Decreased feeding rates of the copepod Acartia tonsa when exposed to playback harbor traffic noise
- 1Research and Technology Centre West Coast, Kiel University, Büsum, Germany
- 2Institute of Evolution and Ecology, University of Tübingen, Tübingen, Germany
Introduction: Copepods present the largest and most diverse group of zooplankton and their feeding behavior can affect top-down and bottom-up processes. Thus, how efficient feeding is executed determines the abundance of copepods’ prey and their predators and, with that, carbon transfer and storage in ecosystems. The rise of anthropogenic underwater noise from shipping, oil exploration and exploitation, wind farm construction and operation, and more, is increasingly changing the marine acoustic environment. This acoustic pollution can have detrimental effects on biological life. Studies on this topic increasingly indicate that anthropogenic underwater noise adversely affects primary producers, marine mammals, fish, and invertebrates. However, little data exist on the effects of anthropogenic underwater noise on the feeding behavior of zooplankton.
Methods: Here, we investigated the ingestion and clearance rates of the copepod Acartia tonsa on a motile phytoplankton as a function of prey density under ambient aquarium sound conditions and, when exposed to playback, harbor traffic noise.
Results: We measured significantly decreased ingestion rates and clearance rates of A. tonsa when exposed to harbor noise compared to ambient conditions. The negative impact of noise on the ingestion rates was found at all given phytoplankton cell densities between 1k to 10k cells ml−1. Clearance rates were fitted to the Rogers random predator equation which revealed significantly decreased capture rates on phytoplankton under the exposure of harbor noise while handling times remained the same in both sound treatments.
Discussion: Our results call for follow-up studies to focus on noise driven community-effects in field experiments to confirm laboratory results and to predict the outcome of a changing world with multiple stressors. Further, the underlying mechanism on how noise affects the feeding behavior of copepods is still unknown. Noise may distract copepods or mask hydromechanical cues of the prey. Noise may also adversely affect copepod physiology or morphology that would lead to changes in the feeding behavior. All potential mechanisms need to be investigated rigorously in future experiments.
Introduction
Research on the feeding ecology of key species is essential to predict human impacts on natural dynamics linked to trophic energy transfer within and between ecosystems. This information is crucial for the integration of strategies that lead to and protect a good environmental status of the marine environment (Directive 2008/56/EC). Crustacean zooplankton, especially copepods, are of exceptional importance due to their linkage between primary production and higher trophic levels. Hence, the magnitude of grazing and predation has direct effects on the community structure of phytoplankton and other planktonic animals as well as (in-)directly on bottom-up carbon transfer (Turner, 2015; Lynam et al., 2017; Steinberg and Landry, 2017). Human activities have led to climate warming and ocean acidification in combination with various pollutants that are continuously added to the oceans (Doney et al., 2012). Those environmental stressors have the potential to affect copepod species abundances and impede top-down and bottom-up planktonic food web structures (Garzke et al., 2016; Cole et al., 2019; Moreno et al., 2022).
In copepod feeding ecology, one pollutant has so far been overlooked even though it has become a major topic in science and politics (Directive 2008/56/EC; Duarte et al., 2021). The increase of anthropogenic underwater noise in marine ecosystems through construction work, energy exploration and exploitation, and ship traffic (Duarte et al., 2021; Jalkanen et al., 2022) is motivating studies to unravel the impacts of this acoustic pollution across sensory modality-based processes in a variety of marine animals (Halfwerk and Slabbekoorn, 2015). The reason for the growing attention is that noise-related effects may have the potential to change the composition of communities and, in turn, compromising essential ecosystem functions through masking and altering morphology, physiology, and behavioral processes in various taxa from primary producers, to small invertebrates to large marine mammals (Erbe et al., 2019; Murchy et al., 2020; Duarte et al., 2021; Solé et al., 2021a).
Shipping, as the main source of continuous underwater noise in the North Sea, can lead to an increase in noise levels of more than 30 dB above the natural ambient sound, especially in coastal areas (Farcas et al., 2020; Kinneging and Tougaard, 2021). Crustaceans, including copepods, produce sound (Tolstoganova, 2002; Jézéquel et al., 2018; Jézéquel et al., 2019; Kühn et al., 2022) and detect and react to hydromechanical disturbances perceived through sensory hair structures (Fields, 2014; Lenz and Hartline, 2014). This mechanoreception is a crucial sensory mechanism for copepod inter- and intraspecific interactions i.e. in feeding, mating, and predator avoidance (Yen and Strickler, 1996; Fields, 2014). In order for a copepod to perceive a fluid signal, these sensory structures, setae located on antennules, must be “moved” (10 nm bend; see Yen et al., 1992) remotely via vibrations and other fluid disturbances inducing “suspicious” fluid velocities and velocity gradients (Yen et al., 1992; Kiørboe et al., 1999) or, potentially, through strong pressure changes (Yen and Okubo, 2002). Some copepods are highly sensitive to vibration frequencies, from 40 Hz to 1 kHz (Yen et al., 1992), that fall in the frequency range of continuous underwater noise (10 Hz to > 10 kHz; Duarte et al., 2021). There is, to our best knowledge, however, no study that investigates the stimulus sound and how its different compounds are perceived by copepods.
Nevertheless, there is an increasing number of studies on the effects of noise on crustaceans: In benthic species, continuous underwater noise altered feeding, predator-avoidance, camouflage (Wale et al., 2013; Carter et al., 2020; Leiva et al., 2021), mating, and metabolic rates (Ruiz-Ruiz et al., 2020). Studies focused on crustacean meroplankton, showing no and negative effects of continuous noise on parameters related to swimming, development, and settlement (e.g. Pine et al., 2012; Sal Moyano et al., 2021). Previous studies on the effects of continuous anthropogenic underwater noise on marine crustacean holoplankton found significant physiological and morphological impacts (Solé et al., 2016; Tremblay et al., 2019; Solé et al., 2021b) from which only two investigated marine copepods (Tremblay et al., 2019; Solé et al., 2021b; see also review Vereide and Kühn, 2023). Further investigations of the effects of anthropogenic underwater noise on copepod behavior are therefore needed.
In the present study we experimentally tested the hypothesis that shipping noise alters the feeding response of the pelagic copepod, Acartia tonsa, on phytoplankton compared to ambient sound conditions. To do so, we investigated the effect of noise at different prey densities. In general, it is known that copepod feeding behavior depends on prey cell density (Frost, 1972), and the effect of environmental stressors varies between different prey densities (see van Dinh et al., 2019 for copepods and Fulfer and Menden-Deuer, 2021 for dinoflagellates). With common functional response equations (Holling, 1959; Rogers, 1972; Abrams, 2022), it is possible to investigate the effect of prey density and noise on the capture rates and handling times in copepods. The capture rate describes the rate at which the consumer encounters and detects prey items per unit of prey density. The handling time is the time spent to process the prey item (Holling, 1959; Rogers, 1972). These models predict that with increasing prey density, the probability of encountering and detecting a prey item is increasing, leading to a decrease in searching and detection time, while handling, feeding, and digestion remain the same (Holling, 1959). Little is known about the effect of underwater noise on copepod foraging efficiency and whether this would be density-dependent. Noise pollution may affect prey encounter, detection, and handling. We predict the effects of underwater noise on copepod feeding may be more pronounced at higher prey densities because of the increased number of prey encounters (Holling, 1959) on which noise can have an effect. In the present study, copepods were fed with green algae and exposed to playbacks of shipping noise from a harbor traffic underwater recording while a control group was incubated under ambient aquarium sound conditions. We quantified the effect of prey cell density on the ingestion and clearance rates in both groups at the end of the experiment. These results will be of relevance for discussions on the inclusion of noise in predictions on future zooplankton-based food web dynamics in a world with multiple stressors (Pirotta et al., 2022).
Methods
Study location
Feeding experiments were performed in the laboratory in September 2021 at the Research and Technology Centre West Coast (FTZ) in Büsum, Kiel University, Germany. Experimental copepods were caught in an artificially built lagoon in Büsum (54°08’01.78”N 8°50’32.11”O) with access to the Wadden Sea but without tidal influences.
Experimental organisms
The pelagic copepod Acartia tonsa (0.5–1.5 mm length) can be found year-round in coastal and estuarine environments at high biomasses (Brylinski, 1981). Acartia sp. use mechanoreception for feeding (DeMott and Watson, 1991; Gonçalves and Kiørboe, 2015) and are sensitive to low-frequency vibrations (≤ 1000 Hz; Yen et al., 1992), which makes it, in addition to its trophic role in ecosystems, the optimal model species for the present noise study. As prey, we used small (< 20 µm) highly motile phytoplankton instead of micro-zooplankton, for instance, ciliates, to exclude potential effects of noise on the escape behavior of small prey animals. The chosen phytoplankton prey, Tetraselmis chuii, is a genus of green algae within the order Chlorodendrales, characterized by a flagellated cell body. Species of this genus are found in both marine and freshwater ecosystems around the world including the German Wadden Sea and are widely used in copepod feeding experiments (Thor et al., 2002; Scholz and Liebezeit, 2012). T. chuii were cultivated and provided by BlueBioTech GmbH, Büsum.
Collecting copepods
A. tonsa were caught with four light traps (see Kühn et al., 2022) deployed for 1–2 h during dark hours (21:00–23:00). The light traps were positioned at 20–50 m distance to the shore towards the center of the lagoon and positioned on the bottom (1.4 m depth). At this location, there is no direct input of underwater noise through boat traffic and pilot sampling showed a permanent high catching success of A. tonsa individuals. Animals caught in the traps were carefully poured into two cooling boxes where they were maintained (<2 h) until selected in the laboratory for experimental acclimatization. A new population sample of A. tonsa was caught for every experimental day. Additionally, the ambient sound of the lagoon was recorded with a SoundTrap-HF [sampling rate: 96 kHz; calibration Information; End-to-End: 176.3 dB (High); RTI Level @ 1kHz 135.4 dB re 1 μPa; Ocean Instruments, Auckland, New Zealand].
Feeding experiment
The caught copepods were brought to the laboratory and anesthetized with 15 g L−1 (sea water) magnesium chloride (adapted from Isinibilir et al., 2020). The animals stopped moving after 1–10 min (high individual variations, pers. obs. SK and FK) and were sorted into groups of roughly same-sized, copepodite, and adult A. tonsa stages, irrespective of sex, under a stereomicroscope (Stemi 508, Carl Zeiss Microscopy GmbH). Nauplii stages were excluded from the experiments. The number of A. tonsa in experimental grazing plastic vials (110 ml Kautex wide neck PET containers) varied slightly with prey density (Table 1) and availability (number of copepods caught in light traps also depended on weather conditions and fluctuations in local natural density). In addition, the number of copepods as grazers was determined to ensure a reduction of optimally 40–50% in phytoplankton cell concentration under ambient sound conditions conservatively enabling the detection of measurable effects, if any, that could go both directions, i.e. decreased or increased feeding rates. Seven to 19 A. tonsa individuals per experimental unit were put together (Table 1), in Eppendorf tubes filled with purified (filtered, UV-treated, and ozonized) North Sea water (provided by IMTE, Büsum). The copepods were checked after 30 min and only those that displayed normal swimming behavior were selected for the experimental runs. This was tested by looking for an increased jumping behavior when triggered with white light while being in the Eppendorf tubes to reduce handling stress. Dead or non-normal swimming copepods (< 5%, pers. obs.) were sorted out. Copepods selected for the experiment were then put in Eppendorf tubes without lids (13 ml) closed with a mesh net (5 µm) and acclimatized for 12 h in the experimental aquarium in the dark without food. The aquarium (100 * 50 * 30 cm) was filled with 130 L purified North Sea water (provided by IMTE) that was filtered continuously. Water temperature, oxygen, and salinity were kept constant at 18 ± 0.2 °C, 9.5 mg L−1 ± 0.1, and 36 mS cm−1 ± 0.1, respectively. An overview of sample preparation, experimental design, and work flow is depicted in Figure 1. The experiments took place in a shed located around 100 m away from the main building of the FTZ to exclude low-frequency building sound during the experiments. The experimental room was additionally equipped with sound absorption material (molded pulp egg-texture cartons) on the walls. Further, similar to a setup used by Amorim et al. (2013), we reduced the influence of ground vibrations by placing the aquarium onto a box (120 * 80 * 16 cm) that was filled with a combination of fine and coarse sand up to the top. A marble slate (120 * 80 * 3 cm) was placed onto that box with 10 equally distributed circular rubber studs (6 cm in diameter and 3 cm in height) on which the aquarium was placed. The whole setup was standing on a wagon with rubber wheels.
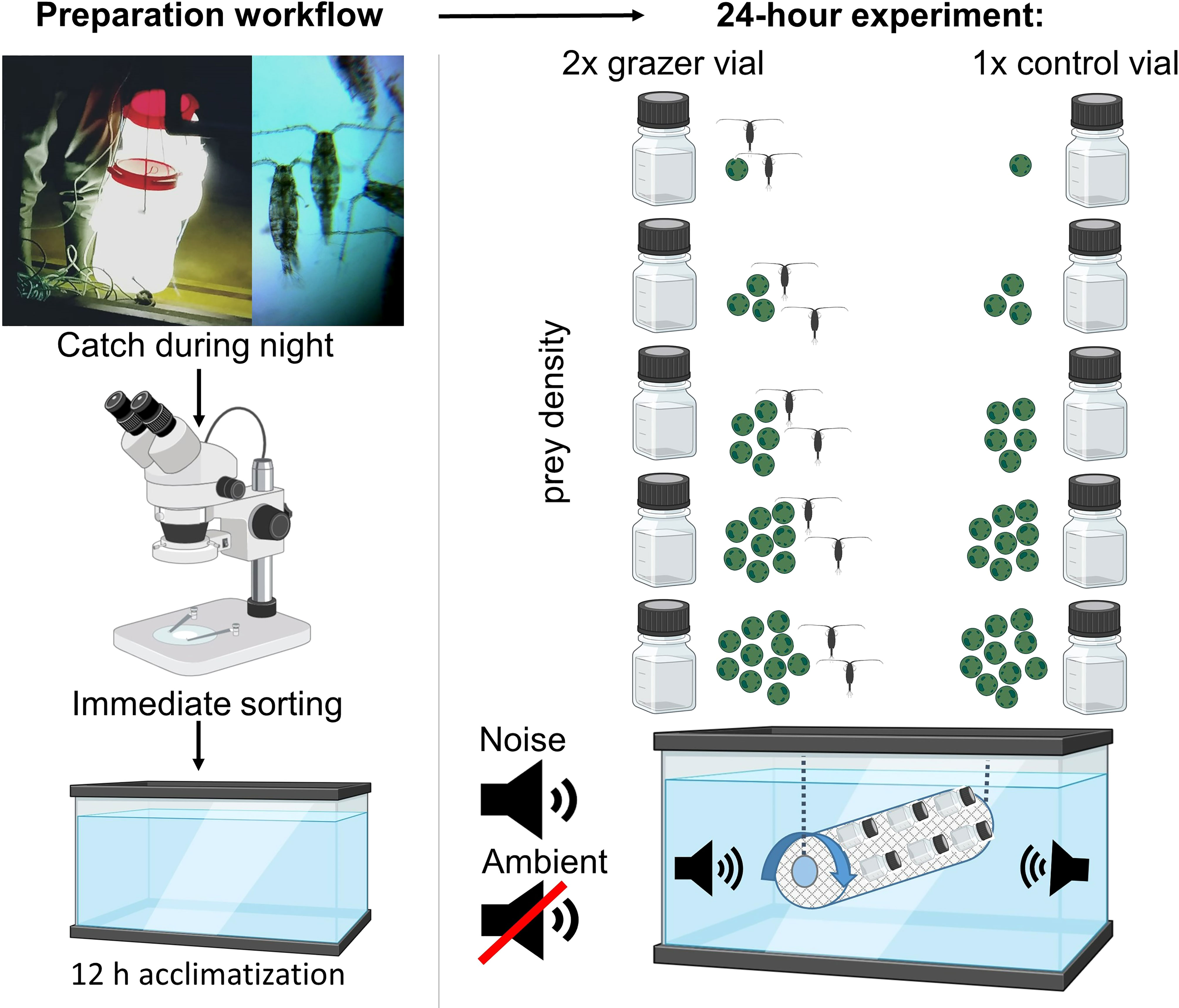
Figure 1 Overview Experimental Setup. Left column: preparation workflow. Capture of copepods Acartia tonsa using light traps at night. Immediate sorting under the stereoscope, and 12 h acclimatization in the dark. Right column: experimental design. Preparation of five different phytoplankton cell concentrations (1000–10000 cells ml−1) using Tetraselmis chuii as prey and filled in experimental vials: control vials and grazer vials. Groups of copepods were then added to the grazer vials after Almeda et al. (2018). Both control and grazer vials with all different cell concentrations were then mounted to an underwater phytoplankton wheel and incubated for 24 hours exposed to either ambient sound or harbor noise playbacks. Partly created with BioRender.com.
After acclimatization, we obtained the functional response curves by quantifying the ingestion and clearance rates through vial incubations. For this, T. chuii was filtered through a 50 μm plankton mesh net to remove cell aggregates. The experimental prey densities (1000, 3000, 5000, 8000, and 10000 cells ml−1) were prepared by diluting the start prey cell density with purified seawater and amending it with 40 μl 110 ml−1 growth medium (provided by BlueBioTech GmbH) to avoid variations in phytoplankton growth between vials. For each phytoplankton cell concentration (prey density level) prepared, we also set aside and preserved vials for later confirmation of estimated initial cell concentrations while preparing the different prey density levels by adding 40 µl of Lugol solution (15 g KI + 500 ml dest H2O + 10g I2) (Almeda et al., 2018). Information on variations in the initial cell concentrations for the different densities can be found in Table S1 in the Supplementary Material. Grazer vials, each equipped with a group of copepods, were either exposed to ambient aquarium sound or to playback of harbor traffic noise. The days with runs alternated between ambient and noise treatments to ensure experimental copepods would have been exposed to similar environmental Wadden Sea conditions prior to being tested. Further, all five levels of different prey densities were tested simultaneously within a run. On each experimental day, one baseline control vial per prey density level was also included in the run to check for baseline phytoplankton mortality or growth in the absence of grazers during incubation. One to two experimental grazer vials on each experimental day were included in the incubation run to obtain the feeding rates of the copepods for all respective different prey density levels either exposed to ambient aquarium sound or to playback harbor traffic noise (Figure 1, Table 2). We consider each vial community as an experimental unit. Copepods are known to feed with abnormally high rates in the beginning of a feeding experiment because of starvation or handling stress (Mullin, 1963 and emphasized in Frost, 1972). Therefore, each run of baseline control vials and experimental grazer vials was incubated for 24 h. Additionally, we conducted the experiments in the dark to ensure constant nocturnal feeding conditions throughout the incubation (see Stearns, 1986). In all experimental grazer vials, copepods were allowed to graze down the suspensions without phytoplankton prey cell replacement.
We built a slowly rotating underwater plankton wheel for continuous phytoplankton mixing in the experimental vials. Pictures of the plankton wheel can be found in Figure S1 in the Supplementary Material. All experimental control and grazer vials were prepared with a window of 3.5 cm diameter fitted with a 5-µm mesh net to ensure exchange with the surrounding aquarium water. The plankton wheel was made of a stainless-steel circular wire frame (35 cm length, 8.5 cm width, and 21 cm width with added vials) onto which a matrix of three by five stainless-steel baskets were mounted. This was done for easy mounting and removing of experimental vials onto and from the plankton wheel. To each of the five columns, one prey density level had been assigned while within each column, the baskets in rows (three rows per column) were randomly assigned to control and experimental grazer vials within respective prey densities. The wheel was placed in the middle of the aquarium’s water column (4.5 cm of water below and above the vials) and was rotated by a synchronous AC 12 V Motor (CHANCS Motor, TYC-50; 1 rpm) connected to the plankton wheel via gear wheels and closed timing belts. An overview of the experimental setup and workflow is summarized in Figure 1 and Table 2.
Sound exposure
The described workflow for the feeding experiments was conducted under ambient aquarium sound conditions and under the exposure of playback harbor traffic noise (Figure 1). Harbor traffic noise was recorded at the Büsum port (54°07’20.63”N 8°51’32.67”E) on June 8, 2021. The underwater sound recordings were done with an AS-1 hydrophone (Nauta Scientific, Milano; (cross-calibrated at the FTZ) sensitivity: −211 dB re 1V/µPa) that were connected with a P48 hydrophone preamplifier (26 dB gain) to a Zoom H5 Handy recorder (gain=3). The whole setup was calibrated at 1 kHz (sensitivity of −188 dB re 1V/µPa). The harbor noise recording reflects a typical composition of harbor traffic with consecutive passings of a small shrimp trawler, a foot passenger ferry, a former larger trawler now used by an operator for guided nature trips, and a sailboat, with a total duration of 20 ' 11". The first boat passed after 1’36” for 38 s. The second boat passed at 8’40” (49 s). The third one passed from 14’34” to minute 15’14” (40 s) of the recording and Boat 4 passed at 17’42” (60 s) (Figure 2A). All boats on the recording (sampling rate: 48 kHz) were passing at a distance of 10–15 m from the hydrophone. The hydrophone was positioned ~10 cm off the harbor wall at 1 m depth. The original recording was played back in the experimental aquarium with two UW30 underwater speakers (Electro-Voice; frequency response 0.1–10 kHz) connected to a self-designed mobile waterproof audio-suitcase amplifier (MAK2x30/4, Bela P. Event-Studiotechnik) that was connected to a laptop. The original sound file was played via Audacity (Version 2.3.0). For comparison of actual harbor noise and its representation in the laboratory, we played back the harbor traffic noise file using the section capturing the first boat passing and recorded it in an experimental vial mounted onto the plankton wheel at a fixed position with a SoundTrap-HF (calibration Information see above; sampling rate: 96 kHz). Finally, the distance of the plankton wheel to the two UW30 speakers and the gain that was added to the original recording for the exposure were both decided upon the playback sound’s similarity towards the original harbor recording. We analyzed the sound recordings in SpectraPlus-SC (V 5.3.0. 12A, Pioneer Hill Software LLC, Sequim, WA, USA) for frequency and power spectral density (PSD) characteristics visualized in spectra and spectrograms [FFT size: 16384 (for sampling rates of 48 kHz) and 32768 (for sampling rates of 96 kHz); Hanning window, 0.5 overlap]. For comparison, the root mean square (rms) power levels were calculated in SpectraPlus-SC from the PSD spectral data of the different sound recordings (Figure 2B). Based on analysis of original recordings and recordings of playbacks in the laboratory, we decided on the following sound exposure setup. One UW30 underwater speaker was placed on both sides along the plankton wheel with a distance of 15 cm to the experimental vials maintaining the noise exposure during the plankton wheel rotation on the right and on the left side. This recording of harbor noise was then played back in an infinite loop from the start to the end of each experimental exposure day via Audacity (Version 2.3.0).
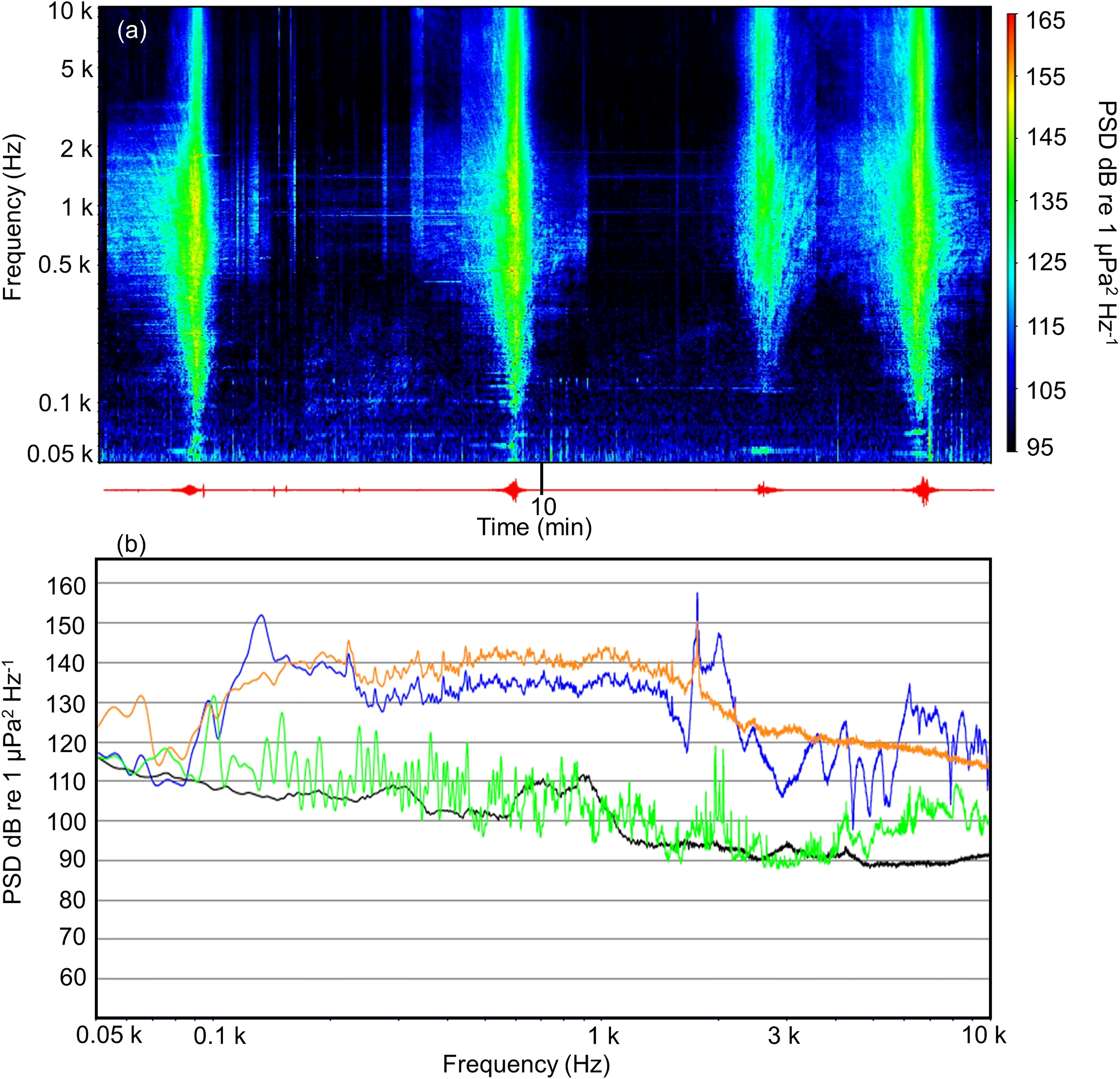
Figure 2 Sound exposure. (A) Spectrogram from the original harbor traffic noise recording. The left y-axis shows the frequency in Hertz. The right y-axis presents the color code for the power spectral density (dB re 1 µ Pa2 Hz−1). The x-axis presents the recording as time series in minutes (red line). (B) Spectrum of the first boat (see (A) 1’36” to 2’14”) passing the hydrophone in the original recording (orange line) and the exposure sound measured in the aquarium in the experimental beaker (blue line). The green line shows the ambient aquarium sound spectrum. The black line represents the ambient field sound from the copepod catching site. All samples had a length of 38 s. The figures are presented in a Hanning window (50% overlap), frequency resolution is 3 Hz, visualized in SpectraPlus-SC (V 5.3.0. 12A).
The spectrogram of the measured original harbor noise recording (Figure 2A) and the spectra of the measured exposures in the aquarium versus harbor noise and ambient sound measured in the field (Figure 2B) are presented. To compare original recordings with its representation as experimental stimuli, Figure 2B shows the spectrum of the harbor noise at the time of the first boat passing (38 s) from the original harbor recordings (173 dB re 1 µPa2 Hz−1) in comparison to the recordings of the playbacks made in the vial in the aquarium (174 dB re 1 µPa2 Hz−1), the ambient aquarium sound treatment (155 dB re 1 µPa2 Hz−1), and the ambient sound in the artificial lagoon (144 dB re 1 µPa2 Hz−1). Note that ambient lagoon sound levels as recorded at the place of copepod origin are lower than ambient sound conditions in the laboratory that included potential noise from the water filter and the plankton wheel motor noise (Figure 2B). For boat noise in the original and in the playback recording, most energy was found between 100 and 2000 Hz. We are fully aware of the fact that the playback is not reflecting true harbor noise with shipping traffic found in the field. In an aquarium setup, the experimental sound exposures will divert from the real frequency-sound level distribution due to aquarium wall reflection, aquarium vibration, and the size of the aquarium that does not allow full soundwave cycles for low frequencies. Further limitations of the acoustic setup are pointed out in the discussion.
After the incubation
The feeding incubation was ended after 24 hours (except one case that ended after 28 h) by gently taking out each experimental vial from the aquarium plankton wheel. Each grazer vial was then visually checked for swimming activity. Here, individuals were noted as “not active” or “active”. A copepod was assigned and noted “not active” when there was no movement or only sinking after a few seconds when triggered with white light. Lugol solution 40 µl (15 g KI + 500 ml dest H2O + 10 g I2) was then added to the baseline control and grazer vial to preserve copepods and phytoplankton before taking out the next one. The plankton wheel was set on hold, and for the noise treatment, the playback was stopped only after all vials were taken out, preserved, and stored in a cooling box. In the laboratory, we counted the initial, baseline control and grazer prey cell concentration using a Fuchs-Rosenthal counting chamber (area: 16 mm2, depth: 0.2 mm, volume: 3.2 μl) under microscopes (100x; Zeiss Axioscope and Leitz Aristoplan). After counting, copepods were removed from the vial and their prosome length (µm) was measured under a microscope using a calibrated Moticam X3 Plus laboratory camera (Software Motic Images Plus Version 3.0.19.108b) attached to a microscope (50 x; Leitz Aristoplan). Sizes were only noted for intact (not damaged) animals. Clearance rates (volume swept clear from prey cells in ml copepod−1 h−1) and ingestion rates (ingested prey cells h−1 copepod−1) were calculated after Frost (1972).
Data and statistical analysis
Descriptive statistics are presented as mean ± standard error if not stated otherwise. The cell concentrations between 1K and 10K cells ml−1 were decided on being treated as a categorical fixed factor with five levels of prey cell concentrations (PC). However, choosing a regression model with cell concentrations as a continuous variable led qualitatively to the same results.
Statistics were performed in R version 3.4.4 (R Core Team, 2022). Figures for data presentation are based on the package ggplot2 (Wickham, 2016).
Mixed-effect model for ingestion rates
A linear mixed effect model (function lmer in package lme4; Bates et al., 2015) was fitted on the obtained data based on results per experimental vial community. We decided on using a mixed model with varying intercept per day to account for potential variation in the copepods that were caught daily from a certain population in the field. Hence, we included the ingestion rate of A. tonsa as the response variable and included the fixed effects of underwater noise treatment (A.N., noise vs. ambient), prey cell concentration (PC, five levels), mean body size per vial (MS, centered), number of copepods per vial (D, integer), and the number of animals “not active” after the incubation (S, integer). In addition to these fixed effects and continuous variables, we included day as a random factor to account for differences in general conditions among different experimental runs. We stepwise selected the optimal model with all reasonable combinations of the fixed variables (see Zuur et al., 2009) based on the lowest Akaike’s information criterion (AIC) with the fewest parameters (ΔAIC < 2; Burnham and Anderson, 2002). Note that p-values were computed using Kenward-Roger standard errors and df (package: jtools, Long, 2022).
Clearance rates feeding response curves
Clearance rates were fitted to the Rogers random predator model as it accounts for prey depletion over time (Rogers, 1972). For this, we used the gnls function in R (package nlme; Pinheiro et al., 2022), in order to get the coefficient estimates for the ambient sound and harbor traffic noise treatment, and integrated the following equation:
Ne is the number of prey items eaten, N0 is the initial number of prey, a is the capture rate, and h and T are handling time and the incubation time, respectively. In order to receive the estimates for the clearance rates’ coefficients a and h, this equation was divided by the initial number of prey (N0) (similar to Hollings disk equation calculations in Schultz and Kiørboe, 2009). Note that the number of prey items eaten (Ne) is found on both sides of this equation which is solved by applying the Lambert W function (Bolker, 2008).
Results
In total, 51 experimental runs – 26 ambient and 25 noise treatment vial communities – were analyzed (Table 2). A total of 688 copepods were either exposed to boat noise playbacks (343 copepods) or to ambient aquarium sound (345 copepods). The mean length of all measurable (see methods) A. tonsa was 647 µm ± 2 µm (n = 602) and width was 204 µm ± 3 µm (n = 235). For within-vial length – width size distributions see Table S2 in the Supplementary Material.
The prey algae Tetraselmis chuii ranged in length from 8 μm to 16 μm (normal distributed; mean = 12 μm ± 0.2; n = 80) and in width from 7 μm to 12 μm (normal distributed; mean = 9 μm ± 0.1; n = 80). In the control vials, which maintained prey algae without copepods, there was no significant difference in growth or mortality between incubation in ambient and noise treatments (Welch’s t-test, n = 26, df = 23.97, t = −0.49, p = 0.631).
Feeding rates
The experimental results on the copepods’ ingestion rates (y-axis) at increasing prey densities from 1000 to 10000 cells ml−1 (x-axis) when exposed to ambient aquarium sound and playback harbor traffic noise are shown in Figure 3. Mean ingestion rates decreased by 48%, 24%, 64%, 48% and 29% at prey densities of 1000, 3000, 5000, 8000, and 10000 cells ml−1, respectively, when exposed to playback harbor traffic noise compared to ambient sound conditions. In both sound treatments, ingestion rates increased with increasing prey density (Figure 3, Table 3). The optimal AIC-based model structure that describes the ingestion rates pattern is shown in Table 4 in bold (neach model = 49, df = 8, AIC = 681, delta = 0.36). It was found that a combination, not an interaction, of the fixed variables sound treatment (A.N.) and density of prey cells ml−1 (PC) described the obtained data from the feeding experiments best. Mean copepod lengths per vial (MS), the density of copepods per vial (D), and the number of animals “not active” in the end of the experiments (S) were not included in the optimal model. The optimal model (R² marginal = 0.76 and R² conditional = 0.77) shows that increasing prey densities (PC) from 1000, over 3000, 5000, 8000, to 10000 cells ml−1, significantly increased copepod ingestion rates (Table 5, Figure 3). Specifically looking into the sound treatments, the model estimated a significant decrease by 330 ingested cells hour−1 copepod−1 when exposed to harbor noise compared to ambient aquarium sound conditions (LMM, n = 51, df = 3.92, t = −4.26, p < 0.010).
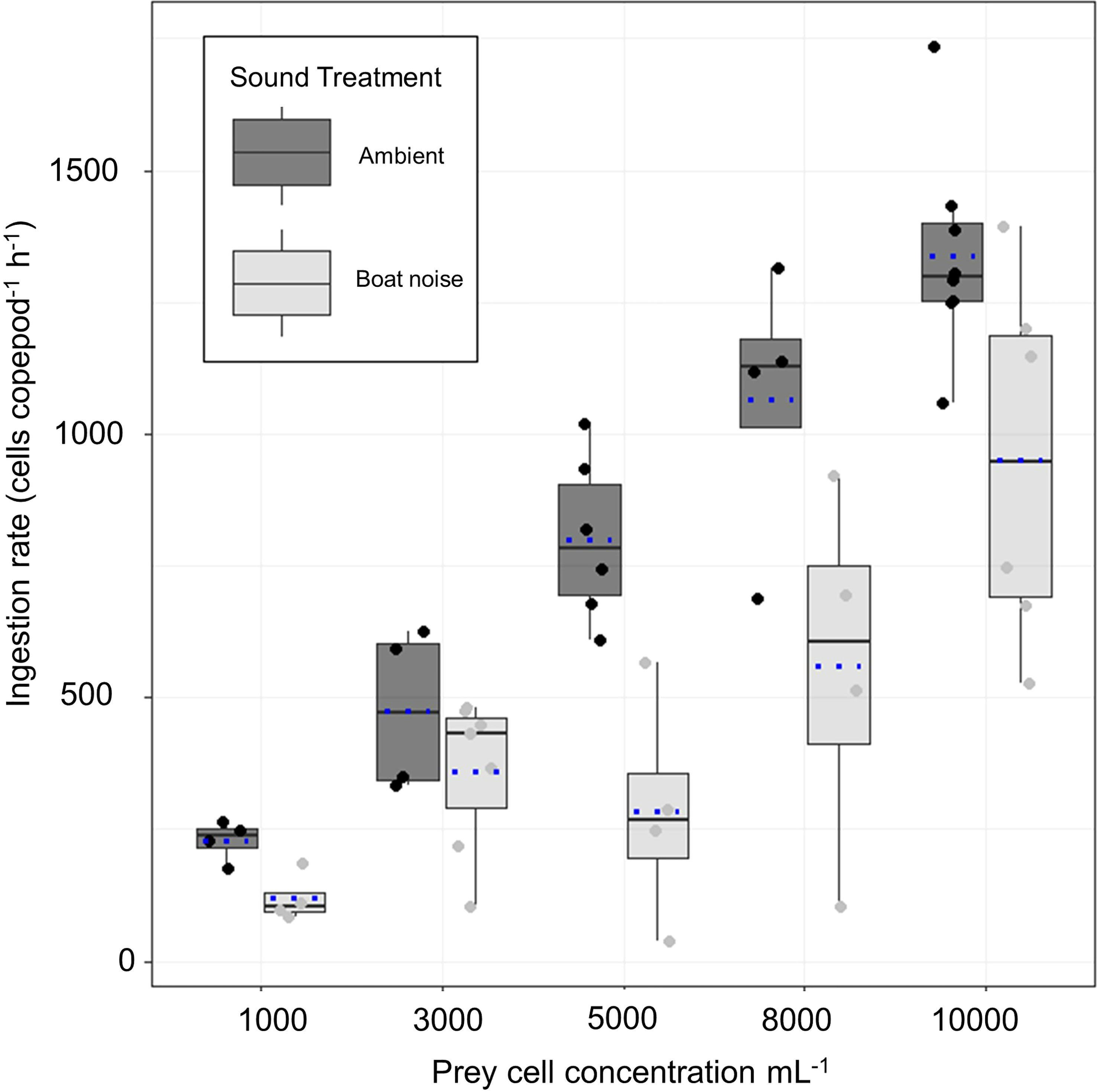
Figure 3 Ingestion rates under ambient aquarium sound and harbor noise conditions. The x-axis shows the initial phytoplankton prey cell concentrations from the categories 1000 to 10000 cells ml−1. The y-axis presents the ingestion rates that are defined as the number of phytoplankton cells consumed per individual copepod per hour (cells copepod−1 h−1) calculated after Frost (1972). The boxplots are drawn from the first to the third quartile with a black horizontal line denoting the median and a blue dashed line denoting the respective mean. The whiskers of the plots reaching to the lowest and highest values that is within 1.5 interquartile range. The jittered dots are presenting all values in the data set. Box plots shaded in grey and corresponding dots represent ingestion rates obtained under ambient sound conditions, while light grey colored boxes show ingestion rates when exposed to underwater harbor noise.
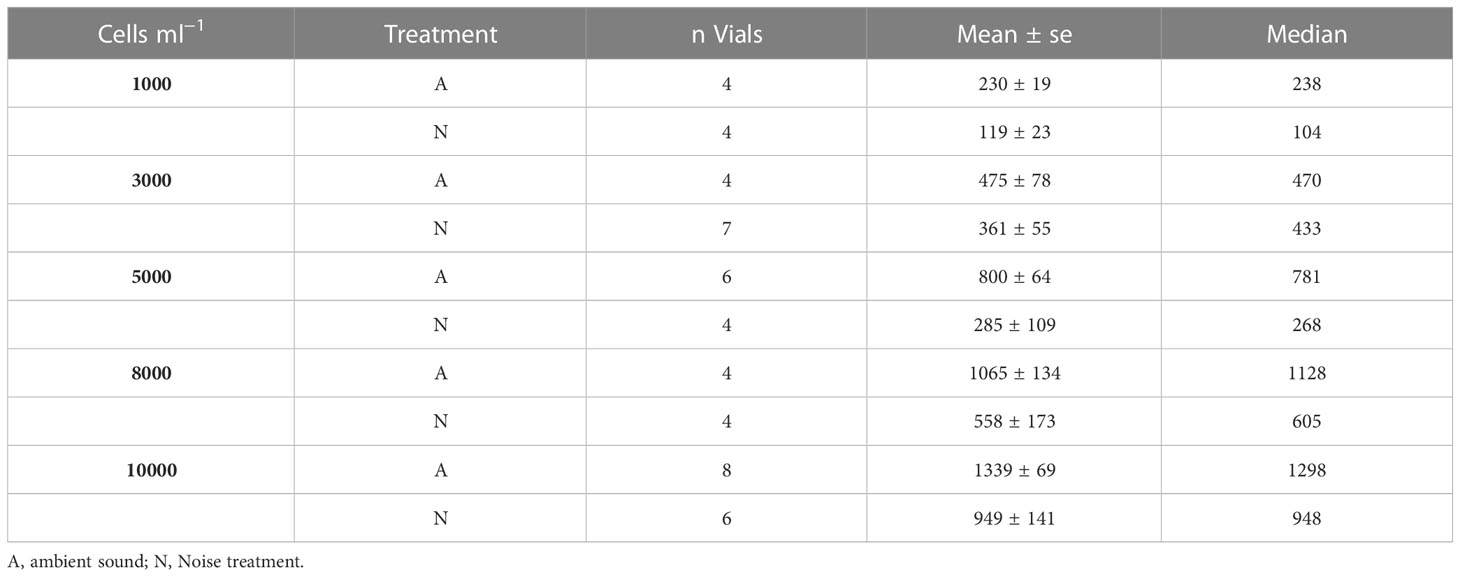
Table 3 Overview descriptive statistics of the calculated ingestion rates (cells copepod−1 h−1) after Frost (1972).
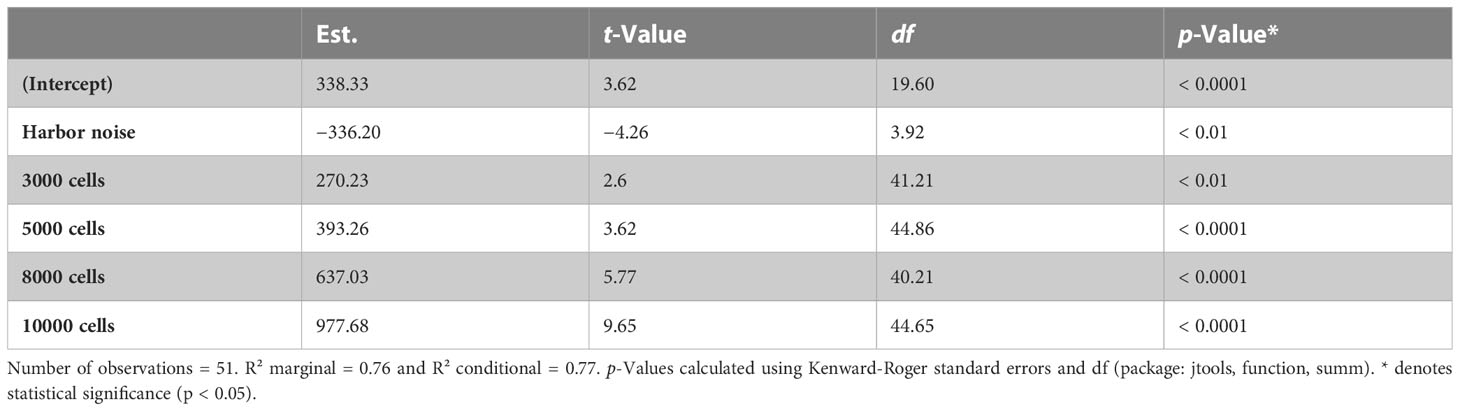
Table 5 Final optimal model summary of estimated noise treatment and prey density effects on copepod ingestion rates.
Figure 4 shows the clearance rates with increasing prey density. For the ambient sound conditions, the estimated capture rate a was 0.013, which significantly decreased by 0.008 under the exposure of harbor traffic noise (GNLS; n = 51, df = 47, t = −3.7, p < 0.001). There was no significant difference in handling time h between ambient (estimate 0.005) and harbor noise conditions (GNLS; n = 51, df = 47, t = −0.12, p = 0.9).
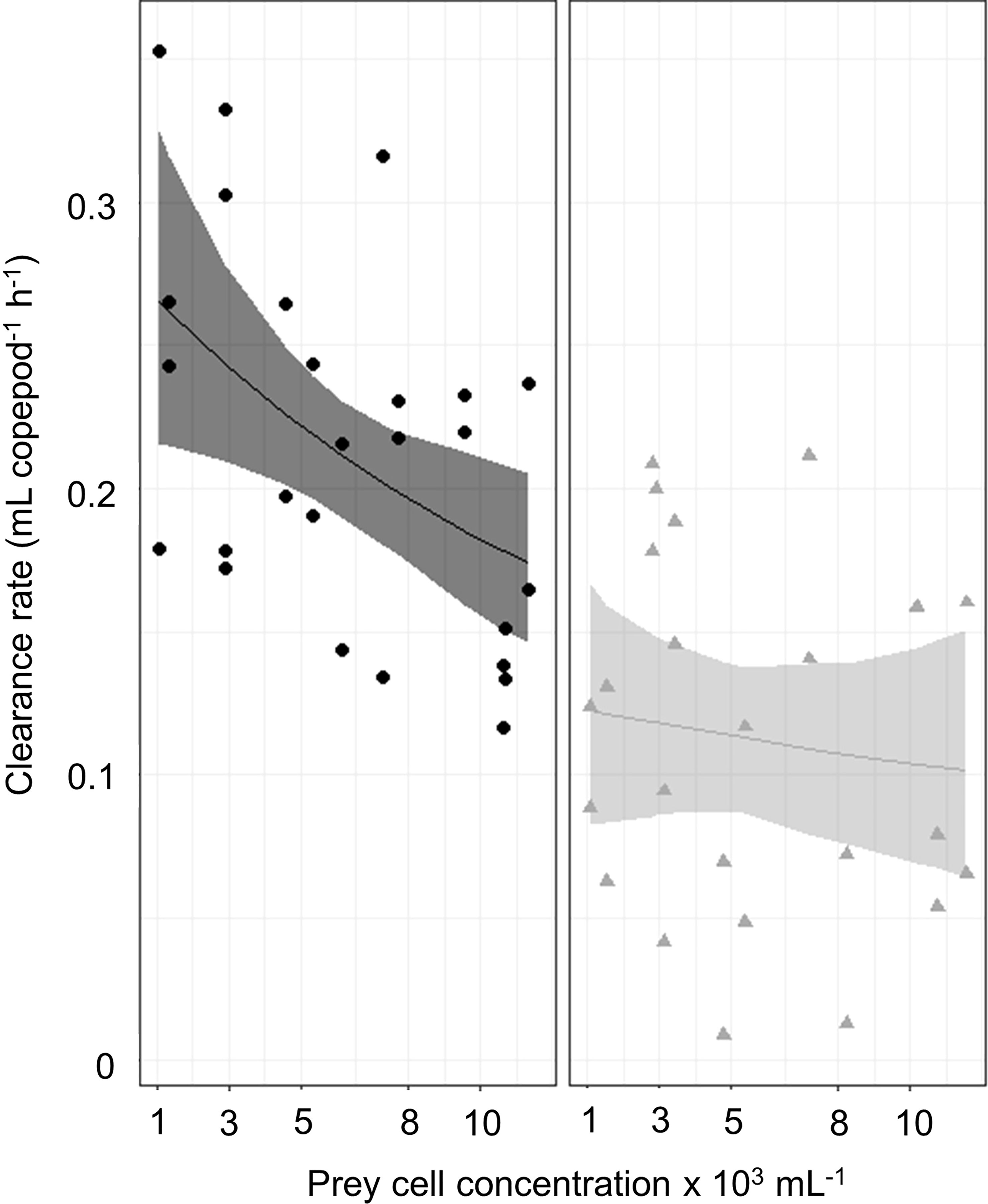
Figure 4 Clearance rates under ambient sound and harbor noise conditions fitted to the Rogers random predator equation. The x-axis shows the initial phytoplankton prey cell concentrations (continuous variable) from min 1042 to max 11563 cells ml−1. The y-axis presents the clearance rate that is defined as the volume of water from which cells are removed by feeding copepods (ml copepod−1 h−1) calculated after Frost (1972). The left panel shows the clearance rate under ambient aquarium sound conditions and the right panel the clearance rate under playback harbor noise exposure. The jittered dots are presenting all values in the data. The 97.5% confidence intervals were calculated using a bootstrap method in R version 3.4.4 (R Core Team, 2022).
Discussion
We present novel data on the impact of anthropogenic underwater noise on the feeding rates of the crustacean zooplankton Acartia tonsa when fed with small phytoplankton. Overall, we found a common pattern for ingestion rates as a function of prey density (Frost, 1972; Almeda et al., 2018) in both sound treatments. However, with a mean decrease of 40%, the animals in added playback harbor noise conditions failed to ingest every second to third prey they would have ingested under ambient sound conditions. In terms of inference, one may rather want to better compare a playback harbor traffic playback treatment versus a treatment playing back ambient lagoon sound. However, despite taking all measures to limit disturbing noise in the setup, in a controlled laboratory setup, operating background noise running the plankton wheel would be higher than any realistically playback of ambient lagoon sound as a silent treatment (Figure 2B). Therefore, we consider our choice of comparing a treatment with added harbor traffic noise playback to ambient aquarium sound conditions showing a similar effect as if there was an underlying playback of silent lagoon recordings added to the ambient sound control. We predicted prey density-dependent effects of noise would be more pronounced and easier to detect at high prey densities. Although we did not find this interaction between prey density and noise exposure, we found an increased dispersion in the data at higher prey density (cf. Table 3, Figure 3). This might be due to variations and plasticity in individual behavioral responses (Liu et al., 2018; Holm et al., 2019) or due to small differences in sensory structures (Yen et al., 1992; Fields, 2014). van Dinh et al. (2019) found a combined effect of prey density and pyrene, especially at high prey densities. They hypothesized that this might be due to the narcotic effect of pyrene that affects the handling time of the copepod Calanus finmarchicus. They calculated lower searching rates and longer handling times, which explains the increased effect at high prey density (van Dinh et al., 2019). In contrast, we calculated a lower capture rate in the noise treatment, while handling time remained the same between the two sound treatments. It therefore appeared that the capture rate of copepods in particular was affected by noise in our study.
Mechanisms of how underwater noise could affect capture rates
One of the mechanisms at hand would be masking or distraction. It is suggested that noise can mask essential natural sound cues for invertebrate settlement (as found in other meroplanktonic species Pine et al., 2012). Copepods detect prey through visual, chemical and mechanical signals (Fields, 2014), and the magnitude of importance for each detection mechanism is feeding mode- and species-specific (see Jakobsen et al., 2005; Fields, 2014), although mechanoreception may be the most common means of perception in different feeding modes (DeMott and Watson, 1991; Gonçalves and Kiørboe, 2015). Acartia tonsa is able to switch between feeding modes and prey types depending on the prey size (Jonsson and Tiselius, 1990), prey motility (Kiørboe et al., 1996), prey density (Kiørboe et al., 1996), and external disturbances such as turbulences (Saiz and Kiorboe, 1995; Kiørboe et al., 1996; Strickler and Costello, 1996). In ambush-feeding mode, copepods wait motionless in the water column until they perceive a hydromechanical signal generated by a potential prey, then reorientate themselves towards the prey, and attack it by directional jumps (Tiselius and Jonsson, 1990; Saiz and Kiorboe, 1995). On the other hand, feeding on small non-motile prey involves the generation of a feeding current with feeding appendages and thoracopods (Tiselius and Jonsson, 1990; Gonçalves and Kiørboe, 2015). Note that, in our current study, we cannot differentiate which feeding mode had been used. Saiz and Kiorboe (1995) studied the effect of turbulent water on the two feeding modes of A. tonsa and found only decreased clearance rates when exposed to turbulences exceeding natural turbulence levels in the field probably due to impaired prey perception (ambush feeding) and eroded feeding currents (suspension feeding). Even though turbulence and sound are not directly comparable, high levels of underwater noise could impair similar mechanisms as turbulences.
The detection of the potential prey, in general, depends on the strength of its velocity difference to the ambient to elicit a behavioral response of the copepod. In the copepods Labidocera madurae and Acartia fossae a velocity strength of only 20 µm s−1 in the vibration frequency range from 40 to 1000 Hz is sufficient to trigger antennal neuroreceptors to fire (Yen et al., 1992) and a study on the escape response of A. tonsa has shown a threshold signal strength or velocity difference for deformation at 150 µm s−1 and accelerations as low as 130 µm s−2 in the near field of a siphon flow (Kiørboe et al., 1999). Due to the high sensitivity of copepods to fluid disturbances, the harbor noise exposure used in the present study may have been above detection thresholds, which could have led to masking or distraction, but further measurements of particle motion velocities in an aquarium setup are needed to test this.
Our results are inconsistent with a feeding experiment by Tremblay et al. (2019) in which A. tonsa was exposed to a noise egg, a waterproof device that produces low-frequency sound (de Jong et al., 2017). Nevertheless, they found a physiological response correlated with oxidative stress (Tremblay et al., 2019). Hydromechanical disturbances from different prey types are sensed by mechanoreceptive setae on the first antenna of calanoid copepods (Yen et al., 1992; Gonçalves and Kiørboe, 2015), such as Acartia sp. Solé et al. (2021b) performed an ultrastructural analysis of the setae on the first antenna of the ectoparasitic copepod species Lepeophtheirus salmonis, which uses mechanoreception similar to calanoids, but for host detection. They found that the setae had fused when L. salmonis was exposed to noise for 4 h. Maximum setae fusion occurred when exposed to a combination of 350 Hz and 500 Hz sound (cf. Figure 2). The mechanism was hypothesized to be related to oxidative stress, possibly followed by acoustic trauma. Similar mechanisms may also occur in A. tonsa upon exposure to harbor noise, where setae fusion may lead to impaired prey perception. However, the noise frequency ranges that affect A. tonsa most may be different from those of L. salmonis.
Whether the impact of noise on the ingestion rates of copepods feeding on phytoplankton is due to masking the hydromechanical signals of potential prey or distraction or related to physiological or morphological changes remains open. The magnitude and direction of responses in zooplankton when exposed to underwater noise is further most probably species- and stage-specific and depends on the sound source level and experimental design (Vereide and Kühn, 2023; Tremblay et al., 2019; Solé et al., 2021b). For future studies, we suggest a combination of empirical and modeling approaches investigating how noise impacts feeding in different copepod species, sexes, and stages.
Acoustic setup design
We were limited to measure only the sound pressure part of the harbor traffic exposure even though particle motion is known being detected by invertebrates rather than sound pressure (Nedelec et al., 2016). Nedelec et al. (2015) and Simpson et al. (2016) measured 20–40 dB (µm s−2)2 Hz−1 higher particle acceleration in laboratory tank experiments compared to in situ recordings while sound pressure levels measured in these tanks were similar to the in situ recordings. We therefore may underestimate the true exposure in terms of particle motion. Further we would like to address that copepods most probably perceive velocity rather than acceleration (Kiørboe et al., 1999) which should be considered when reporting particle motion in sound-related future work on copepods. Playback of harbor traffic noise is partly distorted in small tanks from the original recordings (see Akamatsu et al., 2002; Jones et al., 2019) as seen in Figure 2B. However, at higher frequencies (>1000 Hz), such distortions may be less biologically relevant due to copepod sensitivity to lower frequencies (Yen et al., 1992; Solé et al., 2021b). Further, the continuous rotation of the plankton wheel during incubation imposes some variation onto the vials housing the experimental copepod communities per day, potentially leading to random noise exposures instead of the regular exposure from the looped playback. Differences in noise regularity, from e.g. ship traffic versus operational wind turbine noise, may lead to different behavioral outcomes (Nedelec et al., 2015).
Anthropogenic underwater noise effects on trophic cascades from a zooplankton perspective
We consider our results to be realistic for near-field shipping noise levels, for example in ports or along shipping lanes, servicing e.g. offshore wind farms or the oil industry and construction work.
Ingestion rates are known to be linearly correlated to egg production in A. tonsa and other calanoid copepods species (Kiørboe et al., 1985). Further, copepod growth is limited by food quantity (Anderson et al., 2021). Food quantity, in this case phytoplankton density, is known to vary throughout the year and being site- and species-specific. In general, phytoplankton densities ranging from low (×103 l−1) to high abundances (×106 l−1) especially during spring blooms (Lefebvre et al., 2011; Alprol et al., 2021). Our results on ingestion rates at different prey densities are therefore representative for natural phytoplankton abundances. Reduced feeding due to anthropogenic underwater noise at all phytoplankton prey densities, as presented in this study, may thus lead to decreased egg production, limited growth and development, and, in turn would lead to lower abundances of certain copepod species. Thus, the decrease of certain copepod species affects both the interactions to lower levels, e.g. phytoplankton and smaller zooplankton, as well as to higher trophic levels and thus may alter the fate of organic carbon’s transfer through the food chain (Steinberg and Landry, 2017) and its storage (Turner, 2015).
Our study did not consider ontogenetic and developmental aspects and sex differences. It is known that older copepod developmental stages are more mechanoreception-sensitive compared to younger developmental stages (Fields and Yen, 1997; Kiørboe et al., 1999) and hence adult animals may be more vulnerable to underwater noise. The effects of continuous noise on different developmental stages in copepods should be further investigated like done in a previous study on the effects of impulsive underwater noise on A. tonsa (Vereide et al., 2023). Further, differences in feeding efficiencies between female and male copepods are known but this difference is mainly explained by body size (van Someren Gréve et al., 2017), which was included in the statistical analysis of our study our study. Note that if noise affects the detection and response to hydrodynamic signals from prey, it could also alter the perception of fluid signals from potential mates and predators (Fields, 2014), thus affecting community dynamics.
At this point we cannot extrapolate from the feeding response of a single species on a single prey species when exposed to a single stressor to whole community-level dynamics. Our results, however, underline the need to further investigate the consequences of anthropogenic underwater noise on zooplankton.
In conclusion, we found that elevated noise levels similar to those measured the North Sea (Farcas et al., 2020; Kinneging and Tougaard, 2021) impair copepod feeding. However, noise exposure should be further investigated in the field to disentangle the potential effect of real-life shipping noise from playback noise in small tanks.
Data availability statement
The raw data supporting the conclusions of this article will be made available by the authors, without undue reservation.
Author contributions
SK conceptualized the project. SK, FK, and KH designed the research. SK and FK conducted the experiments. SK analyzed the data. SK wrote the initial draft of the manuscript. SK, FK, and KH wrote the paper. KH supervised and acquired funding and resources. All authors contributed to the article and approved the submitted version.
Funding
This study was funded by the Federal State Funding at Kiel University and the JPI Oceans Noise project ORCHESTRA (03F0932B). We acknowledge financial support by Land Schleswig-Holstein within the funding programme Open Access Publikationsfonds.
Acknowledgments
We would like to thank the staff at the Research and Technology Centre West Coast as well as BlueBioTec, especially Dr. Daniela Martensen-Staginnus, and IMTE (all Büsum, Germany) for supporting the present study. Special thanks to Dawid Jasecki for his support constructing the underwater plankton wheel.
Conflict of interest
The authors declare that the research was conducted in the absence of any commercial or financial relationships that could be construed as a potential conflict of interest.
Publisher’s note
All claims expressed in this article are solely those of the authors and do not necessarily represent those of their affiliated organizations, or those of the publisher, the editors and the reviewers. Any product that may be evaluated in this article, or claim that may be made by its manufacturer, is not guaranteed or endorsed by the publisher.
Supplementary material
The Supplementary Material for this article can be found online at: https://www.frontiersin.org/articles/10.3389/fmars.2023.1134792/full#supplementary-material
References
Abrams P. A. (2022). Food web functional responses. Front. Ecol. Evol. 10. doi: 10.3389/fevo.2022.984384
Akamatsu T., Okumura T., Novarini N., Yan H. Y. (2002). Empirical refinements applicable to the recording of fish sounds in small tanks. J. Acoust. Soc Am. 112 (6), 3073–3082. doi: 10.1121/1.1515799
Almeda R., van Someren Gréve H., Kiørboe T. (2018). Prey perception mechanism determines maximum clearance rates of planktonic copepods. Limnol. Oceanogr. 63 (6), 2695–2707. doi: 10.1002/lno.10969
Alprol A. E., Ashour M., Mansour A. T., Alzahrani O. M., Mahmoud S. F., Gharib S. M. (2021). Assessment of water quality and phytoplankton structure of eight Alexandria beaches, southeastern Mediterranean Sea, Egypt. J. Mar. Sci. Eng. 9 (12), 1328. doi: 10.3390/jmse9121328
Amorim M. C. P., Pedroso S. S., Bolgan M., Jordão J. M., Caiano M., Fonseca P. J. (2013). Painted gobies sing their quality out loud: acoustic rather than visual signals advertise male quality and contribute to mating success. Funct. Ecol. 27, 289–298. doi: 10.1111/1365-2435.12032
Anderson T. R., Hessen D. O., Mayor D. J. (2021). Is the growth of marine copepods limited by food quantity or quality? Limnol. Oceanogr. Lett. 6 (3), 127–133. doi: 10.1002/lol2.10184
Bates D., Mächler M., Bolker B. M., Walker S. C. (2015). Fitting linear mixed-effects models using lme4. J. Stat. Software 67 (1), 1–48. doi: 10.18637/jss.v067.i01
Brylinski J. M. (1981). Report on the presence of Acartia tonsa Dana (Copepoda) in the harbor of Dunkirk (France) and its geographical distribution in Europe. J. Plankton Res. 3, 255–260. doi: 10.1093/plankt/3.2.255
Burnham K. P., Anderson D. R. (2002). “Model selection and inference,” in A practical information-theoretic approach (New York: Springer). doi: 10.1007/b97636
Carter E. E., Tregenza T., Stevens M. (2020). Ship noise inhibits colour change, camouflage, and anti-predator behaviour in shore crabs. Curr. Biol. 30, R211–R212. doi: 10.1016/j.cub.2020.01.014
Cole M., Coppock R., Lindeque P. K., Altin D., Reed S., Pond D. W., et al. (2019). Effects of nylon microplastic on feeding, lipid accumulation, and moulting in a coldwater copepod. Environ. Sci. Technol. 53 (12), 7075–7082. doi: 10.1021/acs.est.9b01853
de Jong K., Schulte G., Heubel K. U. (2017). The noise egg: a cheap and simple device to produce low-frequency underwater noise for laboratory and field experiments. Methods Ecol. Evol. 8 (2), 268–274. doi: 10.1111/2041-210X.12653
DeMott W. R., Watson M. D. (1991). Remote detection of algae by copepods: responses to algal size, odors and motility. J. Plankton Res. 13 (6), 1203–1222. doi: 10.1093/plankt/13.6.1203
Directive 2008/56/EC (2008). Establishing a framework for community action in the field of marine environmental policy (Marine strategy framework directive). OJEU 164, 19–40.
Doney S. C., Ruckelshaus M., Duffy J. E., Barry J. P., Chan F., English C. A., et al. (2012). Climate change impacts on marine ecosystems. Ann. Rev. Mar. Sci. 4 (1), 11–37. doi: 10.1146/annurev-marine-041911-111611
Duarte C. M., Chapuis L., Collin S. P., Costa D. P., Devassy R. P., Eguiluz V. M., et al. (2021). The soundscape of the anthropocene ocean. Science 371 (6529), eaba4658. doi: 10.1126/science.aba4658
Erbe C., Marley S. A., Schoeman R. P., Smith J. N., Trigg L. E., Embling C. B. (2019). The effects of ship noise on marine mammals - a review. Front. Mar. Sci. 6. doi: 10.3389/fmars.2019.00606
Farcas A., Powell C. F., Brookes K. L., Merchant N. D. (2020). Validated shipping noise maps of the northeast Atlantic. Sci. Total Environ. 735, 139509. doi: 10.1016/j.scitotenv.2020.139509
Fields D. M. (2014). “The sensory horizon of marine copepods,” in Copepods: Diversity, habitat and behavior. Ed. Seuront L. (New York, USA: Nova Science Publishers, Inc), 157–179.
Fields D. M., Yen J. (1997). The escape behavior of marine copepods in response to a quantifiable fluid mechanical disturbance. J. Plankton Res. 19 (9), 1289–1304. doi: 10.1093/plankt/19.9.1289
Frost B. W. (1972). Effects of size and concentration of food particles on the feeding behavior of the marine planktonic copepod Calanus pacificus. Limnol. Oceanogr 17 (6), 805–815. doi: 10.4319/lo.1972.17.6.0805
Fulfer V. M., Menden-Deuer S. (2021). Heterotrophic dinoflagellate growth and grazing rates reduced by microplastic ingestion. Front. Mar. Sci. 8. doi: 10.3389/fmars.2021.716349/bibtex
Garzke J., Hansen T., Ismar S. M. H., Sommer U. (2016). Combined effects of ocean warming and acidification on copepod abundance, body size and fatty acid content. PloS One 11 (5), 1–22. doi: 10.1371/journal.pone.0155952
Gonçalves R. J., Kiørboe T. (2015). Perceiving the algae: How feeding-current feeding copepods detect their nonmotile prey. Limnol. Oceanogr. 60 (4), 1286–1297. doi: 10.1002/lno.10102
Halfwerk W., Slabbekoorn H. (2015). Pollution going multimodal: the complex impact of the human-altered sensory environment on animal perception and performance. Biol. Lett. 11 (4), 20141051. doi: 10.1098/rsbl.2014.1051
Holling C. (1959). Some characteristics of simple types of predation and parasitism. Can. Entomol. 91 (7), 385–398. doi: 10.4039/ent91385-7
Holm M. W., Rodríguez-Torres R., Hansen B. W., Almeda R. (2019). Influence of behavioral plasticity and foraging strategy on starvation tolerance of planktonic copepods. J. Exp. Mar. Biol. Ecol. 511, 19–27. doi: 10.1016/j.jembe.2018.11.002
Isinibilir M., Svetlichny L., Mykitchak T., Türkeri E. E., Eryalçın K. M., Doğan O., et al. (2020). Microplastic consumption and its effect on respiration rate and motility of Calanus helgolandicus from the marmara Sea. Front. Mar. Sci. 7. doi: 10.3389/fmars.2020.603321
Jakobsen H. H., Halvorsen E., Winding Hansen B., Visser A. W. (2005). Effects of prey motility and concentration on feeding in Acartia tonsa and Temora longicornis: the importance of feeding modes. J. Plankton Res. 27 (8), 775–785. doi: 10.1093/plankt/fbi051
Jalkanen J.-P., Johansson L., Andersson M. H., Majamäki E., Sigray P. (2022). Underwater noise emissions from ships during 2014–2020. Environ. Pollut. 311, 119766. doi: 10.1016/j.envpol.2022.119766
Jézéquel Y., Bonnel J., Coston-Guarini J., Chauvaud L. (2019). Revisiting the bioacoustics of European spiny lobsters Palinurus elephas: comparison of antennal rasps in tanks and in situ. Mar. Ecol. Prog. Ser. 615, 143–157. doi: 10.3354/meps12935
Jézéquel Y., Bonnel J., Coston-Guarini J., Guarini J. M., Chauvaud L. (2018). Sound characterization of the European lobster Homarus gammarus in tanks. Aquat. Biol. 27, 13–23. doi: 10.3354/ab00692
Jones I. T., Stanley J. A., Bonnel J., Mooney T. A. (2019). Complexities of tank acoustics warrant direct, careful measurement of particle motion and pressure for bioacoustic studies. Proc. Mtgs. Acoust. 37, 10005. doi: 10.1121/2.0001073
Jonsson P., Tiselius P. (1990). Feeding behaviour, prey detection and capture efficiency of the copepod Acartia tonsa feeding on planktonic ciliates. Mar. Ecol. Prog. Ser. 60, 35–44. doi: 10.3354/meps060035
Kiørboe T., Møhlenberg F., Hamburger K. (1985). Bioenergetics of the planktonic copepod Acartia tonsa: relation between feeding, egg production and respiration, and composition of specific dynamic action. Mar. Ecol. Prog. Ser. 26, 85–97. doi: 10.3354/meps026085
Kiørboe T., Saiz E., Viitasalo M. (1996). Prey switching behaviour in the planktonic copepod Acartia tonsa. mar. Ecol. Prog. Ser. 143 (1–3), 65–75. doi: 10.3354/meps143065
Kiørboe T., Saiz E., Visser A. (1999). Hydrodynamic signal perception in the copepod Acartia tonsa. mar. Ecol. Prog. Ser. 179, 97–111. doi: 10.3354/meps179097
Kinneging N. A., Tougaard J. (2021). “Assessment north Sea,” in Report of the EU INTERREG joint monitoring programme for ambient noise north Sea (Jomopans).
Kühn S., Utne-Palm A. C., de Jong K. (2022). Two of the most common crustacean zooplankton Meganyctiphanes norvegica and calanus spp. produce sounds within the hearing range of their fish predators. Bioacoustics 32 (1), 73–89. doi: 10.1080/09524622.2022.2070542
Lefebvre A., Guiselin N., Barbet F., Artigas F. L. (2011). Long-term hydrological and phytoplankton monitoring, (1992–2007) of three potentially eutrophic systems in the eastern English channel and the southern bight of the north Sea. ICES J. Mar. Sci. 68 (10), 2029–2043. doi: 10.1093/icesjms/fsr149
Leiva L., Scholz S., Giménez L., Boersma M., Torres G., Krone R., et al. (2021). Noisy waters can influence young-of-year lobsters’ substrate choice and their antipredatory responses. Environ. pollut. 291, 118108. doi: 10.1016/j.envpol.2021.118108
Lenz P. H., Hartline D. K. (2014). “Mechanoreception in crustaceans of the pelagic realm,” in Nervous systems and control of behavior. Eds. Derby C., Thiel M. (Oxford, UK: Oxford University Press), 293–320.
Liu B., Akiba T., Landeira J. M., Tanaka Y. (2018). Individual-level variability in the behavioral responses of female Oithona davisae (Copepoda: Cyclopoida) to hydromechanical stimuli. La Mer. 56, 21–35.
Long J. A. (2022) Jtools: Analysis and presentation of social scientific data. r package version 2.2.0. Available at: https://cran.r-project.org/package=jtools.
Lynam C. P., Llope M., Möllmann C., Helaouët P., Bayliss-Brown G. A., Stenseth N. C. (2017). Interaction between top-down and bottom-up control in marine food webs. Proc. Natl. Acad. Sci. U.S.A. 114 (8), 1952–1957. doi: 10.1073/pnas.1621037114
Moreno H. D., Köring M., Di Pane J., Tremblay N., Wiltshire K. H., Boersma M., et al. (2022). An integrated multiple driver mesocosm experiment reveals the effect of global change on planktonic food web structure. Commun. Biol. 5 (1), 179. doi: 10.1038/s42003-022-03105-5
Mullin M. M. (1963). Some factors affecting the feeding of marine copepods of the genus Calanus. limnol. Oceanogr. 8 (2), 239–250. doi: 10.4319/lo.1963.8.2.0239
Murchy K. A., Davies H., Shafer H., Cox K., Nikolich K., Juanes F. (2020). Impacts of noise on the behavior and physiology of marine invertebrates: A meta-analysis. Proc. Meet. Acoust. 37 (1), 040002. doi: 10.1121/2.0001217
Nedelec S. L., Campbell J., Radford A. N., Simpson S. D., Merchant N. D. (2016). Particle motion: the missing link in underwater acoustic ecology. Methods Ecol. Evol. 7 (7), 836–842. doi: 10.1111/2041-210x.12544
Nedelec S. L., Simpson S. D., Morley E. L., Nedelec B., Radford A. N. (2015). Impacts of regular and random noise on the behaviour, growth and development of larval Atlantic cod (Gadus morhua). Proc. R. Soc B. 282 (1817), 20151943. doi: 10.1098/rspb.2015.1943
Pine M. K., Jeffs A. G., Radford C. A. (2012). Turbine sound may influence the metamorphosis behaviour of estuarine crab megalopae. PloS One 7 (12), 1–8. doi: 10.1371/journal.pone.0051790
Pinheiro J., Bates D., R Core Team (2022) Nlme: Linear and nonlinear mixed effects models. r package version 3. Available at: https://CRAN.R-project.org/package=nlme.
Pirotta E., Thomas L., Costa D. P., Hall A.J., Harris C.M., Harwood J, et al. (2022). Understanding the combined effects of multiple stressors: A new perspective on a longstanding challenge. Sci. Total Environ. 821, 153322. doi: 10.1016/j.scitotenv.2022.153322
R Core Team (2022). R: A language and environment for statistical computing (Vienna, Austria: R foundation for statistical computing). Available at: https://www.R-project.org/.
Rogers D. (1972). Random search and insect population models. J. Anim. Ecol. 41, 369–383. doi: 10.2307/3474
Ruiz-Ruiz P. A., Hinojosa I. A., Urzua A., Urbina M. A. (2020). Anthropogenic noise disrupts mating behavior and metabolic rate in a marine invertebrate. Proc. Meet. Acoust. 37 (1), 040006. doi: 10.1121/2.0001302
Saiz E., Kiorboe T. (1995). Predatory and suspension feeding of the copepod Acartia tonsa in turbulent environments. Mar. Ecol. Prog. Ser. 122 (1-3), 147–158. doi: 10.3354/meps122147
Sal Moyano M., Ceraulo M., Hidalgo F., Luppi T., Nuñez J., Radford C. A., et al. (2021). Effect of biological and anthropogenic sound on the orientation behavior of four species of brachyuran crabs. Mar. Ecol. Prog. Ser. 669, 107–120. doi: 10.3354/meps13739
Scholz B., Liebezeit G. (2012). Microphytobenthic dynamics in a wadden Sea intertidal flat – part II: Seasonal and spatial variability of non-diatom community components in relation to abiotic parameters. Eur. J. Phycol. 47 (2), 120–137. doi: 10.1080/09670262.2012.665251
Schultz M., Kiørboe T. (2009). Active prey selection in two pelagic copepods feeding on potentially toxic and non-toxic dinoflagellates. J. Plankton Res. 31 (5), 553–561. doi: 10.1093/plankt/fbp010
Simpson S. D., Radford A. N., Nedelec S. L., Ferrari M. C. O., Chivers D. P., McCormick M. I., et al. (2016). Anthropogenic noise increases fish mortality by predation. Nat. Commun. 7 (1), 1–7. doi: 10.1038/ncomms10544
Solé M., Lenoir M., Durfort M., Fortuño J. M., van der Schaar M., de Vreese S., et al. (2021a). Seagrass Posidonia is impaired by human-generated noise. Commun. Biol. 4 (1), 1–11. doi: 10.1038/s42003-021-02165-3
Solé M., Lenoir M., Fontuño J. M., Durfort M., van der Schaar M., André M. (2016). Evidence of cnidarians sensitivity to sound after exposure to low frequency noise underwater sources. Sci. Rep.6 1), 37979–37979. doi: 10.1038/srep37979
Solé M., Lenoir M., Fortuño J.-M., de Vreese S., van der Schaar M., André M. (2021b). Sea Lice are sensitive to low frequency sounds. J. Mar. Sci. Eng. 9 (7), 765. doi: 10.3390/jmse9070765
Stearns D. E. (1986). Copepod grazing behavior in simulated natural light and its relation to nocturnal feeding. Mar. Ecol. Prog. Ser. 30, 65–76. doi: 10.3354/meps030065
Steinberg D. K., Landry M. R. (2017). Zooplankton and the ocean carbon cycle. Annu. Rev. Mar. Sci. 9 (1), 413–444. doi: 10.1146/annurev-marine-010814-015924
Strickler J. R., Costello J. H. (1996). Calanoid copepod behavior in turbulent flows. Mar. Ecol. Prog. Ser. 139 (1/3), 307–309.
Thor P., Cervetto G., Besiktepe S., Ribera-Maycas E., Tang K. W., Dam H. G. (2002). Influence of two different green algal diets on specific dynamic action and incorporation of carbon into biochemical fractions in the copepod Acartia tonsa. J. Plankton R. 24 (4), 293–300. doi: 10.1093/plankt/24.4.293
Tiselius P., Jonsson P. (1990). Foraging behaviour of six calanoid copepods: observations and hydrodynamic analysis. Mar. Ecol. Prog. Ser. 66, 23–33. doi: 10.3354/meps066023
Tolstoganova L. K. (2002). “Acoustical behavior in king crab (Paralithodes camtschaticus),” in Crabs in cold water regions: Biology, management, and economics. Eds. Paul A. J., Dawe E. G., Elner R., Jamieson G. S., Kruse G. H., Otto R. S., Sainte-Marie B., Shirley T. C., Woodby D. (Fairbanks, AK: University of Alaska Sea Grant), 247–254. doi: 10.4027/ccwrbme.2002.19
Tremblay N., Leiva L., Beermann J., Meunier C. L., Boersma M. (2019). Effects of low-frequency noise and temperature on copepod and amphipod performance. Proc. Meet. Acoust 37 (1), 040005. doi: 10.1121/2.0001275
Turner J. T. (2015). Zooplankton fecal pellets, marine snow, phytodetritus and the ocean’s biological pump. Prog. Oceanogr 130, 205–248. doi: 10.1016/j.pocean.2014.08.005
van Dinh K., Olsen M. W., Altin D., Vismann B., Nielsen T. G. (2019). Impact of temperature and pyrene exposure on the functional response of males and females of the copepod Calanus finmarchicus. environ. Sci. pollut. Res. 26 (28), 29327–29333. doi: 10.1007/s11356-019-06078-x
van Someren Gréve H., Almeda R., Lindegren M., Kiørboe T. (2017). Gender-specific feeding rates in planktonic copepods with different feeding behavior. J. Plankton Res. 39 (4), 631–644. doi: 10.1093/plankt/fbx033
Vereide E. H., Kühn S. (2023). “Effects of anthropogenic noise on marine zooplankton,” in Effects of noise on aquatic life: Principles and practical considerations version 6. Eds. Popper A. N., Sisneros J., Hawkins A., Thomsen F. (Springer).
Vereide E. H., Mihaljevic M., Browman H. I., Fields D. M., Agersted M. D., Titelman J., et al. (2023) Effects of airgun discharges used in seismic surveys on development and mortality in nauplii of the copepod Acartia tonsa. Environ. pollut 327, 121469. doi: 10.1016/j.envpol.2023.121469
Wale M. A., Simpson S. D., Radford A. N. (2013). Noise negatively affects foraging and antipredator behaviour in shore crabs. Anim. Behav. 86 (1), 111–118. doi: 10.1016/j.anbehav.2013.05.001
Wickham H. (2016). ggplot2: Elegant Graphics for Data Analysis (New York: Springer). doi: 10.1007/978-3-319-24277-4
Yen J., Lenz P. H., Gassie D. V., Hartline D. K. (1992). Mechanoreception in marine copepods: electrophysiological studies on the first antennae. J. Plankton Res. 14 (4), 495–512. doi: 10.1093/plankt/14.4.495
Yen J., Okubo A. (2002). Particle and prey detection by mechanoreceptive copepods: A mathematical analysis. Hydrobiologia 480, 165–173. doi: 10.1023/a:1021249521259
Yen J., Strickler J. R. (1996). Advertisement and concealment in the plankton: What makes a copepod hydrodynamically conspicuous? Biology. 115 (3), 191–205.
Keywords: underwater noise effects, continuous underwater noise, zooplankton, copepods, ingestion rates, clearance rates, predator–prey, functional response
Citation: Kühn S, King F and Heubel K (2023) Decreased feeding rates of the copepod Acartia tonsa when exposed to playback harbor traffic noise. Front. Mar. Sci. 10:1134792. doi: 10.3389/fmars.2023.1134792
Received: 30 December 2022; Accepted: 24 March 2023;
Published: 20 April 2023.
Edited by:
Marta Solé, BarcelonaTech (UPC), SpainReviewed by:
Maria Paz Sal Moyano, CONICET Mar del Plata, ArgentinaFrédéric Bertucci, L’exploitation et la Conservation (MARBEC), France
Andreas Brutemark, Biotopia, Sweden
Copyright © 2023 Kühn, King and Heubel. This is an open-access article distributed under the terms of the Creative Commons Attribution License (CC BY). The use, distribution or reproduction in other forums is permitted, provided the original author(s) and the copyright owner(s) are credited and that the original publication in this journal is cited, in accordance with accepted academic practice. No use, distribution or reproduction is permitted which does not comply with these terms.
*Correspondence: Saskia Kühn, kuehn@ftz-west.uni-kiel.de
†ORCID: Katja Heubel, orcid.org/0000-0002-7946-5542