooday A. J. (1994). The biology of deep-Sea foraminifera: A review of some advances and their applications in paleoceanography. PALAIOS 9 (1), 14–31. doi: 10.2307/3515075
Gooday A. J., Jorissen F. J. (2012). Benthic foraminiferal biogeography: Controls on global distribution patterns in deep-water settings. Annu. Rev. Mar. Sci. 4 (1), 237–2625. doi: 10.1146/annurev-marine-120709-142737
Hald M., Steinsund P. I. (1996). Benthic foraminifera and carbonate dissolution in the surface sediments of the barents and kara seas. Berichte zur Polarforschung 212, 285–307.
Hansen J., Hoff U., Sztybor K., Rasmussen T. L. (2017). Taxonomy and palaeoecology of two late pleistocene species of vesicomyid bivalves from cold methane seeps at Svalbard (79 n). J. Molluscan Stud. 83 (3), 270–2795. doi: 10.1093/mollus/eyx014
Hansen J., Mohamed M. E., Åström E. K. L., Rasmussen T. L. (2020). New late pleistocene species of acharax from Arctic methane seeps off Svalbard. J. Systematic Palaeontol 18 (2), 197–2125. doi: 10.1080/14772019.2019.1594420
Herguera J. C., Paull C., Perez E., Ussler I. I. I. W., Peltzer E. T. (2014). Limits to the sensitivity of living benthic foraminifera to pore water carbon isotope anomalies in methane vent environments. Paleoceanography 29 (3), 273–2895. doi: 10.1002/2013PA002457
Hill T. M., Kennett J. P., Spero H. J. (2003). Foraminifera as indicators of methane-rich environments: A study of modern methane seeps in Santa Barbara channel, California. Mar. Micropaleontol 49 (1), 123–1385. doi: 10.1016/S0377-8398(03)00032-X
Hustoft S., Bünz S., Mienert J., Chand S. (2009). Gas hydrate reservoir and active methane-venting province in sediments on <20 ma young oceanic crust in the fram strait, offshore NW-Svalbard. Earth Planetary Sci. Lett. 284 (1), 12–24. doi: 10.1016/j.epsl.2009.03.038
Jørgensen B. B. (1978). A comparison of methods for the quantification of bacterial sulfate reduction in coastal marine sediments. Geomicrobiol J. 1, 11–27. doi: 10.1080/01490457809377721
Jakobsson M., Mayer L. A., Bringensparr C., Castro C. F., Mohammad R., Johson P., et al. (2020). The international bathymetric chart of the Arctic ocean version 4.0. Sci. Data 7 (1), 176. doi: 10.1038/s41597-020-0520-9
Jerosch K., Schlüter M., Foucher J.-P., Allais A.-G., Klages M., Edy C. (2007). Spatial distribution of mud flows, chemoautotrophic communities, and biogeochemical habitats at Håkon Mosby Mud Volcano. Marine Geology 243 (1–4), 1–17. doi: 10.1016/j.margeo.2007.03.010
Jorissen F. J., de Stigter H. C., Widmark J. G. V. (1995). A conceptual model explaining benthic foraminiferal microhabitats. Mar. Micropaleontol 26 (1), 3–155. doi: 10.1016/0377-8398(95)00047-X
Jorissen F. J., Fontanier C., Thomas E. (2007). “Paleoceanographical proxies based on deep- sea benthic foraminiferal assemblages characteristics,” in Proxies in late Cenozoic paleoceanography: Part 2: Biological tracers and biomarkers. Eds. Hillaire-Marcel C., Vernal A. (Amsterdam, Netherlands: Elsevier), 263–326.
Kallmeyer J., Ferdelman T. G., Weber A., Fossing H., Jørgensen B. B. (2004). A cold chromium distillation procedure for radiolabeled sulfide applied to sulfate reduction measurements. Limnology Oceanogr: Methods 2 (6), 171–1805. doi: 10.4319/lom.2004.2.171
Klitgaard-Kristensen D., Sejrup H. F., Haflidason H. (2002). Distribution of recent calcareous benthic foraminifera in the northern north Sea and relation to the environment. Polar Res. 21, 275–282. doi: 10.1111/j.1751-8369.2002.tb00081.x
LeKieffre C., Spangenberg J. E., Mabilleau G., Escrig S., Meibom A., Geslin E. (2017). Surviving anoxia in marine sediments: The metabolic response of ubiquitous benthic foraminifera (Ammonia tepida). PloS One 12 (5), e0177604. doi: 10.1371/journal.pone.0177604
Levin L. A. (2005). “Ecology of cold seep sediments: Interactions of fauna with flow, chemistry and microbes,” in Oceanography and marine biology: An annual review. Eds. Gibson R. N., Atkinson R. J. A., Gordon J. D. M. (Boca Raton: CRC Press-Taylor & Francis Group), 1–46. doi: 10.1201/9781420037449.ch1
Lobegeier M. K., Sen Gupta B. K. (2008). Foraminifera of hydrocarbon seeps, gulf of Mexico. J. Foraminiferal Res. 38 (2), 93–116. doi: 10.2113/gsjfr.38.2.93
Lutze G. F., Thiel H. (1989). Epibenthic foraminifera from elevated microhabitats; cibicidoides wuellerstorfi and Planulina ariminensis. J. Foraminiferal Res. 19 (2), 153–1585. doi: 10.2113/gsjfr.19.2.153
Mackensen A., Sejrup H. P., Jansen E. (1985). The distribution of living benthic foraminifera on the continental slope and rise off southwest Norway. Mar. Micropaleontol 9 (4), 275–3065. doi: 10.1016/0377-8398(85)90001-5
Martin R. A., Nesbitt E. A., Campbell K. A. (2010). The effects of anaerobic methane oxidation on benthic foraminiferal assemblages and stable isotopes on the hikurangi margin of eastern new Zealand. Mar. Geol 272 (1), 270–2845. doi: 10.1016/j.margeo.2009.03.024
Melaniuk K. (2021). Effectiveness of fluorescent viability assays in studies of Arctic cold seep foraminifera. Front. Mar. Sci. 8 (198). doi: 10.3389/fmars.2021.587748
Melaniuk K., Sztybor K., Treude T., Sommer S., Rasmussen T. L. (2022). Influence of methane seepage on isotopic signatures in living deep-sea benthic foraminifera, 79° n. Sci. Rep. 12, 1169. doi: 10.1038/s41598-022-05175-1
Millo C., Sarnthein M., Erlenkeuser H., Grootes P. M., Andersen N. (2005). Methane-induced early diagenesis of foraminiferal tests in the southwestern Greenland Sea. Mar. Micropaleontol 58 (1), 1–12. doi: 10.1016/j.marmicro.2005.07.003
Murray J. W. (2006). Ecology and applications of benthic foraminifera (Cambridge: Cambridge University Press).
Niemann H., Lösekann T., de Beer D., Elvert M., Nadalig T., Knittel K., et al. (2006). Novel microbial communities of the haakon mosby mud volcano and their role as a methane sink. Nature 443 (7113), 854–8585. doi: 10.1038/nature05227
Pimenov N., Savvichev A., Rusanov I., Lein A., Egorov A., Gebruk A., et al. (1999). Microbial processes of carbon cycle as the base of food chain of håkon mosby mud volcano benthic community. Geo-Mar Lett. 19, 89–96. doi: 10.1007/s003670050097
Plaza-Faverola A., Bünz S., Johnson J. E., Chand S., Knies J., Mienert J., Franek P. (2015). Role of tectonic stress in seepage evolution along the gas hydrate-charged vestnesa ridge, fram strait. Geophys Res. Lett. 42 (3), 733–7425. doi: 10.1002/2014GL062474
Rasmussen T. L., Thomsen E. (2017). Ecology of deep-sea benthic foraminifera in the north Atlantic during the last glaciation: Food or temperature control. Palaeogeogr Palaeoclimatol Palaeoecol. 472, 15–32. doi: 10.1016/j.palaeo.2017.02.012
Rathburn A. E., Levin L. A., Held Z., Lohmann K. C. (2000). Benthic foraminifera associated with cold methane seeps on the northern California margin: Ecology and stable isotopic composition. Mar. Micropaleontol 38 (3), 247–2665. doi: 10.1016/S0377-8398(00)00005-0
Rybakova E., Galkin S., Bergmann M., Soltwedel T., Gebruk A. (2013). Density and distribution of megafauna at the håkon mosby mud volcano (the barents Sea) based on image analysis. Biogeosciences 10, 3359–3374. doi: 10.5194/bg-10-3359-2013
Schneider A., Crémière A., Panieri G., Lepland A., Knies J. (2017). Diagenetic alteration of benthic foraminifera from a methane seep site on vestnesa ridge (NW Svalbard). Deep Sea Res. Part I: Oceanographic Res. Papers 123, 22–34. doi: 10.1016/j.dsr.2017.03.001
Schönfeld J., Alve E., Geslin E., Jorissen F., Korsu S., Spezzaferri S., et al. (2012). The FOBIMO (FOraminiferal BIo-MOnitoring) initiative–towards a standardised protocol for soft-bottom benthic foraminiferal monitoring studies. Mar. Micropaleontol 94–95, 1–13. doi: 10.1016/j.marmicro.2012.06.001
Smith L. M., Sachs J. P., Jennings A. E., Anderson D. M., deVernal A. (2001). Light δ13C events during deglaciation of the East Greenland continental shelf attributed to methane release from gas hydrates. Geophys Res. Lett. 28 (11), 2217–2220. doi: 10.1029/2000GL012627
Somero G. N., Childress J. J., Anderson A. E. (1989). Transport, metabolism, and detoxification of hydrogen sulfide in animals from sulfide-rich marine environments. Rev. Aquat. Sci. RAQSEL 1 (4).
Sommer S., Linke P., Pfannkuche O., Schleicher T., Schneider J., Deimling V., et al. (2009). Seabed methane emissions and the habitat of frenulate tubeworms on the captain arutyunov mud volcano (Gulf of cadiz). Mar. Ecol. Prog. Ser. 382, 69–86. doi: 10.3354/meps07956
Sztybor K., Rasmussen T. L. (2017). Diagenetic disturbances of marine sedimentary records from methane-influenced environments in the fram strait as indications of variation in seep intensity during the last 35 000 years. Boreas 46 (2), 212–2285. doi: 10.1111/bor.12202
Tanner J. E., Hughes T. H., Connell J. H. (1994). Species coexistence, keystone species, and succession: A sensitivity analysis. Ecology 75 (8), 2204–22195. doi: 10.2307/1940877
Thomas E., Booth L., Maslin M., Shackleton N. J. (1995). Northeastern Atlantic benthic foraminifera during the last 45,000 years: Changes in productivity seen from the bottom up. Paleoceanography 10 (3), 545–5625. doi: 10.1029/94PA03056
Thomsen E., Rasmussen T. L., Sztybor K., Hanken N.-M., Tendal O. S., Uchman A. (2019). Cold-seep fossil macrofaunal assemblages from vestnesa ridge, eastern fram strait, during the past 45 000 years. Polar Res. 38 (0). doi: 10.33265/polar.v38.3310
Torres M. E., Mix A. C., Kinports K., Haley B., Klinkhammer G. P., McManus J., et al. (2003). Is methane venting at the seafloor recorded by δ13C of benthic foraminifera shells? Paleoceanography 18 (3). doi: 10.1029/2002PA000824
Treude T., Krause S., Steinle L., Burwicz E., Hamdan L. J., Niemann H., et al. (2020). Biogeochemical consequences of nonvertical methane transport in sediment offshore northwestern Svalbard. J. Geophys Research: Biogeosci 125 (3), e2019JG005371. doi: 10.1029/2019JG005371
Treude T., Orphan V., Knittel K., Gieseke A., House C. H., Boetius A. (2007). Consumption of methane and CO2 by methanotrophic microbial mats from gas seeps of the anoxic black Sea. Appl. Environ. Microbiol. 73 (7), 2271–2283. doi: 10.1128/AEM.02685-06
Wefer G., Heinze P.-M., Berger W. H. (1994). Clues to ancient methane release. Nature 369 (6478), 282–282. doi: 10.1038/369282a0
Wollenburg J. E., Mackensen A. (1998). Living benthic foraminifers from the central Arctic ocean: Faunal composition, standing stock and diversity. Mar. Micropaleontol 34 (3), 153–185. doi: 10.1016/S0377-8398(98)00007-3
Wollenburg J. E., Mackensen A. (2009). The ecology and distribution of benthic foraminifera at the håkon mosby mud volcano (SW barents Sea slope). Deep Sea Res. Part I: Oceanographic Res. Papers 56 (8), 1336–1370. doi: 10.1016/j.dsr.2009.02.004
Yao H., Hong W.-L., Panieri G., Sauer S., Torres M. E., Lehmann M. F., et al. (2019). Fracture-controlled fluid transport supports microbial methane-oxidizing communities at vestnesa ridge. Biogeosciences 16 (10), 2221–2232. doi: 10.5194/bg-16-2221-2019
Keywords: Arctic, gas hydrate (clathrate), methane (CH4), benthic ecology, protista
Citation: Melaniuk K, Sztybor K, Treude T, Sommer S, Zajączkowski M and Rasmussen T L (2022) Response of benthic foraminifera to environmental successions of cold seeps from Vestnesa Ridge, Svalbard: Implications for interpretations of paleo-seepage environments. Front. Mar. Sci. 9:999902. doi: 10.3389/fmars.2022.999902
Received: 21 July 2022; Accepted: 25 August 2022;
Published: 15 September 2022.
Edited by:
Ricardo Serrão Santos, University of the Azores, PortugalReviewed by:
Abdel Galil Hewaidy, Al-Azhar University, EgyptGabriela J. Arreguín-Rodríguez, Universidad Autónoma de Baja California, Mexico
Copyright © 2022 Melaniuk, Sztybor, Treude, Sommer, Zajączkowski and Rasmussen. This is an open-access article distributed under the terms of the Creative Commons Attribution License (CC BY). The use, distribution or reproduction in other forums is permitted, provided the original author(s) and the copyright owner(s) are credited and that the original publication in this journal is cited, in accordance with accepted academic practice. No use, distribution or reproduction is permitted which does not comply with these terms.
*Correspondence: Katarzyna Melaniuk, Katarzyna.Melaniuk@uit.no