Desalination effects on macroalgae (part b): Transplantation experiments at brine-impacted sites with Dictyota spp. from the Pacific Ocean and Mediterranean Sea
- 1Laboratorio de Investigación Ambiental Acuático, Centro de Estudios Avanzados, HUB-AMBIENTAL UPLA, Universidad de Playa Ancha, Valparaíso, Chile
- 2Doctorado Interdisciplinario en Ciencias Ambientales, Facultad de Ciencias Naturales y Exactas, Universidad de Playa Ancha, Valparaíso, Chile
- 3Departamento de Ciencias del Mar y Biología Aplicada, Universidad de Alicante, Alicante, Spain
- 4Departamento de Ciencias Biológicas, Universidad Autónoma de Chile, Talca, Chile
- 5Centro de Bioinnovación, Universidad de Antofagasta, Antofagasta, Chile
- 6Laboratorio de Toxicología Acuática (AQUATOX), Instituto de Ciencias Naturales Alexander von Humboldt, Universidad de Antofagasta, Antofagasta, Chile
Desalination residual brines are mostly discharged to marine environments, which can produce osmotic stress on sensitive benthic organisms. In this investigation, we performed transplantation experiments nearby desalination plants using two brown macroalgae species from a cosmopolitan genus: Dictyota kunthii (Chile) and Dictyota dichotoma (Spain). Parameters related to photosynthetic activity and oxidative stress were evaluated at 3 and 7 days for D. kunthii, and 3 and 6 days for D. dichotoma; each at 2 different impacted sites and 1 control. We observed that brine exposition at both impacted sites in Chile generated a marked stress response on D. kunthii, reflected in a decrease of primary productivity (ETRmax), light requirement (EkETR), and an excessive thermal dissipation (NPQmax), especially at 7 days. In D. dichotoma, similar impaired photosynthetic activity was recorded but only at the highest brine influence site during day 3. Regarding oxidative stress, both species displayed high levels of H2O2 when exposed to brine-influenced sites. Although in D. kunthii H2O2 content together with lipid peroxidation was higher after 3 days, these returned to baseline values towards day 7; instead, D. dichotoma H2O2 levels increased only at day 6. This easy and practical approach has proven to provide valuable data to address potential impacts of brine discharges at global scale coastal ecosystems.
1 Introduction
The increasing demand of freshwater associated with demographic growth and economic development has led to a global water crisis (Campero and Harris, 2019; Herrera-León et al., 2019; Jones et al., 2019). The latter, aggravated with Climate Change, which is bringing mega droughts events and a decrease in mean precipitations at mediterranean and temperate latitudes (Morrison et al., 2009; Schewe et al., 2014). In this sense, the installation of desalination plants, as a non-conventional source of freshwater, has exponentially increased around the world. Indeed, one of the main exponents of the development and installation of desalination plants in the Mediterranean coasts in Europe is Spain, (Lattemann and Höpner, 2008; Fuentes-Bargues, 2014; Fernández-Torquemada et al., 2019; Sola et al., 2020). On the other hand, in South America, desalination is steadily growing, although mostly used for industrial activities. In this regard, Chile is turning at the forefront in seawater desalination in Latin-America, initially mostly for mining development, but now also for drinking water (Herrera-León et al., 2019; Sola et al., 2019).
The available technological options often used for desalination include multi-effect distillation, multi-stage flash distillation and seawater reverse osmosis (SWRO) (Panagopoulos, 2022a; Panagopoulos, 2022b; Panagopoulos and Giannika, 2022a). However, SWRO plants are the preferred desalination method due to its relative low cost, energetic efficiency and reduced environmental impacts (Fernández-Torquemada and Sánchez-Lizaso, 2007; Elsaid et al., 2020). SWRO from direct marine water intake consist in the use of high pressure to force seawater passage through a semi-permeable membrane that retain salts, among other molecules; thus, generating freshwater plus a residual high-salinity brine (Fernández-Torquemada and Sánchez-Lizaso, 2007; Kress and Galil, 2018). The difference in density between brine and seawater can generate the formation of a saline stratified layer in areas subject to mild currents; if logistic and technological measures are not properly taken, this can have significant effects on benthic communities (e.g. Kirst, 1990; Fernández-Torquemada and Sánchez-Lizaso, 2007; Missimer and Maliva, 2018; Sola et al., 2020). In addition, depending on the seawater source, brine discharges may contain low proportion of chemicals residues used throughout the process, such as coagulants, biocides and anti-scalants (e.g. Hoepner and Lattemann, 2003; Belkin et al., 2017; Panagopoulos, 2022a; Panagopoulos, 2022c; Panagopoulos and Giannika, 2022b). However, the impact magnitude has been mostly observed to be related to the volume, flow and dilution rate of the brine-receptive seawater body, together the osmotic sensitivity of the organism inhabiting the ecosystem (Loya-Fernández et al., 2012; Del Pilar Ruso et al., 2015; Missimer and Maliva, 2018). In this context, the extracellular increase in salts can induce cell stress through excess ions influx into the cell; consequently, reducing the absorption of nutrients, promoting dehydration and overproduction of reactive oxygen species (ROS), among others (Kirst, 1990; Bisson and Kirst, 1995). The imbalance between ROS production and scavenging mechanisms can generate oxidative stress, which beyond certain threshold levels can induce oxidative damage and compromise metabolic processes; for instance, in marine macrophytes as seagrasses and macroalgae, affecting and/or impairing photosynthesis (e.g. Sáez et al., 2015; Moenne et al., 2016; Cambridge et al., 2019; Benaissa et al., 2020; Capó et al., 2020; Celis-Plá et al., 2021). Brine-associated effects on marine macrophytes have been mostly related to seagrasses, also demonstrating high species-specific responses (e.g. Sandoval-Gil et al., 2014; Hak-Jyung and Ho yeon, 2018; Samanta et al., 2019). Although records on macroalgae are scarce, recent in situ transplantation studies nearby brine discharges have been conducted using the brown macroalga Ectocarpus (Rodríguez-Rojas et al., 2020). It was observed that Ectocarpus under brine exposition suffered a disruption of photosynthetic activity, H2O2 accumulation, lipid peroxidation and the up-regulation of genes encoding for antioxidant enzymes, as well as salt-related response genes (Rodríguez-Rojas et al., 2020). In the same line, our latest controlled laboratory experiments also in the brown seaweeds Dictyota kunthii and Dictyota dichotoma under increased salinities (+2 and +7 psu) above their natural ranges, evidenced greater photosynthetic activity and H2O2 content in both species. However, H2O2 accumulation was up to 4-fold higher in D. kunthii under excess salinity with respect to D. dichotoma (Muñoz et al. under review).
Despite the ecological importance of macroalgae as primary producers, ecosystem bioengineers, worldwide distribution and abundance, they have been rarely considered to evaluate desalination impacts on coastal ecosystems. In this respect, besides understanding the potential mechanisms by which macroalgae may withstand brine-derived salinity excess, this information can be also widely applied as biotechnology tools to be incorporated into environmental monitoring programs (EMPs) (Rodríguez-Rojas et al., 2020). In the present study, we examined photosynthetic and oxidative stress responses of two ecosystem-forming species of brown macroalgae belonging to the cosmopolitan genus Dictyota exposed to brines through field-transplantation experiments: D. kunthii (East Pacific Ocean, Chile) and D. dichotoma (Mediterranean Sea, Spain). The information was discussed in terms of the known effects of excess salinity under laboratory-experiments (Muñoz et al. under review) and, given the origin of the tested species and worldwide distribution of the genus, the potential further applications for widespread desalination biomonitoring.
2 Material and methods
2.1 Study site, macroalgae collection and experimental design
In situ transplantation experiments were performed nearby brine discharges of 2 desalination plants. The first was carried out in Antofagasta Bay, Chile (23°32’36.7”S; 70°24’14.3”W), during April 2019 with the brown macroalga D. kunthii. This species has Pacific cross-ocean distribution, from the coasts of Chile, and Perú to New Zealand (Hoffmann and Santelices, 1997). Transplants were emplaced near the SWRO desalination plant “Aguas Antofagasta” discharges, in La Chimba sector, which produces up to 73,440 m3 d-1 of freshwater and around of 89,760 m3 d-1 of brine. The second transplantation experiments were carried out in November 2020 nearby brine discharges in the Alicante Bay, Spain (38°20’43”N; 0°28’59”W), using the alga D. dichotoma. This species can be found between the European Atlantic Ocean to the Mediterranean Sea (Tronholm et al., 2008). The “Alicante I and II” SWRO desalination plants produce up to 136,000 m3 d-1 freshwater and approximately 173,090 m3 d-1 of brines.
Transplantation devices were based in photosynthetic active radiation (PAR)-transparent plastic containers, based in the model constructed by Sáez et al. (2015) and Rodríguez-Rojas et al. (2020). Devices contained a rubber sealing lid (12 cm long, 8 cm wide and 5 cm high), and had multiple perforations of 1 mm to allow water exchange between the macroalgae and seawater (Figure 1). Ten sporophytes (approximately 300 mg of different individuals) were placed inside devices and fixed close to the brine discharges of both SWRO desalination plants. Incremental salinities at different distances from the brine source compared to local natural baseline levels were similar for both desalination plant areas in Chile and Spain. To monitor salinity and temperature fluctuations, conductivity and temperature sensors (CTs; model DEFI, JFE Advantech®) were installed at every transplantation site (see Table 1). D. kunthii biomass was collected at Antofagasta Bay from a non-brine impacted area at ~15 m depth, and immediately (within 2 hours maximum) transplanted to the experimental sites. Transplants of D. kunthii were located at 10 and 30 m (23°32’33.19”S; 70°24’11.46”W) from the brine discharge pipe, both at 15 m depth. A control site without brine influence was located in Bolsico Bay in the Santa Maria Island sector (23°33’14”S; 70°24’24”W), also at 15 m depth. In case of transplants of D. dichotoma, the alga was collected from Alicante Bay at 4 m depth, also at a non-impacted zone. The transplants were located at 4 m depth, at different distances from the brine discharge (38°18 04”N; 0°30’ 81”W), considering a spatial salinity range (Table 1). A control site was located in the Aguamarga beach sector (38°18̕ 76”N;0° 30’ 81”W). Subsamples were collected 3 and 7 days for D. kunthii, and after 3 and 6 days for D. dichotoma. In the latter, transplants were taken one day before than those conduced in Chile to avoid devices loss due to strong swells forecasted for day 7 in the Alicante experiments. Transplantation site characteristics (e.g. depth, distances from each other and the discharges) for both Chile and Spain areas were defined taking into account the salinities derived by the features of the brine dispersion in the local ecosystems. Photosynthetic performance analyses were carried out immediately after collecting the subsamples and the rest of the tissue was frozen in liquid nitrogen, and posteriorly stored at – 80 °C for further laboratory analyses.
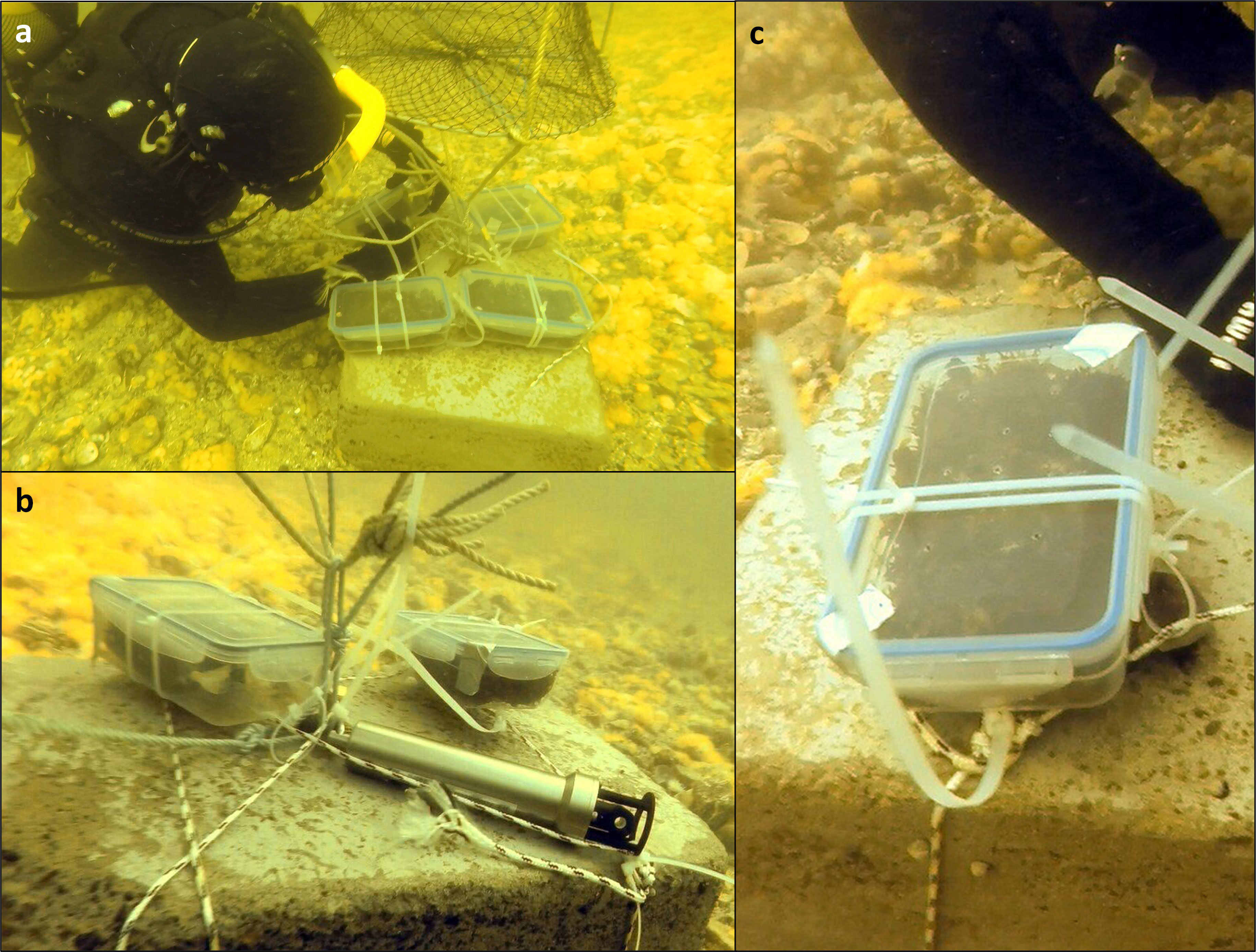
Figure 1 Transplantation devices consisting in plastic containers with one hundred perforations and macroalgae biomass placed inside (A, C). Boxes were anchored with cable ties to artificial concrete reefs and accompanied with a continuous conductivity and temperature (CT) meter (B).
2.2 Photosynthetic performance
Photosynthetic parameters analyzed in this study were the maximum quantum yield of photosystem II (PSII; Fv/Fm), electron transport rate (ETR), the estimator of photosynthetic efficiency (αETR), the saturation irradiance for ETR (EkETR) and the non-photochemical quenching (NPQ); these were obtained by using a pulse-amplitude modulated (PAM) chlorophyll a fluorometer (JUNIOR PAM, Walz GmbH, Effeltrich, Germany) coupled to the Win Control-3.2 software. All calculations were carried out according to Celis-Plá et al. (2014); Celis-Plá et al. (2020) and Rodríguez-Rojas et al. (2020). ETR and NPQ equations were calculated according to Schreiber et al. (1995).
2.3 Concentration of hydrogen peroxide (H2O2)
Hydrogen peroxide levels were estimated spectrophotometrically according to Rodríguez-Rojas et al. (2020), with modification for both species. For each species, ~100 mg fresh weight (FW) biomass were grounded with liquid nitrogen and mixed with 100 µL 10% trichloroacetic acid (TCA) in presence of glass beads (3 mm), 100 µL 10 mM potassium phosphate buffer (pH 7.0) and 100 µL buffer lysis. For D. kunthii, TKR buffer lysis from E.Z.N.A total RNA kit I was applied (Celis-Plá et al., 2020). In the case of D. dichotoma buffer lysis described by Pearson et al. (2006), which contained Tris-EDTA (100mM tris, 50mM EDTA, pH 7.5), NaCl 2M and 2% CTAB, was used. Following protocol steps are explained in detail in Rodríguez-Rojas et al. (2020).
2.4 Concentration of thiobarbituric acid reactive substances
Lipid peroxidation was quantified directly by measuring the TBARS content as described by Rodríguez-Rojas et al. (2020), with modifications for each species. For D. kunthii, fresh biomass (~100 mg) was grounded with liquid nitrogen and mixed with 300 µL 10% TCA. For D. dichotoma ~150 mg, fresh biomass was grounded with liquid nitrogen and mixed with 450 µL 10% TCA. Extracts were vortexed for 20 min. Subsequent protocol steps are described fully in Rodríguez-Rojas et al. (2020).
2.5 Statistical analyses
The effects of brine discharge on photosynthetic activity (Fv/Fm, ETRmax, αETR, EkETR and NPQmax) and oxidative stress parameters (H2O2 and TBARS) in D. kunthii and D. dichotoma were checked by two-way ANOVA followed by a posteriori Student-Newman-Keuls-test (Underwood, 1997). A level of significance of 95% confidence interval (p = 0.05) was used. Homogeneity of variances was tested using Cochran test and visual inspection of the residuals (Underwood, 1997). All these analyses were examined with the software Statistical version 7 (Stat Soft Inc., Tulsa, OK, USA) and statistical package SPSS v.21 (IBM, USA). Principal component analysis (PCA) was constructed with 7 variables related to photosynthesis (Fv/Fm, αETR, ETRmax, EkETR and NPQmax) and oxidative stress (H2O2 and TBARS) using PERMANOVA+ with PRIMER6 package (Anderson et al., 2008). This multivariate analysis was used to decipher potential patterns of responses between the two studied macroalgal species.
2.6 Data availability
All datasets obtained to perform statistical analyses of the entire manuscript correspond to raw numerical data that is not publicly available. Nevertheless, the authors agree on sharing the raw data on request or when necessary.
3 Results
3.1 Photosynthetic activity
Photoinhibition, measured as Fv/Fm, was not observed to vary significantly in D. kunthii during transplantation experiments at day 3 (Two-way ANOVA, p>0.05; Figure 2A); at day 5 a significant increase was only observed during day 7 at the site with low salinity (S1) (Two- way ANOVA, p<0.05; Figure 2B). In contrast, in D. dichotoma a significant photoinhibition was observed at day 3, specially at the highest salinity site (S2), although it stabilized after 6 days (Two- way ANOVA, p<0.05; Figure 2B). The maximum electron transport rate (ETRmax) in D. kunthii was stable at the first 3 days; however, a significative reduction of this parameter was observed after 7 days in both impacted sites (Two- way ANOVA, p<0.05; Figure 2C). In contrast, in D. dichotoma, the ETRmax values were significantly higher in S1 after 3 days of exposition to brines (Two- way ANOVA, p<0.05; Figure 2D). After 6 days, a general decrease of the ETRmax was evidenced at all impacted sites in D. dichotoma. Photosynthetic efficiency (αETR) of D. kunthii did not vary after 3 days, but after 7 days it was significantly increased at both impacted sites (Two- way ANOVA, p<0.05; Figure 3A). In D. dichotoma αETR diminished slightly but significantly at both impacted sites exposed to brine discharge after 3 days (Two- way ANOVA, p<0.05; Figure 3B); however, after 6 days, αETR decreased significantly specially at highest salinities. In D. kunthii the saturation of irradiance (EkETR) showed a decrease tendency at both impacted sites after 3 days, and it remained at low levels after 7 days in both impacted sites compared to control site (Two- way ANOVA, p<0.05; Figure 3C). Conversely, a completely different response was observed in D. dichotoma, where EkETR was significantly higher only in S1 after 3 days, while no differences were evidenced after 6 days when compared to the control site (Two- way ANOVA, p<0.05; Figure 3D). The values estimated in D. kunthii for the maximal non photochemical quenching (NPQmax) did not show significant changes after 3 days, however, they were significantly higher when exposed to high salinities after 7 days (Two- way ANOVA, p<0.05; Figure 4A). On the contrary, an opposite response was measured in the NPQmax of D. dichotoma, where it was significantly reduced in both impacted sites after 3 days, while it remained steady after 6 days (Two- way ANOVA, p<0.05; Figure 4B).
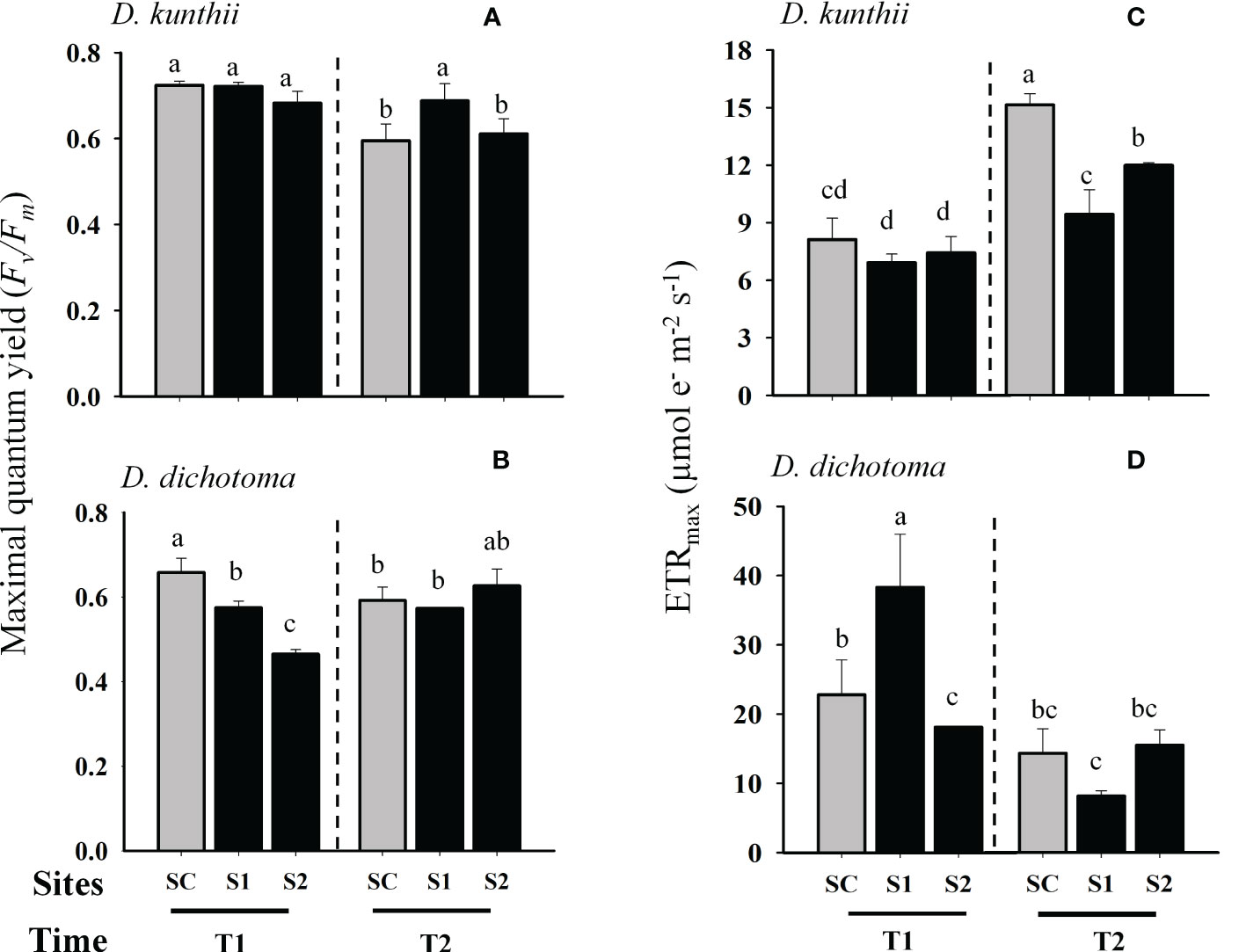
Figure 2 Photosynthetic responses as Maximum quantum yield (Fv/Fm) and Maximal electron transport rate (ETRmax) in D. kunthii and D. dichotoma transplant under the influence of brine discharge from a desalination plant. In D. kunthii, Fv/Fm (A) and ETRmax (C) were measured at 2 impacted sites with low (S1) and high (S2) influence from brine discharge from a desalination a plant located in Antofagasta, Chile and using a control site (SC) 3 (T1) and 7 (T2) days. In D. dichotoma, Fv/Fm (B) and ETRmax (D) were measured at 2 impacted with low (S1) and high (S2) influence from brine discharge from a desalination plant located in Alicante, Spain, using a control site (SC) at 3 (T1) and 6 (T2) days. Data corresponds to mean ± SD (n = 3). Letters represent statistical difference at 95% confidence interval (SNK test, p <0.05).
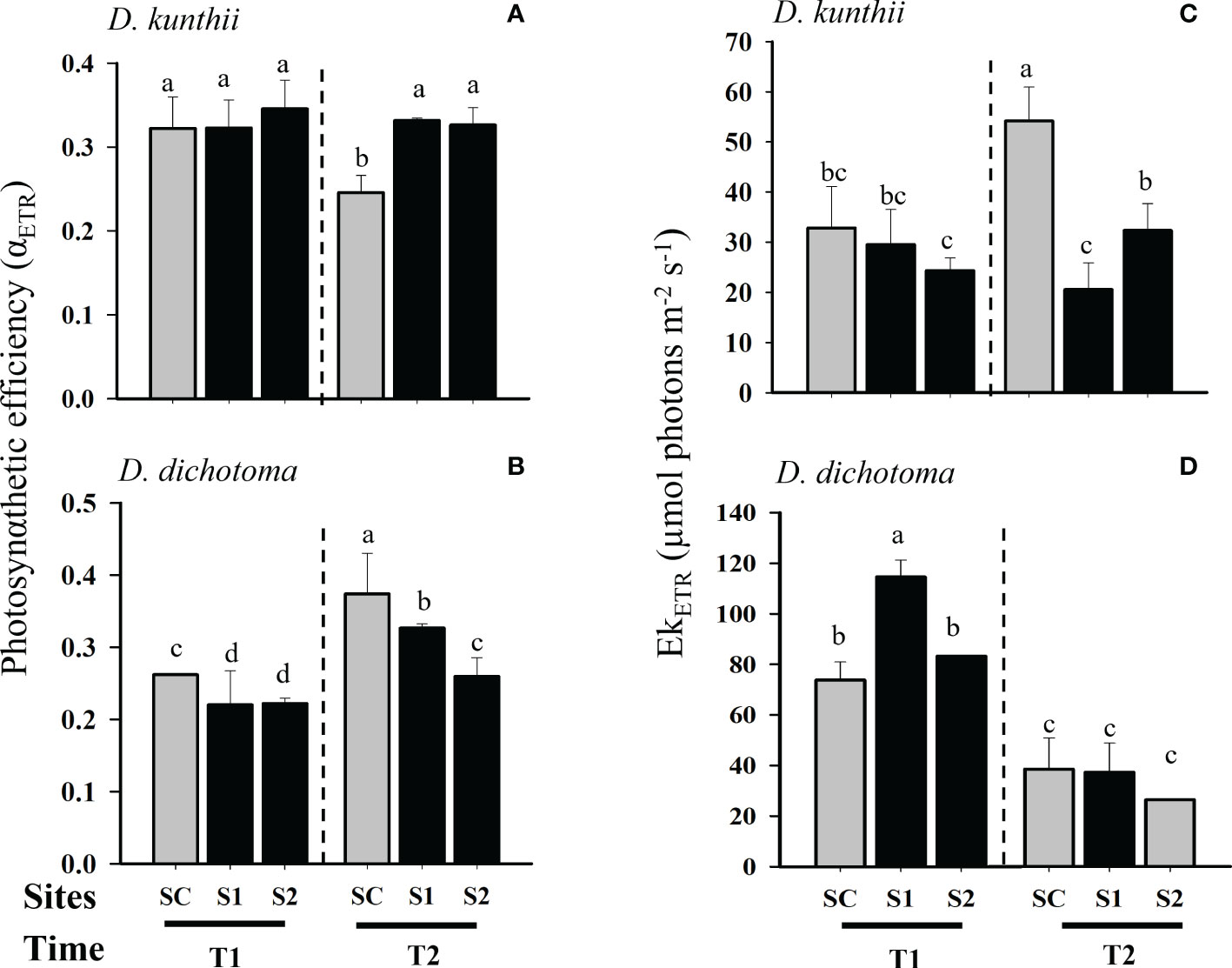
Figure 3 Photosynthetic responses as Photosynthetic efficiency (αETR) and Saturation of irradiance (EkETR) in D. kunthii and D. dichotoma transplants under the influence of brine discharge from a desalination plant. In D. kunthii, αETR (A) and EkETR (C) were measured at 2 impacted sites with low (S1) and high (S2) influence from brine discharge from a desalination plant located in Antofagasta, Chile, and using a control site (SC) at 3 (T1) and 7 (T2) days. In D. dichotoma, αETR (B) and EkETR (D) were measured in 2 impacted sites with low (S1) and high (S2) influence from brine discharge from a desalination plant located in Alicante, Spain, using a control site (SC) at 3 (T1) and 6 (T2) days. Data corresponds to mean ± SD (n = 3). Letters represent statistical difference at 95% confidence interval (SNK test, p <0.05).
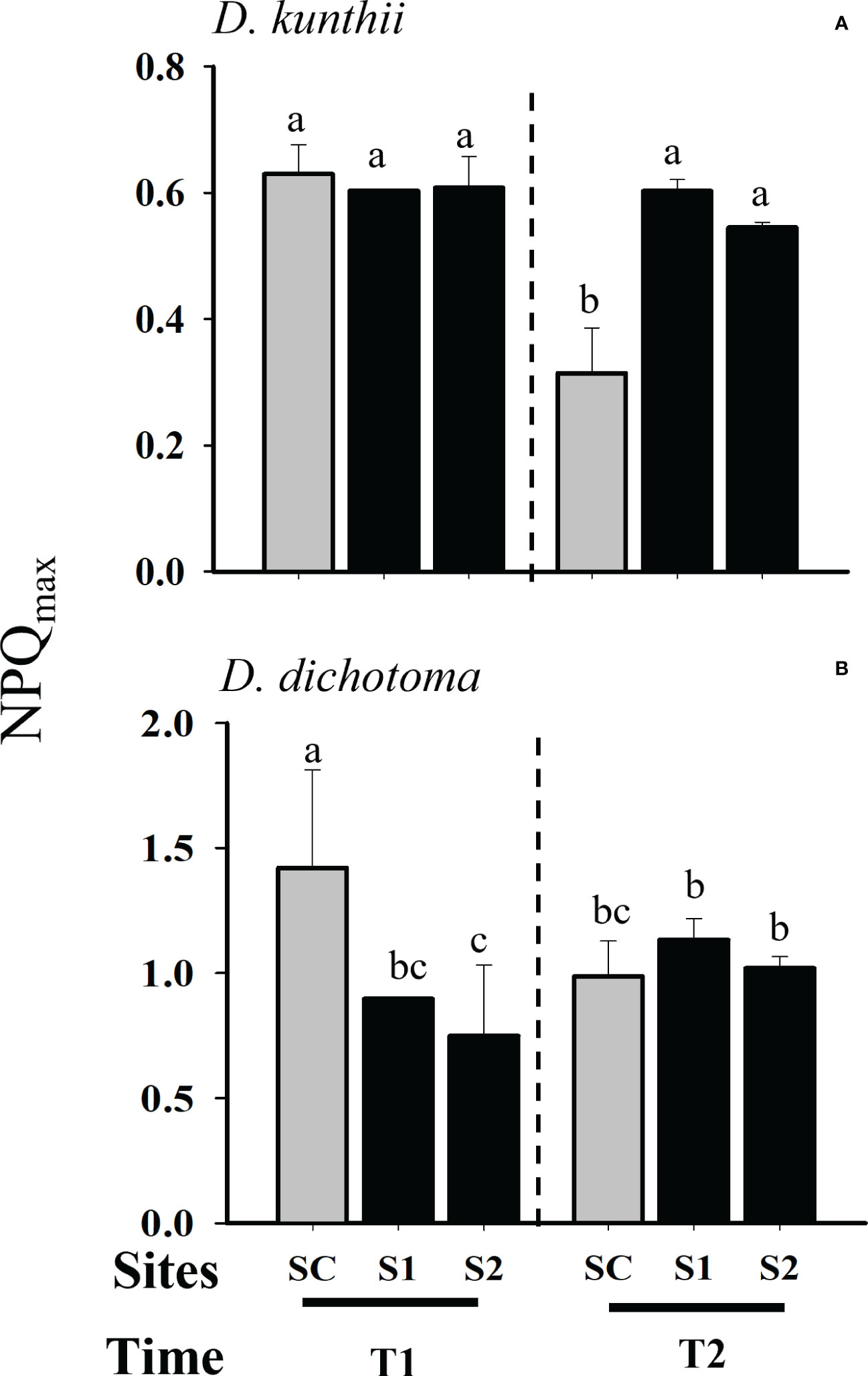
Figure 4 Photosynthetic responses as Maximal non photochemical quenching (NPQmax) in D. kunthii and D. dichotoma transplants under the influence of brine discharge from a desalination plant. In D. kunthii, NPQmax (A) was measured at 2 impacted sites with low (S1) and high (S2) influence from a brine discharge from a desalination plant located in Antofagasta, Chile, and using a control site (SC) at 3 (T1) and 7 (T2) days. In D. dichotoma, NPQmax (B) were measured at 2 impacted sites with low (S1) and high (S2) influence from a brine discharge of a desalination plant located in Alicante, Spain, using a control site (SC) at 3 (T1) and 6 (T2) days. Data corresponds to mean ± SD (n = 3). Letters represent statistical difference at 95% confidence interval (SNK test, p <0.05).
3.2 Quantification of H2O2 and TBARS content
In transplants of D. kunthii we observed that the accumulation of H2O2 was significantly higher in both impacted sites exposed to brine discharge after 3 days (Two- way ANOVA, p<0.05; Figure 5A). However, after 7 days the concentration of H2O2 diminished significantly at all sites, reaching control values. On the other hand, in D. dichotoma we did not evidenced increments in H2O2 in the transplanted samples from the impacted sites after 3 days, while at 6 days the concentrations of H2O2 were significantly higher in both transplants exposed to brine (Two- way ANOVA, p<0.05; Figure 5B). Oxidative damage, through lipid peroxidation in D. kunthii transplants, was only significantly higher at the greatest salinity site (S2) after 3 days (Two- way ANOVA, p<0.05; Figure 5C); the latter was consistent with the rise in H2O2 in this species. After 7 days lipid peroxidation decreased below control values at both impacted sites in this species (Two- way ANOVA, p<0.05; Figure 5C). Regarding D. dichotoma, the levels of lipid peroxidation were similar in all sites after 3 and 6 days, showing no differences with control samples (Two- way ANOVA, p<0.05; Figure 5D).
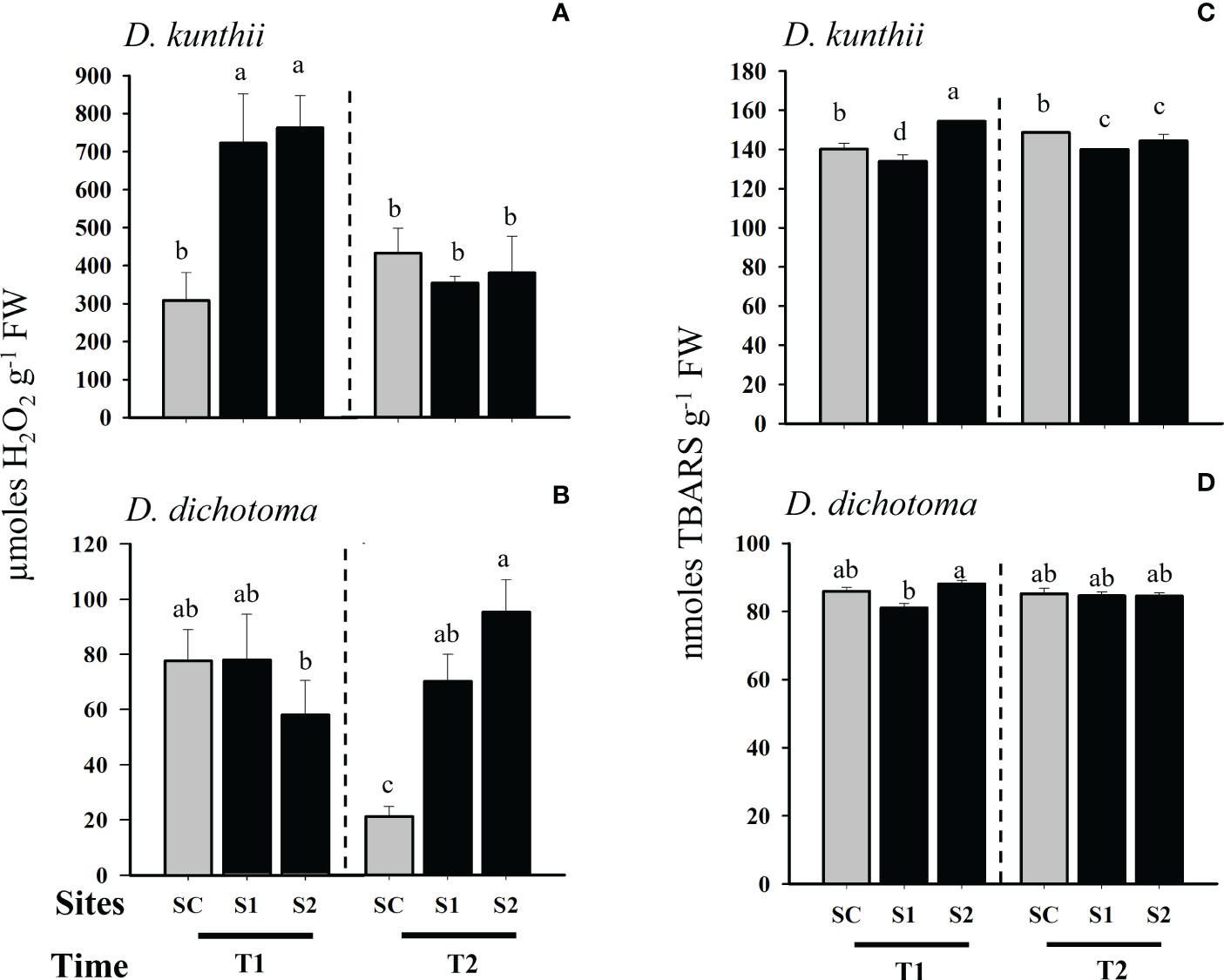
Figure 5 Oxidative stress quantified as H2O2 and TBARS in D. kunthii and D. dichotoma transplants from brine discharge from a desalination plant. In D. kunthii, H2O2 (A) and TBARS (C) were measured in 2 impacted sites with low (S1) and high (S2) influence from brine discharge of a desalination plant located in Antofagasta, Chile, and using a control site (SC) at 3 (T1) and 7 (T2) days. In D. dichotoma, H2O2 (B) and TBARS (D) were measured in 2 impacted sites with low (S1) and high (S2) influence from brine discharge from a desalination plant located in Alicante, Spain, using a control site (SC) at 3 (T1) and 6 (T2) days. Data corresponds to mean ± SD (n = 3). Letters represent statistical difference at 95% confidence interval (SNK test, p <0.05).
3.3 Principal component analysis
The PCA built with 7 variables related to photosynthesis and oxidative stress responses revealed a clear segregation between the two studied species D. kunthii (Sp1) and D. dichotoma (Sp2) along the first axis (Figure 6). The first axis of the PCA represented 98.6% of the total variation; it was mainly characterized by the two oxidative stress-related variables, and in a lower extent by the NPQmax. D. kunthii (Sp1) exhibited high variation in terms of oxidative responses.
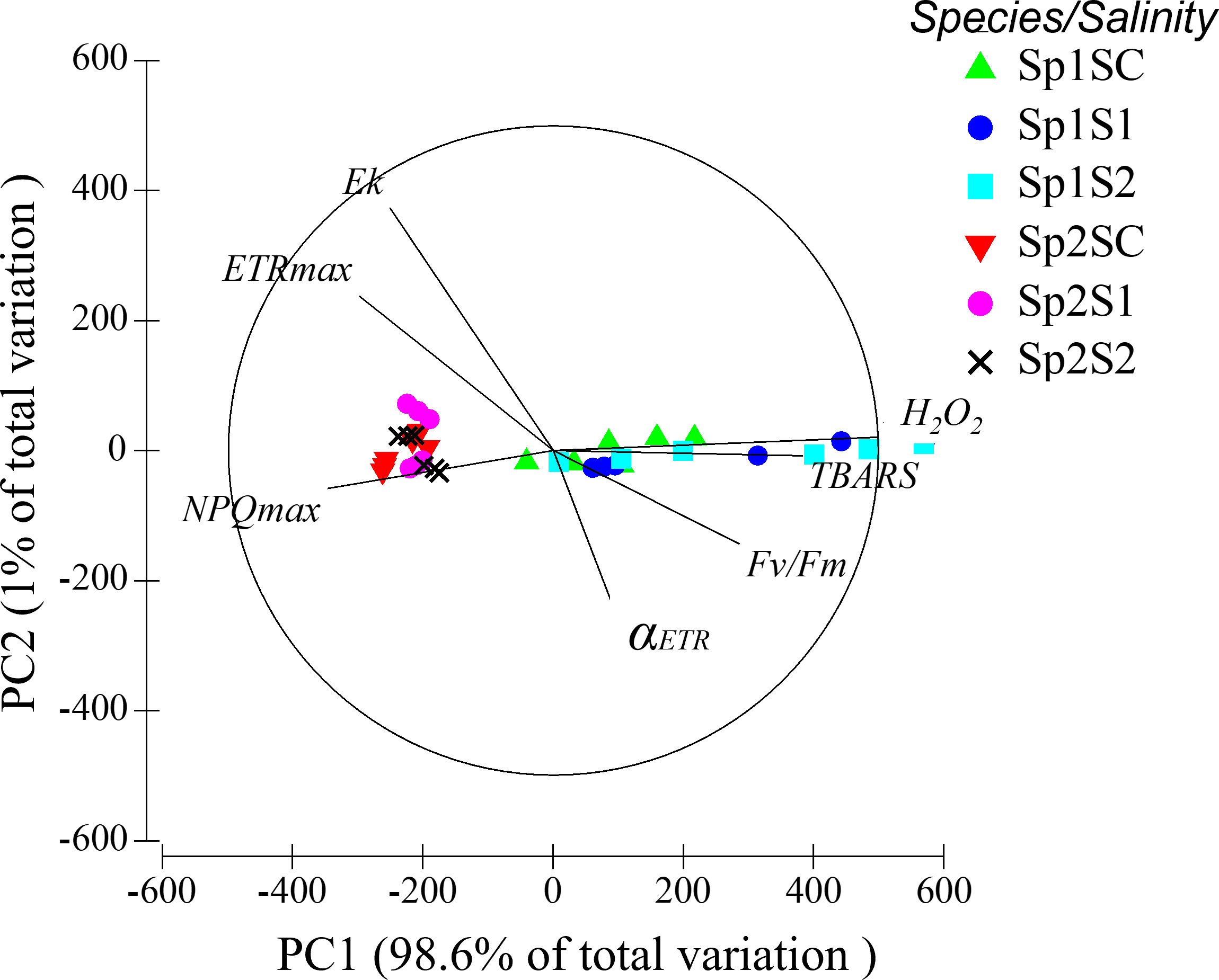
Figure 6 Principal component analysis linked to photosynthetic (Fv/Fm, αETR, ETRmax, EkETR and NPQmax) and oxidative stress (H2O2 and TBARS) parameters in D. kunthii and D. dichotoma transplants from brine discharge from a desalination plant. D. kunthii (Sp1) and D. dichotoma were transplanted in 2 impacted sites with low (S1) and high (S2) influence and control site (SC).
4 Discussion
In this study, 2 in situ transplantation experiments nearby brine discharges from SWRO desalination plants were carried out, using two phylogenetically related brown macroalgae: Dictyota kunthii and Dictyota dichotoma, which inhabit the Chilean and Spanish-Mediterranean coasts, respectively. All the analyses revealed that these macroalgae species displayed differences in their responses when were exposed to desalination brines discharges, although with common patterns that could be used as biomonitoring tools for environmental monitoring programs (EMPs).
During the evaluation of the photosynthetic performance, the maximal quantum yield (Fv/Fm) was used as a representation of inhibition of photosynthesis, which is also intricately linked to the electrons transport rate (ETR) (Celis-Plá et al., 2014). In this sense, D. kunthii transplants exposed to brines from a desalination plant in Chile did not show inhibition of photosynthesis when located nearby the discharges, although there was a decrease of ETRmax at 7 days of brine exposure. In contrast, in D. dichotoma photoinhibition was only observed at 3 days of exposition to brines, and the diminution of ETRmax was only observed in the site with the highest brine influence at 3 days. Previous laboratory-controlled experiments using these 2 species have shown that D. kunthii display a similar response to those observed in the field against salinities, but instead subject to artificial sea salts, while in D. dichotoma differences in photosynthetic parameters were more related to time-exposure when in the field (Muñoz et al. under review). This suggests that brine discharges can impact on primary productivity and photosynthetic capacity in a distinct manner depending on the species tolerance threshold. These may indicate that the 2 different species have distinct timing in pumping within electron transport chains under osmotic stress. In this regard, mesocosms experiments in the seagrass Posidonia australis exposed to several brine dilutions demonstrated that only the highest salinity treatment (54 psu) induced a decrease in ETRmax, compared to control (37 psu), while artificial salts addition to increase salinities (46 to 54 psu), did not generate significant effects, even after 2 weeks (Cambridge et al., 2019). Moreover, field transplantation experiments nearby a brine discharge from a SWRO desalination plant reported inhibition of photosynthesis and low primary productivity after 7 days in the brown seaweed Ectocarpus when exposed to brine salinities of ~36 and ~37 psu compared to a control site at ~34 psu (Rodríguez-Rojas et al., 2020). In overall, this may indicate that desalination discharges do not only stand for excess salinities but perhaps with other components of the brines or due to the mix with natural biological and physicochemical characteristics of the receptor coastal ecosystem. In the cellular context, it has been reported that salt stress can reduce photosynthetic performance by altering chloroplast number and ultrastructure, photosynthetic light harvesting apparatus capacity and inhibition of photosynthetic enzymes (Xia et al., 2004; Sandoval-Gil et al., 2014; Anandraj et al., 2020). In controlled laboratory experiments, Lu et al. (2006) demonstrated that increased salinities with NaCl caused a marked reduction of Fv/Fm in Ulva fasciata after 4 days of exposition. Low ETRmax values obtained in both transplanted species at impacted sites by brine discharges may be explained by chloroplast structure damage and disruption of electron transport chains, mainly due to the accumulation of ROS as H2O2 induced by osmotic stress (Imlay, 2003; Liang et al., 2014; Shetty et al., 2019). In this line, Rodríguez-Rojas et al. (2020) suggested a link between impaired photosynthetic performance and the accumulation of H2O2 in the brown macroalga Ectocarpus transplanted nearby brine discharges in the field. On the other hand, laboratory experiments on 2 diatoms species, Phaeodactylum tricornutum and Chaetoceros gracilis, showed a gradual decrease of rETR as salinity increased from 30 psu (control) to 40, 60, 80 and 90 psu, for up to 48 hours (Liang et al., 2014). To this end, a high salinity combined with distinct external factors that may affect mixing and slow the brine dilution from the discharge (e.g. weather conditions, diffuser malfunction, additives, etc.) could have intensified stress symptoms in D. kunthii, which also demonstrated to be physiologically more sensitive than D. dichotoma to brine exposure.
On the other hand, the increment of saturated irradiance (EkETR) and photosynthetic efficiency (αETR) can be considered as indicators of photoacclimation to different irradiances (Zúñiga et al., 2021). Interestingly, brine exposition both in Chile and Spain induced a higher αETR, although in D. kunthii it was observed at 7 days and in D. dichotoma during the whole experimental time. This suggest a better photoacclimation of D. kunthii when compared to D. dichotoma when exposed to brine discharges. Trends of decay in photoacclimation and αETR were obtained in both species under excess salinities in laboratory-controlled experiments (Muñoz et al. under review), confirming that responses recorded in the field in this study were indeed related to brine-derived osmotic stress. On the other hand, different responses between Dictyota species might reflect inter-specific strategies to capture and use light, which could be closely linked with their vertical tidal distribution and the level of investment in protecting their antenna pigments (Sandoval-Gil et al., 2014). Certainly, a deeper subtidal macroalgae, as D. kunthii (collected at 15 m depth), is more adapted to low light levels in relation to shallower species as D. dichotoma, and therefore more efficient in capturing low light levels. This may explain the observed higher values of αETR and low light requirement to saturate (EkETR) electron transport rates D. kunthii compared to D. dichotoma (Johansson and Snoeijs, 2002; Hanelt et al., 2003; Celis-Plá et al., 2014). Although D. kunthii was transplanted at the same depth than their baseline ecosystem, D. dichotoma had to be transferred from 2 m to 4 m depth. In this regard, research by Celis-Plá et al. (2014) demonstrated a decrease in αETR in the brown macroalga Cystoseira tamariscifolia when was transferred from 0.5 m to 2 m depth. Thus, the re-location of D. dichotoma from shallow to a deeper waters, combined with brines exposure, could have intensified damage to the light harvesting system reducing its photosynthetic efficiency, compared to D. kunthii.
Maximal non-photochemical quenching (NPQmax) is a form of energy dissipation through heat, which also is an indicator of photoprotection and photoinhibition. Indeed, this process finally controls ROS imbalance under stress conditions (Gao et al., 2016; Zúñiga et al., 2021). Our results showed that NPQmax increased similarly in D. kunthii at both brine-influenced sites, but only after 7 days. This suggests that NPQmax may play a late role as an efficient photoprotection mechanism for maintaining photosynthesis under salinity excess in D. kunthii (Gao et al., 2016; Zou et al., 2018). Interestingly, this response was not observed under laboratory-controlled experiments (Muñoz et al. under review), indicating that the environmental complexity of field experiments may have added an additional source of stress. On the other hand, NPQmax decreased in D. dichotoma at impacted sites after 3 days, which was in accord with measurements obtained in laboratory-controlled conditions (Muñoz et al. under review), suggesting that hypersalinity did not induce a heat dissipation processes in this species. In this regard, research on the brown alga Sargassum fusiforme detected an increment of NPQ at high salinities of 30 (control), 45 and 60 psu after 120 min (Gao et al., 2016). Also, in Ulva prolifera the exposition to high salinity (90 psu) for 4 days increased the NPQ (Zheng et al., 2019). In contrast, no significant changes in NPQmax were observed Sargassum polycystum when exposed to high salinity (40 psu) after 1 hour (Zou et al., 2018). Altogether, these results suggest that desalination brines generate a higher stress on the photosynthetic apparatus of D. kunthii compared to D. dichotoma, which is reflected in distinct mechanisms of photoprotection. Moreover, our laboratory and field investigations in Dictyota species and those available in the literature demonstrate that hypersalinity effects on photosynthetic performance are especially relevant in the short-term and dependent of the degree of salinity excess; moreover, the data demonstrate an acclimatation process after days of exposure to increased salinities.
The accumulation of ROS and oxidative stress is a common effect of abiotic perturbations (e.i. hypersalinity); biological pathways are diverse, but most of them lead to disruption of the electron transport chains in mitochondria and chloroplasts (Imlay, 2003; Moenne et al., 2016). The intracellular accumulation of salt ions caused by extracellular hypersalinity and subsequent dehydration can mediate excess H2O2 which, in turn, may induce the overproduction of hydroxyl radical (HO·) by Fenton Haber-Weiss reaction and other ROS; altogether, responsible for oxidative stress and damage (Girotti, 2001; Lesser, 2006; Kumar et al., 2014; Moenne et al., 2016). In this study, as an oxidative stress approach we determined H2O2, showing that D. kunthii can accumulate up to 5-fold more of H2O2 than D. dichotoma in the transplants nearby brine discharges, although this was only observed after 3 days. This is linked with the significant high lipid peroxidation levels observed in D. kunthii at day 3 on the site S2 with higher brine influence, compared to impacted site S1 and controls; however, lipid peroxidation evidenced a rexovery process returning to baseline values at 7 days. An opposite response was observed in D. dichotoma, where the highest levels of H2O2 were identified at day 6 in both brine-impacted sites, although this was not reflected in an increase in lipid peroxidation. These different responses could be explained by dissimilar effectiveness in ROS scavenger mechanisms and/or lipid peroxidation repair systems used against brine-induced ROS excess. In contrast with this field study, in laboratory experiments with high salinities extrapolable to brine discharges, H2O2 concentration increased at 7 days in D. kunthii, whereas in D. dichotoma the increase was at 3 days only; furthermore, without displaying any significant lipid peroxidation levels in both species (Muñoz et al. under review). Thus, this demonstrate that these species have very different tolerance mechanisms to respond under hypersalinity, combined with the potential other fluctuating factor that may be occurring in the natural environment (e.g. temperature, light availability, currents). In this context, in previous transplantation experiments on the brown macroalga Ectocarpus, the influence of brine discharges induced a more marked oxidative stress condition, which was evidenced through the increment of H2O2 concentration at 3 and 7 days, and also lipid peroxidation after 3 days (Rodríguez-Rojas et al., 2020). Similar oxidative stress condition was demonstrated by Capó et al. (25) when individuals of the seagrass P. oceanica collected nearby brine discharge sites from desalination plant (40 psu); these displayed significant higher lipid peroxidation levels compared to control (38 psu). Moreover, in controlled salinity conditions, Luo and Liu (2011) demonstrated that the green macroalga Ulva prolifera subject to ~60 psu (over a control at ~30 psu) suffers an oxidative stress condition due to increased H2O2 and lipid peroxidation levels after 6 days. Other studies by Wang et al. (2019) on red macroalga Pyropia haitanensis demonstrated a significative increase of H2O2 accumulation and lipid peroxidation during hypersalinity condition (110 psu) when compared to control (30 psu) after 24 hours. Likewise, on green alga Ulva compressa, it was demonstrated that increased salinity (82 psu) induced a higher H2O2 accumulation after 6 days of exposition (Muñoz et al., 2020). Thus, our transplantation experiments in the two Dictyota species considered in this study put in evidence that salinity excess due to brine exposure can cause mild negative effects on photosynthetic activity and ROS balance, although with interspecific variations; however, both species shared that displayed a set of tolerant responses to osmotic stress that never compromised their biological viability and survival. Therefore, the tested functional indicators and biomarkers in both D. kunthii and D. dichotoma seem to be reliable environmental biotechnology tools; indeed, and given the cosmopolitan distribution of Dictyota, responses of other species within the genus may be also relevant to follow the extent impact of desalination operations.
5 Conclusions
In this study, we observed hypersalinity-specific tolerance strategies in D. kunthii and D. dichotoma. In this regard, the exposition to brine discharges from desalination plants generated a higher and marked stress response in D. kunthii, through lower primary productivity, decrease in light requirements, an excessive energy dissipation an increased oxidative stress condition. On the other hand, responses of D. dichotoma demonstrated a slight affection in photosynthetic performance, but high accumulation of H2O2 as an early response. Comparing the results with our previously conducted controlled laboratory experiments on both Dictyota species, we observed more marked tolerance responses in field experiments under brine influence, especially in D. kunthii, which may be related with other components of the brine and/or the combination of high salinity with other natural fluctuating environmental conditions of the areas. In the context to desalination biomonitoring, our study suggests that the best biomarkers to monitor desalination plants using species of the genus Dictyota, include photosynthetic parameters such as ETRmax and H2O2 as a representration of oxidative stress conditions. The present study contributes to the understanding of tolerance strategies of two brown macroalgae exposed to the brine discharge by in situ experiments nearby SWRO desalination plants. This knowledge certainly assists in providing environmental biomonitoring tools that could be eventually extrapolated to other species of the genus Dictyota around the World.
Data availability statement
The raw data supporting the conclusions of this article will be made available by the authors, without undue reservation.
Author contributions
PM: conceptualization, field work, methodology, formal analysis, writing original draft, visualization. FR-R: field work, methodology, funding acquisition, writing. PC-P: field work, methodology, formal analysis. AL-M: field work. FB-M: manuscript review and editing. IS: manuscript review and editing. CL: writing, formal analyses, manuscript review. FV: conceptualization, field work, methodology. RO: conceptualization, field work, methodology JS-L: conceptualization, formal analysis, methodology, field work, investigation, resources, review and editing. CS: conceptualization, field work, methodology, visualization, writing review and editing, supervision, funding acquisition. All authors contributed to the article and approved the submitted version.
Acknowledgments
We gratefully thank financial support to ANID FONDECYT Postdoctoral fellowship #3180394, European Commission Marie Skłodowska-Curie Actions #888415, and ANID INES I+D # INID210013. Financial support for mobility granted from SEGIB Scholarship and Fundación Carolina of Spain to PM. Also, we thank TESPOST 04/19 PhD scholarship granted by Universidad de Playa Ancha to PM.
Conflict of interest
The authors declare that the research was conducted in the absence of any commercial or financial relationships that could be construed as a potential conflict of interest.
Publisher’s note
All claims expressed in this article are solely those of the authors and do not necessarily represent those of their affiliated organizations, or those of the publisher, the editors and the reviewers. Any product that may be evaluated in this article, or claim that may be made by its manufacturer, is not guaranteed or endorsed by the publisher.
References
Anandraj A., White S., Naidoo D., Mutanda T. (2020). Monitoring the acclimatization of a Chlorella sp. from freshwater to hypersalinity using photosynthetic parameters of pulse amplitude modulated fluorometry. Bioresource Technol. 309, 123380. doi: 10.1016/j.biortech.2020.123380
Anderson M., Gorley R. N., Clarke K. (2008). PERMANOVA+ for primer: Guide to software and statistical methods (Plymouth, United Kingdom).
Belkin N., Rahav E., Elifantz H., Kress N., Berman-Frank I. (2017). The effect of coagulants and antiscalants discharged with seawater desalination brines on coastal microbial communities: a laboratory and in situ study from the southeastern Mediterranean. Water Res. 110, 321–331. doi: 10.1016/j.watres.2016.12.013
Benaissa M., Rouane-Hacene O., Boutiba Z., Habib D., Guibbolini-Sabatier M. E., Risso-De Faverney C. (2020). Ecotoxicological effects assessment of brine discharge from desalination reverse osmosis plant in Algeria (South Western Mediterranean). Regional Stud. Mar. Sci. 39, 101407. doi: 10.1016/j.rsma.2020.101407
Bisson M. A., Kirst G. O. (1995). Osmotic acclimation and turgor pressure regulation in algae. Naturwissenschaften 82, 461–471. doi: 10.1007/BF01131597
Cambridge M. L., Zavala-Perez A., Cawthray G. R., Statton J., Mondon J., Kendrick G. A. (2019). Effects of desalination brine and seawater with the same elevated salinity on growth, physiology and seedling development of the seagrass Posidonia australis. Mar. pollut. Bull. 140, 462–471. doi: 10.1016/j.marpolbul.2019.02.001
Campero C., Harris L. M. (2019). The legal geographies of water claims: seawater desalination in mining regions in Chile. Water 11, 886. doi: 10.3390/w11050886
Capó X., Tejada S., Ferriol P., Pinya S., Mateu-Vicens G., Montero-González I., et al. (2020). Hypersaline water from desalinization plants causes oxidative damage in Posidonia oceanica meadows. Sci. Total Environ. 736, 139601. doi: 10.1016/j.scitotenv.2020.139601
Celis-Plá P., Kappes J., Figueroa F., Pereda S., Villegas K., Altamirano R., et al. (2021). Solar radiation as an isolated environmental factor in an experimental mesocosm approach for studying photosynthetic acclimation of macrocystis pyrifera (Ochrophyta). Front. Plant Sci. 12, 622150. doi: 10.3389/fpls.2021.622150
Celis-Plá P., Martínez B., Quintano E., García-Sánchez M., Pedersen A., Navarro N. P., et al. (2014). Short-term ecophysiological and biochemical responses of Cystoseira tamariscifolia and Ellisolandia elongata to environmental changes. Aquat. Biol. 22, 227–243. doi: 10.3354/ab00573
Celis-Plá P. S. M., Moenne F., Rodríguez-Rojas F., Pardo D., Lavergne C., Moenne A., et al. (2020). Antarctic Intertidal macroalgae under predicted increased temperatures mediated by global climate change: Would they cope? Sci. Total Environ. 740, 140379. doi: 10.1016/j.scitotenv.2020.140379
Del Pilar Ruso Y., Martínez-García E., Giménez-Casalduero F., Loya-Fernández A., Ferrero-Vicente L. M., Marco-Méndez C., et al. (2015). Benthic community recovery from brine impact after the implementation of mitigation measures. Water Res. 70, 325–336. doi: 10.1016/j.watres.2014.11.036
Elsaid K., Sayed E. T., Abdelkareem M. A., Baroutaji A., Olabi A. G. (2020). Environmental impact of desalination processes: Mitigation and control strategies. Sci. Total Environ. 740, 140125. doi: 10.1016/j.scitotenv.2020.140125
Fernández-Torquemada Y., Carratalá A., Sánchez Lizaso J. L. (2019). Impact of brine on the marine environment and how it can be reduced. Desalination Water Treat 167, 27–37. doi: 10.5004/dwt.2019.24615
Fernández-Torquemada Y., Sánchez-Lizaso J. L. (2007). Monitoring of brine discharges from seawater desalination plants in the Mediterranean. Int. J. Environ. Health 1, 449–461. doi: 10.1504/IJENVH.2007.017870
Fuentes-Bargues J. L. (2014). Analysis of the process of environmental impact assessment for seawater desalination plants in Spain. Desalination 347, 166–174. doi: 10.1016/j.desal.2014.05.032
Gao S., Huan L., Lu X. P., Jin W. H., Wang X. L., Wu M. J., et al. (2016). Photosynthetic responses of the low intertidal macroalga Sargassum fusiforme (Sargassaceae) to saline stress. Photosynthetica 54, 430–437. doi: 10.1007/s11099-015-0181-7
Girotti A. W. (2001). Photosensitized oxidation of membrane lipids: reaction pathways, cytotoxic effects, and cytoprotective mechanisms. J. Photochem. Photobiol. B: Biol. 63, 103–113. doi: 10.1016/S1011-1344(01)00207-X
Hak-Jyung L., Ho yeon Y. (2018). Study of functional verification to abiotic stress through antioxidant gene transformation of Pyropia yezoensis (Bangiales, rhodophyta) APX and MnSOD in chlamydomonas. J. Microbiol. Biotechnol. 28, 1217–1224. doi: 10.4014/jmb.1802.02024
Hanelt D., Wiencke C., Bischof K. (2003). “Photosynthesis in marine macroalgae,” in Photosynthesis in algae. Eds. Larkum A. W. D., Douglas S. E., Raven J. A. (Dordrecht: Springer Netherlands), 413–435.
Herrera-León S., Cruz C., Kraslawski A., Cisternas L. A. (2019). Current situation and major challenges of desalination in Chile. Desalination Water Treat 171, 93–104. doi: 10.5004/dwt.2019.24863
Hoepner T., Lattemann S. (2003). Chemical impacts from seawater desalination plants — a case study of the northern red Sea. Desalination 152, 133–140. doi: 10.1016/S0011-9164(02)01056-1
Hoffmann A., Santelices B. (1997). Flora marina de Chile central (Santiago, Chile: Ediciones Universidad Catolica de Chile), 434.
Imlay J. A. (2003). Pathways of oxidative damage. Annu. Rev. Microbiol. 57, 395–418. doi: 10.1146/annurev.micro.57.030502.090938
Johansson G., Snoeijs P. (2002). Macroalgal photosynthetic responses to light in relation to thallus morphology and depth zonation. Mar. Ecol. Prog. Ser. 244, 63–72. doi: 10.3354/meps244063
Jones E., Qadir M., van Vliet M. T. H., Smakhtin V., Kang S.-m. (2019). The state of desalination and brine production: A global outlook. Sci. Total Environ. 657, 1343–1356. doi: 10.1016/j.scitotenv.2018.12.076
Kirst G. (1990). Salinity tolerance of eukaryotic marine algae. Annu. Rev. Plant Biol. 41, 21–53. doi: 10.1146/annurev.pp.41.060190.000321
Kress N., Galil B. (2018). “Impact of seawater desalination by reverse osmosis on the marine environment,” in Efficient desalination by reverse osmosis (London: IWA Publishing).
Kumar M., Kumari P., Reddy C., Jha B. (2014). Salinity and desiccation induced oxidative stress acclimation in seaweeds. Adv. Botanical Res. 71, 91–123. doi: 10.1016/B978-0-12-408062-1.00004-4
Lattemann S., Höpner T. (2008). Environmental impact and impact assessment of seawater desalination. Desalination 220, 1–15. doi: 10.1016/j.desal.2007.03.009
Lesser M. P. (2006). Oxidative stress in marine environments: biochemistry and physiological ecology. Annu. Rev. Physiol. 68, 253–278. doi: 10.1146/annurev.physiol.68.040104.110001
Liang Y., Sun M., Tian C., Cao C., Li Z. (2014). Effects of salinity stress on the growth and chlorophyll fluorescence of Phaeodactylum tricornutum and Chaetoceros gracilis (Bacillariophyceae). Botánica Marina 57, 469–476. doi: 10.1515/bot-2014-0037
Loya-Fernández Á, Ferrero-Vicente L. M., Marco-Méndez C., Martínez-García E., Zubcoff J., Sánchez-Lizaso J. L. (2012). Comparing four mixing zone models with brine discharge measurements from a reverse osmosis desalination plant in Spain. Desalination 286, 217–224. doi: 10.1016/j.desal.2011.11.026
Luo M. B., Liu F. (2011). Salinity-induced oxidative stress and regulation of antioxidant defense system in the marine macroalga Ulva prolifera. J. Exp. Mar. Biol. Ecol. 409, 223–228. doi: 10.1016/j.jembe.2011.08.023
Lu I. F., Sung M.-S., Lee T.-M. (2006). Salinity stress and hydrogen peroxide regulation of antioxidant defense system in Ulva fasciata. Mar. Biol. 150, 1–15. doi: 10.1007/s00227-006-0323-3
Missimer T. M., Maliva R. G. (2018). Environmental issues in seawater reverse osmosis desalination: Intakes and outfalls. Desalination 434, 198–215. doi: 10.1016/j.desal.2017.07.012
Moenne A., González A., Sáez C. A. (2016). Mechanisms of metal tolerance in marine macroalgae, with emphasis on copper tolerance in chlorophyta and rhodophyta. Aquat. Toxicol. 176, 30–37. doi: 10.1016/j.aquatox.2016.04.015
Morrison J., Morikawa M., Murphy M., Schulte P. (2009). Water scarcity & climate change: growing risks for businesses & investors (Boston, United States: Oakland Pacific Institute), 60. Ceres.
Muñoz P. T., Rodríguez-Rojas F., Celis-Plá P. S. M., Méndez L., Pinto D., Pardo D., et al. (2020). Physiological and metabolic responses to hypersalinity reveal interpopulation tolerance in the green macroalga Ulva compressa with different pollution histories. Aquat. Toxicol. 225, 105552. doi: 10.1016/j.aquatox.2020.105552
Panagopoulos A. (2022a). Process simulation and analysis of high-pressure reverse osmosis (HPRO) in the treatment and utilization of desalination brine (saline wastewater). Int. J. Energy Res 46, 23083–23094. doi: 10.1002/er.8607
Panagopoulos A. (2022b). Techno-economic assessment and feasibility study of a zero liquid discharge (ZLD) desalination hybrid system in the Eastern Mediterranean. Chem. Eng. Process. - Process Intensification 178, 109029. doi: 10.1016/j.cep.2022.109029
Panagopoulos A. (2022c). Brine management (saline water & wastewater effluents): Sustainable utilization and resource recovery strategy through minimal and zero liquid discharge (MLD & ZLD) desalination systems. Chem. Eng. Process. - Process Intensification 176, 108944. doi: 10.1016/j.cep.2022.108944
Panagopoulos A., Giannika V. (2022a). Decarbonized and circular brine management/valorization for water & valuable resource recovery via minimal/zero liquid discharge (MLD/ZLD) strategies. J. Environ. Manage. 324, 116239. doi: 10.1016/j.jenvman.2022.116239
Panagopoulos A., Giannika V. (2022b). Comparative techno-economic and environmental analysis of minimal liquid discharge (MLD) and zero liquid discharge (ZLD) desalination systems for seawater brine treatment and valorization. Sustain. Energy Technol. Assessments 53, 102477. doi: 10.1016/j.seta.2022.102477
Pearson G., Lago-Leston A., Valente M., Serrão E. (2006). Simple and rapid RNA extraction from freeze-dried tissue of brown algae and seagrasses. Eur. J. Phycology 41, 97–104. doi: 10.1080/09670260500505011
Rodríguez-Rojas F., López-Marras A., Celis-Plá P. S. M., Muñoz P., García-Bartolomei E., Valenzuela F., et al. (2020). Ecophysiological and cellular stress responses in the cosmopolitan brown macroalga Ectocarpus as biomonitoring tools for assessing desalination brine impacts. Desalination 489, 114527. doi: 10.1016/j.desal.2020.114527
Sáez C. A., Roncarati F., Moenne A., Moody A. J., Brown M. T. (2015). Copper-induced intra-specific oxidative damage and antioxidant responses in strains of the brown alga Ectocarpus siliculosus with different pollution histories. Aquat. Toxicol. 159, 81–89. doi: 10.1016/j.aquatox.2014.11.019
Samanta P., Shin S., Jang S., Kim J. K. (2019). Comparative assessment of salinity tolerance based on physiological and biochemical performances in Ulva australis and Pyropia yezoensis. Algal Res. 42, 101590. doi: 10.1016/j.algal.2019.101590
Sandoval-Gil J. M., Ruiz J. M., Marín-Guirao L., Bernardeau-Esteller J., Sánchez-Lizaso J. L. (2014). Ecophysiological plasticity of shallow and deep populations of the Mediterranean seagrasses Posidonia oceanica and Cymodocea nodosa in response to hypersaline stress. Mar. Environ. Res. 95, 39–61. doi: 10.1016/j.marenvres.2013.12.011
Schewe J., Heinke J., Gerten D., Haddeland I., Arnell N. W., Clark D. B., et al. (2014). Multimodel assessment of water scarcity under climate change. Proc. Natl. Acad. Sci. 111, 3245–3250. doi: 10.1073/pnas.1222460110
Schreiber U., Bilger W., Neubauer C. (1995). “Chlorophyll fluorescence as a nonintrusive indicator for rapid assessment of in vivo photosynthesis,” in Ecophysiology of photosynthesis (Berlin, Heidelberg: Springer), 49–70.
Shetty P., Gitau M. M., Maróti G. (2019). Salinity stress responses and adaptation mechanisms in eukaryotic green microalgae. Cells 8, 1657. doi: 10.3390/cells8121657
Sola I., Sánchez-Lizaso J. L., Muñoz P. T., García-Bartolomei E., Sáez C. A., Zarzo D. (2019). Assessment of the requirements within the environmental monitoring plans used to evaluate the environmental impacts of desalination plants in Chile. Water 11, 2085. doi: 10.3390/w11102085
Sola I., Zarzo D., Carratalá A., Fernández-Torquemada Y., de-la-Ossa-Carretero J. A., Del-Pilar-Ruso Y., et al. (2020). Review of the management of brine discharges in Spain. Ocean Coast. Manage. 196, 105301. doi: 10.1016/j.ocecoaman.2020.105301
Tronholm A., Sansón M., Afonso-Carrillo J., Clerck O. D. (2008). Distinctive morphological features, life-cycle phases and seasonal variations in subtropical populations of Dictyota dichotoma (Dictyotales, phaeophyceae). Botanica Marina 51, 132–144. doi: 10.1515/BOT.2008.017
Underwood A. J. (1997). Experiments in ecology: their logical design and interpretation using analysis of variance (Cambridge, United Kingdom: Cambridge University Press).
Wang W., Xu Y., Chen T., Xing L., Xu K., Xu Y., et al. (2019). Regulatory mechanisms underlying the maintenance of homeostasis in Pyropia haitanensis under hypersaline stress conditions. Sci. Total Environ. 662, 168–179. doi: 10.1016/j.scitotenv.2019.01.214
Xia J., Li Y., Zou D. (2004). Effects of salinity stress on PSII in Ulva lactuca as probed by chlorophyll fluorescence measurements. Aquat. Bot. 80, 129–137. doi: 10.1016/j.aquabot.2004.07.006
Zheng Z., Gao S., Wang G. (2019). High salt stress in the upper part of floating mats of Ulva prolifera, a species that causes green tides, enhances non-photochemical quenching. J. Phycology 55, 1041–1049. doi: 10.1111/jpy.12881
Zou X.-X., Xing S.-S., Su X., Zhu J., Huang H.-Q., Bao S.-X. (2018). The effects of temperature, salinity and irradiance upon the growth of Sargassum polycystum c. agardh (Phaeophyceae). J. Appl. Phycology 30, 1207–1215. doi: 10.1007/s10811-017-1282-4
Keywords: brown macroalgae, photosynthesis, oxidative stress, brine discharge, transplant
Citation: Muñoz PT, Rodríguez-Rojas F, Celis-Plá PSM, López-Marras A, Blanco-Murillo F, Sola I, Lavergne C, Valenzuela F, Orrego R, Sánchez-Lizaso JL and Sáez CA (2023) Desalination effects on macroalgae (part b): Transplantation experiments at brine-impacted sites with Dictyota spp. from the Pacific Ocean and Mediterranean Sea. Front. Mar. Sci. 10:1042799. doi: 10.3389/fmars.2023.1042799
Received: 13 September 2022; Accepted: 03 January 2023;
Published: 03 February 2023.
Edited by:
Lihua Yang, Sun Yat-sen University, ChinaReviewed by:
Argyris Panagopoulos, National Technical University of Athens, GreeceThierry Thibaut, Aix-Marseille Université, France
Copyright © 2023 Muñoz, Rodríguez-Rojas, Celis-Plá, López-Marras, Blanco-Murillo, Sola, Lavergne, Valenzuela, Orrego, Sánchez-Lizaso and Sáez. This is an open-access article distributed under the terms of the Creative Commons Attribution License (CC BY). The use, distribution or reproduction in other forums is permitted, provided the original author(s) and the copyright owner(s) are credited and that the original publication in this journal is cited, in accordance with accepted academic practice. No use, distribution or reproduction is permitted which does not comply with these terms.
*Correspondence: Claudio A. Sáez, claudio.saez@upla.cl