- 1Key Laboratory of Marine Environment and Ecology, Ministry of Education, Ocean University of China, Qingdao, Shandong, China
- 2College of Environmental Science and Engineering, Ocean University of China, Qingdao, Shandong, China
- 3Yellow Sea Fisheries Research Institute, Chinese Academy of Fishery Sciences, Qingdao, Shandong, China
This study investigated the degradation characteristics of propylbenzenes (PBZs, including isopropylbenzene and n-propylbenzene), with high leakage risks and ecological hazards, by a newly isolated marine microalga named Rhinomonas reticulata S6A which is a promising candidate for eco-friendly bioremediation from marine. About 72% and 56% of n-PBZ and i-PBZ can be degraded after 7 days in culture. The acute toxicity of n-PBZ (96h - EC50 = 2.38 mg/L) was higher than that of i-PBZ (96h - EC50 = 3.65 mg/L). The growth inhibition kinetics of this strain were consistent with the Edwards model (R2 = 0.998) and Aiba model (R2 = 0.999). The optimal concentrations for the degradation of n-PBZ and i-PBZ were calculated to be 2.42 mg/L and 2.78 mg/L, respectively. The degradation trends of PBZs conformed to the zero-order kinetic model, and k increased with initial concentrations. The moderate increase in inoculation density could accelerate the degradation of PBZs, with the maximum specific growth rates (r) of 1.116/d (n-PBZ) and 1.230/d (i-PBZ) at the initial inoculation density of 104 cells/mL, while over-inoculation (initial microalgae density more than 105 cells/mL) was not conducive to the degradation of the pollutants. There is not much data on the biodegradation of PBZs in the aquatic environment, so it would be worthwhile to try to apply the new microalgae to explore the fate of PBZs.
1 Introduction
Approximately 90% of transport mode for global trade is ocean shipping, and maritime trade volumes are set to triple by 2050 (Organisation for Economic Co-operation and Development (OECD), 2022). According to International Oil Pollution Compensation Funds (IOPC Funds), the carriage of propylbenzenes (PBZs) by sea belongs to Hazardous Noxious Substances (HNS) (International Oil Pollution Compensation Funds (IOPC Funds), 2019). Propylbenzenes have two isomers, n-propylbenzene (n-PBZ) and isopropylbenzene (i-PBZ; cumene). n-PBZ is a typical alkylbenzene that has been widely used as a substitute component of jet fuel and diesel oil due to its moderate molecular weight and chain length, and even electric outboard motors discharge sewage containing n-PBZ directly into the sea (Yuan et al., 2017; Peng et al., 2021). i-PBZ, a pivotal organic chemical raw material, is necessary for the production of phenol and acetone (Gollapudi et al., 2021). In particular, the typical composition of C9 aromatics, which are produced during the catalytic reforming of petroleum feedstocks and the cracking process used to produce ethylene (Li et al., 2021a), includes n-PBZ, i-PBZ, 2-ethyltoluene, 3-ethyltoluene, 4-ethyltoluene, 1,2,3-trimethylbenzene, 1,2,4-trimethylbenzene, 1,3,5-trimethylbenzene, and indene (Firth, 2008). Unfortunately, on November 4, 2018, 69.1 tons of industrially cracked C9 aromatics leaked in Quangang District, Fujian Province. As a result, about 5.5 tons of n-PBZ and 4.8 tons of i-PBZ entered the sea, paralyzing the nearby Xiaocuo breeding area and causing sea economic losses (Li et al., 2021a).
According to the European System of Behavioral Classification (“SEBC” classification) documented in the Bonn Agreement (Neuparth et al., 2011), the physical behavior of n-PBZ/i-PBZ after entering the sea falls into the category of “floating volatilization” based on its form (colorless oily liquid), solubility (38.6/58.5 mg/L seawater), density (0.86) and saturation vapor pressure (20°C: 0.33/0.43 kPa) (Peng et al., 2021). Based on the predicted values of bioconcentration factor (BCF) (n-PBZ-375; i-PBZ-356), PBZs has a moderate potential (Franke et al., 1994) for accumulation in aquatic organisms and researchers have also detected n-PBZ and i-PBZ in Anguilla, a widely distributed freshwater fish (Roose and Brinkman, 1998; Roose et al., 2003). However, the BCF test values for n-PBZ and i-PBZ were 38 and 36 based on the test organisms Carassius auratus (Donkin et al., 1989) and Mytilus edulis (Ogata et al., 1984), respectively. In summary, aquatic organisms showed a low to moderate accumulation capacity for PBZs. Although marine biotoxicity data available for PBZs are insufficient to date, the most sensitive species to n-PBZ and i-PBZ were found to be Skeletonema costatum (96 h-EC50 = 1.97 mg/L) (Peng, 2020) and Mysidopsis bahia (96 h-LC50 = 1.3 mg/L) (Peng et al., 2021), respectively. The most tolerant species was Dunaliella salina, with low 96 h-EC50 values of 8.91 mg/L (n-PBZ) and 14.13 mg/L (i-PBZ) (Peng, 2020). Based on the acute toxicity classification of the Group of Experts on the Scientific Aspects of Marine Environmental Protection (GESAMP) (Wells et al., 2002), both PBZs were “moderate toxic” to many marine organisms, such as Cyprinodon variegatus (Glickman et al., 1995), Phaeodactylum tricornutum (Peng, 2020) and Skeletonema costatum (Peng, 2020). Furthermore, the feeding rate of Mytilus edulis was reduced by 50% after 16 h of contamination with n-PBZ (0.86 mg/L) (Donkin et al., 1989).
Due to their wide application and large transportation quota, PBZs are high-risk chemicals that are prone to leakage in marine accidents. In the emergency response strategy for hazardous chemical leakage at sea, the physical method is inefficient, costly, and incomplete, and the chemical method is likely to cause secondary pollution (Duan et al., 2020). In order to avoid marine persistent ecological pollution caused by water-soluble fraction (WAF), bioremediation has become an eco-friendly strategy for emergency response to marine spills. Consistently, Kao and Prosser (2001), proposed that biodegradation is more suitable for the removal of single-ring aromatic hydrocarbons. Studies have indicated that microalgae acclimated to contaminants showed enhanced photosynthesis, growth rates, metabolic functions and/or other cellular processes (Osundeko et al., 2014; Cho et al., 2016; Rocha et al., 2018). In addition, microalgae can metabolize in three modes: autotrophic, heterotrophic and mixed, depending on the carbon sources available in seawater (Sutherland and Ralph, 2019). Alternatively, microalgae can utilize some organics as carbon nutrients, and these organics are metabolized into less toxic substances or be mineralized. It boils down to the fact that the extracellular or intracellular enzymes secreted by microalgae can convert and degrade organic pollutants into small molecular organic substances that can be further absorbed and utilized (Li et al., 2021b). This mechanism of microalgae metabolism provides advantages over that of bacteria and fungi. On the one hand, microalgae induce indirect photodegradation in addition to the direct secretion of enzymes that can oxidize pollutants as part of their degradation mechanism (Zhang et al., 2012; Tian et al., 2019). Algae-induced indirect photodegradation of organic matter is depends on the active oxidizing agents (e.g., hydroxyl radicals (·OH), singlet oxygen (1O2), superoxide (O2·−), hydrogen peroxide (H2O2), peroxyl radicals (·OOR)) generated by photosensitizers, especially photosynthetic pigments, which are rarely produced by bacteria (Wei et al., 2021). On the other hand, both bacteria and fungi produce their respective toxic substances, endotoxin, and mycotoxin, which make these microbes biological pollutants, whereas neither endotoxin nor mycotoxin is found in microalgae (Koh and Khor, 2023). For instance, microalgae are more flexible and can adapt well to the change of living conditions (Xiong et al., 2018), which was the main impetus for studying HNS remediation by microalgae in seawater.
Three mechanisms are involved in the bioremediation of organic pollutants, including biosorption, bioaccumulation, and biodegradation. Biosorption or bioaccumulation can only transfer contaminants and not remove them completely (Homem and Santos, 2011). Several reports indicate that biodegradation plays an important role in the removal of PBZs from the environment. Marine bacteria isolated from Atlantic sediments were able to degrade 37-60% of i-PBZ after 21 d (Walker et al., 1976); in situ biodegradation tests at the North Bay site showed that i-PBZ was completely degraded within 50 d (Acton and Barker, 1992). The bacteria Pseudomonas putida RE204 and Pseudomonas fluorescen IP01 can degrade i-PBZ via meta-cleavage of the aromatic ring and oxidize the side chain to isobutyric acid and 2-oxo-4-pentenoic acid (Aoki et al., 1996; Habe et al., 1996; Eriksson et al., 2005). A soil-derived bacterial community converts i-PBZ to several different metabolites such as acetaldehyde and 4-hydroxy-2-oxovaleric acid (Shahi et al., 2016). Eriksson et al. (2005) studied the anaerobic biodegradation of n-PBZ under iron-reducing conditions and n-PBZ was reduced to propylphenols over 18 weeks. Recently published data showed that the bacterium Pseudomonas frederiksbergensis SI8 was able to partially degrade Jet A commercial aviation fuel (16.8% n-PBZ) within 14 d (Ruiz et al., 2021). In particular, their biodegradation products (e.g. 1-phenylpropanol and 3-isopropyleatechol) have been detected (Peng et al., 2021). It is plausible to suggest that, for some microalgae, their tolerance increases in response to chronic exposure due to the induction of enzymatic pathways to counteract the toxic effects (Chen et al., 2015; Xiong et al., 2017a). Therefore, it is necessary to specifically screen native microorganisms in specific samples of polluted seawater (such as the coast of industrial parks or the sea area of oil spill accidents) (Sakulthaew et al., 2014; Herrero and Stuckey, 2015). Accordingly, in this study, the 28 stations were set up in the three sea areas near China, with the coasts corresponding to different ports, industrial bases, or inland river estuaries (Figure 1, Table S1), to screen for candidate species. The ecological environment of Daya Bay, a semi-enclosed shallow bay located in the northern part of the South China Sea, has been significantly affected by human activities (Yu et al., 2022). The eastern part of the Daya Bay petrochemical base releases organics into the surrounding environment throughout the year, thereby improving the tolerance of organisms in the nearby sea area to pollutants (Wu et al., 2010). Compared with the seawater samples collected from other areas, the seawater at Daya Bay station has a better ability to degrade PBZs (Table S2). Both PBZs were not effectively degraded by enriched samples from sites not listed in Table S2. An axenic microalga named Rhinomonas reticulata S6A was isolated from the seawater sample of Daya Bay, which could transform and further degrade both n-PBZ and i-PBZ in seawater. In this study, the degradation characteristics and acute toxicity of PBZs to R. reticulata S6A were clarified. In addition, a reasonable initial inoculation density of R. reticulata S6A was proposed to provide a theoretical basis for the practical bioremediation strategy.
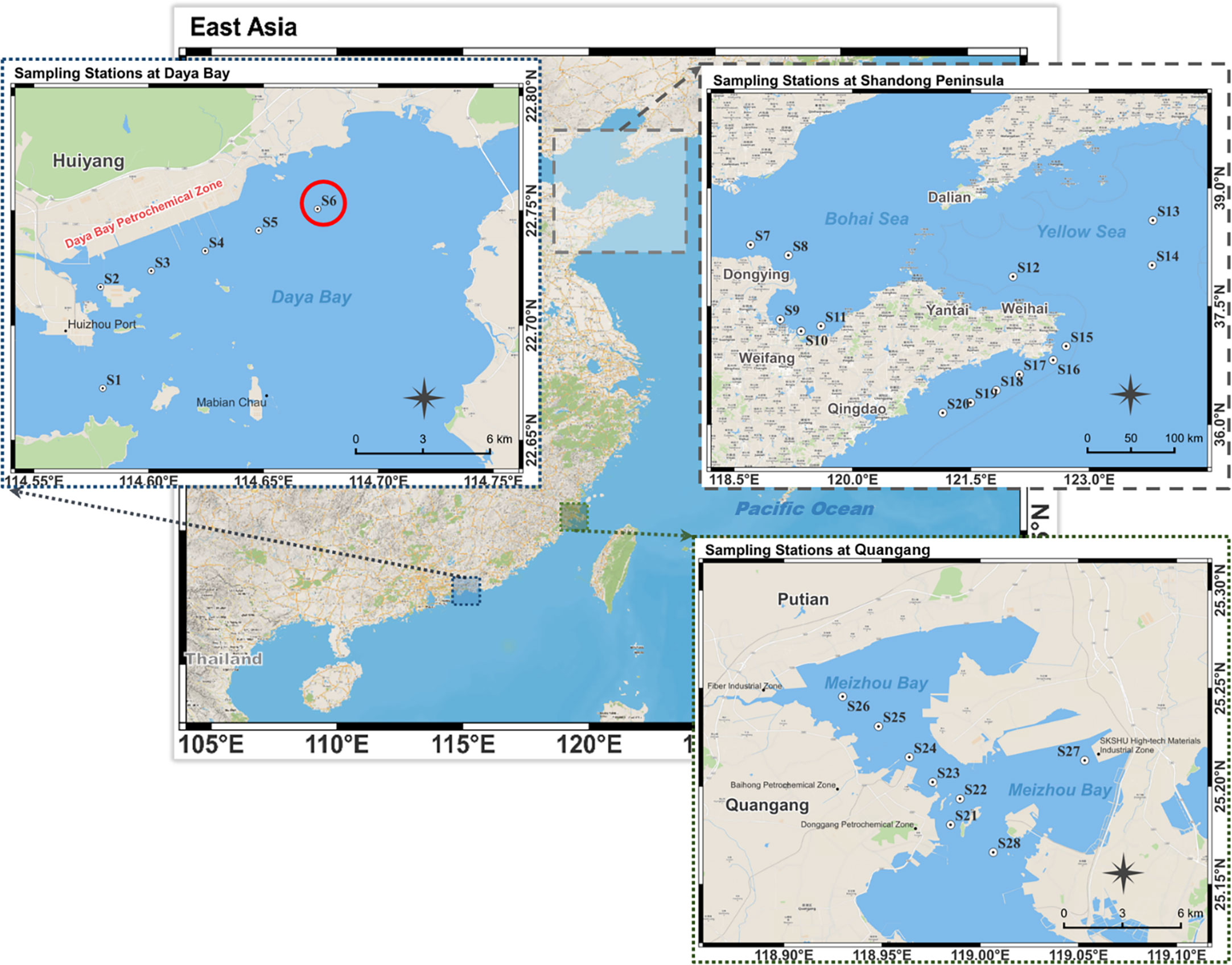
Figure 1 Stations in Daya Bay, Shandong Peninsula and Quangang. The red circle symbol indicates the source locality of R. reticulata S6A.
2 Materials and method
2.1 Chemicals
The samples of the 28 stations used to screen microalgae come from three sea areas near China (Figure 1, Table S1). F/2 medium (Guillard, 1975) were used in this study for culturing, and the composition was as follows (/L): NaNO3, 75 mg; Na2HPO4·H2O, 5 mg; EDTANa2, 4.36 mg; a solution of trace elements (ZnSO4·4H2O, 0.023 g/L; CoCl2·6H2O, 0.012 g/L; FeCl3·6H2O, 3.2 g/L; MnCl2·4H2O, 0.178 g/L; CuSO4·5H2O, 0.010 g/L; Na2MoO4·2H2O, 0.006 g/L), 1 mL; and a solution of vitamins (Vitamin B12, 0.0005 g/L; Vitamin B1, 0.100 g/L; biotin, 0.0005 g/L) 1 mL. NaHCO3 (250 mg/L) was added to provide inorganic carbon source in closed cultivation system. n-PBZ, i-PBZ and 1-chloronaphthalene with a purity higher than 99% were purchased from Tokyo Chemical Industry Co., Ltd (Shanghai, China). Dichloromethane (chromatographic grade) was purchased from Merck Co., Ltd. (Yantai, China). Biochemical reagents mixed antibiotics (Penicillin- Streptomycin-Amphotericin B Solution) and agar powder were purchased from Beijing Solarbio Science & Technology Co., Ltd (Beijing, China). Other reagents were of analytical grade and were purchased from Sinopharm Chemical Reagent Co., Ltd (Shanghai, China).
2.2 Screening and isolation of microalgae
Fresh seawater samples without any treatment were added to a sealed Erlenmeyer flask containing F/2 medium, n-PBZ/i-PBZ (20 mg/L, 0.22-μm filtered) and antibiotics for the first directional culture. The natural seawater (salinity 31, pH 7.90 ± 0.02) used for the next enrichment culture was taken from the coastal waters of Shazikou, Qingdao, filtered for impurities with a 0.45 μm cellulose acetate filter, and sterilized at high temperature (121°C) for 20 minutes. The antibiotics used in this study were a mixture of penicillin (100 U/mL), streptomycin (0.1 mg/mL) and amphotericin B (0.25 μg/mL). The culture was maintained in a shaker incubator at 100 rpm with a photoperiod of 14-h light/10-h dark under a fluorescent light at an intensity of 60 μmol photons/(m2·s) at 25°C. The same three sets of experiments as above were repeated. Next, the residual concentration of n-PBZ/i-PBZ in the culture medium of each station was determined on day seven (Table S2). The culture solution of the station S6 (from Daya Bay, Guangdong, China: 114.67354E, 22.75070N) with the highest degradation rate (n-PBZ: 28.6%; i-PBZ: 24.3%) of both PBZs was selected for isolation of pure algae, and then separated by the dilution and streak plate method.
2.3 Identification of microalgae
Optical microscope (OM) (YS2-H, Nikon, Japan) and scanning electron microscope (SEM) (VEGA3, TESCAN China, Ltd.) were used to observe the size and surface morphology of isolated microalgae. 18S rRNA was sequenced by a commercial service (Tsingke Biotechnology Co., Ltd., Qingdao, China) to perform molecular identification. The 18S rRNA region was amplified by polymerase chain reaction (PCR) with forward primer ITS1 (5’-TCCGTAGGTGAACCTGCGG-3’) and reverse primer ITS4 (5’-TCCTCCGCTTATTGATATGC-3’). The ClustalW and MEGA 5.0 software suites were used for alignment and phylogenetic analysis, respectively.
2.4 The biodegradation of by isolated microalgae
The pure microalgae were pre-cultured in Erlenmeyer flasks with sterile F/2 medium under the same culture conditions as described in section 2.2. Biodegradation experiments were performed in 50-mL glass sample bottles (sealed with Teflon®-lined screw caps) containing 10 mL of F/2 medium with isolated microalgae (104 cells/mL) in exponential phase. A total of five treatments: 0.5, 1, 5, 10, 20 mg/L (dissolved in dimethyl sulfoxide, DMSO) with 30 replicates per treatment were used. The incubation culture was shaken continuously at 100 rpm in an incubator at 25°C for seven days under the same conditions as the preculture. Sterilized control experiments (without microalgae) were performed to calculate the losses due to abiotic effects. Samples were then taken daily to determine cell density and n-PBZ residue (in triplicate). The biodegradation of i-PBZ was the same as that of n-PBZ except for the organic compound.
2.5 Degradation kinetics of PBZs by Rhinomonas reticulata S6A
Degradation kinetics has been widely applied to the removal process of organic compounds due to its good applicability and fitting effect (Kureel et al., 2017; Carvajal et al., 2018; Li et al., 2021b). Therefore, the zero-order (Eq. (1)), first-order (Eq. (2)) and pseudo-first-order (Eq. (3)) kinetic equations were used to elucidate the degradation kinetics of n-PBZ/i-PBZ removal in this study. The model equations were analyzed using Origin 2021 (OriginLab, USA).
where S0 was the initial concentration of n-PBZ/i-PBZ (mg/L); and St represented the concentration of n-PBZ/i-PBZ (mg/L) at time t; and k was the rate constant (/d) in the zero-order, first-order and pseudo-first-order reactions.
According to the model fitting results, the degradation of n-PBZ over time was fitted by the zero-order kinetic equation, and the half-life (t1/2) can be calculated using Eq. (4):
There is a lag phase in the degradation of i-PBZ by R. reticulata S6A, so the degradation data in the lag phase were not included in the kinetic fitting. Therefore, the true degradation time (DT50) of i-PBZ can be considered as the sum of the t1/2 calculated from Eq. (4) and the lag phase time (tlag) (Birch et al., 2017):
2.6 Growth inhibition kinetics
Six common growth inhibition kinetic models were used to characterize the growth process of microalgae exposed to PBZs. The Monod model is the most commonly used model to evaluate the specific growth rate of microorganisms in biodegradation (Jegan et al., 2010):
where, μ is the specific growth rate (/d), μmax is the maximum specific growth rate (/d), KS represents a substrate affinity constant (mg/L), and S is the substrate concentration (mg/L).
The Haldane-Andrews model (Andrews, 1968) is one of the most widely used models. The expression is as follows:
where, Ki is the inhibition constant (mg/L).
Webb (1963) developed a kinetic model that takes into account enzyme kinetics and allosteric effects. The equation is given by the following:
where, K (mg/L) is a positive constant.
Yano et al. (1966) proposed a growth model based on the inhibitory behaviour of microorganisms at high substrate concentrations:
Aiba et al. (1968) proposed an improved version of the Monod model, which relied on an empirical correlation:
Edwards (1970) proposed another model with which to predict the inhibitory effect at higher substrate concentrations. The equation is as follows:
In this study, Origin 2021 (OriginLab, USA) was used to fit the experimental data using a non-linear regression method.
2.7 Effects of inoculation densities on PBZs removal
Under aseptic conditions, degradation batch experiments were performed in 50-mL glass sample bottles with 10 mL F/2 medium containing 5 mg/L n-PBZ (or i-PBZ). Five gradients were used to study the effect of inoculation density: 103, 104, 5×104, 105, 3×105 cells/mL, with 30 replicates per treatment were used. The culture conditions were the same as those described in section 2.4. The cell density of microalgae and the residual concentration of n-PBZ (or i-PBZ) were measured at 24 h intervals (three replicates for cell density and three replicates for n-PBZ/i-PBZ concentration).
2.8 Analytical methods
2.8.1 Determination of microalgae cell density
One milliliter of algal solution was filtered through a 300-μm bolting-silk and mixed well. Then, Accuri C6 Plus flow cytometer (BD, USA) was used to count and calculate the algal cell density (D, cells/mL). The calculation formula is as follows:
where N, Vi and Vm represent the number of living microalgae displayed on the cytometer (cells), the injection volume (0.05 mL) and unit volume, respectively.
The concentrations leading to a 50% growth inhibition at 72 or 96 h (72/96 h-EC50) were calculated by probit analysis (Finney, 1947). The concentration obtained at 5.0 of the probits was the EC50 value.
2.8.2 Determination of fluorescence intensity
According to Chen et al. (2022) and Oukarroum et al. (2018), the fluorescence intensity (RFU) of parameters PerCP and PE in flow cytometry was used to characterize the photosynthesis performance and phycoerythrin content on day 7.
2.8.3 Calculation of specific growth rate
The specific growth rate (μ) was calculated for microalgae according to the following expression:
where N1 and N2 represent the cell densities of microalgae (cells/mL) at times t1 and t2, respectively.
2.8.4 Determination of the concentration of PBZs
For extraction, 5 mL of dichloromethane containing 5 mg/L of 1-chloronaphthalene was added to the bottle and mixed for 5 min, after which the concentration of PBZs in the methylene chloride layer was quantified by GC-MS using an Agilent 6890 gas chromatograph (HP-5MS column, 30 m × 0.25 mm i.d. × 0.25-μm film thickness) coupled to an Agilent 5975 mass spectrometer. The column was held at 100 °C for the first 1 min, increased to 150°C at 25°C/min, and to 200°C at 30°C/min, and the final temperature was held for 1 minute. The inlet and transfer line temperatures were set to 230°C and 280°C, respectively. The selected ion monitoring (SIM) mode was set for data acquisition and analysis.
2.8.5 Removal rate of PBZs
The removal rate (R) of PBZs is calculated according to the following expression:
where, Ct and C0 represent the concentrations of n-PBZ/i-PBZ (mg/L) at t and initial time.
2.9 Statistical analysis
Means and standard deviations of the results were calculated using three replicates of parallel experiments for each measurement. The significance of any differences between the treatment and control groups was evaluated by analysis of Games-Howell’s (heteroscedasticity) or Tukey’s (homogeneity) statistics in SPSS 19.0 (SPSS Co., USA) and differences were considered statistically significant when P< 0.05.
3 Results and discussions
3.1 Isolation and identification of Rhinomonas reticulata S6A
After alignment with other 18S rRNA sequences in GenBank, the similarity between the strain that we isolated from Daya Bay and Rhinomonas reticulata CCAP 979/15 Clone D (GenBank ID HF952563.1) (Figure 2E) was greater than 99%, therefore, the isolated microalgae strain was identified as a strain of Rhinomonas reticulata species and named Rhinomonas reticulata S6A. The 18s rRNA sequence has been submitted to the GenBank database under accession number OQ087126. The ecological environment of Daya Bay, a semi-enclosed shallow bay located in the northern part of the South China Sea, has been significantly affected by human activities (Yu et al., 2022). The eastern part of the Daya Bay petrochemical base releases organics into the surrounding environment throughout the year, thereby improving the tolerance of organisms in the nearby sea area to pollutants (Wu et al., 2010). The Daya Bay Nuclear Power Plant (DNPP) and Lingao Nuclear Power Plant (LNPP) on the northwest coast will discharge hot waste water at a rate of 315 m3/s during operation (Hu et al., 2023), which directly affects the carbon cycle in the nearby marine area and promotes the heterotrophic growth of organisms. Marine aquaculture industry is an important industry in Dapeng town and Nan’ao (two towns in the western coastal area), so the spreading of fertilizers on farmland has increased the concentration of inorganic nitrogen and organic pesticides in Daya Bay to increase (Wu and Wang, 2007). Water quality was particularly poor in summer, as the mean concentration of BOD5 was higher in summer than in other seasons (Wu et al., 2010), suggesting a high load of dissolved organic matter added from land-based resources, such as domestic wastewater, agricultural-related activities and industrial effluents (Wu et al., 2009). Thus, the uncoordinated nutrient structure and complex polluted environment like this may induce the stress tolerance of microbes enhanced (Han et al., 2022; Zhang et al., 2022), which in turn may harvest candidate organisms with biodegradable potential.
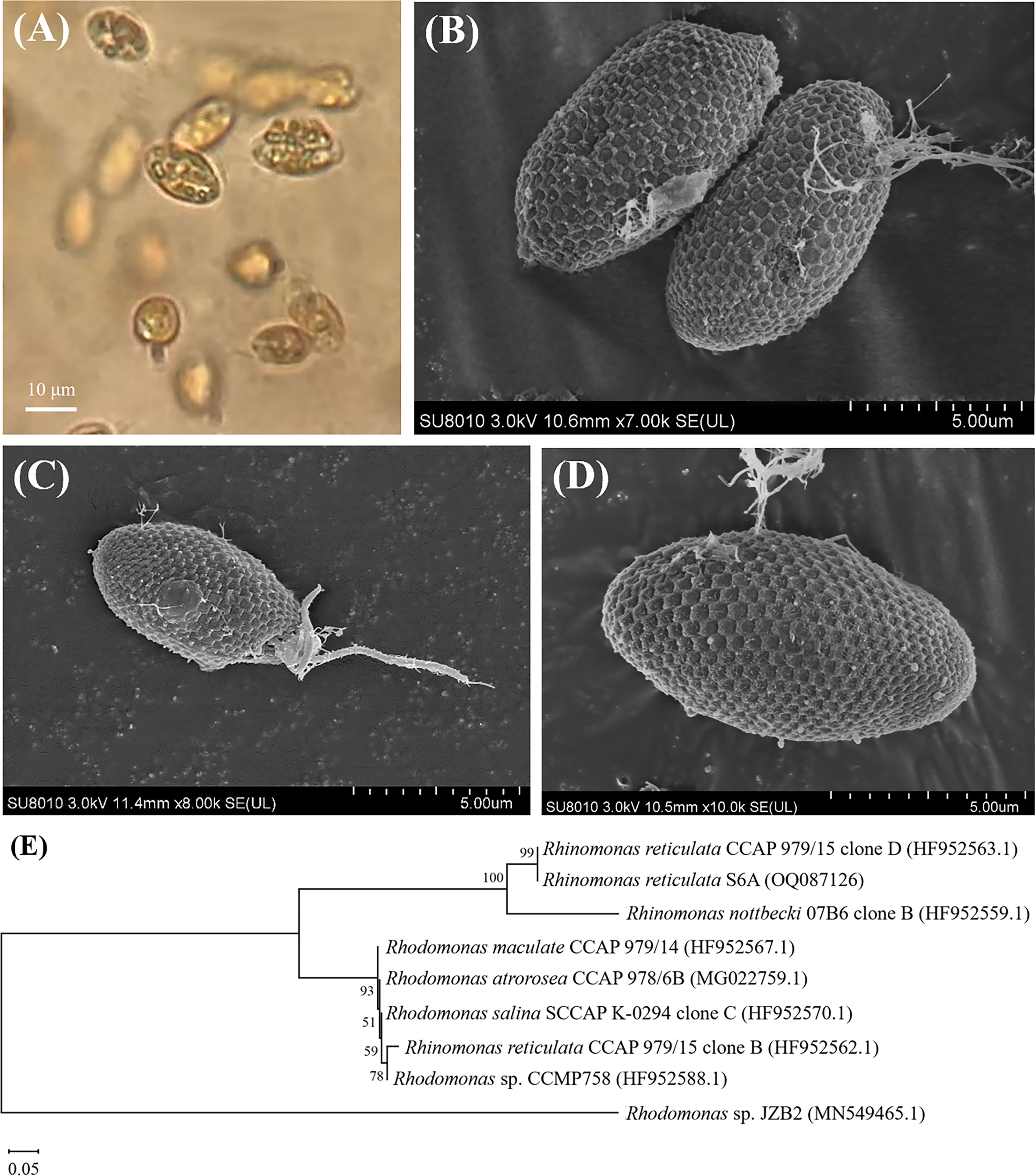
Figure 2 OM (A), SEM (B–D) images and the neighbor joining phylogenetic tree (E) based on the 18S rRNA sequences of R. reticulata S6A.
Surface images of R. reticulata S6A were clearly observed by SEM (Figures 2B–D): the strain was elliptical, with a length of 10.0-17.2 μm and a width of 4.4-8.8 μm, and two isometric flagella protruded from the oral groove, each with a length of 4.4-9.4 μm as reported (Won et al., 2013; Lubiana, 2018). Hexagonal plates were regular hexagons with a side length of 0.4 μm and some fibrils on the surface. R. reticulata S6A, belonging to Cryptophyta, Cryptophyceae, Pyrenomonadaceae, Rhinomonas sp., was observed to have strong motility under the OM (Figure 2A). Moreover, cryptophytes have strong adaptability, and can even become dominant populations in harsh environments (Xu et al., 2019), which can tolerate and even remove various pollutants. Compared with bacteria and fungi, algal-based biodegradation processes can realize the conversion of usable biomass and reduce CO2 emissions (Abdel-Shafy and Mansour, 2016). So far, varieties of microalgae have been used for the biodegradation of monocyclic aromatic hydrocarbons in seawater. For example, Phaeocystis globosa, Nannochloropsis oculata, Dunaliella salina and Platymonas subcordiformis can degrade nonylphenol (Wang et al., 2019a), and Isochrysis galbana can degrade phenol in seawater (Li et al., 2021b). In this study, R. reticulata S6A with the ability to degrade n-PBZ and i-PBZ was identified, providing an efficient and eco-friendly strategy for the removal of pollutants in seawater. Rhinomonas contains the water-soluble pigment Cr-phycoerythrin 545 (PE) that is highly valuable (30-150 $/mg) depending on the application (Majaneva et al., 2014; Latsos et al., 2021). Rhinomonas contains the water-soluble pigment Cr-phycoerythrin 545 (PE) that is highly valuable (30-150 $/mg) depending on the application (Majaneva et al., 2014; Latsos et al., 2021).
3.2 The growth inhibition of R. reticulata S6A by PBZs
The changes in cell density for R. reticulata S6A after exposure to n-PBZ or i-PBZ are shown in Figure 3. The growth of R. reticulata was significantly inhibited (P< 0.05) except for the treatments with the lowest PBZs concentrations (0.5 mg/L) on day 7. For both PBZs, there was little difference between the 0.5 mg/L group and the control after 7 days of exposure. However, the growth lag phase of the treatments of 1 mg/L, 5mg/L and 10mg/L were at least three days, and even, the growth inhibition rate more than 90% (P< 0.05) under the maximum concentration (20 mg/L) exposure. For i-PBZ, only the 10 mg/L and 20 mg/L treatments were significantly inhibited (P< 0.05) at the end of the test. The EC50 values of R. reticulata S6A exposed to n-PBZ and i-PBZ for 72 h and 96 h, respectively, are calculated in Table 1, where the acute toxicity values of other microalgae are also listed. Even though PBZs have similar toxicity levels due to isomerism, it seems that i-PBZ was less toxic than n-PBZ to an organism, and the EC50 value of i-PBZ was 1.44~2.11 times that of n-PBZ. On the one hand, the moderated toxic effects of monocyclic aromatics may be attributed to substituents possessing branched rather than straight chains (Mehta et al., 2002). On the other hand, the high toxicity of n-PBZ to microalgae may be closely related to the higher log KOW than that of i-PBZ (Peng et al., 2021). The n-octanol/water partition coefficient (KOW) is one of the important properties of organic compounds, and log KOW is commonly used to characterize the lipophilicity of compounds, that is, the higher the log KOW value, the better the bioaccumulation ability of organics in organisms (Duan et al., 2017). As such, it is easy to penetrate the biofilm and bind to the active site in the cell, causing physical damage and toxicity to microalgae (Lu et al., 2001). Also, the larger specific surface area of microalgae makes it easier to adsorb organic matter compared to other plankton. An early report recorded that i-PBZ (18h-LC50 = 0.012 mg/L) was four orders of magnitude more toxic to Colpidium colpoda than n-PBZ (18h-LC50 = 33.05 mg/L), which could be associated with i-PBZ degradation products produced by bacteria (Rogerson et al., 1983). The transformation process of i-PBZ by bacteria is dioxygenated and the products are more toxic than the phenols obtained from n-PBZ oxidized by monooxygenation (Peng et al., 2021). For example, i-PBZ is more easily oxidized to a hydroperoxide than n-PBZ, which may be very toxic in itself, or to a phenol (Rogerson et al., 1983), 3-isopropylcatechol and 3-methylcatechol have been reported as products of cumene degraded by Pseudomonas putida RE204 (Eaton et al., 1998). Of these, 3-isopropylcatechol were classified as chronically harmful to fish and green algae (Sun et al., 2019).
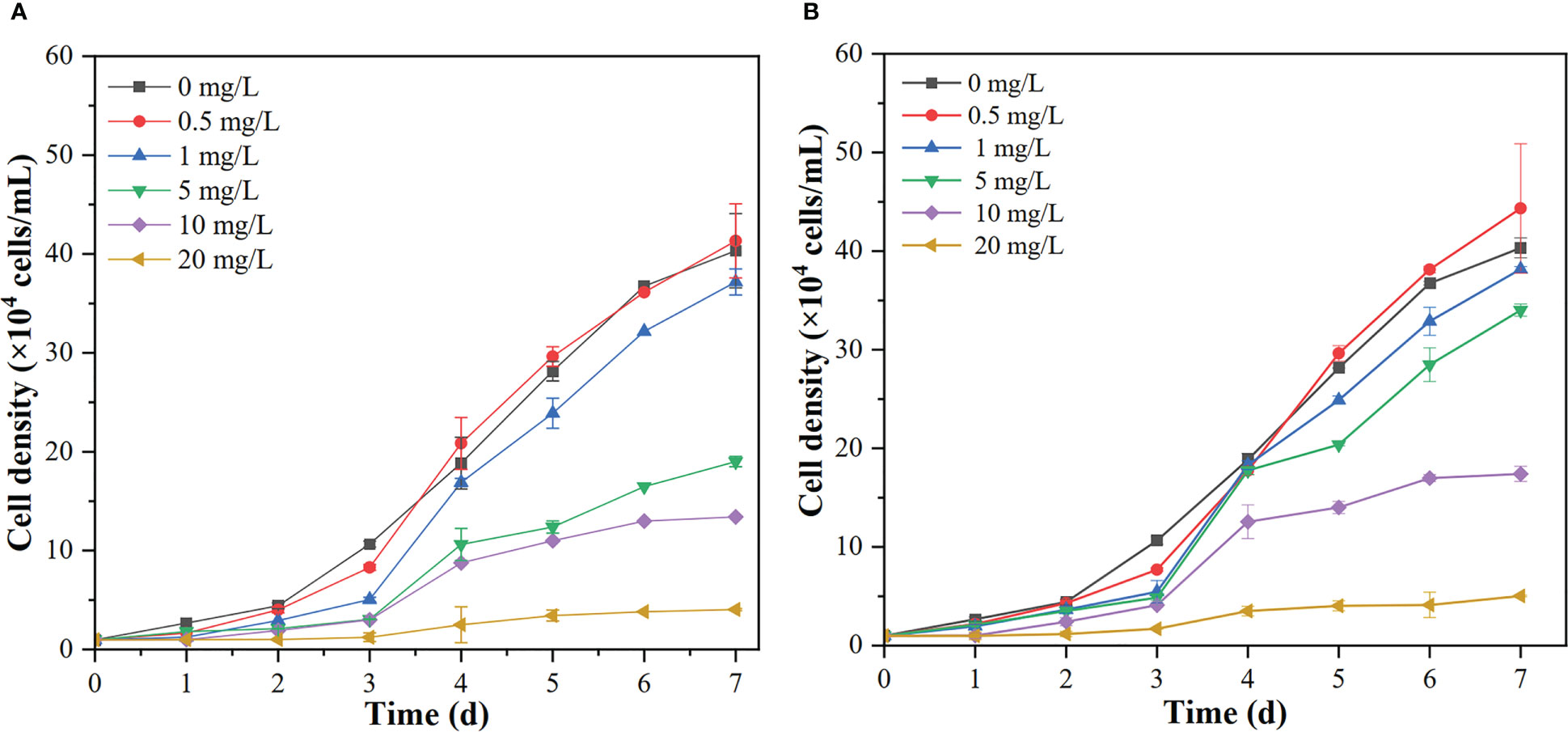
Figure 3 The growth curves of R. reticulata S6A at different concentrations of n-PBZ (A) and i-PBZ (B). Data corresponds to mean ± SD (n = 3).
As the cultivation time progressed, the microalgae began to recover from the stress induced by PBZs, resulting in an increase in the EC50 value in the exponential phase of R. reticulata S6A (Table 1). The increase in EC50 values clearly indicated the decrease in toxicity of PBZs, and the degradation products were non-toxic and/or less toxic than the parent compound. Xiong et al. (2017b) also reported similar observations of an increase in the EC50 values of the emerging contaminant, ciprofloxacin, with an increase in cultivation time. Li et al. (2021a) found that the overall toxicity (in terms of the inhibition rate of luminescence) of PBZs gradually decreased during natural attenuation in seawater, it would take at least 25 days for a complete reduction in toxicity to reach 0%, while this timeline could be accelerated by R. reticulata S6A.
To describe the kinetics of growth inhibition of S6A by PBZs, toxicant concentrations were chosen to be 0.5, 1, 5, 10 and 20 mg/L, and the number of microalgae inoculated was 104 cells/mL. Since the other initial culture conditions were consistent, the concentrations of toxicants were considered as a single substrate factor. The substrate inhibition of microbial growth may be competitive or non-competitive. The Haldane-Andrews, Webb, Yano, Aiba and Edwards models used in this paper are the extended form of the Monod model (Figures S1, S2). These models include the substrate inhibition term (Ki). A low value of Ki indicates that microbes are more sensitive to substrate inhibition, whereas a high value of Ki indicates that microbes are less sensitive to substrate inhibition (El-Naas et al., 2014). The fact that the Ki values of n-PBZ corresponding to each model are smaller than that of i-PBZ also indicates that R. reticulata S6A is more sensitive to n-PBZ than i-PBZ (Table 2). The optimal prediction was based on the Edwards (for n-PBZ) and Aiba (for i-PBZ) kinetic models, which satisfactorily fit the experimental data with higher coefficient (R2) of determination and lower residual sum of squares (RSS) compared to the other five kinetic models. In fact, R. reticulata S6A could grow normally (or be slightly promoted) under the stress of 0.5 mg/L PBZs, but it was strongly inhibited after exposure to 20 mg/L PBZs, and the inhibition constant parameters fitted by the two models were consistent. The first derivatives of the expressions of the Edwards (Eq. (10)) model and Aiba model (Eq. (11)) were as follows:
There, the critical substrate concentration (Sm) values were calculated as 2.42 and 2.78 mg/L, at which the specific growth reached its maximum value, could be obtained, at μ′ = 0. This represented the optimum concentration of n-PBZ and i-PBZ in seawater of the biodegradation by R. reticulata S6A predicted by the Edwards and Aiba kinetic models, respectively. It was further investigated that each value of Sm was similar to the acute toxicity value (96h-EC50) of n-PBZ (2.38 mg/L) or i-PBZ (3.65 mg/L) to R. reticulata S6A.
3.3 The degradation performance of PBZs by Rhinomonas reticulata S6A
As shown in Table 3, PBZs at initial concentrations of 0.5-20 mg/L could be degraded to varying degrees by R. reticulata S6A in 7 d. The removal efficiencies decreased from 72.2% and 55.6% to 15.2% and 24.5% respectively, when R. reticulata S6A was exposed to 20 mg/L n-PBZ and 20 mg/L i-PBZ for 7 d. The two isomers were also slightly degraded in sealed seawater without organisms, which is mutually confirmed with the results of the natural attenuation experiments (Li et al., 2021a). Due to the lack of hydrolysable functional groups and the inability to directly absorb visible light, the two PBZs are not directly hydrolyzed and photolyzed at ambient temperature and pressure (Mopper and Zhou, 1990; Peng, 2020). However, oxidation and indirect photolysis are possible transformation pathways of PBZs on the water surface based on the reaction of the gaseous PBZs molecule with photochemically generated hydroxyl radicals (·OH) (Mopper and Zhou, 1990), and this pathway was found to be inefficient according to Table 3.
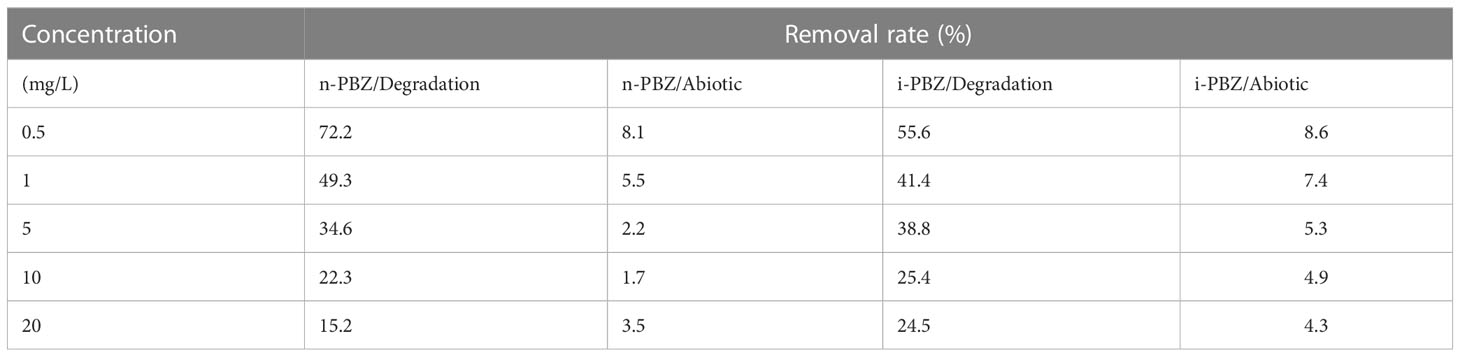
Table 3 The removal rate (including abiotic removal and degradation by R. reticulata S6A) at different initial concentrations of n-PBZ/i-PBZ after 7 d.
The biodegradation process of microalgae can occur either intracellularly or extracellularly, or a combination of both, where the initial degradation occurs extracellularly and the degradation products are further degraded intracellularly (Tiwari et al., 2017). At an initial concentration of 0.5-20 mg/L, the degradation curves of n-PBZ and i-PBZ over 7 d are shown in Figure 4. For n-PBZ, the concentration decreased significantly (P< 0.05) in the treatments of 0.5-5 mg/L after one day of degradation, while the high concentration groups (10 mg/L and 20 mg/L) decreased slowly until a rapidly decay after four or five days. Similar to bacteria, microalgae can release extracellular polymeric substances (EPS) and various types of soluble proteins, combined proteins, soluble polysaccharides, and combined polysaccharides (Vo et al., 2020). From this study, the degradation of n-PBZ in the first day may be the effect of some extracellular enzymes spontaneously secreted by microalgae in the culture of microalgae. For i-PBZ, the treatments of the lower concentration (0.5 mg/L and 1mg/L) began to degrade rapidly on the first day, while in the higher concentration groups (5 mg/L, 10 mg/L and 20 mg/L) were observed three or four days of lag phase (where no significant difference in daily concentrations). This apparent difference from n-PBZ was presumed to be due to the different types of degradative enzymes secreted by microalgae. Even if the reports on microalgal degradative enzymes remain to be scarce, there have been reports where the substrate can induce its degradative enzyme, e.g., several proteins related to oxidative detoxification (monooxygenase, alcohol dehydrogenase, benzaldehyde dehydrogenase, benzoate 1,2-dioxygenase, and catechol 2,3-dioxygenase) were significantly up-regulated in Thalassiosira sp. OUC2 during p-xylene biodegradation (Duan et al., 2020); the phenol biodegradation in Isochrysis galbana was mainly catalysed by intracellular enzymes (Wang et al., 2019b). Besides, it was recently shown that the microalgal degradation of benzo[a]pyrene by Selenastrum capricornutum could be partially attributed to exocellular enzymes (García de Llasera et al., 2022).
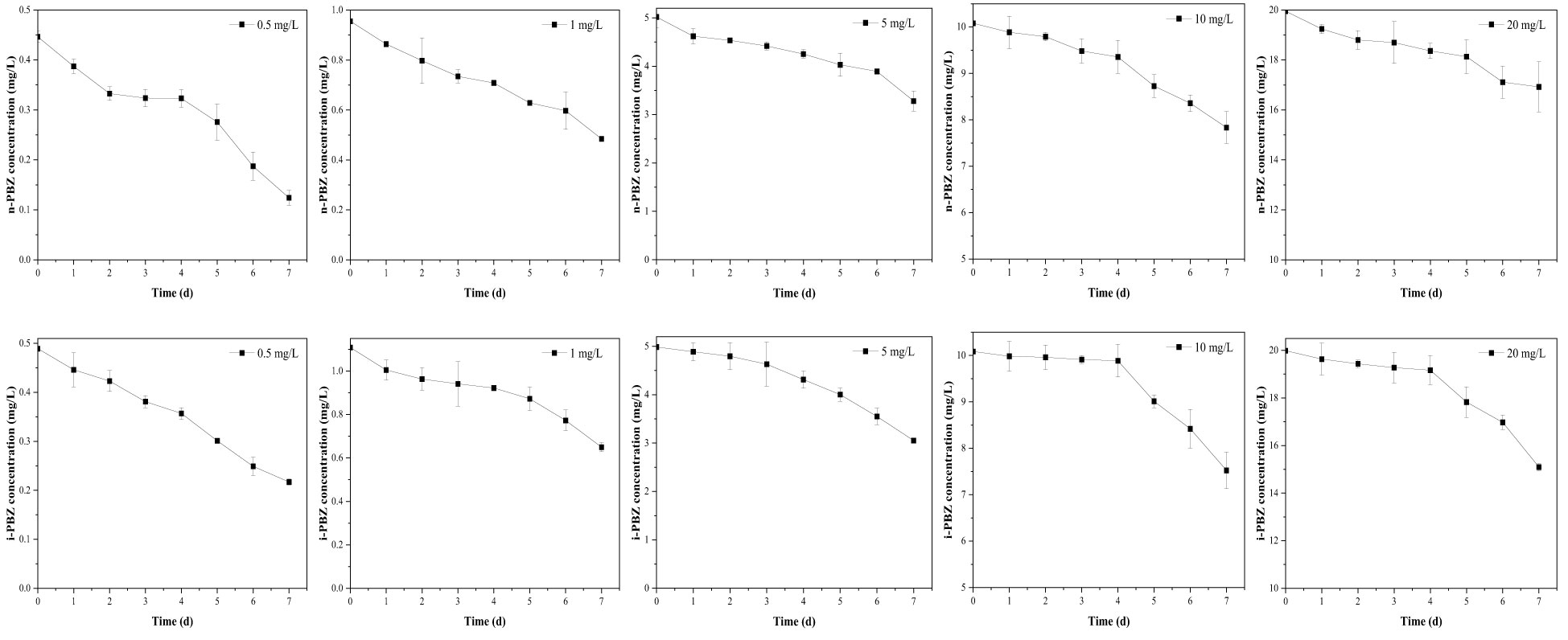
Figure 4 The degradation curves of different concentrations (including 0.5 mg/L, 1 mg/L, 5 mg/L, 10 mg/L, 20 mg/L) of n-PBZ and i-PBZ by R. reticulata S6A. Data corresponds to mean ± SD (n = 3).
To determine the degradation rate constant and half-life of PBZs in each treatment, three kinetic equations (zero-order model, first-order model, and pseudo first-order model) were applied in fitting the changes in concentration of each component with time from Figure 4. The fitting results (Table 4) showed that the predictions via the zero-order kinetic model satisfactorily matched the experimental data with higher correlation coefficients (R2 was between 0.923 and 0.999) compared to the other two kinetic models. The removal constants (K) for the n-PBZ and i-PBZ concentrations determined in this study ranged from 0.04-0.44/d and 0.04-1.37/d, respectively. The EC50 value characterizes the acute toxicity level of the toxicant; the lower the value, the higher the toxicity and also the higher the sensitivity of the organism to the toxicant. Environmental concentrations of toxicants below the EC50 had less effect on growth than those above the EC50, and microalgal growth was not strongly inhibited. At present, the half-life of n-PBZ is shorter than that of i-PBZ because R. reticulata S6A is more sensitive to n-PBZ (lower EC50) and more degradative enzymes may be induced. In general, excessive concentrations of pollutants can inhibit algal growth, increase oxidative stress, disrupt photosynthetic systems, and decrease degradation performance. In particular, R. reticulata S6A, which was more tolerant to i-PBZ, was more adaptable to the stress as culture time increased during the degradation of high concentrations of pollutants. Gibson et al. (1968) observed that the rate of oxidation of i-PBZ by Pseudomonas putida biotype B is faster than that of n-PBZ. This may be attributed to the different initial oxidation of isomers, which depends on the different substituents on the aromatic ring (Jigami et al., 1979). In addition, a gradual increase in k with increasing concentrations of PBZs were observed (Table 4), which also indicated that the efficiency of R. reticulata S6A for PBZs removal were related to the strength of the exogenous stimulus. Up to this point, it can be speculated that moderate microbial sensitivity is beneficial for the degradation of pollutants when the exposure concentration is below the 96h-EC50 value, and strong tolerance is a necessary condition for the degradation of higher pollutant concentrations. In the preliminary work to simulate a real-world propylene spill, 0.1 g of n-PBZ and i-PBZ were added to 10 L of natural seawater and mixed to saturation. After 24 hours of natural attenuation by the calm wind, samples were taken to detect concentrations in seawater of 3.67 and 3.94 mg/L (Li et al., 2021a). Therefore, once PBZs are spilled at sea, the concentration that can be dealt with immediately the next day does not exceed 5 mg/L. However, without bioaugmentation technology, the biodegradation by native organisms of PBZs in natural seawater were very sluggish, with rates of only 0.06/d (n-PBZ) and 0.08/d (i-PBZ). Under the effect of R. reticulata S6A, the biodegradation rates of two PBZs (5 mg/L) were 0.27/d (n-PBZ) and 0.39/d (i-PBZ), respectively, which can effectively promote the removal of PBZs.
3.4 Effect of initial inoculation density
As shown in Figure 5, the growth lag phase of microalgae was obviously shortened when the inoculation density was increased so that it entered the exponential phase on the first day (the initial inoculation density more than 5×104 cells/mL), while the initial inoculation density with the maximum specific growth rate (r) was 104 cells/mL (n-PBZ: 1.116/d, i-PBZ: 1.230/d). In the treatment with the maximum inoculation density (30×104 cells/mL), the plateau phase appeared on the second day of culture and the r value was the minimum, then, the cell density began to decrease on the fourth day (Table 5). Similarly, it was found that the environment of high cell density may be overcrowded which weakens photosynthesis due to shading effects and relative lack of nutrients, which eventually hinders the rapid growth of cells (Liu et al., 2022). In turn, a high initial inoculation density will contribute to the stress resistance of microalgae (Wang et al., 2016). Compared to the more toxic n-PBZ, i-PBZ had less inhibitory effect on R. reticulata S6A, especially for the high inoculation density treatment, and the growth lag phase of the microalgae was short. The treatment with the initial inoculation density of 5×104 and 105 cells/mL reached the peak (45.2×104 cells/mL and 51.4×104 cells/mL) on the fourth and fifth day, respectively, which was 2.43 and 2.23 times that of n-PBZ at the same period.
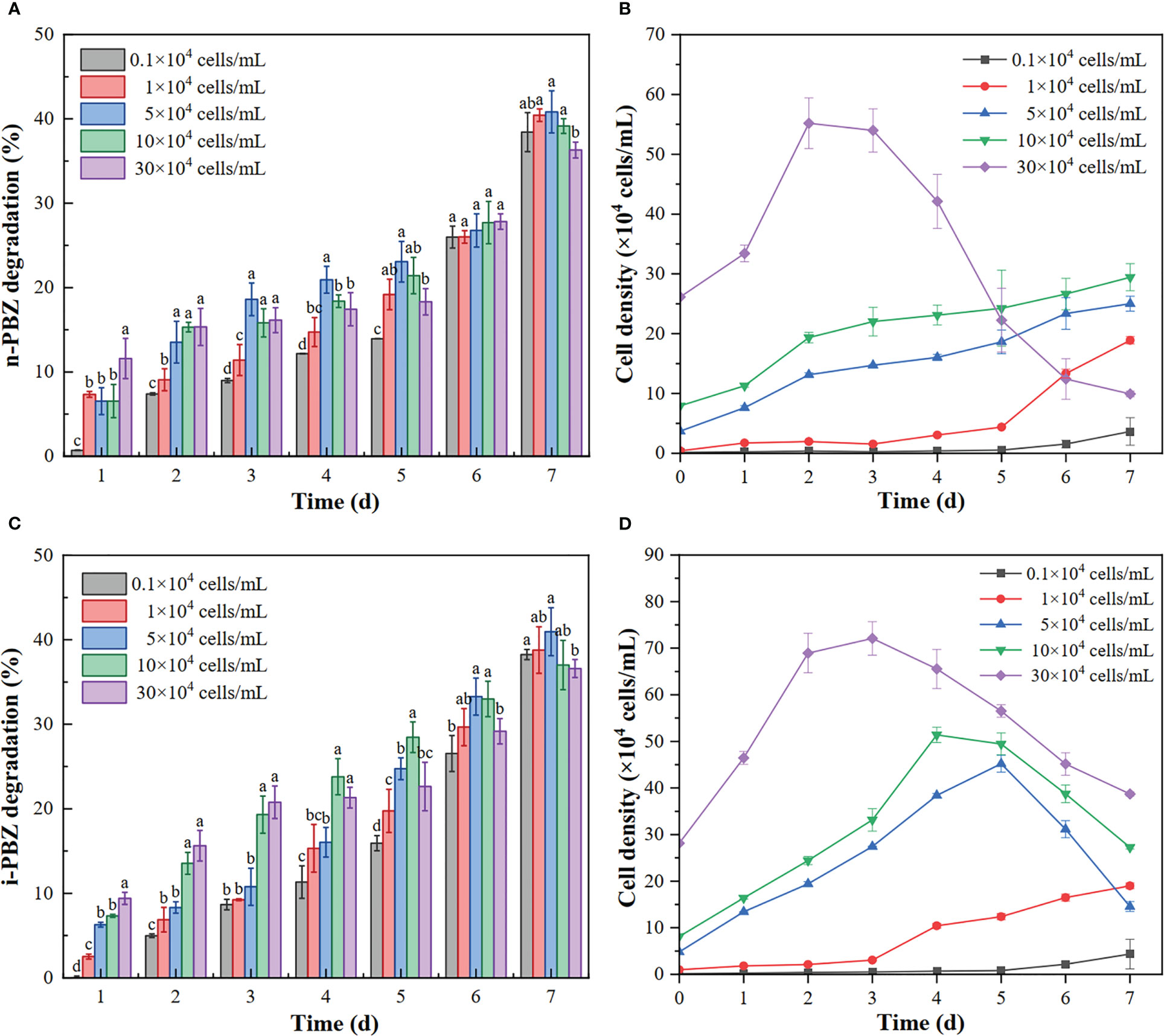
Figure 5 The degradation rate of n-PBZ (A) and i-PBZ (C) and the growth curve of R. reticulata S6A (B: n-PBZ; D: i-PBZ) at different initial inoculation densities. The initial concentration of n-PBZ or i-PBZ was 5 mg/L. Letters indicate statistical difference with 95% confidence interval (P < 0.05, n = 3).
Extensive studies on extracellular proteins in bacterial and fungal biofilms have shown that extracellular enzymes are involved in the degradation of biopolymers, including polysaccharides, proteins, and nucleic acids (Xiao and Zheng, 2016). Interestingly, in the present study, the concentration of degradation on the first day was positively correlated with the initial inoculation density for n-PBZ (R2 = 0.932). The reason may be that the degradation enzymes of n-PBZ were present in the EPS of R. reticulata S6A. Degradation enzymes in the EPS were important in the life cycle of the host algal cells because a wide range of enzymes were present in the EPS and may have been responsible for degrading the EPS to provide energy and carbon sources for the host cells to grow (Zhou et al., 2022). As the initial inoculation density of R. reticulata S6A was increased, the degradation rate of both PBZs tended to increase and then decrease after 7 d (Figure 5), where high algal inoculation density can cause hypoxia in the water column and reduce pollutant removal rates (Zhou et al., 2022). And the degradation rate of PBZs was the lowest when the initial inoculation density was the highest. The photosynthetic pigment content is a sensitive parameter for various environmental stresses (Ravi Kiran and Venkata Mohan, 2021). Figure 6 showed the variation of photosynthetic pigment content with initial inoculum density. It was found that the mean PerCP and PE decreased with increasing initial inoculum density, with a peak at 1×104 cells/mL, and the photosynthetic capacity of the highest density group under n-PBZ stress decreased by about half. This indicated that the photosynthetic capacity may be more significantly affected by the shading effect under n-PBZ stress. For i-PBZ, the change in PE content was more pronounced, with the 1×104 cells/mL group being significantly higher than the other treatment groups, which may be an expression of the resilience of microalgae at lower densities (Srinithi et al., 2023). Studies have shown that phenanthrene concentrations > 5 mg/L affect the photosynthetic activity of Azolla filiculoides due to oxidative damage of phenanthrene on plant cells (Kösesakal and Seyhan, 2023). Furthermore, the microalgae could increase their photosynthetic activity after tolerating the environmental stress (Zhao et al., 2023). In addition, PBZs were degraded in the medium and the negative effect of PBZs on microalgae were reduced when the culture time were increased.
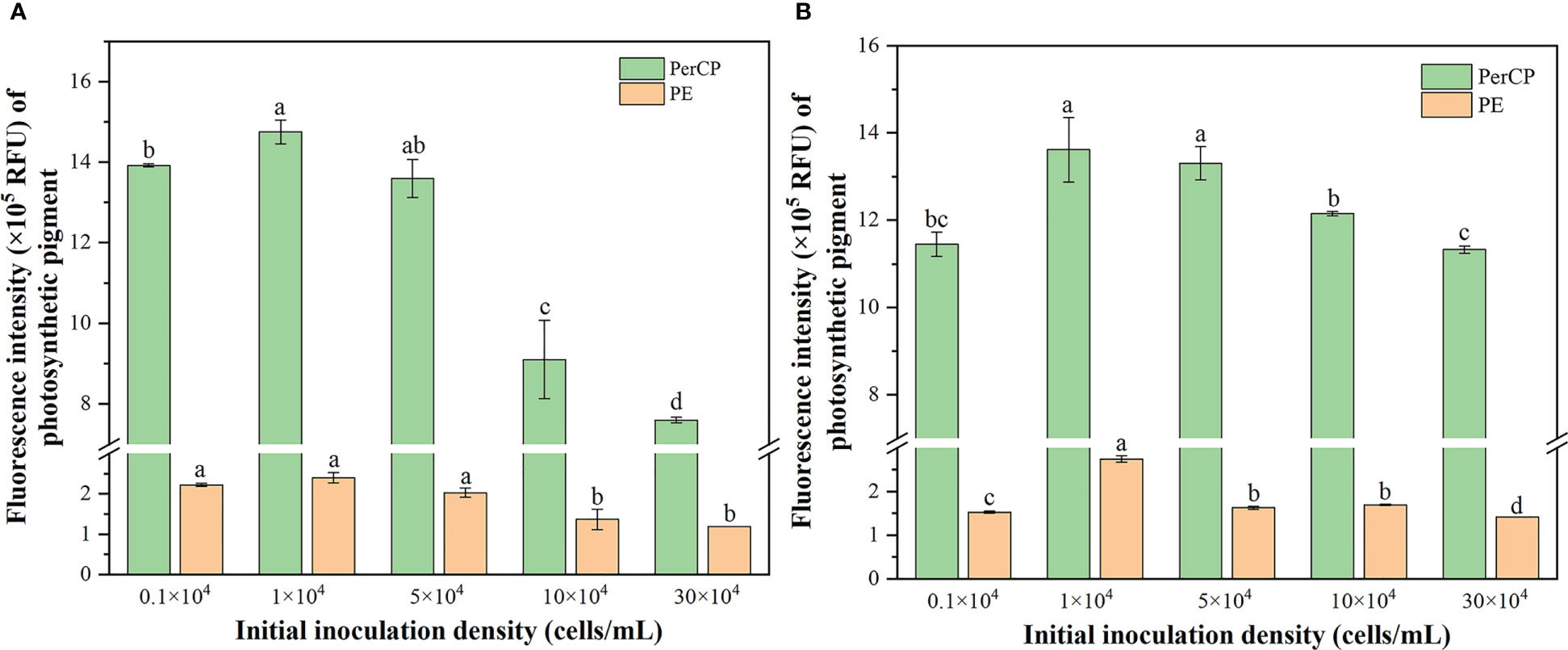
Figure 6 Changes in the mean of PerCP and PE fluorescence of R. reticulata S6A exposed to n-PBZ (A) and i-PBZ (B) at different initial inoculation densities after 7 days.
Overall, no obvious difference in the degradation effect of PBZs was observed at the inoculation density in the range of 103~105 cells/mL, and the degradation rates of n-PBZ and i-PBZ were 38.43~40.83% and 38.25~40.95%, respectively, at 7 d. Some previous studies have found similar results. Likewise, the specific growth rate and the degradation rate of p-xylene of Rhodomonas sp. JZB-2 were the lowest when the initial inoculation density was as high as 106 cells/mL (Liu et al., 2022). In addition, the degradation rate of benzene, toluene, ethylbenzene and xylene (BTEX) would no longer increase when the initial inoculation density of Janibacter sp. SB2 was greater than 2.0 × 107 cells/mL (Jin et al., 2013). In general, higher inoculation density in bioremediation has the potential to induce more degradative enzymes, thereby improving the degradation efficiency of pollutants (Robinson et al., 2009), however, there was no doubt that the technical complication and consumption of pre-cultivation will be increased (Liu et al., 2022). Since an excessive initial inoculation density may have an inhibitory effect on pollutant degradation, and both cost and ecological risks need to be considered, 104 cells/mL was selected as the initial inoculation density for the degradation of PBZs in the follow-up study. This minimizes additional organisms while ensuring stress tolerance to pollutants.
4 Conclusions
An indigenous microalgal strain isolated from Daya Bay, R. reticulata S6A, was used to degrade the n-PBZ and i-PBZ which have been of concern due to recent C9 aromatics spills or other leaks. It has been found that 72.2% and 55.6% of n-PBZ and i-PBZ (with an initial concentration of 0.5 mg/L) can be removed in 7 days. The growth inhibition kinetics of R. reticulata S6A could be described by Edwards model (R2 = 0.998) and Aiba model (R2 = 0.999) under the stress of n-PBZ and i-PBZ, respectively, and the optimal concentration for degradation was 2.42 mg/L (n-PBZ) and 2.78 (i-PBZ). For R. reticulata S6A, n-PBZ (96h - EC50 = 2.38 mg/L) was more toxic than i-PBZ (96h - EC50 = 3.65 mg/L). The degradation process of PBZs conforms to the zero-order kinetic model, and k increased with increasing initial concentration. The moderate increase in inoculation density accelerates the degradation of PBZs, with a maximum specific growth rate (r) of 1.116/d (n-PBZ) and 1.230/d (i-PBZ) at an initial inoculation density of 104 cells/mL, while excessive inoculation density (> 105 cells/mL) of algae reduced the removal performance of microalgae to contaminants. Certainly, more research is needed to reveal the mechanism of the removal pathway, but this work provides an important basis for the degradation potential of PBZs by eukaryotic microalgae, which could be very useful in environmental applications.
Data availability statement
The datasets presented in this study can be found in online repositories. The names of the repository/repositories and accession number(s) can be found in the article/Supplementary Material.
Author contributions
SD and JC: conceptualization, field work, methodology, formal analysis, writing original draft, visualization. FM: conceptualization, methodology, funding acquisition, writing. HL: field work, methodology, formal analysis. HC: field work. YX: manuscript review and editing. All authors contributed to the article and approved the submitted version.
Funding
This work was financially supported by the National Natural Science Foundation of China (Grant no. 42077335).
Conflict of interest
The authors declare that the research was conducted in the absence of any commercial or financial relationships that could be construed as a potential conflict of interest.
Publisher’s note
All claims expressed in this article are solely those of the authors and do not necessarily represent those of their affiliated organizations, or those of the publisher, the editors and the reviewers. Any product that may be evaluated in this article, or claim that may be made by its manufacturer, is not guaranteed or endorsed by the publisher.
Supplementary material
The Supplementary Material for this article can be found online at: https://www.frontiersin.org/articles/10.3389/fmars.2023.1171944/full#supplementary-material
References
Abdel-Shafy H. I., Mansour M. S. M. (2016). A review on polycyclic aromatic hydrocarbons: source, environmental impact, effect on human health and remediation. Egypt. J. Pet. 25, 107–123. doi: 10.1016/j.ejpe.2015.03.011
Acton D. W., Barker J. F. (1992). In situ biodegradation potential of aromatic hydrocarbons in anaerobic groundwaters. J. Contam. Hydrol. 9, 325–352. doi: 10.1016/0169-7722(92)90002-V
Aiba S., Shoda M., Nagatani M. (1968). Kinetics of product inhibition in alcohol fermentation. Biotechnol. Bioeng. 10, 845–864. doi: 10.1002/bit.260100610
Andrews J. F. (1968). A mathematical model for the continuous culture of microorganisms utilizing inhibitory substrates. Biotechnol. Bioeng. 10, 707–723. doi: 10.1002/bit.260100602
Aoki H., Kimura T., Habe H., Yamane H., Kodama T., Omori T. (1996). Cloning, nucleotide sequence, and characterization of the genes encoding enzymes involved in the degradation of cumene to 2-hydroxy-6-oxo-7-methylocta-2,4-dienoic acid in Pseudomonas fluorescens IP01. J. Ferment. Bioeng. 81, 187–196. doi: 10.1016/0922-338X(96)82207-0
Birch H., Hammershøj R., Comber M., Mayer P. (2017). Biodegradation of hydrocarbon mixtures in surface waters at environmentally relevant levels – effect of inoculum origin on kinetics and sequence of degradation. Chemosphere 184, 400–407. doi: 10.1016/j.chemosphere.2017.05.169
Carvajal A., Akmirza I., Navia D., Pérez R., Muñoz R., Lebrero R. (2018). Anoxic denitrification of BTEX: biodegradation kinetics and pollutant interactions. J. Environ. Manage. 214, 125–136. doi: 10.1016/j.jenvman.2018.02.023
Chen B., Yeh H.-Y., Huang C.-H., Lung W. Q. C., Chen Y.-J., Lee P.-T., et al. (2022). Effects of indoor culture conditions on growth and phycoerythrin content of Proteomonas sulcata (Cryptophyta) assessed by flow cytometry. J. Appl. Phycol. 34, 1201–1213. doi: 10.1007/s10811-022-02714-1
Chen J., Zheng F., Guo R. (2015). Algal feedback and removal efficiency in a sequencing batch reactor algae process (SBAR) to treat the antibiotic cefradine. PloS One 10, e0133273. doi: 10.1371/journal.pone.0133273
Cho K., Lee C.-H., Ko K., Lee Y.-J., Kim K.-N., Kim M.-K., et al. (2016). Use of phenol-induced oxidative stress acclimation to stimulate cell growth and biodiesel production by the oceanic microalga Dunaliella salina. Algal Res. 17, 61–66. doi: 10.1016/j.algal.2016.04.023
Di Marzio W., Saenz M. E. (2006). QSARs for aromatic hydrocarbons at several trophic levels. Environ. Toxicol. 21, 118–124. doi: 10.1002/tox.20163
Donkin P., Widdows J., Evans S. V., Worrall C. M., Carr M. (1989). Quantitative structure-activity relationships for the effect of hydrophobic organic chemicals on rate of feeding by mussels (Mytilus edulis). Aquat. Toxicol. 14, 277–293. doi: 10.1016/0166-445X(89)90021-0
Duan W., Du S., Meng F., Peng X., Peng L., Lin Y., et al. (2020). ). the pathways by which the marine diatom Thalassiosira sp. OUC2 biodegrades p-xylene, combined with a mechanistic analysis at the proteomic level. Ecotoxicol. Environ. Saf. 198, 110687. doi: 10.1016/j.ecoenv.2020.110687
Duan W., Meng F., Lin Y., Wang G. (2017). Toxicological effects of phenol on four marine microalgae. Environ. Toxicol. Pharmacol. 52, 170–176. doi: 10.1016/j.etap.2017.04.006
Eaton R. W., Selifonova O. V., Gedney R. M. (1998). Isopropylbenzene catabolic pathway in Pseudomonas putida RE204: nucleotide sequence analysis of the ipb operon and neighboring DNA from pRE4. Biodegradation 9, 119–132. doi: 10.1023/A:1008386221961
Edwards V. H. (1970). The influence of high substrate concentrations on microbial kinetics. Biotechnol. Bioeng. 12, 679–712. doi: 10.1002/bit.260120504
El-Naas M. H., Acio J. A., El Telib A. E. (2014). Aerobic biodegradation of BTEX: progresses and prospects. J. Environ. Chem. Eng. 2, 1104–1122. doi: 10.1016/j.jece.2014.04.009
Eriksson S., Ankner T., Abrahamsson K., Hallbeck L. (2005). Propylphenols are metabolites in the anaerobic biodegradation of propylbenzene under iron-reducing conditions. Biodegradation 16, 253–263. doi: 10.1007/s10532-004-1278-z
European Maritime Spatial Planning Platform (European MSP Platform) (2021) Transport and marine conservation. Available at: https://maritime-spatial-planning.ec.europa.eu/sector-information/transport-and-marine-conservation (Accessed 1, 2023).
Finney D. J. (1947). Probit analysis; a statistical treatment of the sigmoid response curve (Oxford, England: Macmillan).
Firth M. J. (2008). Derivation of a chronic reference dose and reference concentration for trimethylbenzenes and C9 aromatic hydrocarbon solvents. Regul. Toxicol. Pharmacol. 52, 248–256. doi: 10.1016/j.yrtph.2008.08.017
Franke C., Studinger G., Berger G., Böhling S., Bruckmann U., Cohors-Fresenborg D., et al. (1994). The assessment of bioaccumulation. Chemosphere 29, 1501–1514. doi: 10.1016/0045-6535(94)90281-X
Galassi S., Mingazzini M., Vigano` L., Cesareo D., Tosato M. L. (1988). Approaches to modeling toxic responses of aquatic organisms to aromatic hydrocarbons. Ecotoxicol. Environ. Saf. 16, 158–169. doi: 10.1016/0147-6513(88)90030-9
García de Llasera M. P., Fuentes Pérez A. C., Peralta Marín G., Beltrán Calva E. G. (2022). First evidence of extracellular enzymatic degradation of benzo(a)pyrene by the phytoplankton species Selenastrum capricornutum and the influence of temperature. Environ. Adv. 8, 100246. doi: 10.1016/j.envadv.2022.100246
Gibson D. T., Koch J. R., Kallio R. E. (1968). Oxidative degradation of aromatic hydrocarbons by microorganisms. i. enzymic formation of catechol from benzene. Biochem. 7, 2653–2662. doi: 10.1021/bi00847a031
Glickman A. H., Alexander H. C., Buccafusco R. J., Morris C. R., Francis B. O., Surprenant D. C., et al. (1995). An evaluation of the aquatic hazard of cumene (Isopropyl benzene). Ecotoxicol. Environ. Saf. 31, 287–289. doi: 10.1006/eesa.1995.1076
Gollapudi B. B., Williams A. L., Bus J. S. (2021). A review of the genotoxicity of the industrial chemical cumene. Mutat. Res. Rev. Mutat. Res. 787, 108364. doi: 10.1016/j.mrrev.2021.108364
Guillard R. R. L. (1975). “Culture of phytoplankton for feeding marine invertebrates,” in Culture of marine invertebrate animals (New York: Plenum Press).
Habe H., Kimura T., Nojiri H., Yamane H., Omori T. (1996). Cloning and nucleotide sequences of the genes involved in the meta-cleavage pathway of cumene degradation in Pseudomonas fluorescens IP01. J. Ferment. Bioeng. 81, 247–254. doi: 10.1016/0922-338X(96)82216-1
Han T., Qi Z., Shi R., Liu Q., Dai M., Huang H. (2022). Effects of seawater temperature and salinity on physiological performances of swimming shelled pteropod Creseis acicula during a bloom period. Front. Mar. Sci. 9. doi: 10.3389/fmars.2022.806848
Herrero M., Stuckey D. C. (2015). Bioaugmentation and its application in wastewater treatment: a review. Chemosphere 140, 119–128. doi: 10.1016/j.chemosphere.2014.10.033
Homem V., Santos L. (2011). Degradation and removal methods of antibiotics from aqueous matrices – a review. J. Environ. Manage. 92, 2304–2347. doi: 10.1016/j.jenvman.2011.05.023
Hu S., Zhang C., Liu Q., Li T., Huang H., Liu S. (2023). Short-term responses of phytoplankton size-fractionated structure and photosynthetic physiology to thermal effluent in a subtropical coastal bay. Front. Mar. Sci. 10. doi: 10.3389/fmars.2023.1102686
International Oil Pollution Compensation Funds (IOPC Funds) (2019) HNS finder: propylbenzene (all isomers). Available at: https://www.hnsconvention.org/hns-finder/detail/substance/12-1887/?pr=1&tm=b (Accessed 20, 2023).
Jegan J., Vijayaraghavan K., Senthilkumar R., Velan M. (2010). Naphthalene degradation kinetics of Micrococcus sp., isolated from activated sludge. CLEAN – Soil Air Water 38, 38. doi: 10.1002/clen.200900148
Jigami Y., Kawasaki Y., Omori T., Minoda Y. (1979). Coexistence of different pathways in the metabolism of n-propylbenzene by Pseudomonas sp. Appl. Environ. Microbiol. 38, 38. doi: 10.1128/aem.38.5.783-788.1979
Jin H. M., Choi E. J., Jeon C. O. (2013). Isolation of a BTEX-degrading bacterium, Janibacter sp. SB2, from a sea-tidal flat and optimization of biodegradation conditions. Bioresour. Technol. 145, 145. doi: 10.1016/j.biortech.2013.02.004
Kao C. M., Prosser J. (2001). Evaluation of natural attenuation rate at a gasoline spill site. J. Hazard. Mater. 82, 275–289. doi: 10.1016/S0304-3894(00)00361-7
Koh L. M., Khor S. M. (2023). “Concept and significance of microbial consortium in the biodegradation process,” in Handbook of biodegradable materials. Eds. Ali G. A. M., Makhlouf A. S. H. (Cham: Springer International Publishing), 137–177. doi: 10.1007/978-3-031-09710-2_67
Kösesakal T., Seyhan M. (2023). Phenanthrene stress response and phytoremediation potential of free-floating fern Azolla filiculoides lam. Int. J. Phytorem. 25, 207–220. doi: 10.1080/15226514.2022.2069224
Kureel M. K., Geed S. R., Giri B. S., Rai B. N., Singh R. S. (2017). Biodegradation and kinetic study of benzene in bioreactor packed with PUF and alginate beads and immobilized with Bacillus sp. M3. Bioresour. Technol. 242, 92–100. doi: 10.1016/j.biortech.2017.03.167
Latsos C., van Houcke J., Blommaert L., Verbeeke G. P., Kromkamp J., Timmermans K. R. (2021). Effect of light quality and quantity on productivity and phycoerythrin concentration in the cryptophyte Rhodomonas sp. J. Appl. Phycol. 33, 729–741. doi: 10.1007/s10811-020-02338-3
Li H., Tan J., Sun T., Wang Y., Meng F. (2021b). Acclimation of Isochrysis galbana parke (Isochrysidaceae) for enhancing its tolerance and biodegradation to high-level phenol in seawater. Ecotoxicol. Environ. Saf. 207, 111571. doi: 10.1016/j.ecoenv.2020.111571
Li D., Wu J., Liu J., Li A., Meng F. (2021a). Natural attenuation characteristics and comprehensive toxicity changes of C9 aromatics under simulated marine conditions. J. Environ. Sci. 109, 26–35. doi: 10.1016/j.jes.2021.02.029
Liu J., Du S., Meng F., Li D. (2022). Enhanced biodegradation of para-xylene by the marine cryptophyte Rhodomonas sp. JZB-2 by changing the concentrations of nutrients, iron, and vitamins. J. Appl. Phycol. 34, 2107–2116. doi: 10.1007/s10811-022-02775-2
Lu G.-H., Yuan X., Zhao Y.-H. (2001). QSAR study on the toxicity of substituted benzenes to the algae (Scenedesmus obliquus). Chemosphere 44, 437–440. doi: 10.1016/S0045-6535(00)00214-9
Lubiana K. M. F. (2018). Taxonomy and molecular phylogeny of cryptophyceae (Cryptophyta) from cultures (Brazil: Instituto de Biociências). doi: 10.11606/T.41.2018.tde-01062018-102632
Majaneva M., Remonen I., Rintala J.-M., Belevich I., Kremp A., Setälä O., et al. (2014). Rhinomonas nottbecki n. sp. (Cryptomonadales) and molecular phylogeny of the family pyrenomonadaceae. J. Eukaryotic Microbiol. 61, 480–492. doi: 10.1111/jeu.12128
Mehta A., Ouzounov S., Jordan R., Simsek E., Lu X., Moriarty R. M., et al. (2002). Imino sugars that are less toxic but more potent as antivirals, In vitro, compared with n-n-Nonyl DNJ. Antivir. Chem. Chemother. 13, 299–304. doi: 10.1177/095632020201300505
Mopper K., Zhou X. (1990). Hydroxyl radical photoproduction in the sea and its potential impact on marine processes. Science 4981, 661–664. doi: 10.1126/science.250.4981.661
Neuparth T., Moreira S., Santos M. M., Reis-Henriques M. A. (2011). Hazardous and noxious substances (HNS) in the marine environment: prioritizing HNS that pose major risk in a European context. Mar. pollut. Bull. 62, 21–28. doi: 10.1016/j.marpolbul.2010.09.016
Ogata M., Fujisawa K., Ogino Y., Mano E. (1984). Partition coefficients as a measure of bioconcentration potential of crude oil compounds in fish and shellfish. Bull. Environ. Contam. Toxicol. 33, 561–567. doi: 10.1007/BF01625584
Organisation for Economic Co-operation and Development (OECD) (2022) The ocean. ocean shipping and shipbuilding. Available at: https://www.oecd.org/ocean/topics/ocean-shipping/ (Accessed 21, 2022).
Osundeko O., Dean A. P., Davies H., Pittman J. K. (2014). Acclimation of microalgae to wastewater environments involves increased oxidative stress tolerance activity. Plant Cell Physiol. 55, 1848–1857. doi: 10.1093/pcp/pcu113
Oukarroum A., Halimi I., Siaj M. (2018). Cellular responses of Chlorococcum sp. algae exposed to zinc oxide nanoparticles by using flow cytometry. Water Air Soil pollut. 230, 230. doi: 10.1007/s11270-018-4051-3
Peng L. H. (2020). Toxicological effects of the main components of C9 aromatics on marine microalgae (China: Ocean University of China).
Peng L., Lin Y., Meng F., Wu J., Zheng Y., Sun T., et al. (2021). Environmental fate and aquatic effects of propylbenzenes and trimethylbenzenes: a review. Chemosphere 264, 128533. doi: 10.1016/j.chemosphere.2020.128533
Ravi Kiran B., Venkata Mohan S. (2021). Photosynthetic transients in Chlorella sorokiniana during phycoremediation of dairy wastewater under distinct light intensities. Bioresour. Technol. 340, 125593. doi: 10.1016/j.biortech.2021.125593
Robinson C., Brovelli A., Barry D. A., Li L. (2009). Tidal influence on BTEX biodegradation in sandy coastal aquifers. Adv. Water Resour. 32, 16–28. doi: 10.1016/j.advwatres.2008.09.008
Rocha G. S., Parrish C. C., Lombardi A. T., da Graça Gama Melão M. (2018). Biochemical and physiological responses of Selenastrum gracile (Chlorophyceae) acclimated to different phosphorus concentrations. J. Appl. Phycol. 30, 2167–2177. doi: 10.1007/s10811-018-1418-1
Rogerson A., Shiu W. Y., Huang G. L., Mackay D., Berger J. (1983). Determination and interpretation of hydrocarbon toxicity to Ciliate protozoa. Aquat. Toxicol. 3, 215–228. doi: 10.1016/0166-445X(83)90042-5
Roose P., Brinkman U. A. T. (1998). Improved determination of VOCs in marine biota by using on-line purge and trap-gas chromatography-mass spectrometry. Analyst. 123, 2167–2173. doi: 10.1039/A803610A
Roose P., Thuyne G. V., Belpaire C., Raemaekers M., Brinkman U. A. T. (2003). Determination of VOCs in yellow eel from various inland water bodies in Flanders (Belgium). J. Environ. Monit. 5, 876–884. doi: 10.1039/B307862K
Ruiz O. N., Radwan O., Striebich R. C. (2021). GC–MS hydrocarbon degradation profile data of Pseudomonas frederiksbergensis SI8, a bacterium capable of degrading aromatics at low temperatures. Data Brief 35, 106864. doi: 10.1016/j.dib.2021.106864
Sakulthaew C., Comfort S., Chokejaroenrat C., Harris C., Li X. (2014). A combined chemical and biological approach to transforming and mineralizing PAHs in runoff water. Chemosphere 117, 1–9. doi: 10.1016/j.chemosphere.2014.05.041
Shahi A., Rai B. N., Singh R. S. (2016). Analysis of metabolites and carbon balance in the biofilteration of cumene using loofa sponge as biofilter media. Appl. Biochem. Biotechnol. 180, 338–348. doi: 10.1007/s12010-016-2102-z
Srinithi R., Sangavi P., Nachammai K. T., Gowtham Kumar S., Langeswaran K. (2023). “Chapter 22 - perspective of algae materials 2.0,” in Algae materials developments in applied microbiology and biotechnology. Eds. Arunkumar K., Arun A., Raja R., Palaniappan R. (India: Academic Press), 383–397. doi: 10.1016/B978-0-443-18816-9.00009-5
Sun J., Wei B., Mei Q., An Z., Wang X., He M. (2019). Ozonation of 3-methylcatechol and 4-methylcatechol in the atmosphere and aqueous particles: mechanism, kinetics and ecotoxicity assessment. Chem. Eng. J. 358, 456–466. doi: 10.1016/j.cej.2018.10.074
Sutherland D. L., Ralph P. J. (2019). Microalgal bioremediation of emerging contaminants - opportunities and challenges. Water Res. 164, 114921. doi: 10.1016/j.watres.2019.114921
Tian Y., Zou J., Feng L., Zhang L., Liu Y. (2019). Chlorella vulgaris enhance the photodegradation of chlortetracycline in aqueous solution via extracellular organic matters (EOMs): role of triplet state EOMs. Water Res. 149, 35–41. doi: 10.1016/j.watres.2018.10.076
Tiwari B., Sellamuthu B., Ouarda Y., Drogui P., Tyagi R. D., Buelna G. (2017). Review on fate and mechanism of removal of pharmaceutical pollutants from wastewater using biological approach. Bioresour. Technol. 224, 1–12. doi: 10.1016/j.biortech.2016.11.042
USEPA (2023)Chemicals: isopropylbenzene. In: ECOTOX knowledgebase. Available at: https://cfpub.epa.gov/ecotox/search.cfm (Accessed 20, 2023).
Vo H. N. P., Ngo H. H., Guo W., Nguyen K. H., Chang S. W., Nguyen D. D., et al. (2020). Micropollutants cometabolism of microalgae for wastewater remediation: effect of carbon sources to cometabolism and degradation products. Water Res. 183, 115974. doi: 10.1016/j.watres.2020.115974
Walker J. D., Calomiris J. J., Herbert T. L., Colwell R. R. (1976). Petroleum hydrocarbons: degradation and growth potential for Atlantic ocean sediment bacteria. Mar. Biol. 34, 1–9. doi: 10.1007/BF00390780
Wang Y., Meng F., Li H., Zhao S., Liu Q., Lin Y., et al. (2019b). Biodegradation of phenol by Isochrysis galbana screened from eight species of marine microalgae: growth kinetic models, enzyme analysis and biodegradation pathway. J. Appl. Phycol. 31, 445–455. doi: 10.1007/s10811-018-1517-z
Wang L., Xiao H., He N., Sun D., Duan S. (2019a). Biosorption and biodegradation of the environmental hormone nonylphenol by four marine microalgae. Sci. Rep. 9, 5277. doi: 10.1038/s41598-019-41808-8
Wang L., Xue C., Wang L., Zhao Q., Wei W., Sun Y. (2016). Strain improvement of Chlorella sp. for phenol biodegradation by adaptive laboratory evolution. Bioresour. Technol. 205, 264–268. doi: 10.1016/j.biortech.2016.01.022
Wei L., Li H., Lu J. (2021). Algae-induced photodegradation of antibiotics: a review. Environ. pollut. 272, 115589. doi: 10.1016/j.envpol.2020.115589
Wells P. G., Duce R. A., Huber M. E. (2002). Caring for the sea–accomplishments, activities and future of the united nations GESAMP (the joint group of experts on the scientific aspects of marine environmental protection). Ocean Coast. Manage. 45, 77–89. doi: 10.1016/S0964-5691(02)00047-9
Won N. S., Donghee G., Misun S., Woongghi S. (2013). Ultrastructure of the flagellar apparatus in Rhinomonas reticulata var. atrorosea (Cryptophyceae, cryptophyta). Algae 28, 331–341. doi: 10.4490/algae.2013.28.4.331
Wu M.-L., Wang Y.-S. (2007). Using chemometrics to evaluate anthropogenic effects in daya bay, China. Estuarine Coast. Shelf Sci. 72, 732–742. doi: 10.1016/j.ecss.2006.11.032
Wu M.-L., Wang Y.-S., Sun C.-C., Wang H., Dong J.-D., Yin J.-P., et al. (2010). Identification of coastal water quality by statistical analysis methods in daya bay, south China Sea. Mar. pollut. Bull. 60, 852–860. doi: 10.1016/j.marpolbul.2010.01.007
Wu M.-L., Wang Y.-S., Sun C.-C., Wang H., Lou Z.-P., Dong J.-D. (2009). Using chemometrics to identify water quality in daya bay, China. Oceanologia 51, 217–232. doi: 10.5697/oc.51-2.217
Xiao R., Zheng Y. (2016). Overview of microalgal extracellular polymeric substances (EPS) and their applications. Biotechnol. Adv. 34, 1225–1244. doi: 10.1016/j.biotechadv.2016.08.004
Xiong J.-Q., Kurade M. B., Jeon B.-H. (2017a). Biodegradation of levofloxacin by an acclimated freshwater microalga, Chlorella vulgaris. Chem. Eng. J. 313, 1251–1257. doi: 10.1016/j.cej.2016.11.017
Xiong J.-Q., Kurade M. B., Kim J. R., Roh H.-S., Jeon B.-H. (2017b). Ciprofloxacin toxicity and its co-metabolic removal by a freshwater microalga Chlamydomonas mexicana. J. Hazard. Mater. 323, 212–219. doi: 10.1016/j.jhazmat.2016.04.073
Xiong J.-Q., Kurade M. B., Jeon B. H. (2018). Can microalgae remove pharmaceutical contaminants from water? Trends Biotechnol. 36, 3044. doi: 10.1016/j.tibtech.2017.09.003
Xu K., Hu S., Tang X. (2019). The complete plastid genome of a marine microalgae Cryptophyceae sp. CCMP2293 (Cryptophyta). Mitochondrial DNA Part B 4, 2159–2160. doi: 10.1080/23802359.2019.1623725
Yano T., Nakahara T., Kamiyama S., Yamada K. (1966). Kinetic studies on microbial activities in concentrated solutions. Agric. Biol. Chem. 30, 42–48. doi: 10.1080/00021369.1966.10858549
Yu X., Sun L., Zhu X., Bian G., Zhou W., Cao Q., et al. (2022). Distribution characteristics and risk assessment of heavy metals in seawater, sediment and shellfish in the inner and outer daya bay, guangdong. Front. Mar. Sci. 9. doi: 10.3389/fmars.2022.1064287
Yuan W., Li Y., Dagaut P., Wang Y., Wang Z., Qi F. (2017). A comprehensive experimental and kinetic modeling study of n-propylbenzene combustion. Combust. Flame 186, 178–192. doi: 10.1016/j.combustflame.2017.08.010
Zhang J., Fu D., Wu J. (2012). Photodegradation of norfloxacin in aqueous solution containing algae. J. Environ. Sci. 24, 743–749. doi: 10.1016/S1001-0742(11)60814-0
Zhang L., Li G., Xiang C., Huang Y., Fu X., Zheng C., et al. (2022). Plankton metabolism in coastal waters of the guangdong-Hong Kong-Macao greater bay: regional variance and driving factors. Front. Mar. Sci. 9. doi: 10.3389/fmars.2022.844970
Zhao Q., Zhu L., Jiang L., Zhao Q. (2023). Photosynthetic and transcriptomic responses of Chlorella sp. to tigecycline. Algal Res. 71, 71. doi: 10.1016/j.algal.2023.103033
Keywords: propylbenzenes, Rhinomonas reticulata, biodegradation, aquatic toxicity toxicity, kinetic
Citation: Du S, Cui J, Meng F, Li H, Cui H and Xia Y (2023) Bioremediation of propylbenzenes by a novel marine microalga Rhinomonas reticulata S6A isolated from Daya Bay: acute toxicity, growth kinetics and biodegradation performance. Front. Mar. Sci. 10:1171944. doi: 10.3389/fmars.2023.1171944
Received: 22 February 2023; Accepted: 21 April 2023;
Published: 08 May 2023.
Edited by:
Nadia A. Samak, Chinese Academy of Sciences (CAS), ChinaReviewed by:
Haikun Zhang, Chinese Academy of Sciences (CAS), ChinaXupeng Cao, Chinese Academy of Sciences (CAS), China
El-Sayed Salama, Lanzhou University, China
Copyright © 2023 Du, Cui, Meng, Li, Cui and Xia. This is an open-access article distributed under the terms of the Creative Commons Attribution License (CC BY). The use, distribution or reproduction in other forums is permitted, provided the original author(s) and the copyright owner(s) are credited and that the original publication in this journal is cited, in accordance with accepted academic practice. No use, distribution or reproduction is permitted which does not comply with these terms.
*Correspondence: Fanping Meng, bWVuZ2ZhbnBpbmdAb3VjLmVkdS5jbg==
†These authors have contributed equally to this work