- 1Research Group Plankton, Institute of Marine Research, Bergen, Norway
- 2Department of Biological Sciences (BIO), University of Bergen, Bergen, Norway
- 3Bjerknes Centre for Climate Research, Bergen, Norway
The glacier lanternfish Benthosema glaciale is a key myctophid with a wide distribution in the northern Atlantic. It is a species that has a strong vertical migration capacity and have the potential to move between the surface waters and mesopelagic depths in a diel cycle (DVM), mainly depending on ambient light conditions. We investigated the feeding ecology of B. glaciale across the Norwegian, Iceland, Irminger and Labrador Sea basins. An important component of Benthosema diet was various types of calanoid copepods, but with some additional variability across the deep basins. ‘House’s’ of Appendicularia were only found in stomachs from the Labrador basin and were positively selected for here. The large calanoid Calanus hyperboreus was strongly selected for in the Iceland Sea, while its smaller counterpart C. finmarchicus was negatively selected for here. Fish from the Irminger, Labrador and Norwegian Seas displayed a high number of empty stomachs while no fish stomachs were found empty in the Iceland Sea. Contrary to expectation due to being located at the highest latitude, Benthosema in the Iceland Sea had significantly higher condition factor (for both small and large fish) and liver indices compared to fish from other basins, but the abundance of small fish in the sampled population here was very low. This contrasts with the “light environment exclusion” (LEE) hypothesis, which propose that the extreme light environment at higher latitudes restricts feeding opportunities for mesopelagic fish at high latitudes. It is suggested that improved classification of prey through stomach analyses should aim to allow bioluminescent organisms to be separated from non-bioluminescent prey if feasible. This would allow improved resolution to analyse stomach contents and certainly progress our understanding of the success of myctophids across variable habitats.
1 Introduction
The glacier lantern fish (Benthosema glaciale, Reinhardt 1837) is widely distributed in the deep-water of the North Atlantic (Gjøsæter and Kawaguchi, 1980; Sameoto, 1988, 1989; Hudson et al., 2014; Klevjer et al., 2020a), the Mediterranean Sea (Goodyear et al., 1972; Contreras et al., 2015), and in fjords of western Norway (Gjøsæter, 1973a; Gjøsæter, 1973b; Giske et al., 1990; Suneetha and Salvanes, 2001; Kristoffersen and Salvanes, 2009). B. glaciale is the most common species of the family Myctophidae in the Atlantic Ocean north of 35°N (Halliday, 1970; Gjøsæter, 1981; Albikovskaya, 1988), and it is abundant from the Davis Strait to Cape Hatteras in the west, and from north of Svalbard to Cape Verde in the South (cf. Albikovskaya, 1988; Sameoto, 1989; Knutsen et al., 2017). In the North Atlantic this species might considerably contribute to the biomass in pelagic trawl catches, especially when sampling includes mesopelagic layers (Themelis and Halliday, 2012; Pepin, 2013; Halliday et al., 2015).
Thus, B. glaciale represent a significant component of the mesopelagic fish fauna across the North Atlantic, and it is possibly one of the most studied mesopelagic fishes. The majority of studies however have limited spatial extents and there are few direct studies on gradients in basic biology and ecology over larger areas (Caiger et al., 2021). Comparative studies across larger areas rely mainly on comparing standardized acoustic backscatter (cf. Klevjer et al., 2016; Klevjer et al., 2020a; Klevjer et al., 2020b), synthesizing published data (e.g., Caiger et al., 2021) or the analyses of diversity and patterns of mesopelagic species distributions (e.g., Olivar et al., 2017; García-Seoane et al., 2021).
As several other mesopelagic fish, B. glaciale performs Diel Vertical Migrations (DVMs) living at mesopelagic depths during daytime and ascending to the surface water at night to feed (Robinson et al., 2010; Dypvik et al., 2012b). B. glaciale often feed more intensively in the late evening or at night, but this species is capable to feed both day and night (cf. Gjøsæter, 1973b; Kinzer, 1977; Sameoto, 1988, 1989; Bagøien et al., 2001; Pepin, 2013). Both Gjøsæter (1973b) and Bagøien et al. (2001) comment on Benthosema feeding, they respectively state that “ … B. glaciale feeds most intensively during the evening, but the diurnal variation is probably not very great” and “Benthosema had little well-digested food in their stomachs at all times of the day, and there was no diel pattern in stomach fullness or degree of digestion”. This suggests that Benthosema daytime feeding do occur in west-Norwegian fjords, similarly so-called inverse vertical migration occurs here, where Benthosema migrate towards the shallower part of the mesopelagic to daytime feed during winter (Dypvik et al., 2012a; Dypvik et al., 2012b).
The Euro-BASIN initiative in 2013 was a cross-Atlantic survey that utilized standardized sampling of plankton and fish species, allowing a comparative study of environmental influences on mesopelagic communities and their dynamics in four major basins; the Norwegian Sea (Nor), the Iceland Sea (Ice), the Irminger Sea (Irm), and the Labrador Sea (Lab) (Drinkwater et al., 2020; Melle et al., 2020; Strand et al., 2020; Klevjer et al., 2020a; Klevjer et al., 2020b). B. glaciale dominated the fish catches in the Norwegian Sea and Iceland Sea, but other species were more important in Irminger and Labrador Seas (Klevjer et al., 2020a). Several studies suggest that B. glaciale in basins covered by these investigations have quite extensive vertical distribution. In the slopes of the Nova Scotia shelf region Benthosema glaciale have been registered in low concentration to 950 m depth (Sameoto, 1988) in the period April till July. In the Norwegian Sea we have extremely few registrations of B. glaciale from MOCNESS stratified tows below 600 m depth (cf. IMR, database), suggesting that the very cold intermediate and deep-waters of this region (Blindheim and Østerhus, 2005), sets some limitations to the vertical depth range of the species here.
While the areas covered by the cruise are frequently implicitly viewed as one unit, the “North Atlantic”, the Atlantic crossing highlighted that gradients are present, both in hydrography (Drinkwater et al., 2020) and biology (Melle et al., 2020; Naustvoll et al., 2020; Strand et al., 2020; Klevjer et al., 2020a). The Norwegian and Iceland Sea basins are colder and fresher than the Irminger and Labrador basins, but all basins are influenced by advection of North Atlantic water from the south and by differing degrees of Arctic waters from the North (Drinkwater et al., 2020). The Norwegian Sea and Iceland Sea also have a different light environment due to their higher latitudes than the Irminger and Labrador Seas, and the crossing showed differences in daytime depths of mesopelagic organisms across the 4 basins (Klevjer et al., 2020b).
Particularly relevant in the present context is the finding that the DVM of the mesopelagic acoustic scattering layer became gradually more restricted from around 63°N in the Norwegian Sea to 67°N in the Iceland Sea (Norheim et al., 2016). At 67°N, the DVM was halted at ~300 m depth at night likely due to a night-time light that at this time (May) was 2-3 orders of magnitude higher than at 63°N. Further studies highlighted large differences in phenology and phytoplankton composition across the areas at the time of crossing (Naustvoll et al., 2020), for zooplankton large differences in composition, abundances, phenological state and vertical distributions were also evident (Strand et al., 2020). For the macroplankton and micronekton components differences were large enough (Klevjer et al., 2020a) that a summary paper suggested differently structured pelagic ecosystems in the eastern and western parts (Melle et al., 2020).
The aim of the present paper is primarily to derive a broader understanding of the feeding ecology of the important mesopelagic species B. glaciale across four distinctly different ocean basins of the North Atlantic beyond the British Isles. We provide a basin-wide comparative analysis of size dependent diet, feeding selectivity and energetic status of B. glaciale in relation to prey availability, gross environmental conditions including the ambient light environment.
2 Materials and methods
2.1 Areas of investigation
Benthosema glaciale were collected along with physical data, zooplankton and echosounder data between 1 May and 14 June 2013 onboard the research vessel RV G.O. Sars during the Euro-BASIN expedition, comprising the westward leg and the return crossings of the North Atlantic Ocean basins between Bergen, western Norway and Nuuk, western Greenland (cf. Drinkwater et al., 2020; Naustvoll et al., 2020; Strand et al., 2020; Klevjer et al., 2020a; Klevjer et al., 2020b) (Figure 1). The deep basins covered during the expedition were the Norwegian Sea (Nor), Iceland Sea (Ice), Irminger Sea (Irm) and Labrador Sea (Lab).
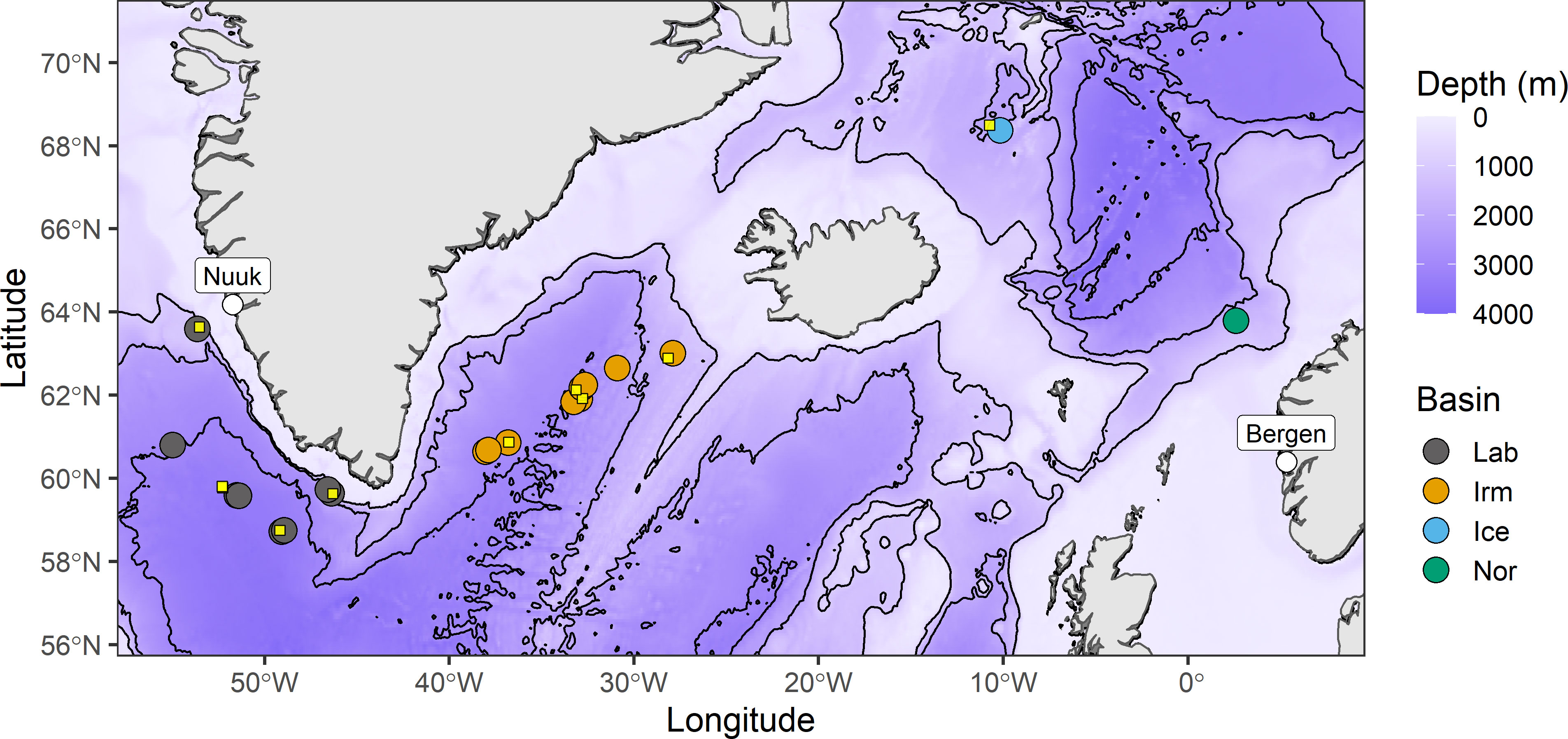
Figure 1 Map of the surveyed area from the Euro-BASIN expedition in May-June 2013. Coloured circles denote trawl stations from which fish stomachs were collected and processed. Small yellow squares show the position of the MOCNESS sampling stations that subsequently were used to calculate Ivlev’s electivity indices for Benthosema glaciale prey items (see section 2.7).
2.2 Zooplankton samples
Mesozooplankton from each basin was sampled with a MOCNESS (Multiple Opening/Closing Net and Environmental Sensing System; Wiebe et al., 1985) towed obliquely (1000-0 m), acquiring depth stratified samples from eight pre-determined vertical strata: 1000 - 800 m, 800 – 600 m, 600 – 400 m, 400 – 200 m, 200 – 100 m, 100 – 50 m, 50 – 25 m, and 25 – 0m (Strand et al., 2020). The MOCNESS has a 1m2 opening at a towing angle of 45° and was equipped with 9 nets having a mesh size of 180 µm including cod-end buckets with identically meshed filtration gauze. On board the research vessel all samples were fixed in 4% buffered formalin. In the laboratory on land, zooplankton were enumerated and identified to the lowest taxonomic level possible using a stereo microscope, in accordance with the IMR standard taxonomic processing scheme (Hassel et al., 2017). Individuals in all species and groups were staged where possible and counted. Strand et al. (2020) have given a detailed account of the mesozooplankton ecology across the four basins during the same expedition, and also provide further details of gear and methods applied.
2.3 Trawl sampling
Samples of B. glaciale for stomach content analysis were collected from three Harstad trawl hauls and 16 Macroplankton trawl hauls. The Macroplankton trawl has a nominal 6x6 m trawl opening and mesh size of 3 mm square light opening (8 mm stretched, knot to knot) from the trawl mouth along the entire trawl length to the cod-end (Hassel et al., 2017; Klevjer et al., 2020a). The trawl was operated like a single net oblique tow (cf. Wiebe et al., 2015), from the surface to the desired depth and back up (V-haul), while the research vessel moved constantly forward at a speed of about 2 knots (Mjanger et al., 2019). Trawl hauls that were not V-hauls were aimed trawling on acoustic registrations. Due to harsh weather conditions when the expedition was in the Norwegian and Iceland Seas, B. glaciale stomach contents were sampled from only one trawl haul in each of these areas, while it was collected from 8 and 9 trawl hauls in the Labrador and Irminger Seas, respectively (Table 1).
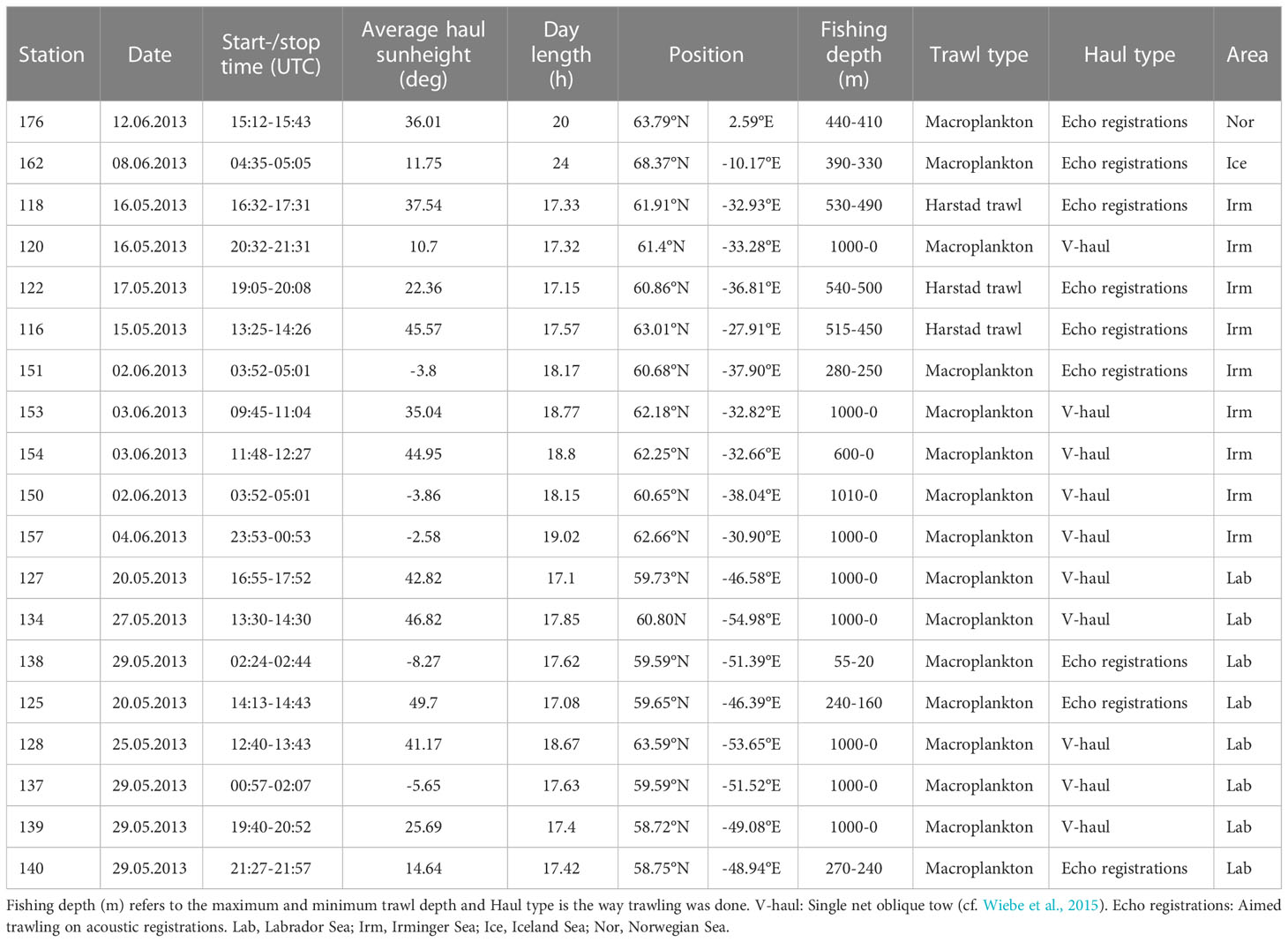
Table 1 Overview of trawl stations for the collection of B. glaciale stomach data as well as date and time of day (UTC) the trawling took place.
Since the Harstad trawl is a graded trawl, catches from this trawl should not be considered quantitative for mesopelagic fish, and size distributions from this trawl are also biased. To assess in-situ biomass levels and size distributions we therefore use data from the nongraded Macroplankton trawl only (cf. Klevjer et al., 2020a). Abundances and biomasses for B. glaciale are only based on data collected in oblique hauls 0-1000 m with the Macroplankton trawl. To obtain better estimates for all areas we have therefore included data also from trawls not listed in Table 1 (cf. Klevjer et al., 2020a). For quantitative hauls volumes of water filtered were calculated as the product of trawled distance (including vertical excursions), and trawl opening area. Surface integrated abundances per length group were calculated from counts of measured individuals scaled up by subsample sizes, and mass densities were estimated based on the empirical length- weight regression (see below).
Most trawl hauls for B. glaciale stomach contents were conducted during “daytime” (Table 1), with average sun height during the haul above the horizon. In the Irminger Sea three of a total of nine hauls were conducted during night-time (2 and 4 May) where the sun was <-2.5° below the horizon. Of the eight hauls in the Labrador Sea, two on 29 May were night hauls, and in both cases the sun was <-5° below the horizon. The haul in the Norwegian Sea was conducted early afternoon on 12 June, while the most northern haul in the Icelandic Sea at 68.37°N was conducted during midnight sun conditions and early morning hours when the sun was 11.75° above the horizon. For an in depth analysis of DVM in relation to irradiance and water mass absorption properties in the study areas, we refer to Klevjer et al. (2020b).
2.4 Fish preservation and processing
As part of the standard work up of the trawl samples, length measurements of all fish including B. glaciale were conducted, including a total weight of these same specimens. However, occasionally samples had such a high number of fish that sub-sampling was necessary. In addition, a randomly selected subsample of the total trawl catch including B. glaciale, was preserved in 96% ethanol and stored until further processing in the laboratory. A total of 230 B. glaciale individuals from the preserved samples were processed at the zooplankton laboratory, Institute of Marine Research (IMR) (Table 2). For each of the basins, fish were randomly selected from the preserved samples until 30 individuals with non-empty stomachs had been processed from each ocean region. The individuals that were processed from each trawl station, were selected based on an Excel random number generator. Based on the length distribution of all fish captured, we define fish larger and equal to 50 mm as “large”, while fish smaller than 50 mm is called “small”. This is not an entirely arbitrary choice but is supported by findings for instance at the Flemish Cap where fish length at 50% maturity (L50) was 47-49 mm SL (García-Seoane et al., 2014) giving a rough separation between immature and mature individuals.

Table 2 Overview of number Benthosema glaciale stomachs analyzed per basin, number of empty stomachs, the standard length (SL) and weight ranges (minimum and maximum) in millimeter (mm) and gram (g) respectively.
Individual fish were measured for standard length (SL, mm) from the fish’s snout to the bone knot in the caudal peduncle and for total length (TL, mm) from the fish’s snout to the end of the caudal fin (Figure 2). The fish that had been preserved was dried on paper towels so that excess alcohol could be removed and then weighed to the nearest 0.1 mg using a digital scale, either the A&D GR-200 or the Mettler Toledo xs205 Dual Range balance. Fish were sexed by examining the luminous organ on the caudal peduncle. Males have a dorsal supracaudal light organ while females have 1-2 ventral infracaudal organs. If these were blurred or damaged, the fish were sexed by inspecting the gonads. Liver and gonads were then taken out of the abdomen of the ethanol preserved fish and each were weighed to the nearest 0.1 mg before they were put in pre-weighed aluminium dishes and dried in a drying oven at 60 degrees for 24 hours until constant weight. To obtain a proxy for somatic weight, the fish was weighed also when liver and gonads had been dissected out.
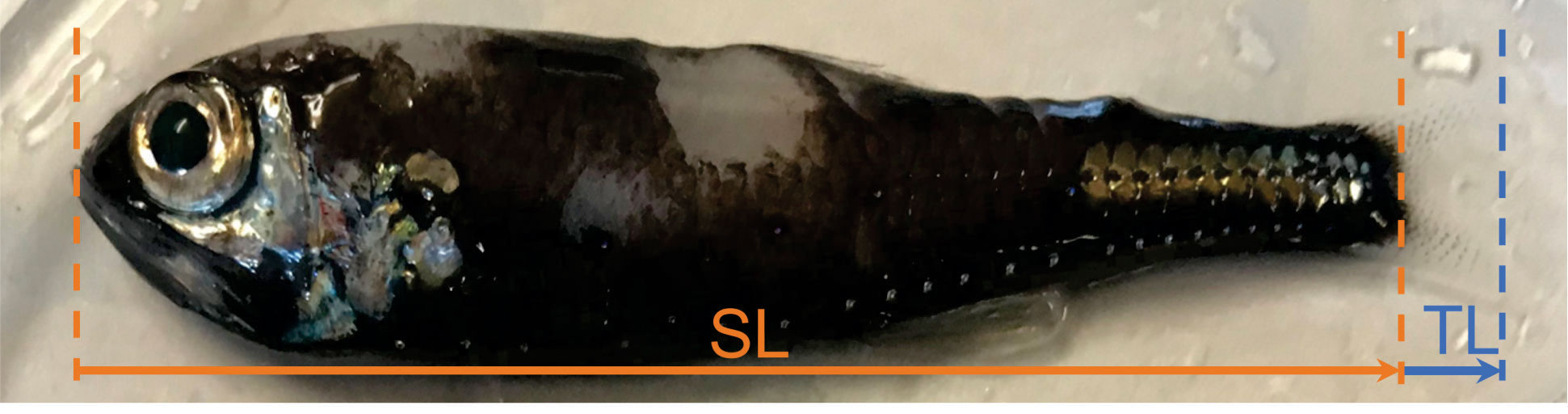
Figure 2 Glacier lantern fish (Benthosema glaciale). Standard length (SL) is measured from the tip of the snout to the tail root (orange arrow). If total length (TL) is measured the caudal fin (blue arrow) is included. Photo: Espen Strand.
2.5 Adjustments of ethanol preserved samples and determining relative condition factors
Long-term ethanol preservation of B. glaciale generates over 39.4% weight loss and length shrinkage of ~2.3% (Kristoffersen and Salvanes, 1998). The fresh wet weight (g) for each individual (Wf) was converted from ethanol preserved weight (Wp) following Eq. 1 (Kristoffersen and Salvanes, 1998).
The fresh standard length (SLf) was converted from ethanol preserved standard length of glacier lantern fish (SLp) following Eq. 2 (Kristoffersen and Salvanes, 1998):
The fresh weights and lengths are used in the data analyses. A function of weight (Wf) and length (SLf) relationship for B. glaciale was based on all fish processed for stomach content, liver and gonads (N = 230) from all basins pooled and was obtained using the nls (nonlinear least-squares) function in R (R Core Team, 2021). The predicted overall weight-length relationship is given by the equation: Wreg (g) = 3.391x10-6 × SL(mm)3.287.
From this the relative condition factor (Kn) was calculated following Le Cren (1951) as the ratio of fresh fish weight (Wf) divided by the estimated weight (Wreg) from the fitted regression equation for an individual’s fresh length (SLf). Kn is also considered a simple proxy for the energy status of an individual fish.
The equations above were also used when converting liver and gonad weights to their original fresh weights, LWf and GWf respectively. Gonad and liver indicators were also computed from the Gonad Weight (GWf) and Liver Weight (LWf), often respectively named the gonadosomatic and hepatosomatic indices, GSI = GWf/Wf and HSI = LWf/Wf.
2.6 Stomach content analyses
The fish stomachs were extracted from the anterior end of the oesophagus to the pyloric cecum (pylorus) and then examined using a Leika MZ775 microscope at 6.3-50x magnification. The stomachs were first weighed to the nearest 0.1 mg, opened and the contents were carefully separated from the stomach inside epithelium. Prey were counted and determined to species or the nearest taxonomic group and prey groups with different digestive levels were established, when possible, but later pooled. The remains of a species or group that could not be counted, was weighed separately. Residues of stomach contents that could not be separated into groups were determined to the highest possible taxonomic level and put in pre-weighed aluminium trays (“tare weight”). For each prey group of equal digestion dry weight was determined to the nearest 0.1 mg after drying at 60° C for a minimum of 24 hours to constant weight. Total length of amphipods (Themisto sp.), shrimps (Sergestes sp.) and krill (Euphausiacea) were measured to the nearest mm below. For the ostracods (Ostracoda) the total length of the animal’s shell was measured to the nearest mm below. Arrow worms (Chaetognatha) was rarely found in the fish stomachs. If so, only remnants of the spiny jaws or bristles could be identified. Hence any length and weight measurements are not representative for this prey group. Only prey localized from the anterior end of the oesophagus and fish stomach were included in the present analyses while prey localized in the oral cavity and throat were excluded. Lastly, the empty stomachs were weighed after content removal as a control where a full stomach minus prey contents should equal the empty stomach.
2.7 Diet and prey selection
When comparing diet of B. glaciale between basins separate calculations for small: < 50 mm (n = 134) and large B. glaciale ≥ 50 mm (n = 96), were performed by comparing prey categories expressed as percentage of body weight. The only exception is the calculation of Ivlev’s electivity index when the numbers of prey items are used. To examine if B. glaciale selects some prey over others in the water column in each of the basins, a modified version of Ivlev’s electivity index Di (see Ivlev, 1961; Jacobs, 1974) is used. The index is interpreted to reflect positive selection for a prey i when 0< Di <1 and negative selection -1 < Di < 0. The following equation as modified by Jacobs (1974) is used:
where ri is the proportion of prey i found in the predator’s stomach (i.e. number of prey in one category divided by total prey items) and pi = the proportion of prey available in the same area as the fish were caught. This proportion is based on the measured density (individuals m-3) of the same prey categories from the MOCNESS samples.
Based on knowledge of the vertical distribution and feeding of B. glaciale we assumed that feeding occurred irrespective of depth and time of day, and acoustic data from the 38 kHz EK60 echosounder were used to decide on which of the zooplankton samples (depth strata, cf. Table 3) from the MOCNESS hauls that overlapped with the acoustic registrations. This approach allowed us to eliminate zooplankton data from depth ranges with low abundance of B. glaciale. The depth range of residence for B. glaciale was estimated to be the depth range comprising 90% of the total diffuse backscatter (total backscatter minus backscatter from schooling components, see Klevjer et al., 2020a; Klevjer et al., 2020b) as measured from the Simrad 38 kHz frequency transducer. This assumes that B. glaciale is the dominant component of the total backscatter. For more detailed information about acoustic setup and processing, see Klevjer et al., 2020a; Klevjer et al., 2020b.
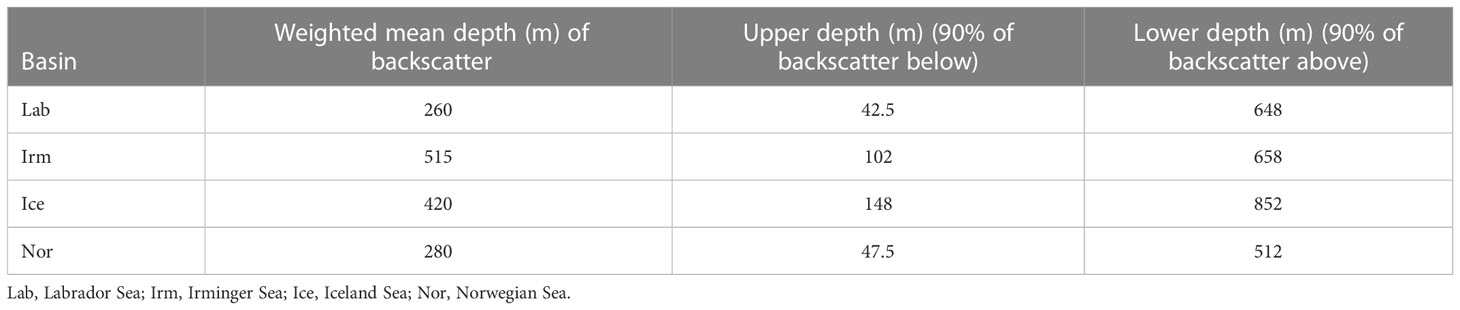
Table 3 Weighted Mean Depth (WMD) and estimated depth ranges of B. glaciale in the different basins based on vertical extension of 80% of total 38 kHz backscatter for non-schooling components.
To ensure a reasonable spatial and temporal overlap between fish analysed from trawl catches and MOCNESS samples, only MOCNESS samples from stations that were taken less than 30 nautical miles and less than 2 days prior or after trawls were included in the data analysis. Furthermore, only MOCNESS data from depths where the acoustic registration where observed (Table 3) were used. This meant that zooplankton data from the layers 1000-100 m in the Iceland Sea, 800-100 m in the Irminger Sea and 800-25 m in the Labrador Sea were included. Given that MOCNESS taxonomic analyses are performed on individuals of much better quality (no digestion), all recorded species/group in the zooplankton material were grouped the same way as for the prey categories found in the stomachs of B. glaciale. Thus, the stomach group of e.g. Copepoda will for the similar MOCNESS group contain the sum of numerous species but not species defined in other existing stomach prey groups (e.g. C. finmarchicus) even though it is possible that an item classified as “Copepoda” in the stomach samples in fact were C. finmarchicus. Species/groups that were found in the MOCNESS samples but never recorded in the stomachs were excluded.
Analysed stomachs from trawl catches without a corresponding MOCNESS sample were discarded for the prey electivity analysis. This meant that no data from the Norwegian Sea was available for the analysis since trawl and MOCNESS data were too far apart to be compared. All analysed stomachs, not taking fish length into consideration, corresponding to each MOCNESS sample were pooled in terms of prey items for each prey group. This numerical prey distribution was then used to calculate the Ivlev electivity index by comparing the same prey group densities found in the MOCNESS. In basins where more than one MOCNESS dataset was used, the presented Ivlev index is the mean of each set of calculated indices.
The diet composition of B. glaciale was investigated with multivariate analysis using the PRIMER software v7 and the PERMANOVA package (Anderson et al., 2008; Clarke and Gorley, 2015). Individuals with empty stomachs or with only digested material were not included in these analyses. Prey data (expressed as the ratio between prey weight and predator weight) were square root transformed before the analysis to down-weight the important of the dominant species (Clarke et al., 2014). Bray-Curtis similarities were calculated for each pair of predator to produce a similarity matrix, with prey items as variables (columns) and predators as samples (rows). Unidentified prey and rare prey (i.e., only one or two stomachs among all the stomachs analyzed) were not included in the matrix. In addition, these data were further analyzed with a non-metric Multidimensional Scaling (nMDS) to clarify diet differences. A two-dimensional ordination approach was adopted because the stress level (≤ 0.1) was acceptably low, with no real prospect of a misleading interpretation (Clarke et al., 2014). In addition, to confirm the patterns observed, differences among basins and between small and large individuals (< 50 mm and ≥ 50 mm SL, respectively) were tested with PERMANOVA (with 9999 permutations) upon a Bray-Curtis similarity matrix with prey. When differences were found, pair-wise PERMANOVA tests were accomplished. The PERMANOVA assumes homogeneity of the multivariate dispersion (Anderson et al., 2008). Thus, a PERMDISP test (with 9999 permutations) was applied to test for homogeneity of the multivariate dispersion. The majority of data analysis and visualisation was done using the R library «tidyverse» (Wickham et al., 2019).
3 Results
3.1 Size composition and abundance
The data from all processed 230 fishes, females and males, from all basins were merged for the estimation of the weight-length relationship. Since the condition factor were similar for males and females (127 females, 101 males, 2 indeterminate) with no significant relationships (unpaired t-test; p=0.313), the weight-length relationship combines data from males and females (cf. Figure 3).
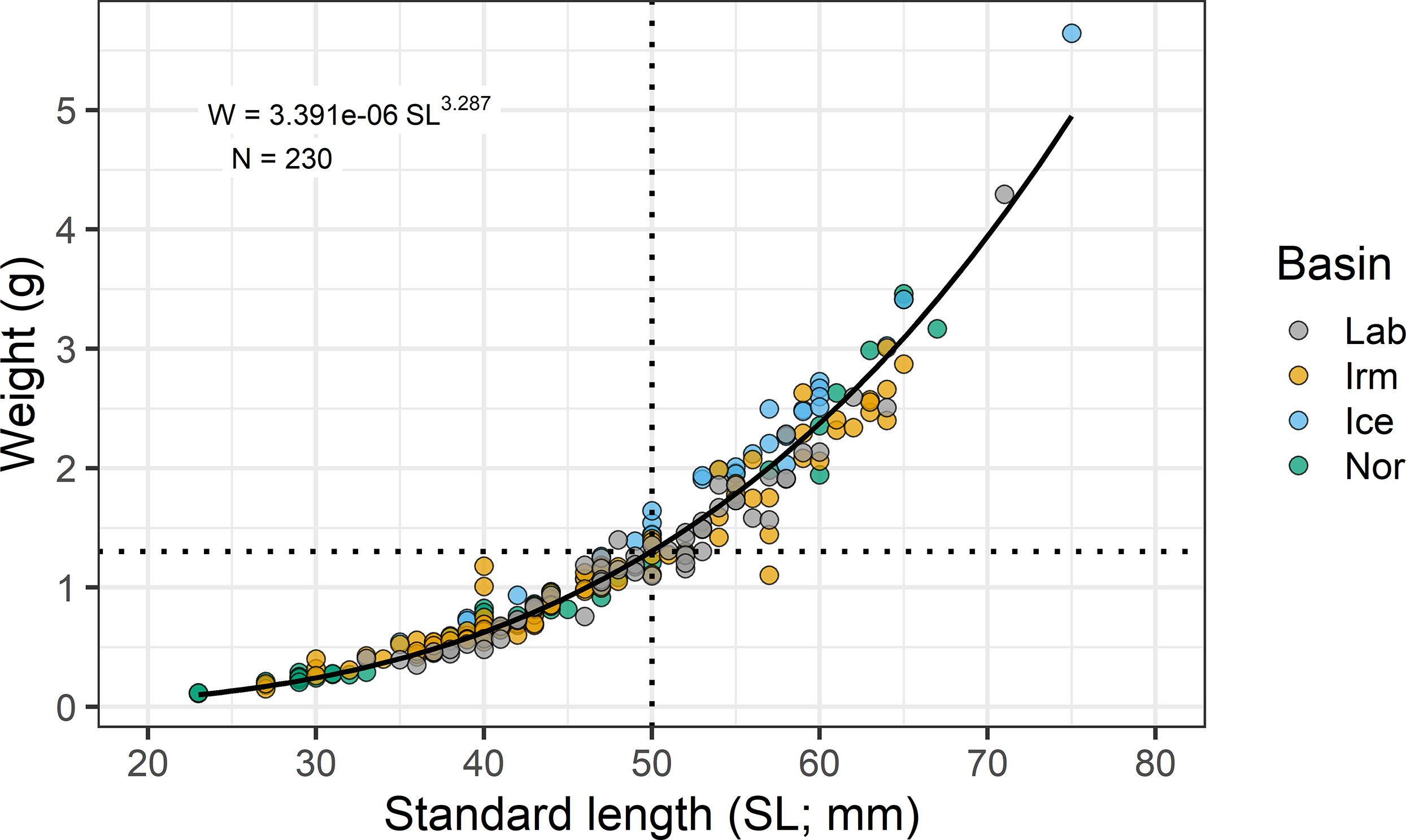
Figure 3 Weight-length relationship for B glaciale. Data from all fish processed for stomach content, liver and gonads (N = 230) from all basins are pooled.
The B. glaciale individuals caught in the Norwegian Sea basin were smaller than those in the other basins with most individuals smaller than 40 mm, while the individuals in the Iceland Sea were larger than 40 mm (Figure 4A). The populations from the Irminger and Labrador Seas had similar size composition with most individuals between 30 and 50 mm length (Figure 4A). Both the measured abundance (number of individuals) and biomass (weight of individuals) of B. glaciale were highest in the Labrador and Irminger Sea basins (Figure 4B). The density of B. glaciale was lowest in the Iceland Sea basin (Figure 4B), but the biomass was lowest in the Norwegian Sea basin, because the samples from the Iceland Sea basin consisted of much larger individuals, with > 50% of the fish being 60 mm or longer (Figure 4C).
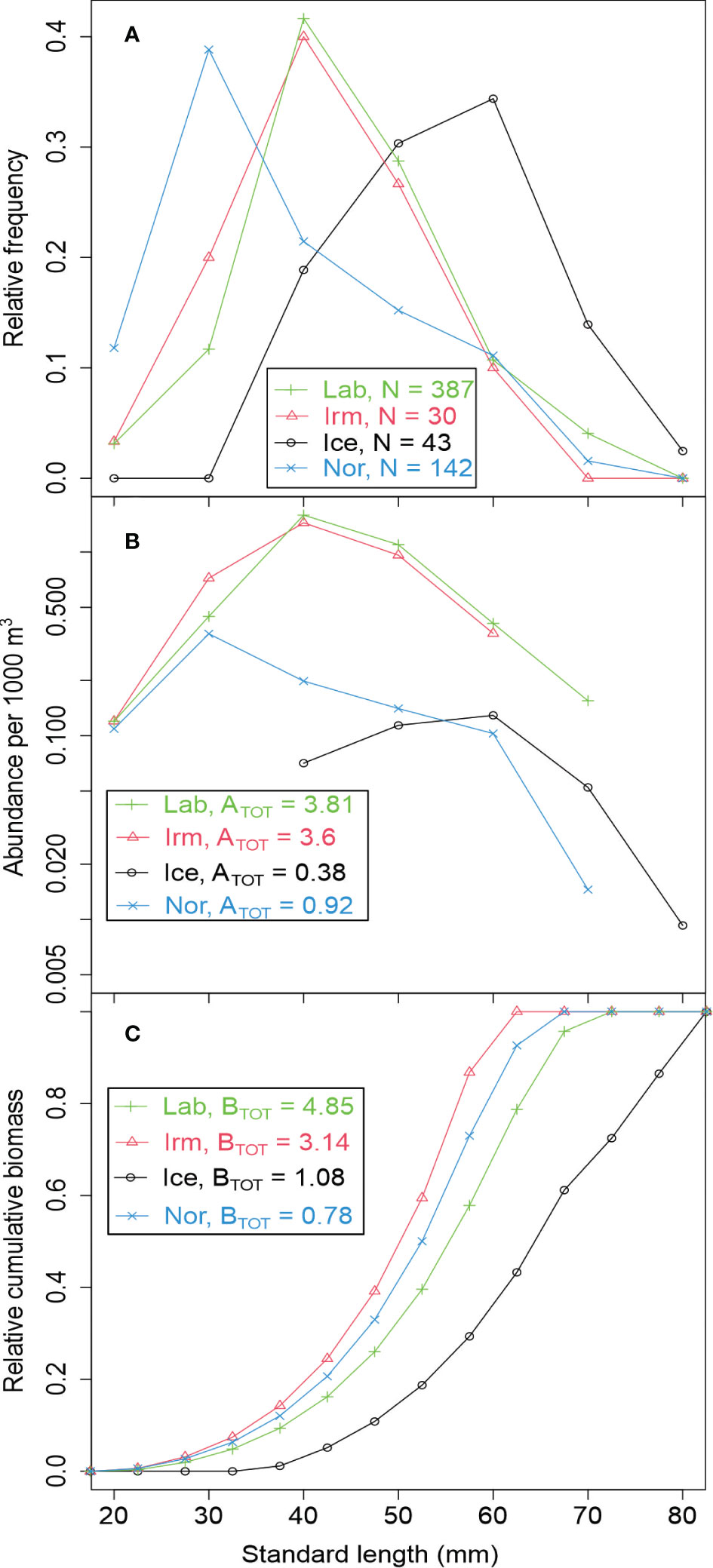
Figure 4 (A) Relative length distributions of B glaciale for each basin by length groups. N is the number fish measured for each basin. (B) Abundance in number of fish by length group per 1000 m3 for each basin. ATOT is summed abundance of B glaciale per 1000 m3 (all length groups). (C) Cumulative biomass versus standard length of B glaciale. BTOT is average biomass (g WW) per 1000 m3. Based on Macroplankton trawl catches (~1000-0 m) from the BASIN cruise (see Klevjer et al. (2020a) close to stations with trawls sampled for stomach contents (Table 1). Minor differences in total biomass levels compared to Klevjer et al. (2020a) is expected due to slightly different subselection of trawls.
3.2 Condition factor, gonad, and liver weights
The energy status measured as the relative condition factor of B. glaciale differed between the basins with fish from the Iceland Sea basin having significantly higher condition factor than all other basins for both small and large B. glaciale (Figure 5). Larger fish (SL ≥ 50 mm) in the Labrador and Irminger basins had median condition values below 1.0 indicating a poorer condition compared to those in the Norwegian and Iceland Seas.
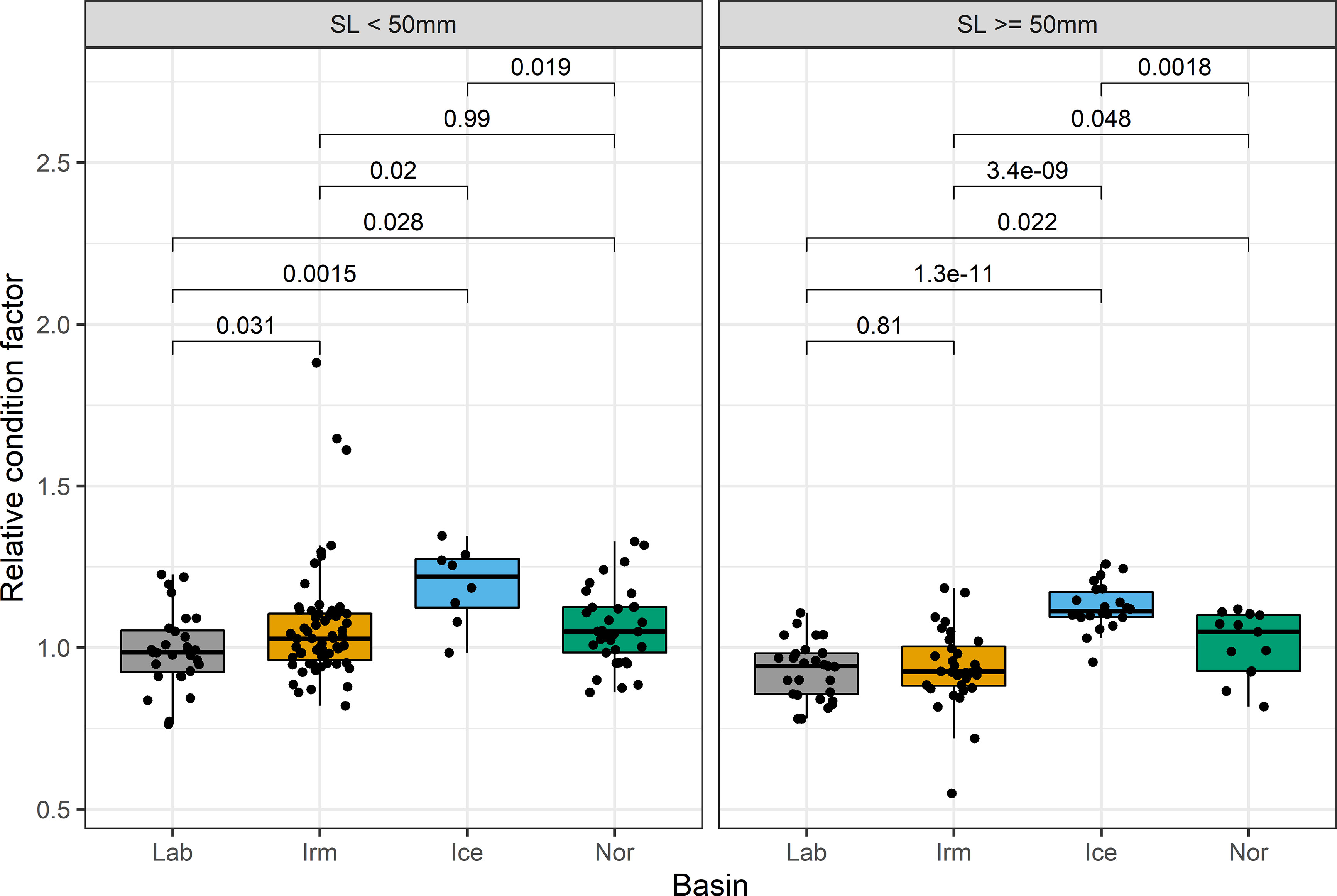
Figure 5 Relative condition factor (Weight/expected weight given length) for B glaciale from the Labrador Sea (Lab), Irminger Sea (Irm), Icelandic Sea (Ice) and Norwegian Sea (Nor). Numbers refer to p-value of t-test (two-sided unpaired) between groups. Boxplot show median (vertical line) 25 and 75% quartiles (hinges) and whiskers (~95% confidence interval). All data points are shown as dots.
Males had lower gonad weights compared to females with median weights below 0.5% of body weight in all basins and for both length groups (Figure 6). When measuring energy status using the liver indicator, B. glaciale from the Iceland Sea basin had significantly higher values compared to the other 3 basins (t-test; Lab: p < 0.001, Irm: p = 0.015, Nor: p = 0.023; Figure 6) with both length groups pooled. Not enough data were available to run these tests also considering length groups, but the input data were organ weight/total weight and size is thus implicitly considered.
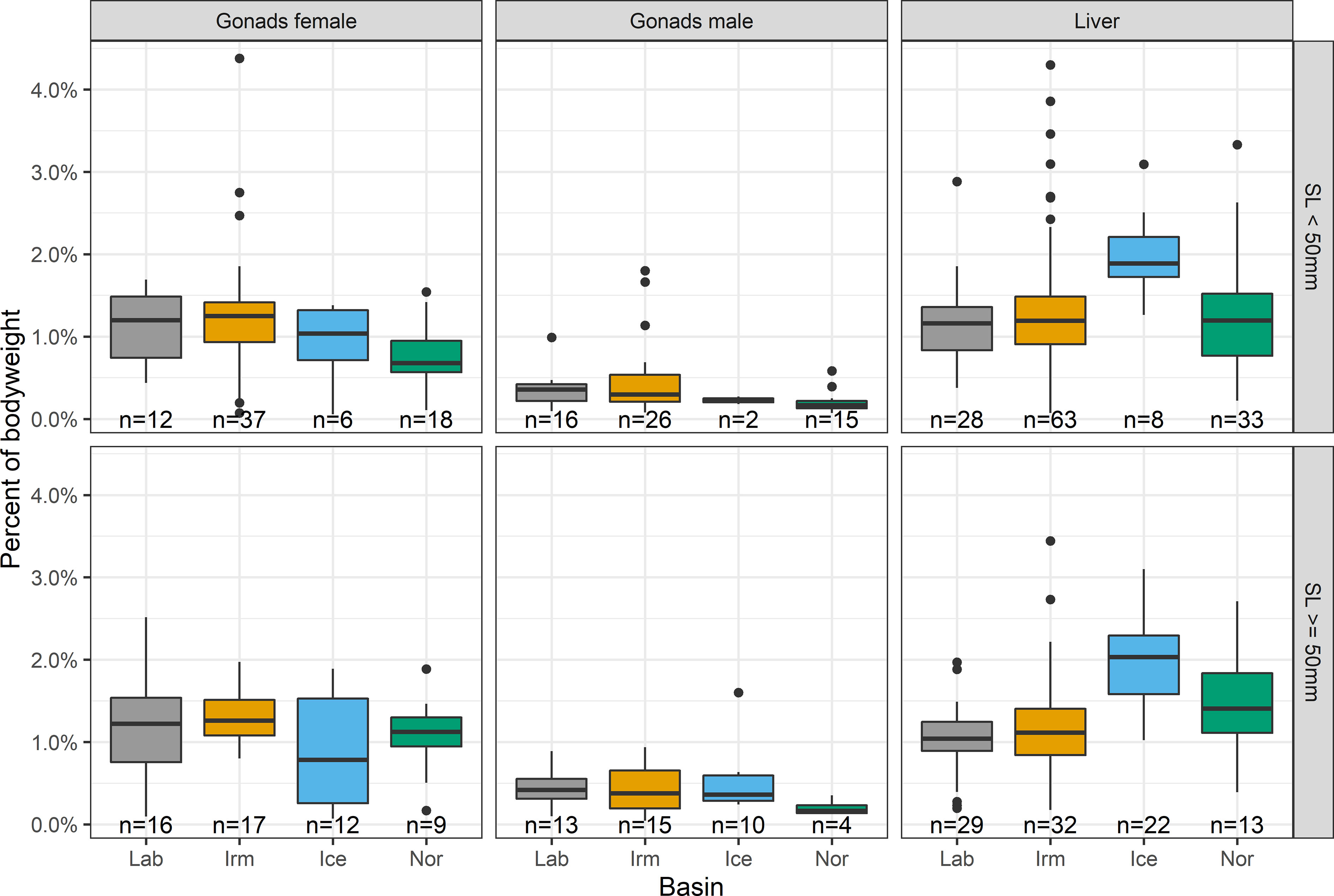
Figure 6 Liver and gonad weight indicators (females and males) as percentage of body weight, in the four basins for large (SL≥ 50 mm) and small (SL< 50 mm) Benthosema glaciale. Basins are shown along the x-axis: Labrador Sea (Lab), Irminger Sea (Irm), Iceland Sea (Ice) and Norwegian Sea (Nor). Boxplot show median (vertical line) 25 and 75% quartile (hinges) and whiskers (~95% confidence interval). Data falling outside the whiskers are shown as dots. n refers to the number of fish gonads and livers that could be weighed in each group.
The results suggested that there were slightly lower investment in the female gonads of fish from the Iceland Sea basin, particularly for the larger individuals (≥ 50 mm), since median gonad weight relative to body weight was the lowest for this group of females.
3.3 Diet
There were large differences in stomach contents (Figure 7). Most of the individuals in the Irminger Sea had empty stomachs, both for the large (SL ≥ 50 mm) fish (> 60%) and for the small fish (SL < 50 mm) (~ 75%). In fish from the Labrador and Norwegian Sea basins 30-50% were empty for both length groups. Large individuals in the Labrador Sea (SL ≥ 50 mm) had the lowest maximum stomach fullness recorded, with the maximum stomach weight registered being only ~0.5% of body weight (Figure 7). In contrast, no individuals from the Iceland Sea had empty stomachs, and B. glaciale from that basin had the highest maximum stomach weight registered in any fish, with stomach contents measuring > 4.5% of the body weight (Figure 7). For all basins, overall small fish show a higher maximum stomach fullness than larger fish.
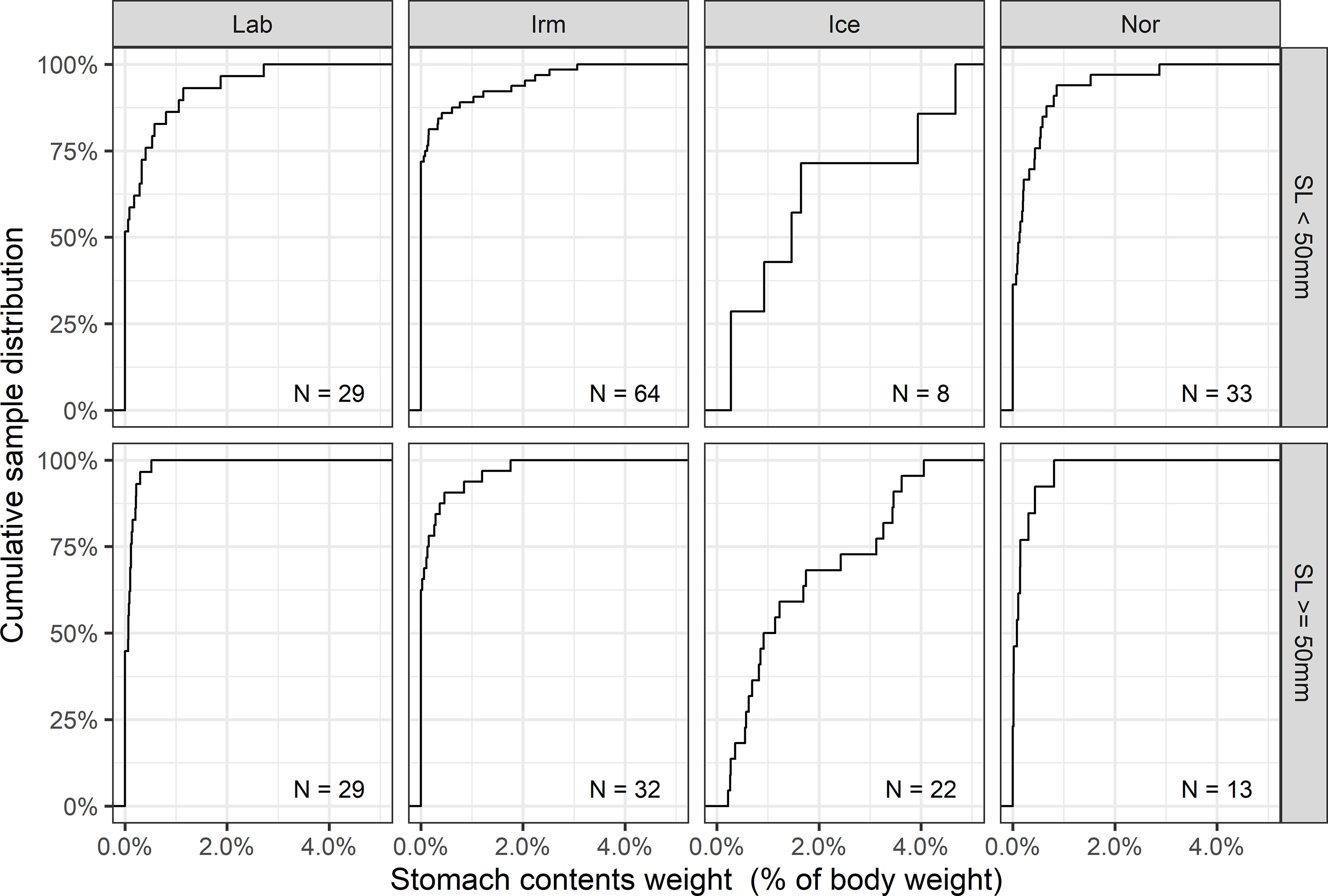
Figure 7 Cumulative distribution of prey weight in the stomachs relative to body weight. Numbers denote sampled individuals in each group.
C. hyperboreus was the dominating prey item in terms of biomass for both size groups of B. glaciale individuals from the Iceland Sea basin (Figure 8A) and made up between 50-75% of the identifiable prey (Figure 8B). Amphipods were common prey items in all basins and this group was especially important for larger B. glaciale individuals. For smaller B. glaciale amphipods were much less important with the exception individuals from the Norwegian Sea. Paraeuchaeta sp. was also a common prey group in all basins and length groups, except for large individuals from the Norwegian Sea. Note however, that around 50% of the stomach contents in large fish from the Norwegian Sea could not be identified, and a large fraction of prey items could only be classified to the Copepoda level due to the level of digestion. Thus, if Paraeuchaeta sp. was present, it might have been part of the Copepoda group, although their large tail (caudal rami) is quite robust and could have been possible to identify even in heavily digested stomach content. Euphausiids were present in fish of all lengths in all basins, except in individuals from the Labrador Sea. Appendicularia were part of the B. glaciale diet only in the Labrador basin. It is noted that most of the appendicularians were registered based on the presence of their ‘house’ in the Benthosema stomachs, while the animal itself is hard to find, presumably because it is small and easily digestible.
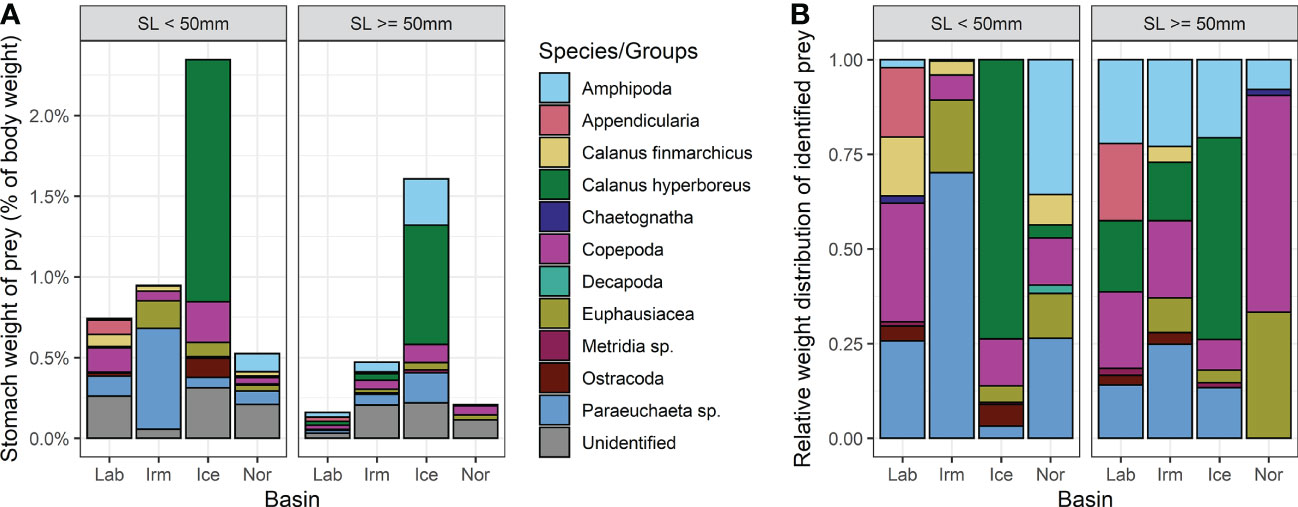
Figure 8 (A) Average stomach weight of prey expressed as percent of body weight. (B) Relative average weight of identified prey items found in the examined stomachs (i.e. unidentified prey not shown). All empty stomachs are excluded. Legend applies to both panels.
There were statistically significant differences in diet among basins (PERMANOVA, p<0.001), but not between length groups (PERMANOVA, p= 0.259) or in the interaction between basin and length groups (PERMANOVA, p= 0.0541). All the basins have different diets (PERMANOVA, pairwise-test, p<0.001), except Irm and Nor (PERMANOVA, pairwise-test, p= 0.0743). In addition, the PERMDISP test showed homogeneity of dispersions for both basin and length groups (PERMDISP, p= 0.1694 and PERMDISP, p= 0.4491, respectively), confirming the PERMANOVA results. When samples were ordinated in MDS plots (Figure 9), most of the samples from the Iceland Sea (Ice) were grouped in the upper left-central part of the plot whereas the samples from the Labrador Sea (Lab) tended to be grouped in the upper right-central part of the plot. Stomach samples from Norwegian and Irminger Seas were mainly located in the lower half of the plot without a clear separation between them.
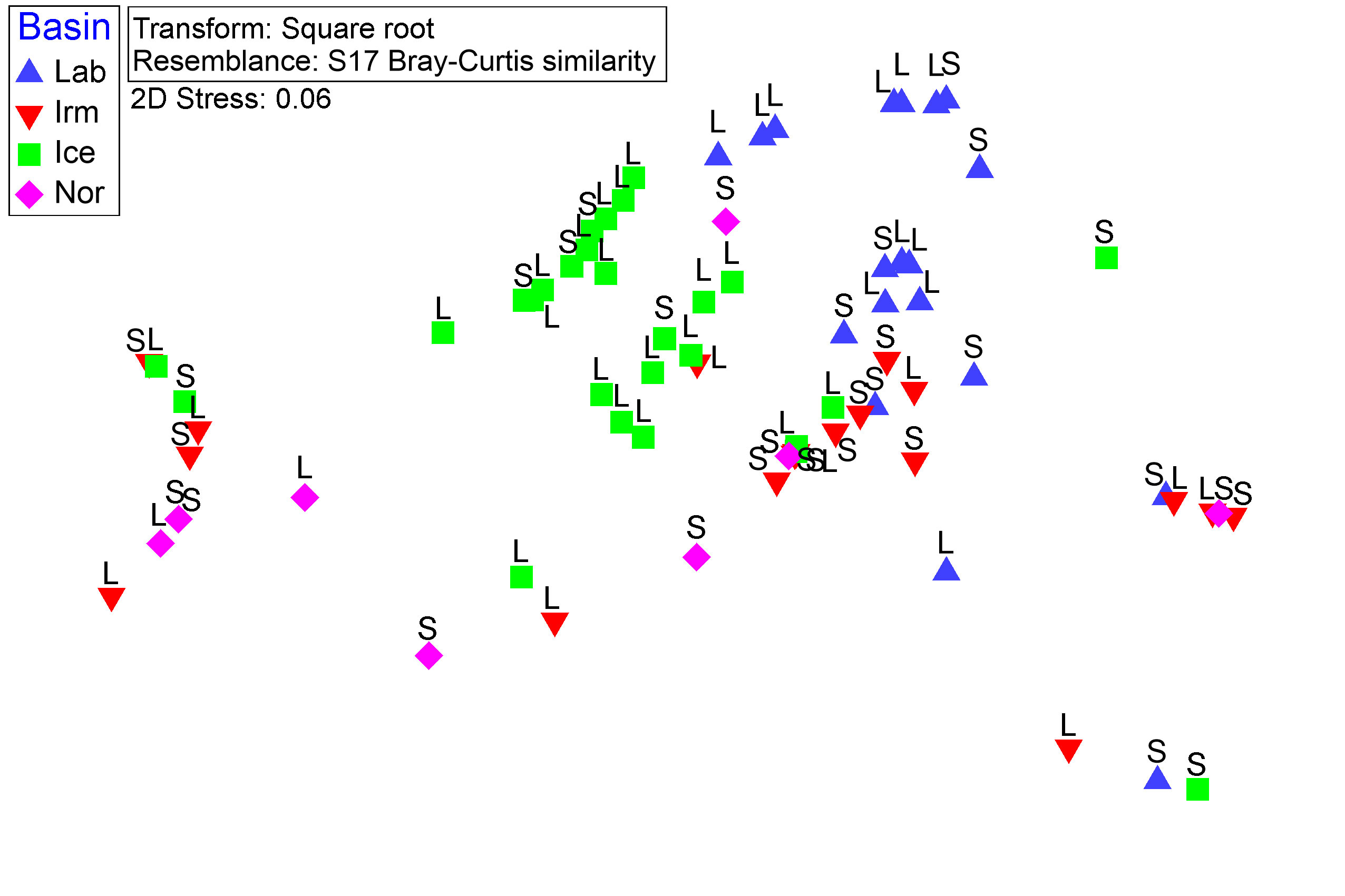
Figure 9 Non-metric Multidimensional Scaling (nMDS) of Benthosema glaciale diet. Prey weight as input (expressed as the ratio between prey weight and predator weight). Symbols represent the basins (Lab=Labrador Sea, Irm=Irminger Sea, Ice=Iceland Sea, and Nor=Norwegian Sea) where S and L are fish length groups (S=small fish and L= large fish, SL< 50 mm and SL≥ 50 mm respectively).
3.4 Prey selectivity
A total of eight MOCNESS profiles were deemed to be taken in close enough proximity to the trawl samples with B. glaciale to allow for examination of prey selectivity in B. glaciale (Figure 10). C. finmarchicus and other copepods were numerically the dominating groups on all 8 stations. Appendicularia were numerically important in the upper 50 meters on two of the three Labrador stations.
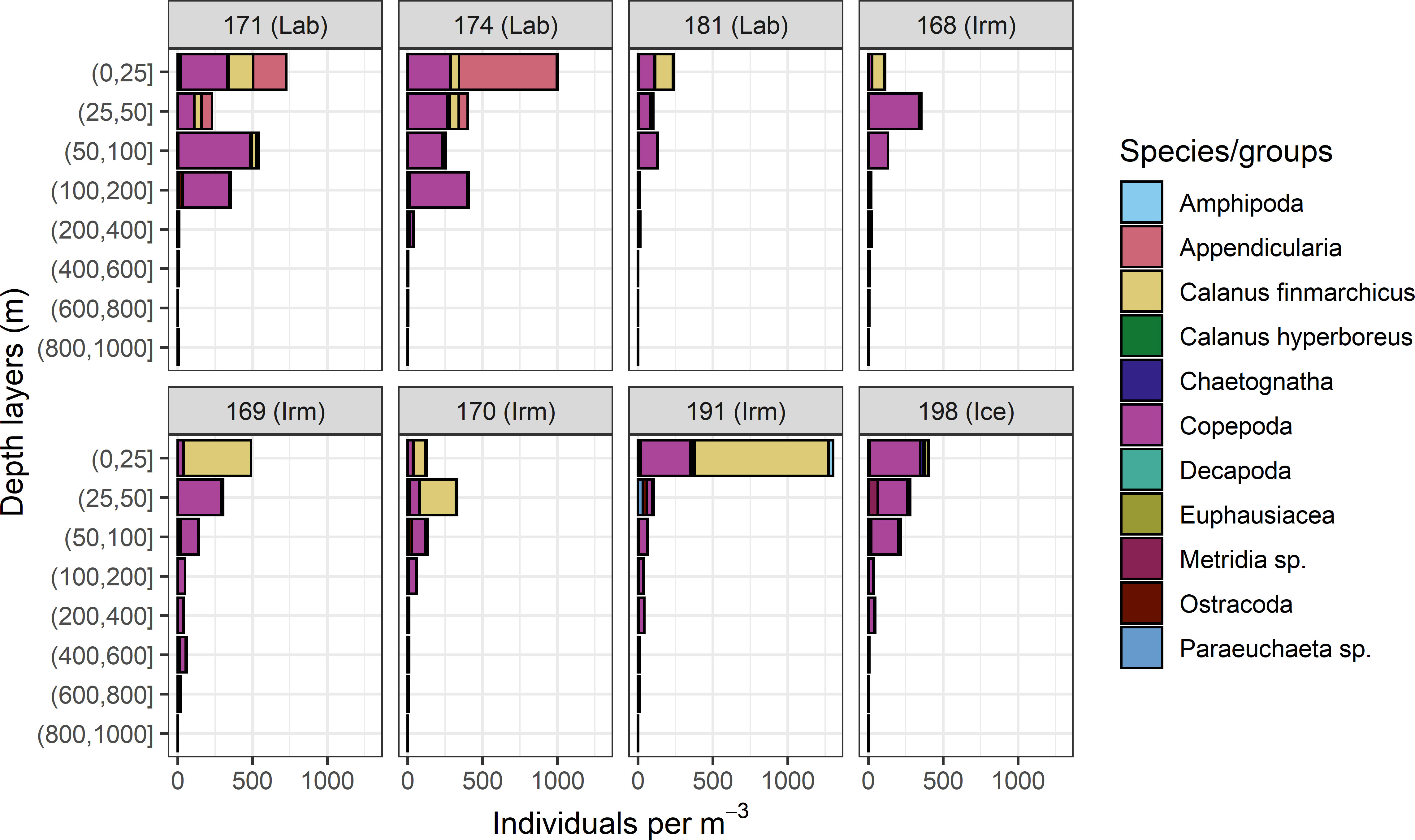
Figure 10 The composition of zooplankton taxonomic groups from MOCNESS hauls used for the Ivlev electivity index calculations. The data are grouped using the same categories as those obtained for the stomach contents. Each panel show the basins and the associated CTD station number where the sample was obtained. St 168-171 show data from the westward leg, while st174-198 are data from the eastward return to Norway (see Drinkwater et al., 2020).
The Ivlev’s electivity index (cf. Figure 11) suggest that Chaetognaths, Copepoda, Metridia sp. and ostracods were negatively selected, that is, possibly avoided or not preferred in the Icelandic, Labrador and the Irminger Seas, while Paraeuchaeta sp. were selected in all those basins. The index also suggests that in the Iceland Sea B. glaciale strongly selected for C. hyperboreus but avoided C. finmarchicus and other copepods. Euphausiids were actively selected for in the Iceland Sea and Irminger basin, while Appendicularia were positively selected for in the Labrador Sea. In the Iceland and Irminger Seas the latter group was not recorded in either stomachs or MOCNESS samples, but Appendicularians were present in MOCNESS samples at depths not used to compute the Ivlev index. Further, Amphipods were selected for in the Iceland and Labrador Seas, but with a much higher positive index in the Iceland Sea. The clearly negative index for Amphipods in the Irminger Sea suggests that this group was not preferred here.
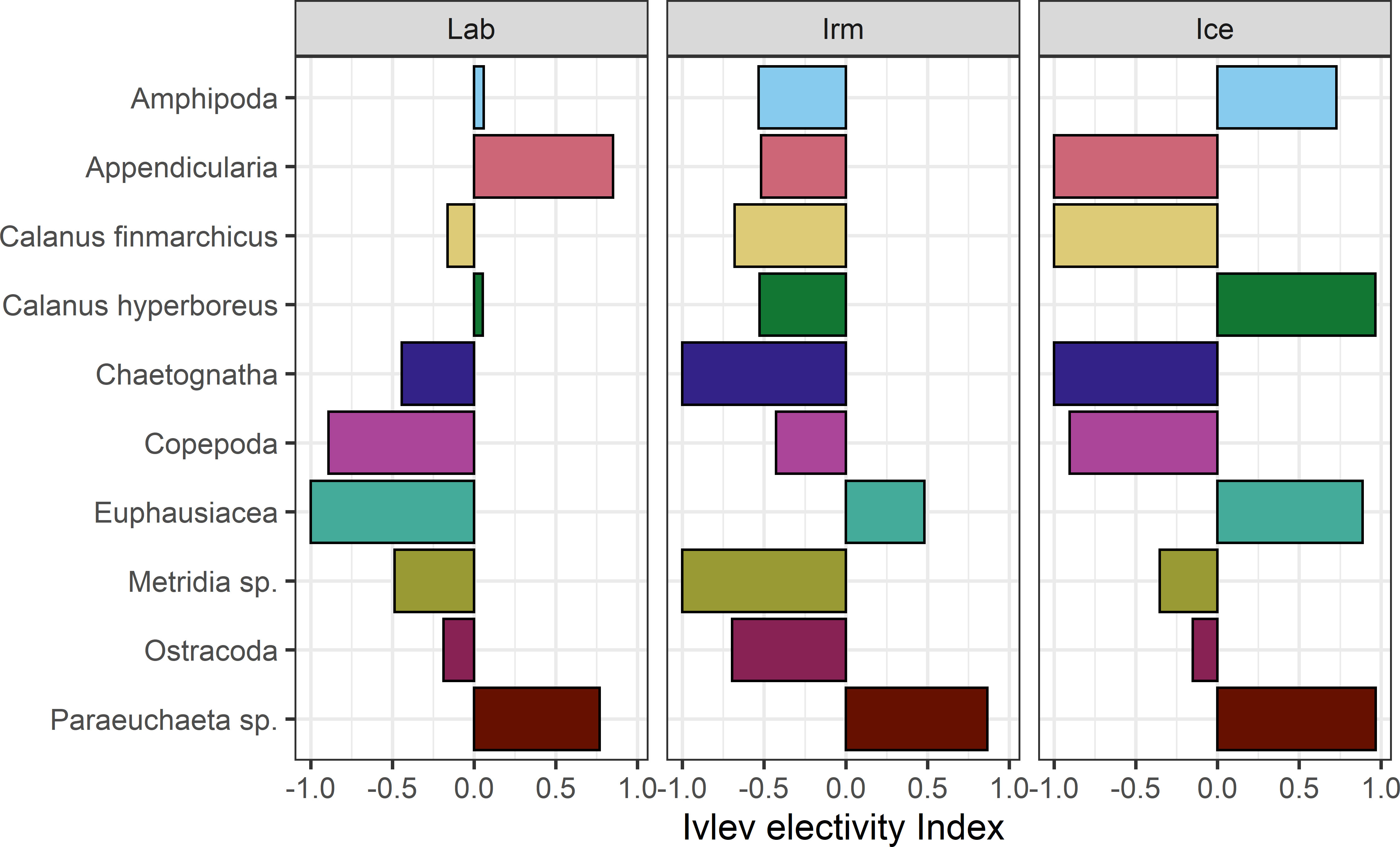
Figure 11 Ivlev’s electivity index calculated based on mean numerical abundance (number of prey items in stomach) for both length groups combined and compared to in situ density of zooplankton aggregated to the same taxonomic groups obtained from vertically stratified MOCNESS samples. Negative index values reflect negative selection and positive values reflect positive selection.
4 Discussion
Glacier lantern fish (Benthosema glaciale, Reinhardt 1837) is probably the most important and most widely distributed myctophid in the northern North Atlantic (Gjøsæter and Kawaguchi, 1980). It is also a key mesopelagic in the deep fjords of western Norway (Gjøsæter, 1973a; Gjøsæter, 1973b; Giske et al., 1990; Baliño and Aksnes, 1993; Suneetha and Salvanes, 2001; Kristoffersen and Salvanes, 2009). A significant characteristic of the species, like in many other mesopelagic fishes, is its diel vertical migration range that might extend close to 700 m depth in some oceanic regions of the North-Atlantic (cf. Halliday, 1970) and even as deep as 950 m (Sameoto, 1988). In the Norwegian Sea however, only very few B. glaciale have been found below 600 m depth in stratified MOCNESS samples (cf. IMR, database), suggesting that the very cold intermediate and deep-waters of this region (Blindheim and Østerhus, 2005), can pose some limitations to the vertical depth range of the species here. Such variability in the depth extension of a key mesopelagic species is possibly also reflected in the vertical extension of the Deep Scattering Layer’s for the examined regions, although admittedly other scatterers can also be involved (cf. Klevjer et al., 2016; Klevjer et al., 2020b).
Another important aspect of the B. glaciale habitat is how its vertical distribution can be directly impacted by the abundance and vertical distribution of associated mesopelagic species, the ambient prey field and abundance of key food organisms. The understanding of ecosystem functioning should take into consideration the elaborate biological interactions that could result in these systems. The somewhat complex and variable acoustics as well as Macroplankton trawl and stratified zooplankton data (cf. Figure 10) across the four basins examined (cf. Strand et al., 2020; Klevjer et al., 2020a; Klevjer et al., 2020b), underlines this potential intricacy.
Also, the vertical migration pattern displayed by B. glaciale varies with season and environmental conditions (Dypvik et al., 2012a; Dypvik et al., 2012b), and does not appear to be identical over the species geographic and environmental range (Klevjer et al., 2020b). Particularly during summer, vertical migration of mesopelagics in the Norwegian and Iceland Seas of which B. glaciale is a key component, is apparently more restricted towards northern latitudes (cf. Norheim et al., 2016). The vertical migration behavior is an important element in the oceans biological carbon pump, as vertical migrants that feed in the epipelagic waters during night-time, bring ingested material back to the depths of the oceans (cf. Davison et al., 2013: Jónasdóttir et al., 2015; St. John et al., 2016; Cavan et al., 2019). At depth carbon could be released as fecal pellets during egestion (particulate organic carbon, POC), as dissolved organic carbon (DOC), dissolved inorganic carbon (DIC) during respiration (Steinberg et al., 2000; Robinson et al., 2010; Cavan et al., 2019; Saba et al., 2021) or as fish gut precipitated carbonate (cf. Wilson et al., 2009). The importance of the latter component is currently largely unknown, but likely to increase in the future and even become a more important component in the inorganic carbon production (Wilson et al., 2009; Foran et al., 2013).
According to the “light exclusion” hypothesis (Kaartvedt, 2008; Langbehn et al., 2022) mesopelagic fish at high latitudes are expected to face adverse feeding conditions, as the absence of periods of sufficient darkness during the polar summer is hypothesized to restrict migration into surface waters at night. Such restricted migration was indeed observed in the Iceland Sea a month before our sampling, i.e., the shallowest nighttime depth of the mesopelagic acoustic scattering layer was around 300 m (Norheim et al., 2016). Our observations, however, do not support that this DVM restriction led to a reduction in the food intake. At the contrary, a surprisingly high proportion of individuals had prey items in their stomachs in the Icelandic Sea at 68°N, and the high condition factor also suggest that high latitude B. glaciale were successful in their feeding activity (Figures 5–7) despite the evidence for restricted DVM (Norheim et al., 2016). Given that the shallowest DVM depth of B. glaciale was around 300 m, which correspond to the main acoustic scattering layer (Figure 3 in Norheim et al., 2016), this means that the zooplankton prey concentration at this depth likely was adequate for feeding. This is in contrast to the assumption of light exclusion hypothesis assuming that sufficient feeding is ensured by DVM into the productive surface layer.
4.1 Size and growth
There is considerable spatial variation in the size distribution of B. glaciale and the diet typically is assumed to change with size (Sameoto, 1988, 1989; García-Seoane et al., 2013; Pepin, 2013), although some changes to these general patterns have been shown (Hudson et al., 2014). The estimated growth rate and maximum length of the species also vary. For instance, in the Norwegian fjords B. glaciale has a higher growth rate (k = 0.38-0.6) and lower asymptotic length (Linf = 65-70 mm) than in the Norwegian Sea (k = 0.18, Linf = 106 mm), but compare to values found for the Flemish Cap population (k=0.47, Linf= 69.5 mm). Such traits can be related to the area’s temperature regime (Kristoffersen and Salvanes, 2009), although other effects might also contribute, such as restricted exchange of organisms between stocks (cf. Suneetha and Salvanes, 2001; Kristoffersen and Salvanes, 2009). This could have restricted gene flow even between closely located “populations” (cf. Song et al., 2016), for instance affecting fjord and regional stocks in the North Atlantic basins differently. However, some interesting differences between the examined basins can be traced, e.g. the smallest individuals dominating the size distribution in the Norwegian Sea, peaking at ~3 cm and between 3-5 cm standard length in the Irminger and Labrador Seas. In the northwest of the Atlantic Ocean previous studies have found comparable size distributions of adult fish (4-7 cm) (Halliday, 1970; García-Seoane et al., 2013, 2014; 2015), while data from the mid-Atlantic ridge (Hudson et al., 2014), suggests a bimodality in the size structure although it might be biased showing just the individuals examined for stomach content (from different trawls), rather than being a true standardized and quantitative size distribution. In the Icelandic Sea (~68°N-70°30’N) north-east of Iceland, the size distribution in our data peaked at 6 cm standard length and very few individuals were found below 4 cm standard length here. A similar tendency towards larger individuals was observed from the region of the Nova Scotian slope to the Davis Strait, while in the upper Baffin Bay (>72°30’N) with a much more restricted light regime and very cold temperatures, no Benthosema were found (Sameoto, 1988, 1989). The low temperatures in the Iceland basin (between 0-2°C in the upper ~180 m, and mainly below 0°C deeper in the water column (cf. Drinkwater et al., 2020) possibly pose challenges to the Benthosema population there. These conditions do not seem to restrict survival of larger and older B. glaciale but possibly affect recruitment, similar to what was suggested for the Davis Strait population (Sameoto, 1989). Whether this happens because adult gonad maturation is affected at some stage or if it could be due to poor survival of eggs and larvae in these more extreme environments, is currently not understood.
4.2 Across basin diet differences, and prey selectivity
Diet and foraging are well studied in B. glaciale from Canadian and Labrador areas, and numerous studies describe diet also in Norwegian fjords and along the mid-Atlantic ridge south of Iceland. However, such data are largely lacking from central areas of the Norwegian, Iceland and Irminger Seas. Our results showed that the most important prey group of glacier lantern fish in the studied areas of the North Atlantic are copepods, which is consistent with previous studies (Gjøsæter, 1973a; Sameoto, 1988, 1989; Dypvik, 2010; Dypvik et al., 2012a; García-Seoane et al., 2013; Pepin, 2013; Hudson et al., 2014). However, significant differences in diet have been detected between basins (with the exception of the Norwegian and the Irminger Sea (cf. Figure 9). In some regions of the Atlantic Ocean, other species are also important prey for B. glaciale (e.g., krill in the Northeast Atlantic and ostracods off Northwest Africa) (Gjøsæter, 1973b; Kinzer, 1977; Roe and Badcock, 1984; Baliño and Aksnes, 1993). During the current study krill were positively selected in the Iceland and Irminger Seas, but negatively selected in the Labrador Sea. In the Flemish Cap, western Atlantic, a very broad spectrum of prey were recorded which also included euphausiids, amphipod, ostracods, chaetognaths, appendicularians, gastropods and polychaetes (García-Seoane et al., 2013). However, during our study the latter two groups were not registered but amphipods were relatively important across all basins for large B. glaciale, and for smaller fish in the Norwegian Sea. In terms of stomach weight relative to body weight amphipods were clearly the most important for large B. glaciale in the Iceland Sea. Arrow worms (Chaetognatha) were probably considerably underrepresented in the stomachs since only remnants of spiny jaws or bristles could be identified. Their importance is therefore very uncertain and that they were negatively selected in all basins could be an artifact of insufficient data. Comparatively in the colder environment of the Davis Strait chaetognath remains in B. glaciale stomachs were much higher compared to the Nova Scotia region (Sameoto, 1988, 1989), probably because the abundance of chaetognaths in the Davis Strait region were much higher compared to the more south-eastern Nova-Scotia population. In the current study appendicularians (Appendicularia) were quite important as prey in the Labrador Sea but were not found in the Iceland and Norwegian Sea fish stomachs. Also, in the western Atlantic, the Grand Bank and Flemish Cap region, appendicularians were represented in the diet (Albikovskaya, 1988; García-Seoane et al., 2013, their Table 1), but their importance seemed minor.
In the Iceland Sea, C. finmarchicus and chaetognaths were not recorded in any stomachs, though these groups were present in the MOCNESS samples. This results in an Ivlev index of -1 independent on the density of these species in situ. This was also the case for chaetognaths and Metridia sp. in the Irminger Sea, as well as for euphausiids in the Labrador Sea. However, the interpretation of the modified Ivlev index should be approached with caution as there are both statistical and ecological concerns regarding such indices, including the modified index by Jacobs (1974), (cf. Strauss, 1979; Gras and Saint-Jean, 1982).
Paraeuchaeta sp. was a quite important prey component in most basins and length groups, except for large B. glaciale in the Norwegian Sea, and positively selected for in all three basins examined (cf. Figures 8, 11). Paraeuchaeta is a genus of carnivorous copepods that has an extensive vertical distribution (cf. Fleddum et al., 2001; Kaartvedt et al., 2002 and references herein) including the species Paraeuchaeta norvegica in the Norwegian Sea, as well as in the deep west-Norwegian fjords (Bagøien, 1999). Thus, it is not surprising that a mesopelagic fish would find this reasonable large (up to ~6 mm adult prosome length, Mauchline, 1999) and visible copepod (Vestheim et al., 2005 and references therein), over a considerable depth range when it is vertically migrating. It is a nearly omnipresent source of food found regularly also deep in the water column which might be preferred to food items that are occasionally less abundant (e.g. Dale et al., 2001) or that at least seems to be preferred by a wide size range of B. glaciale (cf. Kawaguchi and Mauchline, 1982). That this carnivorous copepod has some distinctive features that might make it easier to identify in stomach content, potentially causing a bias in the selectivity pattern is a possibility, although we think in the current material it is less likely.
Benthosema glaciale, like most myctophids, is planktivorous and likely vision is crucial for feeding, predator avoidance and mating (Turner et al., 2009). In its vertical range/habitat light is found both in the form of downwelling light and as light originating from bioluminescent sources, and both “forms” of light may be important to visual feeding (Aksnes and Utne, 1997; Turner et al., 2009; Nilsson et al., 2014). Of the important items found in the diet, Euphausiids, Metridia sp., some other copepods, ostracods and appendicularians are known to produce bioluminescence (cf. Galt and Sykes, 1983; Herring, 1988; Lapota et al., 1989), a distinct feature of many prey organisms and which in some regions during night may increase the encounter rate with bioluminescent zooplankton (cf. Roe and Badcock, 1984 - Metridia; Berge et al., 2012; Cronin et al., 2016).
It is likely that both prey abundance, prey size, bioluminescence and overall visibility, all play an important part of B. glaciale selecting one prey over another during foraging (Kawaguchi and Mauchline, 1982; Roe and Badcock, 1984; Herring, 1988; Sameoto, 1988; Hudson et al., 2014). Secondary issues are geographic location, time of the year, dominant water masses, competitors or lack thereof, and the zooplankton community composition (cf. Gjøsæter, 1973a, b; Gjøsæter and Kawaguchi, 1980; Sameoto, 1988, 1989; Kristoffersen and Salvanes, 2009; García-Seoane et al., 2013). Both Sameoto (1988) and García-Seoane et al. (2013) found that size range of prey increased with fish body length, but Sameoto (1988) also found no significant statistical difference in the length range of prey particles in fish of lengths between 20 to 79 mm total length. These fish continued to feed on the same small prey as smaller fish (see also Gjøsæter, 1973b; Kawaguchi and Mauchline, 1982), while fish between 10-19 mm showed a much higher percent frequency of particle sizes <3 mm compared to larger fish. Fish <30 mm SL were not caught during the investigations by García-Seoane et al. (2013), but the diet of B. glaciale >30 mm varied ontogenetically showing increased prey size with increasing predator size as shown also for a larger span of fish sizes [<15 - 62.5 mm] (Kawaguchi and Mauchline, 1982). Our results seem to show that somewhat larger prey, e.g. amphipods, are common in larger Benthosema, while it is more inconclusive with respect to smaller prey and in particular smaller copepods. Having separate stomach content categories for smaller, intermediate, and larger sized copepods not taxonomically identified, could probably improve B. glaciale future stomach content analyses.
The dominance of older and larger B. glaciale in the Iceland Sea preferentially feeding on one of the largest species of pelagic copepods in the Arctic, C. hyperboreus while B. glaciale recruits (see Kawaguchi and Mauchline, 1982) are basically absent, contrasts what is observed in boreal and more temperate waters. That B. glaciale strongly selected for C. hyperboreus in the Iceland Sea, but avoided C. finmarchicus and other copepods, does indicate that size, fat content, visibility and maybe abundance/availability of C. hyperboreus are superior to any other characteristics of prey otherwise present in the water column here.
The overall observation for all basins, that small fish show a higher maximum stomach fullness than larger fish is intriguing. Ontogenetic variation in DVM patterns have been previously described in mesopelagic fish such as Mueller’s pearlside (Maurolicus muelleri), where juvenile fish stays higher in the water column than their adult counterpart. Giske and Aksnes (1992) argued that this behavior could be explained by juveniles being willing to take higher risk for increased food intake in order to more quickly reach maturity. Since we do not have vertically stratified data on B. glaciale length composition, we can only speculate as to the higher stomach contents seen in smaller fish in our study is related to different DVM strategies.
4.3 Abundance, recruitment, condition factors and the light exclusion hypothesis
Available data suggests that abundances of myctophids declines at high latitudes (Sameoto, 1989; Knutsen et al., 2017) and this has been hypothesized to be an effect of the extreme light environment at these latitudes (Kaartvedt, 2008 “light environment exclusion” (LEE) hypothesis), with continuous daylight during summer “preventing” DVM into the productive upper waters and continuous darkness during winter leaving visual predators less efficient at detecting prey. This mechanism was analysed in a recent model study simulating myctophid feeding, growth and survival along a latitudinal gradient from 50°N to 85°N (Langbehn et al., 2022). Their simulation suggests that because of LEE, myctophids are increasingly unable to accumulate sufficient surplus energy to reproduce, particularly at latitudes higher than ~65°N. While the model reproduces the northward restriction in the DVM range as observed in Norheim et al. (2016), the expectation of summer starvation at latitudes higher than 65°N (Figure 3 in Langbehn et al., 2022) is in error with our observation at 68.37°N in the Iceland Sea. The condition factor and the liver indicator for B. glaciale were clearly higher than in the more southerly basins, even for “small” (in our case defined as <50 mm) individuals (Figures 5, 6). Stomach contents also show that these individuals, probably being sampled at larger depths on average (Table 3) appear to be more successful in finding and ingesting prey (Figures 7, 8), as no fish from the Iceland Sea basin had empty stomachs, and most individuals had much more stomach contents than those in the more southern located basins (Figures 7, 8). The individuals of our sample were obtained at a fishing depth of 330 – 390 m (station 162 in Table 1) with a WMD of 420 m (cf. Table 3) in a region where DVM appears to be constrained to depths deeper than 300 m (Norheim et al., 2016) but nevertheless appear to thrive. The model expectation involving summer starvation in Langbehn et al. (2022) results from an assumed zooplankton prey field largely confined to the upper 100 m which is not in accordance with our observations. In this respect, it should be noted that the highest densities of C. hyperboreus is indeed considered to reside in the upper 100 m in the Iceland Sea in June (cf. Astthorsson and Gislason, 2003). However, our own data from the same basin and month, not far from the stations of Astthorsson and Gislason (2003), do show that C. hyperboreus is clearly present also below 100 m, but at lower densities.
It is possible that B. glaciale in this region could undertake asynchronous excursions (cf. Pearre, 2003) to the upper 100 m with the highest densities of potential prey (see Astthorsson and Gislason, 2003 and our Figure 10), thus minimizing predation risk while covering their energy demand even if light conditions are unfavourable. A similar behaviour for B. glaciale using irregular excursion to the upper 30 m at daytime has been suggested by Sameoto (1988). Such “bold” behaviour has been documented for a small fraction of the population of the mesopelagic fish Maurolicus muelleri in Norwegian fjords (Christiansen, 2021; Christiansen et al., 2021). However, we currently have no evidence from acoustic or net data that B. glaciale does undertake such excursions, and previous studies have described B. glaciale as having sluggish behaviour during daytime (Kaartvedt et al., 2009), typically showing small, intermittent vertical relocations (ascent/descent) with pauses between vertical steps (Kaartvedt et al., 2008; Dypvik et al., 2012b).
What our data show is that the fishes that have managed to cope with the high latitude environment are doing fine, but that very few smaller B. glaciale are among these. This may suggest that it is the recruitment that is affected, i.e. that the constraint(s) affects spawning or the smaller life-stages of the B. glaciale. Smaller individuals require smaller food items potentially being distributed higher in the water column and higher mortality in smaller life stages might reflect higher DVM risk exposure as expressed in the LEE hypothesis (Kaartvedt, 2008; Langbehn et al., 2022). There is an indication in our data that the relative gonad size of both large (SL≥ 50 mm) and small B. glaciale (SL< 50 mm) is lower in the Iceland and Norwegian Seas (Figure 6), which may suggest that less energy is diverted to reproduction here. The condition factor for both the small and large B. glaciale (cf. Figure 5) and the liver indicator (Figure 6) for both length groups combined, indicate that energy stored were significantly higher for fish in the Iceland Sea compared to the other basins. High condition factors (liver indicator, relative weight and amount of fat stored) have been related to the period just prior to the subsequent spawning season (e.g., Nunes et al., 2011). In the Northwest Atlantic the spawning period for B. glaciale occurs from January to April (Mazhirina, 1988), while in the Northeast Atlantic from June to July (Gjøsæter, 1981). Thus, the samples in the Irminger and Labrador Seas were possibly collected after the spawning period. Samples in the Iceland Sea were collected on 8 June, maybe just in the beginning of the spawning season, whereas the Norwegian Sea was sampled 4 days thereafter, probably also very early on in the maturation cycle. This seems to be supported by the condition, gonad and liver indices of the fish in these basins (Figures 5, 6). Spawning period or not, the stomach fullness of B. glaciale in the Iceland Sea was much higher than what was observed for fish in the other basins (Figure 7). No fish in the Iceland Sea was found with an empty stomach. The dominating prey of B. glaciale in the Iceland Sea is the large copepod, Calanus hyperboreus, very rich in fat – as determined from the size of the lipid sacs of some key Calanus species and copepodite stages (cf. Vogedes et al., 2010), and our data shows directly that B. glaciale are successfully feeding in this area during the summer.
However, the number of fish investigated is restricted, and there is also the possibility of differences in phenology, but the patterns revealed above, clearly warrants further investigation. The estimated abundance of large B. glaciale was higher in the Iceland Sea (Figure 4) than in the Norwegian Sea, which is hard to reconcile with increased mortality. Previous studies have shown that B. glaciale in the Norwegian Sea had slower growth, attained a larger size and showed a similar mortality rate compared to populations in western Norwegian fjords further south (Kristoffersen and Salvanes, 2009). Similar patterns are evident in distributions of myctophids in both the Northern Atlantic (Klevjer et al., 2020a) and the Southern Ocean, Scotia-Weddel sector [~48-62°S] (Saunders et al., 2017; Dornan et al., 2019; Escobar-Flores et al., 2020): poleward ecosystems show decreased abundances of small individuals but despite the drop in abundance implied by this, biomass levels across comparable gradients appear similar (Escobar-Flores et al., 2020; Klevjer et al., 2020a). These patterns are all consistent with low mortality rates for larger individuals. Large myctophids have larger energy stores, may feed on larger zooplankton (cf. Sameoto, 1988; García-Seoane et al., 2013) and may consequently show different vertical distribution and migration patterns than smaller individuals (Giske et al., 1990; Dypvik et al., 2012b). Hence, the LEE hypothesis may still be valid for smaller myctophids as well as for the younger life-stages of the larger myctophids, but the hypothesis does not appear to be compatible with patterns seen for large individuals in either the North Atlantic or the Southern Ocean. Furthermore, since parts of the Antarctic zone is also at “somewhat lower latitudes” [~48-62°S] (Saunders et al., 2017), and lack of recruitment is also indicated for parts of the Antarctic zone at considerably lower latitudes than in the north (e.g. where the light environment is not as extreme), other factors are likely to be important. In the Norwegian Sea ecosystem high backscattering levels from mesopelagic organisms are restricted to the relatively warm Atlantic water-masses (Dale et al., 1999; Knutsen et al., 2017), while for instance in the northern reaches of Baffin Bay having very low water temperatures neither larvae nor adults of B. glaciale could be traced (Sameoto, 1989). Since the latitudinal gradients in both the northern and southern Atlantic are also characterised by transitions towards colder temperatures, a relationship between water temperature and myctophid recruitment is a possibility. Based on samples from 12 research cruises spanning a 10-year period, Karaseva (1986) suggested that the thermal niche of larval B. glaciale is restricted to 6.0 to 14.5°C, with the realised niche expanding for young, post-metamorphose stages (5.0 to 16.0°C, Karaseva, 1986). A recent study has found a clear correlation between temperature and distribution of mesopelagic components across polar fronts in both hemispheres (Chawarski et al., 2022), suggesting a link between temperatures and trophic structure in the mesopelagic zone.
Interestingly, in the southern regions of Baffin Bay (~67°30’N-70°30’N) both myctophids Benthosema and Lampanyctus have been found in stomachs of feeding northern fulmars/black-legged kittiwakes during early summer (9 June-12 July), in a period with a prevailing midnight sun in the whole region (LeBlanc et al., 2019). These birds are visual feeders and are only capable of diving less than 2 m (cf. Wahl, 1984; Garthe and Furness, 2001), it is therefore highly surprising that they have access to mesopelagic fish. However, this might suggest that current understanding of mesopelagic vertical migration is inadequate, and that the LEE-hypothesis needs further scrutiny and associated refinement. Sameoto (1988) also found that B. glaciale off the Nova Scotian shelf could undertake brief excursions to the copepod rich layer in the upper 30 m of the water column during daytime. This find is an additional hint that the species could undertake brief excursions towards the surface layers as needed.
4.4 Methodological issues
Most of the relevant literature on Benthosema feeding (Gjøsæter, 1973b; Roe and Badcock, 1984; Sameoto, 1988, 1989; Dypvik et al., 2012b; García-Seoane et al., 2013) suggest that this fish is a dynamic forager, its main feeding period being in the epipelagic domain during night, but that it could also feed at depth during the day, although some variation to this theme is indicated for some investigations (cf. Dypvik et al., 2012a; Dypvik et al., 2012b). Stomach contents are therefore expected to contain a mix of freshly consumed prey and older contents. Another issue that has been raised regarding feeding, is micro-scale aggregation or patchiness of prey that can be important at the individual level as it has been documented that a single myctophid fish could have a monospecific or monosexual prey in the stomach (Scotto di Carlo et al., 1982). Individuals feeding directly on such aggregated prey, could lead to biased feeding patterns within an analysed depth range. To “follow-the-fish” like done during this study and sample also the water column for key prey organisms and according to the vertical extension of the acoustic representation of the fish, seems like a worthwhile approach, but is still not perfect since collecting both the fish and its potential prey simultaneously is difficult. Applying a vertical stratified sampling program using a pelagic trawl where a remotely controlled trawl opening or cod-end closure could have increased the opportunity to better detail where in the water column feeding occurred at the time of sampling. However, this is a an very time-consuming approach that was not possible to undertake with a pelagic trawl during the current expedition. Using a traditional pelagic trawl as outlined above has also some methodological pitfalls, because you cannot simultaneously undertake a vertically stratified sampling of Benthosema’s zooplankton prey, which would likely be the preferred approach. Interestingly, a combined sampling of Benthosema and its prey was however conducted way back in the 1980’s (Sameoto, 1988, 1989), then using the recently developed BIONESS system (Sameoto et al., 1980), a multiple net sampler with ten 1-m2 nets of mesh size 243 µm towed at speed of ~3 knots.
Timing of trawling in relation to timing of ingestion is and will possibly continue to be a challenge although metabarcoding based on stomach content DNA (scDNA, cf. Mychek-Londer et al., 2020) could help determine which types of prey might additionally be present in the undetermined fraction of fish stomachs examined. Such an approach could also aid the documentation of prey bioluminescence. However, stomach content analyses allow the taxonomic examination of the prey including its weight and abundance. If having at hand information on the bioluminescence characteristics of Benthosema prey found in situ, would maybe be a better way to progress the analyses of mesopelagic fish stomach content.
Data availability statement
The datasets presented in this study can be found at PANGAEA - Data Publisher for Earth and Environmental Science (Knutsen et al., 2022a, https://doi.org/10.1594/PANGAEA.951040, Knutsen et al., 2022b, https://doi.org/10.1594/PANGAEA.950983, Knutsen et al., 2022c, https://doi.org/10.1594/PANGAEA.951043).
Ethics statement
Ethical review and approval were not required for the animal study because The Institute of Marine Research (IMR) fully adheres to Norwegian laws relevant to Ethics in Science as well as Animal Welfare. The legal and institutional framework within which IMR operate is detailed in OECD (2012, Part III, Chapter 21, p. 373-398). Under the current legal framework, there is no special permit requirements for at sea research and monitoring activities which do not involve experiments with live animals.
Author contributions
All authors contributed to the article and approved the submitted version. The data presented in the paper is partly a reanalysis of the data of SMS’s MSci thesis and partly additional data. AS, ES, DA, TAK, TK and WM were responsible for and conducted the practical work onboard the research vessel when samples were collected. WM was the cruise leader and responsible for the overall project conceptualization, the scientific mission, investigation, administration, and funding. AS had the idea to this diet study. SS did the lab work and the analysis and interpretation of the MSci thesis data under supervision of AS, DA, CB, ES and the scientific support of EG-S. TAK, ES and EG-S reworked the analyses and made all figures. All authors have contributed to conceptualization, writing and reviewed drafts of the paper with TK as the lead author.
Funding
The financial support of the Institute of Marine Research that made the mission with G.O. Sars possible is greatly appreciated. The EU is thanked for support through the SUMMER project, grant agreement number no. 817806, Euro-BASIN (Integrated Project on Basin Scale Analysis, Synthesis and Integration), funded by Framework Programme 7, Contract 264933, as well as through the MEESO project, grant agreement number no. 817669. Also, the Research Council of Norway is thanked for the financial support through “Harvesting marine cold-water plankton species - abundance estimation and stock assessment” - (Harvest II, RCN 203871), “Harvesting the mesopelagics - ecological and management implications” (HARMES, RCN 280546) and through the project “Hypoxia effects on fish in west Norwegian fjords: harnessing the power of multidisciplinary studies” (HypOnFjordFish, RCN 301077).
Acknowledgments
We gratefully acknowledge the cooperative effort and support provided by the Captains and Crew of the RV G.O. Sars during the six-weeks long trans-Atlantic expedition. The skilled support of Monica B. Martinussen (IMR’s Plankton Group) on the taxonomic analyses of Benthosema stomach content is greatly appreciated. Our colleague TAK, who played a pivotal role in the Euro-BASIN field work and in the analyses and writing of this article, died suddenly 13 October 2022 prior to publication of this paper. We would therefore like to dedicate this work to his memory. The work is also considered a contribution to the Norwegian Sea Ecosystem Programme at IMR. We remain thankful for the comments of two reviewers that have clearly improved the paper.
Conflict of interest
The authors declare that the research was conducted in the absence of any commercial or financial relationships that could be construed as a potential conflict of interest.
Publisher’s note
All claims expressed in this article are solely those of the authors and do not necessarily represent those of their affiliated organizations, or those of the publisher, the editors and the reviewers. Any product that may be evaluated in this article, or claim that may be made by its manufacturer, is not guaranteed or endorsed by the publisher.
References
Aksnes D. L., Utne A. C. W. (1997). A revised model of visual range in fish. Sarsia 82, 137–147. doi: 10.1080/00364827.1997.10413647
Albikovskaya L. K. (1988). Some aspects of the biology and distribution of glacier lanternfish (Benthosema glaciale) over the slopes of Flemish cap and Eastern grand bank. NAFO SC Stud. 12, 37–42.
Anderson M. J., Gorley R. N., Clarke R. K. (2008). Permanova+ for primer: Guide to software and statistical methods (Plymouth, UK: Primer-E Ltd).
Astthorsson O. S., Gislason A. (2003). Seasonal variations in abundance, development and vertical distribution of Calanus finmarchicus, C. hyperboreus and C. glacialis in the East icelandic current. J. Plank. Res. 25 (7), 843–854.
Bagøien E. (1999). Predatory impact of invertebrates and fish on overwintering calanus (Norway: Department of Biology, University of Oslo).
Bagøien E., Kaartvedt S., Aksnes D. L., Eiane K. (2001). Vertical distribution and mortality of overwintering calanus. Limnol. Oceanogr. 46, 1494–1510. doi: 10.4319/lo.2001.46.6.1494
Baliño B., Aksnes D. L. (1993). Winter distribution and migration of the sound scattering layers zooplankton and micronekton in masfjorden western Norway. Mar. Ecol. Prog. Ser. 102, 35–35. doi: 10.3354/meps102035
Berge J., Båtnes A. S., Johnsen G., Blackwell S. M., Moline M. A. (2012). Bioluminescence in the high Arctic during the polar night. Mar. Biol. 159, 231–237. doi: 10.1007/s00227-011-1798-0
Blindheim J., Østerhus S. (2005). The Nordic seas, main oceanographic features (Washington, DC: American Geophysical Union (AGU)), Vol. 158. 1–27. doi: 10.1029/158GM03
Caiger P. E., Lefebve L. S., Llopiz J. K. (2021). Growth and reproduction in mesopelagic fishes: a literature synthesis. ICES J. Mar. Sci. 78, 765–781. doi: 10.1093/icesjms/fsaa247
Cavan E. L., Laurenceau-Cornec E. C., Bressac M., Boyd P. W. (2019). Exploring the ecology of the mesopelagic biological pump. Prog. Oceanogr. 176, 102125. doi: 10.1016/j.pocean.2019.102125
Chawarski J., Klevjer T. A., Coté D., Geoffroy M. (2022). Evidence of temperature control on mesopelagic fish and zooplankton communities at high latitudes. Front. Mar. Sci. 9. doi: 10.3389/fmars.2022.917985
Christiansen S. (2021). From acoustic scattering layers to individuals - behaviour of a mesopelagic fish at different scales. dissertation presented for the degree of philosophiae doctor (PhD), department of biosciences, faculty of mathematics and natural sciences no. 2463 (Norway: University of Oslo), 1–76.
Christiansen S., Klevjer T. A., Røstad A., Aksnes D. L., Kaartvedt S. (2021). Flexible behaviour in a mesopelagic fish (Maurolicus muelleri). ICES J. Mar. Sci. 78, 1623–1635. doi: 10.1093/icesjms/fsab075
Clarke K. R., Gorley R. N., Somerfield P. J., Warwick R. M. (2014). Change in marine communities: an approach to statistical analysis and interpretation. 3rd edition (Plymouth: Primer-E Ltd).
Contreras T., Pilar Olivar M., Bernal A., Sabatés A. (2015). Comparative feeding patterns of early stages of mesopelagic fishes with vertical habitat partitioning. Mar. Biol 162, 2265–2277. doi: 10.1007/s00227-015-2749-y
Cronin H. A., Cohen J. H., Berge J., Johnsen G., Moline M. A. (2016). Bioluminescence as an ecological factor during high Arctic polar night. Sci. Rep. 6, 36374. doi: 10.1038/srep36374
Dale T., Bagøien E., Melle W., Kaartvedt S. (1999). Can predator avoidance explain varying overwintering depth of Calanus in different oceanic water masses? Mar. Ecol. Prog. Ser. 179, 113–121. doi: 10.3354/meps179113
Dale T., Kaartvedt S., Ellertsen B., Amundsen R. (2001). Large-Scale oceanic distribution and population structure of calanus finmarchicus, in relation to physical environment, food and predators. Mar. Biol. 139, 561–574. doi: 10.1007/s002270100605
Davison P. C., Checkley D. M., Koslow J. A., Barlow J. (2013). Carbon export mediated by mesopelagic fishes in the northeast pacific ocean. Prog. Oceanogr. 116, 14–30. doi: 10.1016/j.pocean.2013.05.013
Dornan T., Fielding S., Saunders R. A., Genner M. J. (2019). Swimbladder morphology masks southern ocean mesopelagic fish biomass. Proc. R. Soc B 286, 20190353. doi: 10.1098/rspb.2019.0353
Drinkwater K. F., Sundby S., Wiebe P. H. (2020). Exploring the hydrography of the boreal/arctic domains of north Atlantic seas: Results from the 2013 BASIN survey. Deep–Sea Res. II 180, 1–15. doi: 10.1016/j.dsr2.2020.104880
Dypvik E. (2010). Invers døgnvandring og fødebiologi hos nordlig lysprikkfisk (Benthosema glaciale) i masfjorden. master thesis (Biological Institute, University of Oslo), 1–69.
Dypvik E., Klevjer T. A., Kaartvedt S. (2012a). Inverse vertical migration and feeding in glacier lanternfish (Benthosema glaciale). Mar. Biol. (Norway) 159, 443–453. doi: 10.1007/s00227-011-1822-4
Dypvik E., Røstad A., Kaartvedt S. (2012b). Seasonal variations in vertical migration of glacier lanternfish Benthosema glaciale. Mar. Biol. 159, 1673–1683. doi: 10.1007/s00227-012-1953-2
Escobar-Flores P. C., O’ Driscoll R. L., Montgomery J. C., Ladroit Y., Jendersie S. (2020). Estimates of density of mesopelagic organisms in the southern ocean derived from bulk acoustic data collected by ships of opportunity. Polar Biol. 43, 43–61. doi: 10.1007/s00300-019-02611-3
Fleddum A., Kaartvedt S., Ellertsen B. (2001). Distribution and feeding of the carnivorous copepod Paraeuchaeta norvegica in habitats of shallow prey assemblages and midnight sun. Mar. Biol. 139, 719–726. doi: 10.1007/s002270100618
Foran E., Weiner S., Fine M. (2013). Biogenic fish-gut calcium carbonate is a stable amorphous phase in the gilt-head seabream, Sparus aurata. Sci. Rep. 3, 1700. doi: 10.1038/srep01700
Galt C. P., Sykes P. F. (1983). Sites of bioluminescence in the appendicularians Oikopleura dioica and O. labradoriensis (Urochordata: Larvacea). Mar. Biol. 77, 155–159. doi: 10.1007/BF00396313
García-Seoane E., Bernal Bajo A., Saborido-Rey F. (2014). Reproductive ecology of the glacier lanternfish Benthosema glaciale. Hydrobiologia 727, 137–149. doi: 10.1007/s10750-013-1796-y
García-Seoane E., Dalpadado P., Vázquez A. (2013). Feeding ecology of the glacier lanternfish Benthosema glaciale (Actinopterygii myctophidae) in the Flemish cap (North Atlantic ocean). Hydrobiologia 717, 133–146. doi: 10.1007/s10750-013-1579-5
García-Seoane E., Fabeiro M., Silva A., Meneses I. (2015). Age-based demography of the glacier lanternfish (Benthosema glaciale) in the Flemish cap. Mar. Freshw. Res. 66 (1), 78–85. doi: 10.1071/MF13229
García-Seoane E., Wienerroither R., Mork K. A., Underwood M. J., Melle W. (2021). Biogeographical patterns of meso- and bathypelagic fish along a northeastern Atlantic transect. ICES J. Mar. Sci 78 (4), 1444–1457. doi: 10.1093/icesjms/fsaa255
Garthe S., Furness R. W. (2001). Frequent shallow diving by a northern fulmar feeding at Shetland. Waterbirds: Int. J. Waterbird Biol. 24 (2), 287–289. doi: 10.2307/1522045
Giske J., Aksnes D. L. (1992). Ontogeny, season and trade-offs: Vertical distribution of the mesopelagic fish Maurolicus muelleri. Sarsia 77 (3-4), 253–261. doi: 10.1080/00364827.1992.10413510
Giske J., Aksnes D. L., Baliño B. M., Kaartvedt S., Lie U., Nordeide J. T., et al. (1990). Vertical distribution and trophic interactions of zooplankton and fish in masfjorden Norway. Sarsia 75, 65–81. doi: 10.1080/00364827.1990.10413442
Gjøsæter J. (1973a). Age growth and mortality of the myctophid fish Benthosema glaciale (Reinhardt) from Western Norway. Sarsia 52, 1–14.
Gjøsæter J. (1973b). The food of the myctophid fish Benthosema glaciale (Reinhardt) from western Norway. Sarsia 52, 53–58.
Gjøsæter J. (1981). Growth production and reproduction of the myctophid fish Benthosema glaciale from western Norway and adjacent seas. FiskDir. Skr. Ser. HavUnders. 17, 79–108.
Gjøsæter J., Kawaguchi K. (1980). “A review of the world resources of mesopelagic fish,” in FAO fisheries technical paper no. 193 (Rome: FAO), 1–151.
Goodyear R. H., Zahurance B. F., Pligh W. L., Gibbs R. H. J. R. (1972). “Ecology and vertical distribution of Mediterranean midwater fishes,” in Mediterranean Biological studies final report. report to U.S. navy office of naval research contract no. N00014-67-A-399-000-7 (Washington, D.C.: Smithsonian Institution), 91–229.
Gras R., Saint-Jean L. (1982). Comments about ivlev’s electivity index. Rev. Hydrobiol. Trop. 15 (1), 33–37.
Halliday R. (1970). Growth and vertical distribution of the glacier lanternfish, Benthosema glaciale, in the northwestern Atlantic. J. Fish. Res. Bd. Canada 27, 105–116.
Halliday R. G., Clark K. J., Themelis D. E. (2015). The biology of Benthosema glaciale and Ceratoscopelus maderensis (Myctophidae) in the slope Sea off Nova Scotia, Canada. J. Northw. Atl. Fish. Sci. 47, 75–89. doi: 10.2960/J.v47.m708
Hassel A., Endresen B., Martinussen M. B., Gjertsen K., Knutsen T., Johannessen M. E. (2017). HÅNDBOK FOR PLANKTON. PRØVETAKING OG ANALYSE. prosedyrer for prøvetaking og pre-analyse av dyre- og planteplankton på forskningsfartøy og i laboratorium på land versjon 4.0 (mars 2017) (Bergen, Norway: Institute of Marine Research). 1–155.
Herring P. J. (1988). “Copepod luminescence. hydrobiologia 167/168,” in Biology of copepods. Eds. Boxshall G. A., Schminke H. K. (Norway: ©Kluwer Academic Publishers), 183–195.
Hudson J. M., Steinberg D. K., Sutton T. T., Graves J. E., Latour R. J. (2014). Myctophid feeding ecology and carbon transport along the northern mid-Atlantic ridge. Deep-Sea Res. Part I: Oceanogr. Res. Pap. 93, 104–116. doi: 10.1016/j.dsr.2014.07.002
Ivlev V. S. (1961). Experimental ecology of the feeding of fishes (New Haven: Yale University Press New York), 1–302.
Jacobs J. (1974). Quantitative measurement of food selection. Oecologia 14, 413–417. doi: 10.1007/BF00384581
Jónasdóttir S. H., Visser A. W., Richardson K., Heath M. R. (2015). Seasonal copepod lipid pump promotes carbon sequestration in the deep north Atlantic. Proc. Natl. Acad. Sci. 112 (39), 12122–12126. doi: 10.1073/pnas.1512110112
Kaartvedt S. (2008). Photoperiod may constrain the effect of global warming in arctic marine systems. J. Plankton Res. 30 (11), 1203–1206. doi: 10.1093/plankt/fbn075
Kaartvedt S., Dale T., Bagøien E., Viken T. (2002). Bi-modal vertical distribution of the carnivorous copepod Paraeuchaeta norvegica. J. Plankton Res. 24, 155–158. doi: 10.1093/plankt/24.2.155
Kaartvedt S., Røstad A., Klevjer T. A., Staby A. (2009). Use of bottom-mounted echo sounders in exploring behavior of mesopelagic fishes. Mar. Ecol. Prog. Ser. 395, 109–118. doi: 10.3354/meps08174
Kaartvedt S., Torgersen T., Klevjer T. A., Røstad A., Devine J. A. (2008). Behavior of individual mesopelagic fish in acoustic scattering layers of Norwegian fjords. Mar. Ecol. Prog. Ser. 360, 201–209. doi: 10.3354/meps07364
Karaseva E. M. (1986). “Peculiarities of distribution larvae and juveniles of lanternfish bentosema glaciale in the open waters of the north Atlantic,” in Life history, distribution and migrations of commercial fish in the Atlantic and pacific oceans (Kaliningrad: AtlantNIRO), 77.
Kawaguchi K., Mauchline J. (1982). Biology of myctophid fishes (family myctophidae) in the rockall trough northeastern Atlantic ocean. Biol. Oceanogr. 1 (4), 337–373. doi: 10.1080/01965581.1982.10749447
Kinzer J. (1977). “Observations on feeding habits of the mesopelagic fish benthosema glaciale (Myctophidae) off NW Africa,” in Oceanic sound scattering prediction. Eds. Anderson W. R., Zahuranec B. J. (New York: Plenum Press), 381–392.
Klevjer T. A., Irigoien X., Røstad A., Fraile-Nuez E., Benítez-Barrios V. M., Kaartvedt S. (2016). Large Scale patterns in vertical distribution and behaviour of mesopelagic scattering layers. Sci. Rep. 6, 19873. doi: 10.1038/srep19873
Klevjer T., Melle W., Knutsen T., Aksnes D. L. (2020b). Vertical distribution and migration of mesopelagic scatterers in four north Atlantic basins. Deep-Sea Res. Part II 180, 104811. doi: 10.1016/j.dsr2.2020.104811
Klevjer T., Melle W., Knutsen T., Strand E., Korneliussen R., Dupont N., et al. (2020a). Micronekton biomass distribution improved estimates across four north Atlantic basins. Deep-Sea Res. Part II 180, 104691. doi: 10.1016/j.dsr2.2019.104691
Knutsen T., Wiebe P. H., Gjøsæter H., Ingvaldsen R. B, Lien G.. (2017). High latitude epipelagic and mesopelagic scattering layers - A reference for future arctic ecosystem change. Front. Mar. Sci. 4, 334. doi: 10.3389/fmars.2017.00334
Knutsen T., Strand E., Klevjer T. A., Vea Salvanes A. G., Thorsen Broms C., Myhre Sunde S., et al. (2022b). Metrics of benthosema glaciale collected during RV G.O. sars cruise 2013107 across the north Atlantic in 2013. PANGAEA. doi: 10.1594/PANGAEA.950983
Knutsen T., Strand E., Klevjer T. A., Vea Salvanes A. G., Thorsen Broms C., Myhre Sunde S., et al. (2022c). Stomach contents of benthosema glaciale collected during RV G.O. sars cruise 2013107 across the north Atlantic in 2013. PANGAEA. doi: 10.1594/PANGAEA.951043
Knutsen T., Strand E., Klevjer T. A., Salvanes A. G. V., Thorsen Broms C., Myhre Sunde S., et al. (2022a). Benthosema glaciale diet across the north Atlantic. PANGAEA. doi: 10.1594/PANGAEA.951040
Kristoffersen J. B., Salvanes A. G. V. (1998). Effects of formaldehyde and ethanol preservation on body and otoliths of Maurolicus muelleri and Benthosema glaciale. Sarsia 83, 95–102. doi: 10.1080/00364827.1998.10413675
Kristoffersen J. B., Salvanes A. G. V. (2009). Distribution, growth, and population genetics of the glacier lanternfish (Benthosema glaciale) in Norwegian waters: contrasting patterns in fjords and the ocean. Mar. Biol. Res. 5, 596–604. doi: 10.1080/17451000903042479
Langbehn T. J., Aksnes D. L., Kaartvedt S., Fiksen Ø., Ljungström G., Jørgensen C. (2022). Poleward distribution of mesopelagic fishes is constrained by seasonality in light. Global Ecol. Biogeogr. 31, 546–561. doi: 10.1111/geb.13446
Lapota D., Geiger M. L., Stiffey A. V., Rosenberger D. E., Young D. K. (1989). Correlations of planktonic bioluminescence with other oceanographic parameters from a Norwegian fjord. Mar. Ecol. Prog. Ser. 55, 217–227. doi: 10.3354/meps055217
LeBlanc M., Gauthier S., Garbus S. E., Mosbech A., Fortier L. (2019). The co-distribution of Arctic cod and its seabird predators across the marginal ice zone in Baffin bay. Elem Sci. Anth 7, 1. doi: 10.1525/elementa.339
Le Cren C. D. (1951). The length-weight relationship and seasonal cycle in gonad weight and condition in perch perca fluviatilis. J. Anim. Ecol. 20 (2), 201–219. doi: 10.2307/1540
Mauchline J. (1999). ICES identification leaflets for plankton (Fiches d’Identification du plancton). LEAFLET NO. 182, copepoda, family: Euchaetidae, genus: Euchaeta (Copenhagen, Denmark: International Council for the Exploration of the Sea), 1–10. doi: 10.17895/ices.pub.5162
Mazhirina G. P. (1988). Some information on the development of ovaries in Benthosema glaciale from different areas of the north Atlantic. NAFO Scientific Council Res. Doc. 88 (21), 1–11.
Melle W., Klevjer T., Drinkwater K., Strand E., Naustvoll L. J., Wiebe P. H., et al. (2020). Structure and functioning of four north Atlantic ecosystems - a comparative study. Deep-Sea Res. II 180, 104838. doi: 10.1016/j.dsr2.2020.104838
Mjanger H., Svendsen B. V., Senneset H., Fotland Å., Fuglebakk E., Skage M. L., et al. (2019). Handbook for sampling fish, crustaceans and other invertebrates, version 5.0 (Bergen, Norway: Institute of Marine Research). 1–149.
Mychek-Londer J. G., Chaganti S. R., Heath D. D. (2020). Metabarcoding of native and invasive species in stomach contents of great lakes fishes. PloS One 15 (8), e0236077. doi: 10.1371/journal.pone.0236077
Naustvoll L. J., Melle W., Klevjer T., Drinkwater K. F., Strand E., Knutsen T. (2020). Dynamics of phytoplankton species composition biomass and nutrients in the north Atlantic during spring and summer - a trans-Atlantic study. Deep-Sea Res. Part II 180, 104890. doi: 10.1016/j.dsr2.2020.104890
Nilsson D.-E., Warrant E., Johnsen S. (2014). Computational visual ecology in the pelagic realm. Phil. Trans. R. Soc B 369, 20130038. doi: 10.1098/rstb.2013.0038
Norheim E., Klevjer T. A., Aksnes D. L. (2016). Evidence for light-controlled migration amplitude of a sound scattering layer in the Norwegian Sea. Mar. Ecol. Prog. Ser. 551, 45–52. doi: 10.3354/meps11731
Nunes C., Silva A., Soares E., Ganias K. (2011). The use of hepatic and somatic indices and histological information to characterize the reproductive dynamics of Atlantic sardine sardina pilchardus from the Portuguese coast. Mar. Coast. Fish. 3 (1), 127–144. doi: 10.1080/19425120.2011.556911
Olivar M. P., Hulley P. A., Castellón A., Emelianov M., López C., Tuset V. M., et al. (2017). Mesopelagic fishes across the tropical and equatorial Atlantic: Biogeographical and vertical patterns. Prog. Oceanogr. 151, 116–137. doi: 10.1016/j.pocean.2016.12.001
Pearre J. S. (2003). Eat and run? the hunger/satiation hypothesis in vertical migration: history, evidence and consequences. Biol. Rev. Camb Philos. Soc. 78 (1), 1–79. doi: 10.1017/s146479310200595x
Pepin P. (2013). Distribution and feeding of Benthosema glaciale in the western Labrador Sea: fish–zooplankton interaction and the consequence to calanoid copepod populations. Deep Sea Res. Part I: Oceanogr. Res. Pap. 75, 119–134. doi: 10.1016/j.dsr.2013.01.012
R Core Team (2021). R: A language and environment for statistical computing (Vienna Austria: R Foundation for Statistical Computing Vienna Austria). Available at: https://www.R-project.org/.
Robinson C., Steinberg D. K., Anderson T. R., Arístegui J., Carlson C. A., Frost J. R., et al. (2010). Mesopelagic zone ecology and biogeochemistry – a synthesis. Deep Sea Res. Part II 57, 1504–1518. doi: 10.1016/j.dsr2.2010.02.018
Roe H. S. J., Badcock J. (1984). The diel migrations and distributions within a mesopelagic community in the north East atlantic. 5. vertical migrations and feeding of fish. Prog. Oceanogr. 13, 389–424. doi: 10.1016/0079-6611(84)90014-4
Saba G. K., Burd A. B., Dunne J. P., Hernández-León S., Martin A. H., Rose K. A., et al. (2021). Toward a better understanding of fish-based contribution to ocean carbon flux. Limnol. Oceanogr. 66, 1639–1664. doi: 10.1002/lno.11709
Sameoto D. (1988). Feeding of lantern fish Benthosema glaciale off the Nova Scotia shelf. Mar. Ecol. Prog. Ser. 44, 113–129. doi: 10.3354/meps044113
Sameoto D. (1989). Feeding ecology of the lantern fish Benthosema glaciale in a subarctic region. Polar Biol. 9, 169–178. doi: 10.1007/BF00297172
Sameoto D. D., Jarozynski L. O., Fraser W. B. (1980). BIONESS, a new design in multiple net zooplankton samplers. J. Fish. Res. Board Can. 37, 722–724. doi: 10.1139/f80-093
Saunders R. A., Collins M. A., Stowasser G., Tarling G. A. (2017). Southern ocean mesopelagic fish communities in the Scotia Sea are sustained by mass immigration. Mar. Ecol. Prog. Ser. 569, 173–185. doi: 10.3354/meps12093
Scotto di Carlo B., Costanzo G., Fresi E., Guglielmo L., Ianora A. (1982). Feeding ecology and stranding mechanisms in two lanternfishes, Hygophum benoiti and Myctophum punctatum. Mar. Ecol. Prog. Ser. 9, 13–24. doi: 10.3354/meps009013
Song N., Liu M., Yanagimoto T., Sakurai Y., Han Z.-Q., Gao T.-X. (2016). Restricted gene flow for Gadus macrocephalus from yellow Sea based on microsatellite markers: Geographic block of tsushima current. Int. J. Mol. Sci. 17, 467. doi: 10.3390/ijms17040467
Steinberg D. K., Carlson C. A., Bates N. R., Goldthwait S. A., Madin L. P., Michaels A. F. (2000). Zooplankton vertical migration and the active transport of dissolved organic and inorganic carbon in the Sargasso Sea. Deep-Sea Res. I 47, 137–158. doi: 10.1016/S0967-0637(99)00052-7
St. John M. A., Borja A., Chust G., Heath M., Grigorov I., Mariani P., et al. (2016). A dark hole in our understanding of marine ecosystems and their services: Perspectives from the mesopelagic community. Front. Mar. Sci. 3. doi: 10.3389/fmars.2016.0003
Strand E., Klevjer T., Knutsen T., Melle W. (2020). Ecology of mesozooplankton across four north Atlantic basins. Deep-Sea Res. Part II 180, 104844. doi: 10.1016/j.dsr2.2020.104844
Strauss R. E. (1979). Reliability estimates for ivlev's electivity index, the forage ratio, and a proposed linear index of food selection. Trans. Am. Fish. Soc. 108, 344–352. doi: 10.1577/1548-8659(1979)108<344:REFIEI>2.0.CO;2
Suneetha K. B., Salvanes A. G. V. (2001). Population genetic structure of the glacier lanternfish Benthosema glaciale (Myctophidae) in Norwegian waters. Sarsia 86, 203–212. doi: 10.1080/00364827.2001.10420476
Themelis D. E., Halliday R. G. (2012). Species composition and relative abundance of the mesopelagic fish fauna in the slope Sea off Nova Scotia. Northeastern Nat. 19 (2), 177–200. doi: 10.1656/045.019.0204
Turner J. R., White E. M., Collins M. A., Partridge J. C., Douglas R. H. (2009). Vision in lanternfish (Myctophidae): Adaptations for viewing bioluminescence in the deep-sea. Deep Sea Res. I 56 (6), 1003–1017. doi: 10.1016/j.dsr.2009.01.007
Vestheim H., Kaartvedt S., Edvardsen B. (2005). State-dependent vertical distribution of the carnivore copepod pareuchaeta norvegica. J. Plankton Res. 27 (1), 19–26. doi: 10.1093/plankt/fbh144
Vogedes D., Varpe Ø., Søreide J. E., Graeve M., Berge J., Falk-Petersen S. (2010). Lipid sac area as a proxy for individual lipid content of arctic calanoid copepods. J. Plank. Res. 32 (10), 1471–1477. doi: 10.1093/plankt/fbq068
Wahl T. (1984). Observations on the diving behavior of the northern fulmar. West. Birds 15, 131–133.
Wickham H., Averick M., Bryan J., Chang W., McGowan L. D., François R., et al (2019). Welcome to the tidyverse. J. Open Source Softw. 4 (43), 1686. doi: 10.21105/joss.01686
Wiebe P. H., Allison D., Kennedy M., Moncoiffé G. (2015). A vocabulary for the configuration of net tows for collecting plankton and micronekton. J. Plankton Res. 37 (1), 21–27. doi: 10.1093/plankt/fbu101
Wiebe P. H., Morton A. W., Bradley A. M., Backus R. H., Craddock J. E., Barber V., et al. (1985). New development in the MOCNESS an apparatus for sampling zooplankton and micronekton. Mar. Biol. 87, 313–323. doi: 10.1007/BF00397811
Keywords: Benthosema glaciale, feeding ecology, North-Atlantic distribution, diet, mesopelagic fish, open ocean, high latitude
Citation: Knutsen T, Strand E, Klevjer TA, Salvanes AGV, Broms C, Sunde SM, Aksnes DL, García-Seoane E and Melle W (2023) Feeding ecology of Benthosema glaciale across the North Atlantic. Front. Mar. Sci. 10:1086607. doi: 10.3389/fmars.2023.1086607
Received: 01 November 2022; Accepted: 26 January 2023;
Published: 16 February 2023.
Edited by:
Ricardo Serrão Santos, University of the Azores, PortugalReviewed by:
Atsushi Yamaguchi, Hokkaido University, JapanFilipe Porteiro, University of the Azores, Portugal
Copyright © 2023 Knutsen, Strand, Klevjer, Salvanes, Broms, Sunde, Aksnes, García-Seoane and Melle. This is an open-access article distributed under the terms of the Creative Commons Attribution License (CC BY). The use, distribution or reproduction in other forums is permitted, provided the original author(s) and the copyright owner(s) are credited and that the original publication in this journal is cited, in accordance with accepted academic practice. No use, distribution or reproduction is permitted which does not comply with these terms.
*Correspondence: Tor Knutsen, dG9yLmtudXRzZW5AaGkubm8=
†Deceased
‡ORCID: Tor Knutsen, orcid.org/0000-0003-3531-5611