Compounding deep sea physical impacts on marine microbial motility
- 1Marine Biology Research Division, Scripps Institution of Oceanography, University of California, San Diego, La Jolla, CA, United States
- 2Institute for Chemical Research, Kyoto University, Kyoto, Japan
- 3Department of Physics, Kindai University, Osaka, Japan
Introduction: Approximately three-fourths of all pelagic marine prokaryotes live in the deep sea, an environment characterized by high hydrostatic pressure and, in most cases, low temperature. Labile organic matter is often scarce within these settings, providing a competitive advantage to motile cells that can access the nutrients within a greater seawater volume. Because many cells present at depth are shallow water-adapted microbes descending from more productive surface waters, deep-sea conditions could significantly reduce their motility and, consequently, their biogeochemical activities.
Methods: In this study, we address this possibility by examining the impact of deep-sea physical conditions on the motility of three representative marine microbes belonging to the cosmopolitan genera Halomonas, Alcanivorax, and Shewanella. Growth-dependent motility agar assays and growth-independent microscopy assays were employed at four pressures and two temperatures.
Results: At pressures equivalent to bathyal and abyssal depths (10 – 50 Megapascals), decreases in temperature (30°C – 4°C or 23°C – 7°C depending on the assay) had a greater negative impact on motility than pressure. In addition, the high-pressure and low-temperature impacts were additive. Exposure to high pressure and/or low temperature had varying degrees of effect on flagellar function, depending on the strain and the magnitude of the applied stress. These ranged from short-term impacts that were quickly reversible to long-term impacts that were detrimental to the function of the flagellum, leading to complete loss of motility.
Discussion: These findings highlight the sensitivity of motility systems of piezosensitive mesophilic marine bacteria to the combined pressure/temperature conditions present in the deep sea, phenotypes that in situ are likely to manifest themselves in the modulation of diverse microbial activities.
1 Introduction
The ocean – particularly at the microscale – is remarkably heterogeneous, and marine microorganisms face a wide range of physical and chemical gradients (Stocker, 2012). To thrive in such a dynamic environment, microbes utilize motility and chemotaxis to search out favorable niches (Grossart et al., 2001; Fenchel, 2002; Stocker and Seymour, 2012). For motile microbes, this microenvironment is largely defined by that organism’s motility range (Stocker, 2012); the more motile a microbe (i.e., the faster their swimming speed and the more chemotactic they are), the larger the volume they can exploit. Additionally, motility and chemotaxis can enhance a microbe’s ability to degrade organic matter by aiding in attachment (Grossart et al., 2001; Kiørboe et al., 2002; Mueller et al., 2007; Dewangan and Conrad, 2020) and increasing encounter rates (Stocker et al., 2008; Lambert et al., 2019; Raina et al., 2023). A significant fraction of marine prokaryotes are found in the deep sea (>1,000 meters below sea level, mbsl). Deep-sea pelagic prokaryotes account for nearly 75% of the total prokaryotic biomass and 50% of the total prokaryotic production in the global ocean (Aristegui et al., 2009), and abundance estimates for benthic deep-sea environments are comparable to those for the marine water column (Kallmeyer et al., 2012; Parkes et al., 2014). In the deep sea, where labile organic matter is typically less abundant (Wakeham and Lee, 1993; Lochte et al., 1998; Lee, 2002), microbial motility may be particularly important (Barbara and Mitchell, 2003; Mitchell and Kogure, 2006; Campanaro et al., 2008; Lauro and Bartlett, 2008; Stocker et al., 2008; Taylor and Stocker, 2012; Peoples and Bartlett, 2017; Keegstra et al., 2022). These environments are broadly characterized by low temperature (between 0 – 4°C) and high hydrostatic pressures (38 Megapascals (MPa) on average) (Knauss and Garfield, 2017), two physical parameters known to impact a wide range of cellular processes, including motility (Bartlett, 1992; Bartlett, 2002).
Studies evaluating the activity of deep-sea microbial communities have found conflicting results, with some studies showing high microbial activity under in situ conditions (Poremba, 1994; Patching and Eardly, 1997; Peoples et al., 2018; Garel et al., 2019), and others suggesting that deep-sea microbial communities are more active at atmospheric pressure (Amano et al., 2022). These seemingly contradictory findings are attributed to differences in hydrological conditions. When waters are stratified, autochthonous deep-sea microbes better adapted to in situ conditions thrive. However, deep-sea microbial communities in areas impacted by perturbations, such as deep mixing events and large influxes of sinking particles, tend to be less adapted to deep ocean conditions, presumably because they are more heavily influenced by surface-derived piezosensitive and mesophilic microbes (Bianchi and Garcin, 1994; Tamburini et al., 2013; Garel et al., 2021). It has additionally been shown that particle-associated microbes, which can be carried from shallower waters to the deep, are negatively impacted by increases in hydrostatic pressure (Turley, 1993; Tamburini et al., 2006; Tamburini et al., 2009). Because marine microorganisms play a pivotal role in global biogeochemical cycling and ocean productivity (Azam, 1998; Strom, 2008), having a detailed understanding of pressure and temperature (P-T) impacts on the cellular processes - including motility - of piezosensitive mesophilic microorganisms is of fundamental importance, including their consequences to assessments of deep ocean carbon sequestration (Levin, 1992; Azam and Malfatti, 2007).
The pressure at the ocean surface (atmospheric pressure) is 0.1 MPa, and hydrostatic pressure increases by 10 MPa every 1,000 meters of water depth. Studies of non-piezophilic mesophiles indicate that bacterial motility is one of the most temperature-sensitive (Adler and Templeton, 1967; Maeda et al., 1976; Nishiyama et al., 2013) and pressure-sensitive (Meganathan and Marquis, 1973; Bartlett, 1992; Bartlett, 2002; Eloe et al., 2008) cellular processes. Temperature changes of just a few degrees can dramatically change swimming speeds (Adler and Templeton, 1967), and pressures as low as 10 MPa (equivalent to ~1,000 mbsl) have been shown to impact both the formation of newly synthesized flagella and the function of preexisting flagella (Meganathan and Marquis, 1973). While there is relatively little information on the impacts of pressure on the motility of organisms from other domains, there is some evidence that shallow-water protists may have similar levels of pressure-sensitive motility (Otter and Salmon, 1979). Since lower temperatures decrease pressure tolerance in many marine and terrestrial bacterial species (Zobell and Johnson, 1949; Zobell and Oppenheimer, 1950; Paul and Morita, 1971; Yayanos et al., 1983; Trent and Yayanos, 1985; Yayanos, 1986; Marquis, 1994; Pledger et al., 1994; Fichtel et al., 2015) and decreasing temperature and increasing pressure have additive effects on membrane physical structure (Allen et al., 1999; Denich et al., 2003; Cario et al., 2015; Manisegaran et al., 2019), both parameters should be viewed in concert with one another. Only a handful of studies have assessed the impact of high hydrostatic pressure on microbial motility (Regnard, 1891; Zobell and Oppenheimer, 1950; Zobell, 1970; Meganathan and Marquis, 1973; Eloe et al., 2008; Wang et al., 2008; Nishiyama and Sowa, 2012; Nishiyama et al., 2013). Of these, three have focused on the enteric bacterium Escherichia coli (Meganathan and Marquis, 1973; Nishiyama and Sowa, 2012; Nishiyama et al., 2013), two have utilized piezophilic species (Eloe et al., 2008; Wang et al., 2008), and the remaining have provided very preliminary results using piezosensitive marine bacteria (Regnard, 1891; Zobell and Oppenheimer, 1950; Zobell, 1970). While Eloe et al. (2008) includes the non-piezophilic Photobacterium profundum 3TCK in their analysis as a comparison to the piezophilic P. profundum SS9, this is the only non-piezophilic marine microorganism that has been utilized in quantitative studies of pressure impacts on motility. Additionally, while previous work has assessed the influence of low temperature and high hydrostatic pressure on microbial flagellar rotation of the non-piezophilic mesophile E. coli (Nishiyama et al., 2013), to our knowledge, the combined influence of high pressure and low temperature on marine microbial motility is unknown.
In this study, we employ both culture- and microscopy-based analyses to examine the effects of high hydrostatic pressure and low temperature on the motility of three marine bacteria belonging to the genera Halomonas, Alcanivorax, and Shewanella. This work is part of a multi-institution consortium research program associated with the Gulf of Mexico Research Initiative (GoMRI) (Weiman et al., 2021), and one of these strains (Halomonas sp. strain 10BA) has been the subject of inquiries from other groups also belonging to this consortium (Dewangan and Conrad, 2018; Dewangan and Conrad, 2019; Dewangan and Conrad, 2020; Dewangan et al., 2021; Zhao and Ford, 2022). The strains were isolated from the Gulf of Mexico following the 2010 Deepwater Horizon (DWH) oil spill at depths ranging from 46 to 1509 mbsl, with corresponding in situ temperatures ranging from ~23-30°C (Camilli et al., 2010) to ~4-7°C (Hazen et al., 2010). They were chosen because they belong to some of the more prominent microbial genera that responded to the influx of hydrocarbons in the Gulf of Mexico following DWH (Hazen et al., 2010; Kostka et al., 2011; Gutierrez et al., 2013; Kimes et al., 2013; Joye et al., 2016; Yang et al., 2016). Additionally, the genera Halomonas, Alcanivorax, and Shewanella are widely distributed within the global ocean, inhabiting various environments throughout the water column (Golyshin et al., 2003; Arahal and Ventosa, 2006; Lemaire et al., 2020).
2 Materials and methods
2.1 Study strains
The strains used in this study (Table 1) were generously provided by Dr. Romy Chakraborty and Dr. Gary Andersen at the Lawrence Berkeley National Laboratory. They were isolated from the Gulf of Mexico following the DWH oil spill using hydrocarbon-soaked bead traps (Andersen et al., 2012). Briefly, Bio-Traps® (Molecular Insights, Knoxville, TN, USA) were baited with MC-252 crude oil and deployed at multiple depths on a drilling platform approximately 600 meters from the Deepwater Horizon platform. The traps were deployed from August through September 2010, and isolates were subsequently obtained on media that contained MC-252 as the sole carbon source. Three strains – 10BA GOM-1509m, 18 GOM-1509m, and 36 GOM-46m (herein referred to as strains 10BA, 18, and 36, respectively) – were chosen for this study. Strains 10BA and 18 were isolated from 1509 mbsl, and strain 36 was isolated from 46 mbsl.

Table 1 Summary of study strains utilized in this study, including closest cultured relative (based on 16S rRNA sequencing and Maximum-Likelihood phylogenetic analysis), isolation depth (meters), optimum growth temperature (°C), optimum growth pressure (Megapascals, MPa), and flagellation type.
The 16S rRNA gene sequences of the study strains were determined following PCR using the general bacterial primers 27F and 1492R (Lane, 1991). To obtain near full-length 16S rRNA sequences, Sanger sequencing was performed in both the forward and reverse direction (Retrogen, Inc., San Diego, CA, USA) and the sequences were aligned using MEGA X version 10.2.6 (Kumar et al., 2018). These partial 16S sequences were deposited into the National Center for Biotechnology Information (NCBI) GenBank repository with the accession numbers MZ424455 for strain 10BA, MZ424456 for strain 18, and MZ424457 for strain 36. MEGA X was used to align these sequences with similar 16S rRNA sequences obtained from GenBank and to construct a maximum-likelihood phylogenetic tree (Figure 1). The flagellation of each strain (Table 1) was determined using the previously described NanoOrange flagellar staining method (Grossart et al., 2000) using a Nikon C1 confocal fluorescence microscope (Nikon, Tokyo, Japan).
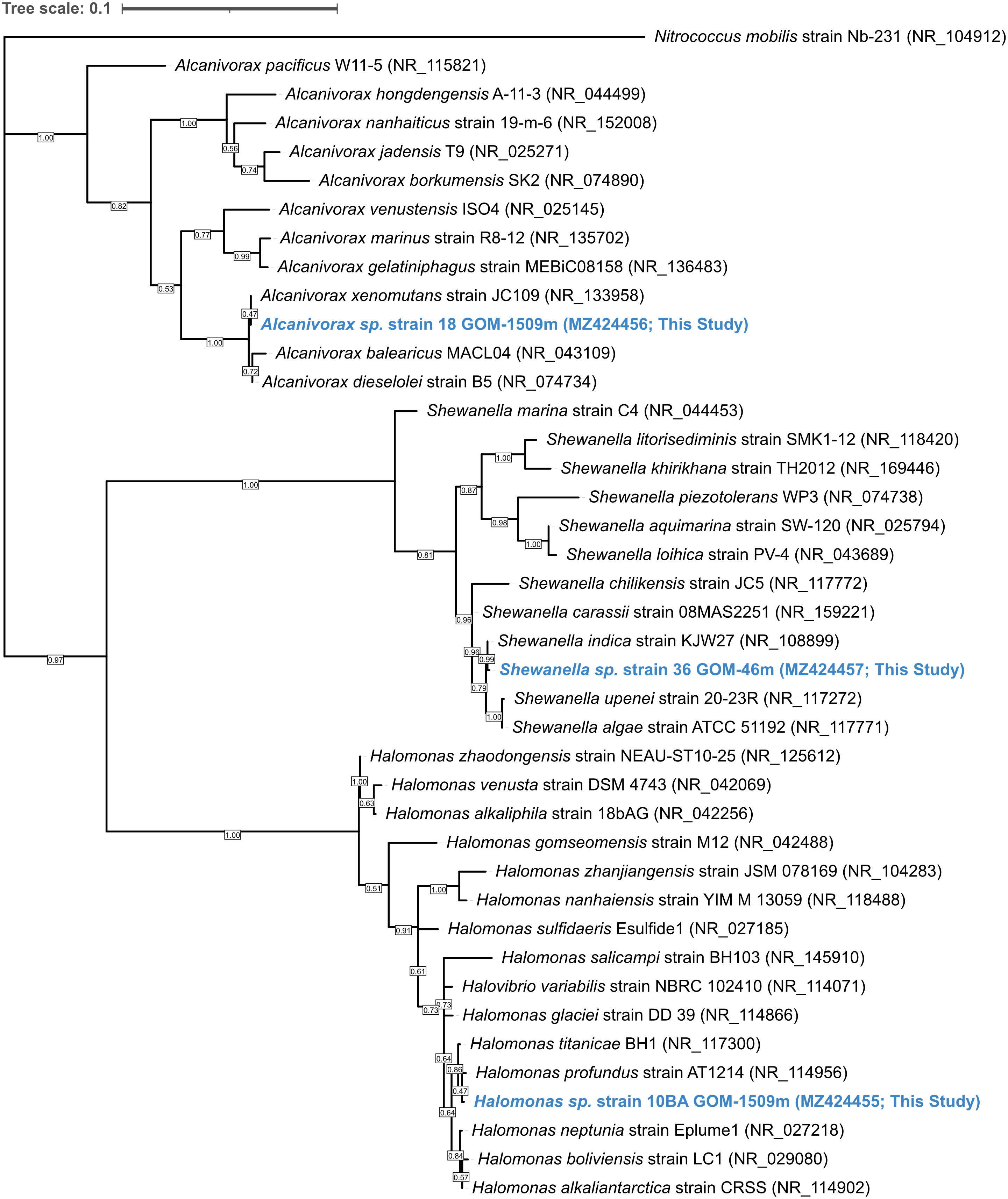
Figure 1 Maximum-likelihood phylogenetic tree based on 16S rRNA gene sequences showing the relationship between strains 10BA GOM-1509m, 18 GOM-1509m, and 36 GOM-46m (blue) and members of the genera Halomonas, Alcanivorax, and Shewanella, respectively. GenBank accession numbers used are shown in parentheses. The sequence of Nitrococcus mobilis strain Nb-231 (NR_104912.1) was used as an outgroup. Bootstrap values (based on 1000 replications) greater than 50% are shown at branch points. Bar, 0.01 substitutions per nucleotide position.
2.2 Growth conditions
All three strains were grown in Marine Broth 2216 (HiMedia Laboratories, Mumbai, India) at a concentration of 28 g/L. For assessments of pressure and temperature impacts on growth rate (Section 2.3) and long-term motility (Section 2.4.1), the medium was supplemented with a buffer (100 mM HEPES, pH 7.5) and an alternate electron acceptor (100 mM NaNO3). As standard techniques for cultivating microorganisms at high hydrostatic pressure limit oxygen availability (Yayanos, 2001), these amendments help support growth in an oxygen-limited environment. When these amendments were added, they were added for all pressure-temperature conditions tested, including atmospheric pressure (0.1 MPa).
2.3 Pressure & temperature effects on growth
The growth rate of each study strain under various temperature (4°C and 30°C) and pressure (0.1, 10, 25, and 50 MPa) conditions was determined (Figure 2, solid lines). While these pressures correspond to depths beyond those relevant to the DWH oil spill, it is common for non-piezophiles to tolerate relatively high pressures (Yayanos and Pollard, 1969). Additionally, these pressures are relevant to other bathyal and abyssal environments where Halomonas, Alcanivorax, and Shewanella are found (Golyshin et al., 2003; Arahal and Ventosa, 2006; Lemaire et al., 2020), and we wanted to investigate P-T impacts across a broader ecological area.
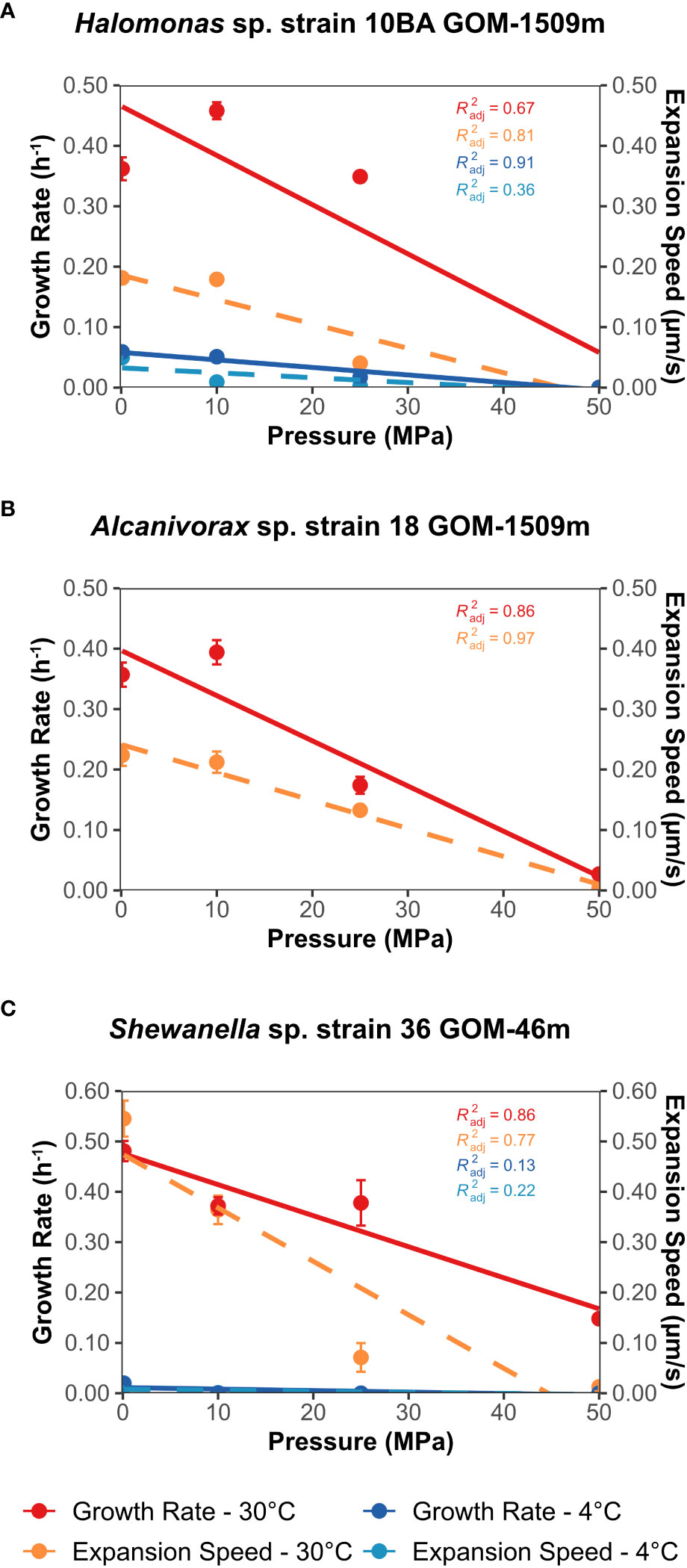
Figure 2 Growth rate (solid lines, h-1) and expansion speed (dashed lines, µm/second) as a function of pressure (MPa) at both 4°C and 30°C. Expansion speed was derived from the long-term motility vial experiments. (A) Halomonas sp. strain 10BA GOM-1509m; (B) Alcanivorax sp. strain 18 GOM-1509m; (C) Shewanella sp. strain 36 GOM-46m. Error bars represent the standard deviation of the growth rate and expansion speed across three biological replicates. Data were fitted with a linear regression line; associated R2 values are shown.
To determine the growth rate of these strains as a function of pressure and temperature, growth curves were generated using standard techniques for high-pressure incubation (see Supplementary Methods). From these growth curves, the growth rate was determined using R-shiny-microorganisms (Garel et al., 2022), a Shiny-web application that employs a logistic Verhulst model to estimate growth rate. Default parameters were used; for P-T conditions with little or no growth, the application reported an error, which we report as a growth rate of zero. Linear regression lines were added to the plots of growth rate (hr-1) vs. pressure (MPa) (Figure 2).
To assist in our analysis of the impacts of pressure and temperature on the growth of our three study strains, we calculated the P50, which we define as the pressure at which the growth rate is 50% less than that observed at atmospheric pressure (see Supplementary Methods).
2.4 Pressure & temperature effects on motility
Similar to the growth assays (Section 2.3), we calculated the P50 of swimming motility for both the long-term and short-term motility assays (see Supplementary Methods). In this case, we define the P50 as the pressure at which motility (i.e., expansion speed, swimming speed, or percentage of motile cells) is 50% less than that observed at atmospheric pressure.
2.4.1 Long-term (growth dependent) effects
Two approaches were employed to evaluate the effects of pressure and temperature on the motility of the study strains. The first method was an adaptation of that used by Eloe et al. (2008) to follow the growth and movement of cells swimming through a semi-solid agar matrix. A 5 mL glass serum vial fitted with a pressurizable 2-leg rubber septum (DWK Life Sciences, New Jersey, USA) was filled entirely with low percentage (0.3%) agar Marine Medium 2216 supplemented with 100 mM HEPES buffer (pH 7.5) and 100 mM NaNO3. Vials were utilized instead of the previously employed transfer pipette bulbs because vials allow for significantly improved visualization of the expanding “cloud” of motile cells. Experiments using the well-studied piezophile P. profundum SS9 and strain 10BA (this study) found no statistically significant difference between growth in pressurizable polyethylene pipette bulbs and glass serum vials fitted with a pressurizable rubber septum (Supplementary Figure S1). Thicker walled glass vials were used at 4°C than at 30°C to provide greater resistance to cracking under the combined conditions of high pressure and low temperature (DWK Life Sciences, New Jersey, USA; at 30°C: Catalog No. 223685; at 4°C: Catalog No. 223738).
For these experiments, stationary phase cell cultures were inoculated in a straight line through the center of the agar using a needle and syringe. Triplicate vials were placed into pressure vessels and incubated at one of two temperatures (4°C and 30°C) and one of four pressures (0.1, 10, 25, and 50 MPa), as previously described (Yayanos, 2001). Periodically, the vials were briefly removed from the pressure vessels, and microbial motility was assessed by measuring the visible growth away from the central inoculation site. In general, vials were decompressed and recompressed approximately ten times during the experiment; time outside the pressure vessels was limited to the extent possible, typically less than 10 minutes. While decompression and recompression were unavoidable, it should be noted that there is some evidence suggesting this may negatively impact microbial growth dynamics (Cario et al., 2022). The widest part of the cell plume was measured when determining the distance traveled. These measurements did not include tufts of increased motility as they did not represent the overall population behavior (see Results).
From this data (see Supplementary Figure S3), we calculated an expansion speed for each temperature/pressure condition tested (Figure 2). The expansion speed is the rate at which the cell front moved outward from the central inoculation line and was calculated using the equation:
Where se = expansion speed, t0 = time at the onset of the experiment, t = time at the end of the experiment (i.e., when the edge of the vial was reached or when the experiment ended if the edge was never reached), D0 = distance traveled at time t0, and Dt = distance traveled at time t. These expansion speeds were plotted as a function of pressure and fitted with a linear regression line (Figure 2, dashed lines).
2.4.2 Short-term (growth independent) effects
The second motility evaluation method utilized a high-pressure chamber for optical microscopy, as described previously (Nishiyama and Sowa, 2012). The high-pressure chamber is made of nickel alloy (Hastelloy C276) and is equipped with two optical windows (BK7). It is optimized to obtain high-quality images while maintaining a stable hydrostatic pressure up to 150 MPa. The chamber was connected to a hand pump (HP-150, Syn-Corporation, Japan) via a separator, which was used to reduce the total dead volume of buffer solution in the pressure line. The internal temperature of the chamber was controlled by running temperature-regulated water from a thermostat bath through tubing within the chamber. The high-pressure chamber was attached to an inverted microscope (Eclipse Ti, Nikon, Japan), and microscopic observation was performed using a long-working distance objective lens (CFI ELWD ADM40XC, Nikon, Japan). Phase-contrast videos were acquired by a charge-coupled device camera (WAT-120N+, Watec, Japan).
These experiments were performed at 23°C and 7°C, the closest temperatures the instrument could achieve to the strains’ optimum and in situ temperatures (30°C and 4°C, respectively). The instrument could not reach temperatures above room temperature and exhibited diminished video quality below 7°C. For these experiments, the study strains were grown to mid-log phase in Marine Broth 2216 at the experimental temperature. Cultures were diluted in fresh medium (1:10 or 1:20, depending on cell density) and then gently pipetted into and enclosed within the chamber. A standard operating procedure developed for this microscopy system was employed (Nishiyama and Kojima, 2012; Nishiyama and Sowa, 2012; Nishiyama et al., 2013). Briefly, the hydrostatic pressure within the chamber was increased to ~100 MPa in increments of 20 MPa and then decreased in a similar stepwise fashion. We halted pressure increases when motility nearly ceased; thus, the maximum pressure varied across strains and temperature conditions. At each pressure, phase-contrast videos of the cells were recorded at 30 frames s-1 for approximately 20 seconds. The pressure was increased at a rate of ~10 MPa per second; the pressure was regulated with an accuracy of ±1 MPa; the experimental temperature was controlled with a precision of ±1°C. The total elapsed time for pressure treatment of a population of cells was approximately 4 minutes. After pressure was released, cells were removed from the chamber, and the assay was repeated with a fresh culture. All strains were examined in triplicate using biological replicates.
Microscopy data were analyzed using previously described methods (Nishiyama and Arai, 2017). Briefly, the percentage of motile cells was determined by selecting a 1-second portion of the video file and manually counting the motile and non-motile cells in the plan of focus. A total of 10 frames per video were analyzed, giving an n = 30 across all three biological replicates for each pressure. For swimming speed, the SimplePTA plug-in (Nishiyama and Arai, 2017) for Fiji (Schindelin et al., 2012) was used to track cells and determine the cell’s average velocity. A total of ten cells per video were tracked, giving an n = 30 across all three biological replicates for each pressure. Relatively large standard deviations are common with this type of assay, as has been previously observed (Nishiyama and Sowa, 2012). To determine if increased pressure led to a statistically significant change in the percentage of motile cells or swimming speed, a paired two-tailed t-test was performed between atmospheric pressure (0.1 MPa) and each incremental pressure step.
2.5 Recovery of swimming motility after pressure exposure
The recovery of swimming motility after pressure treatment was also assessed using the high-pressure microscope chamber. As described above, microbial cultures were grown to mid-log phase in Marine Broth 2216, diluted, and inserted into the chamber. After recording a 20-second video to document the motility of the strain before high hydrostatic pressure exposure, the pressure was increased to 80, 120, and 100 MPa for strains 10BA, 18, and 36, respectively. These were the pressures at which the motility of each strain was significantly impacted but not halted during the short-term (growth-independent) high-pressure microscope experiments. After approximately 3 minutes of exposure to these sustained high pressures, the video camera was turned on and the pressure was rapidly decreased to 0.1 MPa, after which recording continued for approximately 3 minutes. These experiments were all performed at 23°C; pressure was increased and decreased at a rate of approximately 10 MPa per second. These experiments are designed to examine if pressure causes perturbation of the motility system or if the effects of high pressure are immediately reversible.
Using the data analysis methods described above, the percentage of motile cells and the swimming speed were determined before, during, and after exposure to high hydrostatic pressure. To determine if increased pressure led to a statistically significant decrease in either percentage of motile cells or swimming speed, a paired one-tailed t-test was performed between pre-exposure and each timepoint post-exposure.
3 Results
3.1 Strain identification and characterization
Strains 10BA, 18, and 36 were isolated from the Gulf of Mexico at water depths of 1509, 1509, and 46 mbsl, respectively (Table 1). Corresponding in situ water temperatures were ~23-30°C at 46 mbsl (Camilli et al., 2010) and ~4.7°C at 1509 mbsl (Hazen et al., 2010). Comparison of each study strain’s 16S rRNA gene sequence with sequences present in GenBank indicates that strain 10BA GOM-1509m belongs to the genus Halomonas, strain 18 GOM-1509m to the genus Alcanivorax, and strain 36 GOM-46m to the genus Shewanella. Based on Maximum-Likelihood phylogenetic analysis (Figure 1), the closest phylogenetic relative of strain 10BA is H. profundus AT1214 (NR_114956), the closest relative of strain 18 is A. xenomutans JC109 (NR_13358), and the closest relative of strain 36 is S. indica KJW27 (NR_108899).
All three study strains grow optimally in Marine Broth 2216 at 30°C (data not shown). NanoOrange flagellar straining (Grossart et al., 2000) revealed that Halomonas sp. strain 10BA is peritrichously flagellated, consistent with what is known about motile members of the genus Halomonas (Franzmann et al., 1988). Motile members of the genus Alcanivorax employ a variety of flagellation types (e.g., peritrichous, single polar) (Fernandez-Martinez et al., 2003; Yang et al., 2018), and our analysis revealed that Alcanivorax sp. strain 18 has a single polar flagellum. Similarly, Shewanella sp. strain 36 has a single polar flagellum, which is consistent with most members of this genus (Janda and Abbott, 2014), although other flagellation types have been observed in some Shewanella species (Wang et al., 2008; Bubendorfer et al., 2012).
3.2 Pressure & temperature effects on growth
Halomonas sp. strain 10BA exhibited its maximum growth rate and yield when grown at 30°C and 10 MPa (Figure 2A; Supplementary Figure S2A, Supplementary Table S1), making it a piezomesophile, albeit a modest one (Scoma, 2021). While only slightly diminished growth was observed at 0.1 and 25 MPa, strain 10BA did not grow at 30°C and 50 MPa. At this optimum temperature, its upper-pressure limit is comparable to that of E. coli (Zobell and Johnson, 1949; Zobell and Cobet, 1962). When grown at 4°C, the pressure optimum of strain 10BA was reduced to 0.1 MPa (Figure 2A; Supplementary Figure S2B, Supplementary Table S1), and growth rate and yield decreased as pressure increased; no growth was observed at 4°C and 50 MPa. The growth rate P50 for strain 10BA was 35 MPa at 30°C and 24 MPa at 4°C (Supplementary Table S2).
Alcanivorax sp. strain 18 displayed slightly more pressure-sensitive mesophilic growth properties than Halomonas sp. strain 10BA. Although at 30°C its growth rate was higher at 10 MPa than 0.1 MPa, this was not statistically significant (p-value = 0.2) and its growth yield was maximum at 0.1 MPa (Figure 2B; Supplementary Figure S2C, Supplementary Table S1). At 25 MPa its growth yield decreased a modest 10% but its growth rate decreased by over 50%. Growth was barely detectable at 50 MPa for this strain. Strain 18 did not grow at 4°C under the conditions tested. The P50 for strain 18 was 30 MPa at 30°C (Supplementary Table S2).
Shewanella sp. strain 36 exhibited comparable growth at 0.1 MPa and 10 MPa at 30°C, reaching its maximum growth rate at 0.1 MPa and its maximum growth yield at 10 MPa (Figure 2C; Supplementary Figure S2E, Supplementary Table S1). An increase in pressure led to a gradual decrease in growth, but unlike the other strains in this study, strain 36 exhibited growth at 30°C and 50 MPa. It is curious that, at 30°C, strain 36 is the most pressure tolerant of the study strains despite having been isolated from the sea surface, in contrast to the other strains isolated from depth. However, this piezotolerance was not accompanied by concomitant adaptation to low temperatures. When grown at 4°C, the maximum growth rate and yield were at atmospheric pressure (Figure 2C; Supplementary Figure S2F; Supplementary Table S1). At this lower temperature, just 10 MPa of pressure led to an approximately 50% decrease in growth yield. It should be noted that, although there was obvious – albeit weak – growth at 10 MPa and 4°C (Supplementary Figure S2F), the logistic model used to calculate the growth rate reported an error. Thus, the reported growth rate for this P-T condition is zero. No growth was observed at 25 or 50 MPa at 4°C. The P50 for strain 36 was 39 MPa at 30°C and just 3 MPa at 4°C (Supplementary Table S2). However, the P50 value for this lower temperature is likely an underestimation due to limitations of the logistic model used to calculate the growth rate.
The P-T growth properties of all three strains are most consistent with a principally shallow-water existence, despite the source of the Halomonas and Alcanivorax strains being bathypelagic water.
3.3 Pressure & temperature effects on motility
We next assessed P-T effects on 1) growth and motility through a low percentage agar mix, as well as 2) motility in a liquid environment in which individual cell responses were recorded using a temperature-controlled high-pressure microscope system.
3.3.1 Long-term (growth-dependent) effects
Halomonas sp. strain 10BA was motile at 0.1, 10, and 25 MPa at 30°C, showing marginally increased expansion speeds at 10 MPa compared to 0.1 MPa (Figure 2A; Supplementary Figure S3A). At 4°C, strain 10BA was only motile at 0.1 and 10 MPa (Figure 2A; Supplementary Figure S3B), despite exhibiting growth at pressures up to 25 MPa at this temperature. The P50 for strain 10BA’s long-term motility was 23 MPa at 30°C and 11 MPa at 4°C (Supplementary Table S2), suggesting that the long-term motility of this strain is more pressure sensitive than its growth.
Despite exhibiting growth at 50 MPa, Alcanivorax sp. strain 18 was only motile at pressures up to 25 MPa at 30°C (Figure 2B; Supplementary Figure S3C). Interestingly, the P50 for strain 18’s long-term motility (27 MPa) is approximately the same as its P50 for growth (26 MPa) (Supplementary Table S2). Since strain 18 did not grow at 4°C, no motility vial experiments were performed at this temperature.
At 30°C, Shewanella sp. strain 36 was only motile at pressures up to 25 MPa (Figure 2C; Supplementary Figure S3E), despite exhibiting growth at all pressures tested (Figure 2C; Supplementary Figure S2E). It should be noted that, for a single replicate at 30°C, a small tuft of motile cells derived from the inoculation site was observed at the 96-hour time point (Supplementary Figure S6), possibly the result of a random mutation (Foster, 2007). At 4°C, strain 36 was only motile at 0.1 MPa, despite exhibiting growth at 0.1 and 10 MPa. The P50 for strain 36’s long-term motility was 21 MPa at 30°C and 6 MPa at 4°C (Supplementary Table S2), again suggesting that the long-term motility of this strain is more pressure sensitive than its growth.
We conclude from these growth-based motility experiments that low temperature dramatically reduces motility in the two strains examined at 4°C. Additionally, we conclude that pressure impacts were variable among the strains up to 10 MPa (corresponding to a depth of 1,000 m) but were highly inhibitory at pressures of 25 and especially 50 MPa (corresponding to depths of 2,500 m and 5,000 m, respectively). Much of the impact is due to growth inhibition; however, the magnitude of the expansion speed reductions is greater than that of the growth reductions that resulted from increasing pressure. Pressure impacts on motility were especially severe at 4°C.
3.3.2 Short-term (growth-independent) effects
A representative compilation of the videos acquired during the high-pressure microscopy experiment can be found in Supplementary Materials (Supplementary Video S1). This example shows a single replicate of Shewanella sp. strain 36 as it was pressurized from 0.1 to 120 MPa.
Halomonas sp. strain 10BA was pressurized to a maximum pressure of 80 MPa at 23°C and 60 MPa at 7°C (Figures 3A, B). At both experimental temperatures, similar trends were observed. The percentage of motile cells remained reasonably steady up to 60 MPa at 23°C and 40 MPa at 7°C, then decreased at pressures above these thresholds (Figure 3A). Conversely, the swimming speed decreased relatively linearly throughout the pressurization process at both temperatures (Figure 3B). The P50 for the percentage of motile cells was 64 MPa at 23°C and 57 MPa at 7°C; the P50 for swimming speed was 66 MPa at 23°C and 63 MPa at 7°C (Supplementary Table S3). Upon depressurization to 0.1 MPa at both experimental temperatures, the swimming motility of strain 10BA recovered to approximately the same level observed before pressurization, except for the percentage of motile cells at 23°C, which was significantly higher after depressurization (mean ± standard deviation; 80 ± 7%) than at the onset of the experiment (62 ± 9%) (p-value<< 0.005) (Figure 3A).
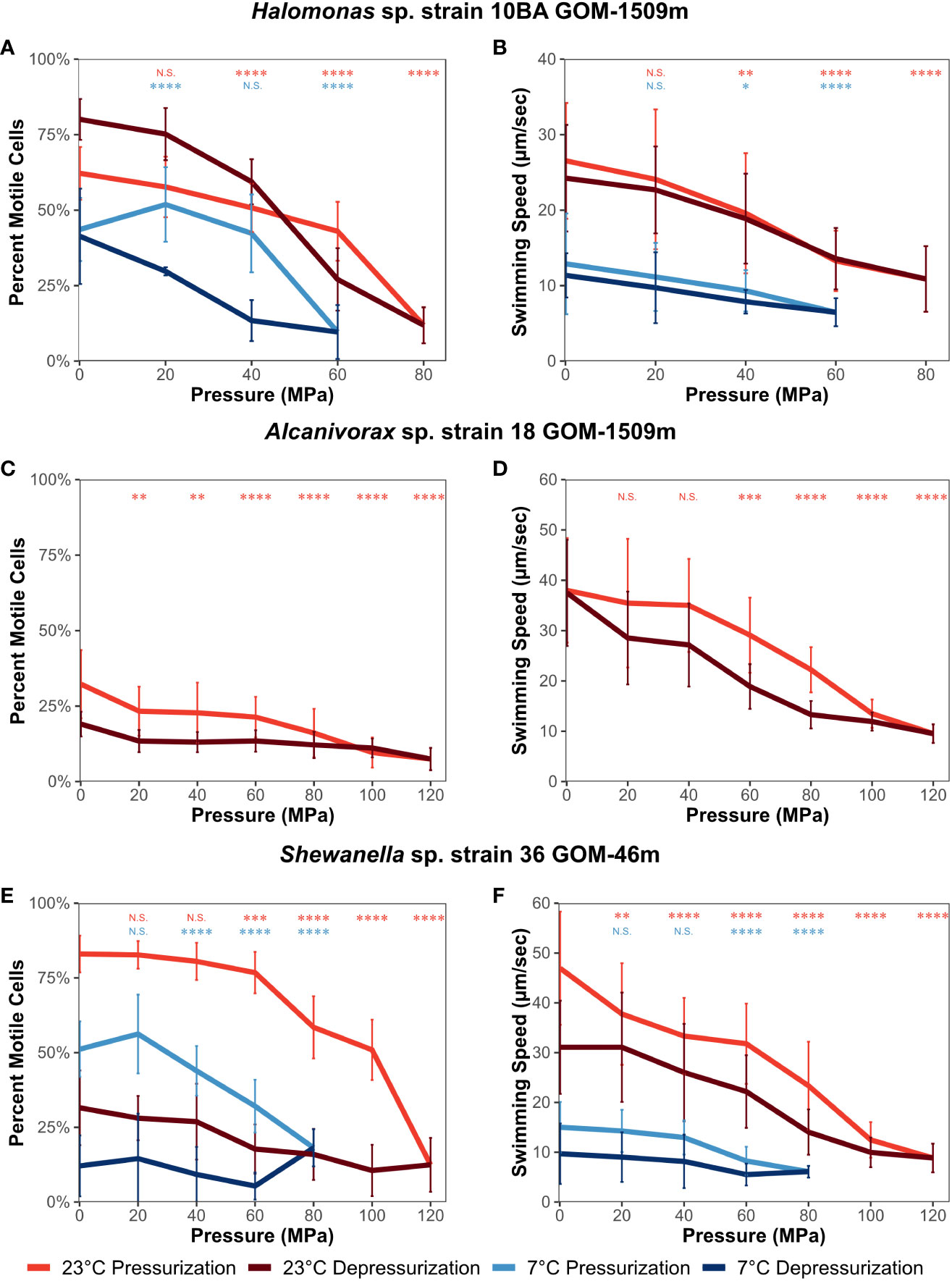
Figure 3 Percentage of motile cells and swimming speed (µm/second) of Halomonas sp. strain 10BA GOM-1509m (A, B), Alcanivorax sp. strain 18 GOM-1509m (C, D), and Shewanella sp. strain 36 GOM-46m (E, F). Assessed as a function of pressure and temperature (23°C and 7°C) using a high-pressure microscope chamber. Error bars represent standard deviation (n = 30). A paired two-tailed t-test was performed to determine if values differed significantly from those at atmospheric pressure (0.1 MPa). N.S., not significant; *, p ≤ 0.05; **, p ≤ 0.01; ***, p ≤ 0.001; ****, p ≤ 0.0001.
Alcanivorax sp. strain 18 was pressurized to a maximum pressure of 120 MPa at 23°C (Figures 3C, D). Since strain 18 did not grow at 7°C, no high-pressure microscopy experiments were performed at this temperature. Strain 18 had the smallest percentage of motile cells among the strains examined. For example, at the onset of the experiment, the percentage of motile cells was 32 ± 11%, significantly lower than that of strain 10BA (62 ± 9%) (Figure 3C). While strain 18’s percentage of motile cells decreased steadily as pressure increased (Figure 3C, p-value ≤ 0.01), its swimming speed remained stable up to 40 MPa, above which it gradually decreased (Figure 3D, p-value ≤ 0.001). The P50 for strain 18 was 76 MPa for the percentage of motile cells and 88 MPa for swimming speed (Supplementary Table S3). Upon depressurization, the swimming speed fully recovered to the level observed at the onset of the experiment (Figure 3D), while the percentage of motile cells only recovered to approximately 60% of the starting value (p-value<< 0.005) (Figure 3C).
Shewanella sp. strain 36 was pressurized to a maximum of 120 MPa at 23°C and 80 MPa at 7°C (Figures 3E, F). At 23°C, the percentage of motile cells remained steady up to ~60 MPa before significantly decreasing, and swimming speed decreased gradually as pressure increased. Alternatively, at 7°C, motility remained relatively constant up to 20 MPa (percentage of motile cells) or 40 MPa (swimming speed), above which it gradually decreased (Figures 3E, F). The slight increase in the percentage of motile cells observed at 20 MPa is not statistically significant (p-value = 0.23). It should also be noted that at the onset of the experiment, the temperature decrease significantly impacted the swimming speed of strain 36 (47 ± 11 µm s-1 at 23°C, 15 ± 5 µm s-1 at 7°C). The P50 for the percentage of motile cells was 102 MPa at 23°C and 73 at 7°C; the P50 for swimming speed was 74 MPa at 23°C and 72 at 7°C (Supplementary Table S3). Interestingly, the motility of strain 36 responded differently to depressurization than the other study strains. While the percentage of motile cells only recovered to 38% and 24% of that observed before pressurization at 23°C and 7°C, respectively, the swimming speed recovered to 66% and 65% of that observed before pressurization at 23°C and 7°C, respectively. Although the error bars overlap, these represent statistically significant decreases in swimming speed (p-value ≤ 0.0001 for 23°C and ≤ 0.01 for 7°C) (Figure 3F).
To rule out cell death caused by exposure to high hydrostatic pressure as an explanation for the reduction in motility of the strains, we evaluated the colony-forming units (CFU) before and after pressurization (see Supplementary Methods). No significant change in CFU was observed for any of the strains at either 23°C or 7°C (Supplementary Figure S7).
We conclude from these microscopy-based motility analyses that cells exposed to high pressure over a period of minutes are far more pressure-resistant than cells incubated at high pressure over periods of days. The ability to recover motility (both the percentage of motile cells and swimming speed) following high-pressure exposure varied among the strains, with Shewanella sp. strain 36 being particularly sensitive to these impacts. And as with the long-term growth-based motility measurements, the effects of increased pressure and decreased temperature were additive, although the magnitude of pressure’s impact on motility is often less at lower temperatures than at higher temperatures.
3.4 Recovery of swimming motility after pressure exposure
The significant loss of swimming motility (both the percentage of motile cells and swimming speed) observed after depressurization across two of the three study strains prompted us to examine motility recovery following a 3-minute high-pressure exposure at 23°C (Figure 4). Each strain was exposed to the maximum pressure at which it maintained motility during the short-term pressure exposure experiment – 80, 120, and 100 MPa for strains 10BA, 18, and 36, respectively.
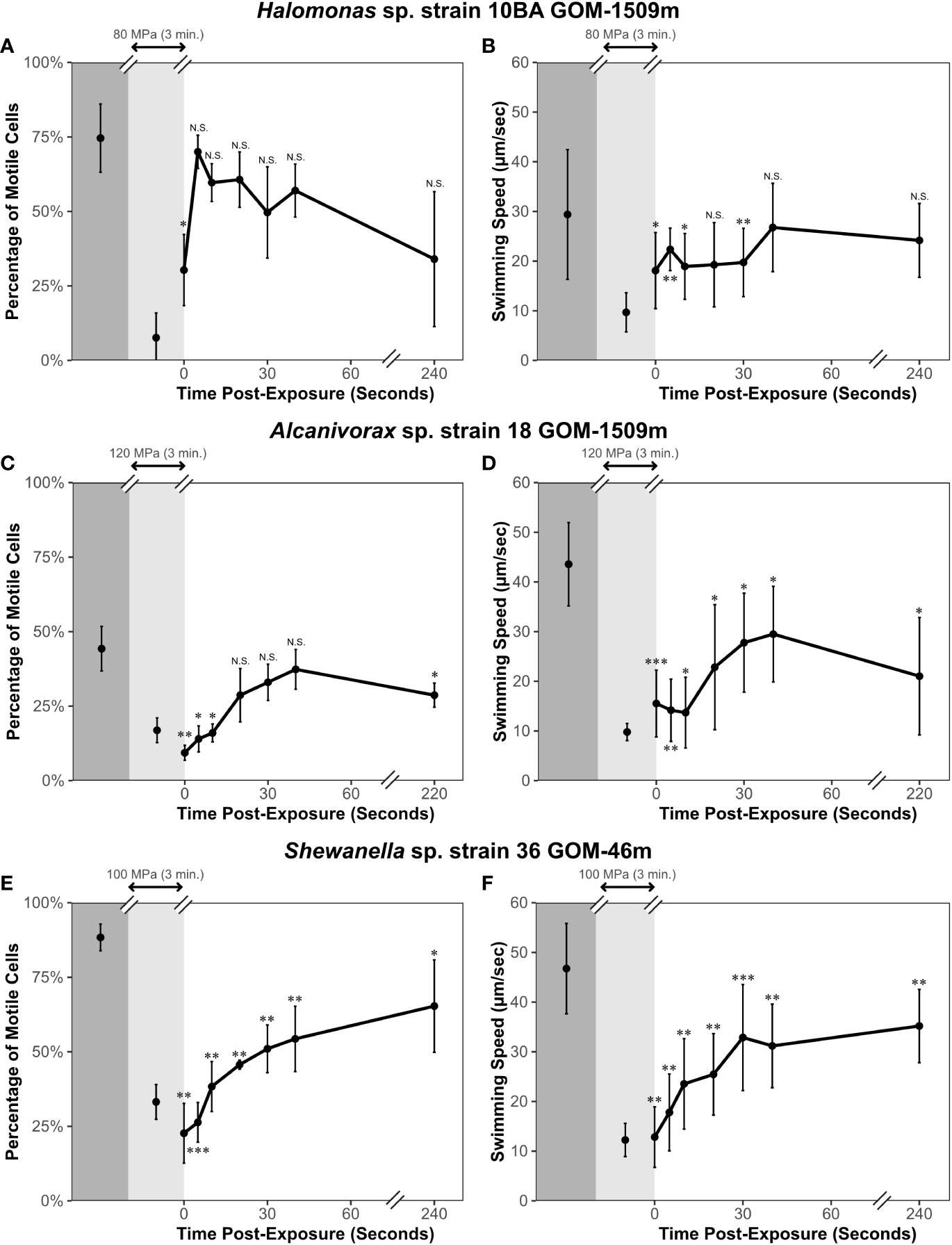
Figure 4 Percentage of motile cells and swimming speed of study strains before (dark gray shading), during (light gray shading), and immediately after (white shading) exposure to pressure at 23°C. Halomonas sp. strain 10BA GOm-1509m (A, B) was pressurized to 80 MPa; no data for replicate 3 at 240 seconds post-exposure, so n = 20 for this data point. Alcanivorax sp. strain 18 GOM-1509m (C, D) was pressurized to 120 MPa. Shewanella sp. strain 36 GOM-46m (E, F) was pressurized to 100 MPa. Error bars represent standard deviation (n = 30 unless otherwise stated). A paired one-tailed t-test was performed to determine if values differed significantly from those pre-exposure. N.S., not significant; *p ≤ 0.05; **p ≤ 0.01; ***p ≤ 0.001.
Halomonas sp. strain 10BA’s motility recovered rapidly after depressurization. Within 5 seconds after depressurization, the percentage of motile cells was not significantly different from that observed before pressurization (Figure 4A). Similarly, the swimming speed recovered to levels statistically equivalent to those observed pre-exposure within 40 seconds after depressurization (Figure 4B).
For Alcanivorax sp. strain 18, the percentage of motile cells recovered rapidly (within 40 seconds) after depressurization, while the swimming speed only recovered to ~60% of that observed before pressurization (Figures 4C, D). Nearly 4 minutes post-exposure, the swimming speed was still significantly different from pre-exposure levels (Figure 4D). These results contrast the short-term motility experiment results (Figure 3). The observed difference in the percentage of motile cells between the short-term motility experiments (Figure 3) and these motility recovery experiments (Figure 4) could be due to hysteresis. During the short-term motility experiments, the cells were tracked for less than 30 seconds after decompression, which may not have been enough time for the cells to regain swimming activity after pressure release. This phenomenon has been observed in other studies assessing the impacts of short-term pressure exposure on motility (Nishiyama and Sowa, 2012). Alternatively, the swimming speed results suggest that more prolonged exposure to high hydrostatic pressure damages some aspect(s) of this strain’s energetics or mechanisms of flagellar function.
Finally, for Shewanella sp. strain 36, both the percentage of motile cells and swimming speed significantly differed from pre-exposure levels, only recovering by approximately 75% at 4 minutes post-depressurization (Figures 4E, F).
We conclude from these motility recovery experiments that the motility or flagella of various microbes – and perhaps even distinct cells within the same culture – can be differentially impacted by pressure. Each strain responded differently after pressure was released. Halomonas sp. strain 10BA was the only strain whose motility recovered fully in both the short-term motility experiments (Figure 3) and these motility recovery experiments (Figure 4).
4 Discussion
Microbial motility plays an important role within the ocean (Grossart et al., 2001; Fenchel, 2002; Stocker et al., 2008; Stocker and Seymour, 2012; Keegstra et al., 2022). However, even though over 88% of the ocean volume is considered to be within the deep sea (Knauss and Garfield, 2017), very little is known about the influence of deep-sea environmental conditions (i.e., high hydrostatic pressure and low temperature) on marine microbial motility. This is even more so the case when two or more environmental factors are examined in concert.
In this study, we examined the swimming motility of three strains belonging to cosmopolitan marine bacterial genera obtained from the Gulf of Mexico shortly after the onset of the DWH oil spill. Although two of the three strains were isolated from bathypelagic depths, the strains’ temperature and pressure growth preferences indicate that strains 18 and 36 are best described as non-piezophilic mesophiles, while strain 10BA is a modestly piezophilic mesophile. In all likelihood, they have evolved for optimal activity, growth, and reproduction in shallow waters, and the two strains obtained from deep-sea depths were delivered there from above. It has been shown that, under certain hydrological conditions, surface-derived marine microbes are delivered to the deep ocean through perturbations such as deep mixing and large influxes of sinking particles (Bianchi and Garcin, 1994; Tamburini et al., 2013; Garel et al., 2021; Amano et al., 2022). Despite this, very little attention has been paid to the effects of pressure and temperature on the motility of piezosensitive, mesophilic marine microbes, a process that, together with chemotaxis, is a critical aspect of nutrient acquisition (Raina et al., 2023). Thus, the characterization of the motility behavior of shallow water-adapted microbes under physical conditions approximating those present in situ is highly relevant to considerations of factors modulating deep-sea microbial activity.
The motility of no other mesophilic marine microbe has been examined as a function of both pressure and temperature or in terms of the percentage of motile cells or recovery from high-pressure exposure. However, the swimming speed of one non-piezophile has been investigated at elevated pressure via high-pressure microscopy (Eloe et al., 2008). This is P. profundum strain 3TCK, a member of a species that includes piezophilic and psychrophilic members, but which was isolated from shallow-water surficial sediments (Campanaro et al., 2005). Curiously, as with the marine microbes studied here, it could maintain some swimming speed (~ 5 µm s-1) even at hadal pressures. Furthermore, its short-term motile pressure range was far greater than that of the model enteric microbe E. coli but less than that of its piezophilic relative.
Our motility assays included measurements of long-term growth-dependent motility through a low percentage agar matrix and short-term single-cell-based recordings of the percentage of motile cells and their swimming speed within a pressurizable viewing chamber connected to a microscope. When comparing these two methods, significant differences were observed in the P-T effects on motility. When incubated at elevated pressure over days to weeks, the P50 of motility in semi-solid agar was only ~35 and 13.5 MPa at 30 and 4°C, respectively (Supplementary Table S2). Conversely, when exposed to pressure for only seconds to minutes in the microscopy assays, the 50% decrease in the percentage of motile cells and swimming speed respectively required pressures of at least 80 and 76 MPa at 23°C, and 65 and 67 MPa at 7°C (Supplementary Table S3). These stark differences inherently arise from the nature of the two methods. While the short-term, growth-independent motility assay uncovers the immediate impacts of pressure on the function of preexisting flagella in vivo, the long-term, growth-dependent assay also requires cells to reproduce and form new flagella. Flagellar function alone is energetically costly (Mitchell, 2002; Keegstra et al., 2022), and flagellar formation is an additional energetic expense (Terashima et al., 2008) that involves the biosynthesis of six different components (Macnab, 2003) involving at least 24 core genes, with some bacteria requiring over 50 genes (Macnab, 2004; Liu and Ochman, 2007). It should also be noted that some of this difference could be attributed to the unavoidable differences in cultivation methods between the two motility experiments.
The long-term motility assays indicated that motility was more pressure and temperature sensitive than growth for all strains. This is consistent with prior work suggesting that the motility of non-piezophilic mesophiles is one of the most pressure-sensitive (Meganathan and Marquis, 1973; Bartlett, 1992; Bartlett, 2002; Eloe et al., 2008) and temperature-sensitive (Adler and Templeton, 1967; Maeda et al., 1976; Nishiyama et al., 2013) microbial processes. We found that, at 30°C, the P50 values for growth rate are significantly higher than the P50 values for growth-dependent expansion speed (p-value ≤ 0.05) (Supplementary Table S2). At 4°C, Halomonas sp. strain 10BA’s P50 value for growth rate is more than double its P50 value for expansion speed, again suggesting that motility is more sensitive to pressure than growth rate. While Shewanella sp. strain 36 shows the opposite trend at 4°C, as noted in the Results, the P50 value for this strain’s growth rate at 4°C is likely an underestimation due to limitations of the method used to estimate the growth rate.
Additionally, we found that while decreased temperature alone had a significantly more significant impact on motility than the pressures employed, the combination of low temperature and high hydrostatic pressure exerted compounding deleterious effects on motility. In both motility experiments, expansion and swimming speed at atmospheric pressure were at least 50% lower at 4-7°C compared to 23-30°C. At higher temperatures, a decrease of this magnitude required pressures of at least 24 MPa and 66 MPa for the long-term and short-term motility experiments, respectively. At lower temperatures, motility was more sensitive to pressure. For example, in the long-term growth-dependent motility experiments, while Halomonas sp. strain 10BA and Shewanella sp. strain 36 are motile up to 25 MPa at 30°C, they are only motile up to 10 MPa and 0.1 MPa, respectively, at 4°C (Supplementary Figure S3). However, we found that at lower temperatures, the magnitude of the perturbation that pressure exerts on motility is less than that observed at higher temperatures. This can be seen in the short-term motility experiments with Shewanella sp. strain 36, where the swimming speed goes from 47 µm s-1 at 0.1 MPa to 23 µm s-1 at 80 MPa at 23°C but only from 15 to 6 µm s-1 over the same pressure span at 7°C. This shows that while the combined effect of low temperature and high pressure is especially bad, the magnitude at which pressure impacts motility decreases at lower temperatures, perhaps because the swimming ability is already so severely compromised. These findings also highlight the importance of considering both in situ temperature and pressure when studying microbial processes in the deep ocean, including various global biogeochemical cycles (Azam, 1998; Strom, 2008).
Although beyond the scope of this study, there are many possible explanations for the additive, deleterious impacts of low temperature and high pressure on motility. First, both high pressure and low temperature are well-known to impact protein synthesis and protein-protein quaternary interactions (Pollard and Weller, 1966; Yayanos and Pollard, 1969; Broeze et al., 1978; Jones et al., 1987; Pavlovic et al., 2005; Gayan et al., 2017; Roche and Royer, 2018), which could impact flagellar synthesis and assembly. The assembly of flagellar and motility proteins into a large and complex flagellar organelle structure is estimated to be much more pressure-sensitive than protein synthesis (Meganathan and Marquis, 1973). Second, decreased ion flux through the rotor/stator motor components could impact torque generation and thus hook and filament rotation, as pressure has been shown to affect similar processes in other bacteria (Matsumura and Marquis, 1977; Marquis and Bender, 1987; Wouters et al., 1998; Pavlovic et al., 2005). Similarly, temperature has been shown to impact the rotational speed of flagellar motors, possibly due to impacts on ion translocation (Yuan and Berg, 2010; Baker et al., 2011).
An additional possible target of P-T effects on microbial cells which could influence motility is the lipid membrane as the flagellar basal body – which plays a crucial role in both the formation of new flagella and the function of previously formed flagella – spans across all three layers of bacterial cell membranes (cytoplasmic membrane, periplasmic space, outer membrane) (Macnab, 2003). Previous studies have shown that high pressure and low temperature have compounding negative effects on lipid membranes (summarized in Bartlett, 2002), with changes in the unsaturated fatty acid composition of membranes being one example of a lipid change that is essential for bacterial adaptation in the deep sea (Allen et al., 1999; Bartlett et al., 2008). It has been estimated that for the phase state of lipid membranes, a change in pressure of 100 MPa is equivalent to a change in temperature of 20°C (Bartlett, 2002). We estimate that for the growth-based motility assays examined in this study, a change in pressure of only 40 MPa was equivalent to a change in temperature of 20°C (see Supplementary Methods). In contrast, the short-term microscopy assays had values much closer to that observed for lipids.
In addition to swimming motility, chemotaxis is a similarly important aspect of microbial life in the ocean (Barbara and Mitchell, 2003; Stocker et al., 2008; Stocker and Seymour, 2012; Smriga et al., 2016; Keegstra et al., 2022). Hydrocarbon chemotaxis, in particular, likely plays an important role in the degradation and bioremediation of both naturogenic and anthropogenic hydrocarbons in the ocean (Mason et al., 2012; Lacal et al., 2013; Joye et al., 2018; Lacal, 2018; Zhou et al., 2022). In this study, we observed anecdotal evidence of pressure impacts on chemotaxis. Alcanivorax sp. strain 18 appeared to exhibit a “run-reverse-flick” swimming pattern, which has been found in other monotrichously flagellated bacteria as a form of chemotaxis (Xie et al., 2011; Son et al., 2013; Bubendorfer et al., 2014; Ortega et al., 2020; Grognot et al., 2021). In one of the replicates, an apparent decrease in the frequency of this “run-reverse-flick” swimming pattern was observed at 23°C when pressure increased from 0.1 to 20 MPa (Supplementary Figure S8, Supplementary Video S2), which could be indicative of a decrease in the cell’s ability to randomize orientation and hence engage in the biased random walk that is at the heart of chemotaxis. One prior study (Nishiyama et al., 2013) showed that, at 20°C, extremely high hydrostatic pressure (≥120 MPa) was required to induce a counterclockwise to clockwise reversal of the flagellar motor in an E. coli mutant deficient in chemotaxis signaling (ΔcheY), while at 9°C, pressures ≤60 MPa were required to induce reversal. While an intriguing finding, that study by Nishiyama et al. (2013) is the field’s only insight into P-T impacts on chemotaxis. Thus, future work with these strains will focus on elucidating the effect of low temperature and high hydrostatic pressure on microbial hydrocarbon chemotaxis.
While the impact of pressure on shallow water-adapted marine microbes varies, as exemplified by our study, we generally observed that the major effects of pressure began at ~40 MPa, equivalent to 4,000 mbsl. Given that approximately 55% of the ocean volume experiences pressures of 40 MPa or greater (Knauss and Garfield, 2017), P-T effects on both motility and chemotaxis, as a function of the rich diversity of prokaryotes present within the ocean, could have profound consequences on the rates of microbially-mediated chemical transformations at depth.
Data availability statement
The datasets presented in this study can be found in online repositories. The names of the repository/repositories and accession number(s) can be found below: https://www.ncbi.nlm.nih.gov/, MZ424455. https://www.ncbi.nlm.nih.gov/, MZ424456. https://www.ncbi.nlm.nih.gov/, MZ424457. The raw datasets for all other experiments from this study can be found in the Gulf of Mexico Research Initiative (GRIIDC) database (https://data.gulfresearchinitiative.org) under unique dataset identifiers (UDI) R5.x285.000:0006 and R5.x285.000:0016.
Author contributions
KM and MN performed experimental work. KM, MN, TK, and DB performed data analysis. KM and DB wrote the manuscript. All authors contributed to the article and approved the submitted version.
Funding
This work was supported by the Gulf of Mexico Research Initiative for the RFP V Investigator Grant entitled “Role of Microbial Motility for Degradation of Dispersed Oil” (GoMRI 2015-V-328) (GoMRI R-16-0037) awarded to DB, and by JSPS KAKENHI (JP15H01319 and JP22H05697 awarded to MN, JP17H04598 awarded to TK).
Acknowledgments
We thank Dr. Romy Chakraborty, Dr. Gary Andersen, and Dr. Stephen Techtmann for generously providing the microbial strains used in this study. We also thank Dr. Jun Kawamoto and Dr. Takuya Ogawa for arranging the high-pressure microscopy study. Finally, we thank Dr. Alvaro Muñoz-Plominsky, Luke Fisher, and Miguel Desmarais for reading, commenting on, and enhancing drafts of this manuscript.
Conflict of interest
The authors declare that the research was conducted in the absence of any commercial or financial relationships that could be construed as a potential conflict of interest.
Publisher’s note
All claims expressed in this article are solely those of the authors and do not necessarily represent those of their affiliated organizations, or those of the publisher, the editors and the reviewers. Any product that may be evaluated in this article, or claim that may be made by its manufacturer, is not guaranteed or endorsed by the publisher.
Supplementary material
The Supplementary Material for this article can be found online at: https://www.frontiersin.org/articles/10.3389/fmars.2023.1181062/full#supplementary-material
References
Adler J., Templeton B. (1967). Effect of environmental conditions on motility of Escherichia coli. J. Gen. Microbiol. 46, 175–17+. doi: 10.1099/00221287-46-2-175
Allen E. E., Facciotti D., Bartlett D. H. (1999). Monounsaturated but not polyunsaturated fatty acids are required for growth of the deep-sea bacterium Photobacterium profundum SS9 at high pressure and low temperature. Appl. Environ. Microbiol. 65 (4), 1710–1720. doi: 10.1128/AEM.65.4.1710-1720.1999
Amano C., Zhao Z. H., Sintes E., Reinthaler T., Stefanschitz J., Kisadur M., et al. (2022). Limited carbon cycling due to high-pressure effects on the deep-sea microbiome. Nat. Geosci. 15 (12), 1041–104+. doi: 10.1038/s41561-022-01081-3
Andersen G., Dubinsky E. A., Chakraborty R., Hollibaugh J. T., Hazen T. C. (2012). Evolution of hydrocarbon-degrading microbial communities in the aftermath of the deepwater horizon oil well blowout in the gulf of Mexico (San Francisco, California: American Geophysical Union).
Arahal D. R., Ventosa A. (2006). “The Family Halomonadaceae,” in The Prokaryotes. Eds. Dworkin M., Falkow S., Rosenberg E., Schleifer K.-H., Stackebrandt E. (New York, NY: Springer Science+Business Media, LLC), 811–835.
Aristegui J., Gasol J. M., Duarte C. M., Herndl G. J. (2009). Microbial oceanography of the dark ocean's pelagic realm. Limnology Oceanography 54 (5), 1501–1529. doi: 10.4319/lo.2009.54.5.1501
Azam F. (1998). Microbial control of oceanic carbon flux: the plot thickens. Science 280 (5364), 694–696. doi: 10.1126/science.280.5364.694
Azam F., Malfatti F. (2007). Microbial structuring of marine ecosystems. Nat. Rev. Microbiol. 5 (10), 782–791. doi: 10.1038/nrmicro1747
Baker M., Inoue Y., Takeda K., Ishijima A., Berry R. (2011). Two methods of temperature control for single-molecule measurements. Eur. Biophysics J. Biophysics Lett. 40 (5), 651–660. doi: 10.1007/s00249-010-0667-y
Barbara G. M., Mitchell J. G. (2003). Bacterial tracking of motile algae. FEMS Microbiol. Ecol. 44 (1), 79–87. doi: 10.1111/j.1574-6941.2003.tb01092.x
Bartlett D. H. (2002). Pressure effects on in vivo microbial processes. Biochim. Et Biophys. Acta - Protein Structure Mol. Enzymology 1595 (1-2), 367–381. doi: 10.1016/S0167-4838(01)00357-0
Bartlett D. H., Ferguson G., Valle G. (2008). “Adaptations of the psychrotolerant piezophile photobacterium profundum strain SS9,” in High-Pressure Microbiology. American Society for Microbiology.
Bianchi A., Garcin J. (1994). Bacterial response to hydrostatic pressure in seawater samples collected in mixed-water and stratified-water conditions. Mar. Ecol. Prog. Ser. 111 (1-2), 137–141. doi: 10.3354/meps111137
Broeze R. J., Solomon C. J., Pope D. H. (1978). Effects of low temperature on In vivo and In vitro protein synthesis in Escherichia coli and Pseudomonas fluorescens. J. Bacteriology 134 (3), 861–874. doi: 10.1128/jb.134.3.861-874.1978
Bubendorfer S., Held S., Windel N., Paulick A., Klingl A., Thormann K. M. (2012). Specificity of motor components in the dual flagellar system of Shewanella putrefaciens CN-32. Mol. Microbiol. 83 (2), 335–350. doi: 10.1111/j.1365-2958.2011.07934.x
Bubendorfer S., Koltai M., Rossmann F., Sourjik V., Thormann K. M. (2014). Secondary bacterial flagellar system improves bacterial spreading by increasing the directional persistence of swimming. Proc. Natl. Acad. Sci. United States America 111 (31), 11485–11490. doi: 10.1073/pnas.1405820111
Camilli R., Reddy C. M., Yoerger D. R., Van Mooy B., Jakuba M. V., Kinsey J. C., et al. (2010). Tracking hydrocarbon plume transport and biodegradation at deepwater horizon. Science 330 (6001), 201–204. doi: 10.1126/science.1195223
Campanaro S., Treu L., Valle G. (2008). Protein evolution in deep sea bacteria: an analysis of amino acids substitution rates. BMC Evolutionary Biol. 8, 15. doi: 10.1186/1471-2148-8-313
Campanaro S., Vezzi A., Vitulo N., Lauro F. M., D'angelo M., Simonato F., et al. (2005). Laterally transferred elements and high pressure adaptation in Photobacterium profundum strains. BMC Genomics 6, 15. doi: 10.1186/1471-2164-6-122
Cario A., Grossi V., Schaeffer P., Oger P. M. (2015). Membrane homeoviscous adaptation in the piezo-hyperthermophilic archaeon Thermococcus barophilus. Front. Microbiol. 6, 12. doi: 10.3389/fmicb.2015.01152
Cario A., Oliver G. C., Rogers K. L. (2022). Characterizing the piezosphere: the effects of decompression on microbial growth dynamics. Front. Microbiol. 13, 12. doi: 10.3389/fmicb.2022.867340
Denich T. J., Beaudette L. A., Lee H., Trevors J. T. (2003). Effect of selected environmental and physico-chemical factors on bacterial cytoplasmic membranes. J. Microbiological Methods 52 (2), 149–182. doi: 10.1016/S0167-7012(02)00155-0
Dewangan N. K., Conrad J. C. (2018). Adhesion of marinobacter hydrocarbonoclasticus to surfactant-decorated dodecane droplets. Langmuir 34 (46), 14012–14021. doi: 10.1021/acs.langmuir.8b02071
Dewangan N. K., Conrad J. C. (2019). Rotating oil droplets driven by motile bacteria at interfaces. Soft Matter 15 (45), 9368–9375. doi: 10.1039/C9SM01570A
Dewangan N. K., Conrad J. C. (2020). Bacterial motility enhances adhesion to oil droplets. Soft Matter 16 (35), 8237–8244. doi: 10.1039/D0SM00944J
Dewangan N. K., Tran N., Wang-Reed J., Conrad J. C. (2021). Bacterial aggregation assisted by anionic surfactant and calcium ions. Soft Matter 17 (37), 8474–8482. doi: 10.1039/D1SM00479D
Eloe E. A., Lauro F. M., Vogel R. F., Bartlett D. H. (2008). The deep-sea bacterium Photobacterium profundum SS9 utilizes separate flagellar systems for swimming and swarming under high-pressure conditions. Appl. Environ. Microbiol. 74 (20), 6298–6305. doi: 10.1128/AEM.01316-08
Fenchel T. (2002). Microbial behavior in a heterogeneous world. Science 296 (5570), 1068–1071. doi: 10.1126/science.1070118
Fernandez-Martinez J., Pujalte M. J., Garcia-Martinez J., Mata M., Garay E., Rodriguez-Valera F. (2003). Description of Alcanivorax venustensis sp nov and reclassification of Fundibacter jadensis DSM 12178(T) (Bruns and berthe-corti 1999) as Alcanivorax jadensis comb. nov., members of the emended genus Alcanivorax. Int. J. Systematic Evolutionary Microbiol. 53, 331–338. doi: 10.1099/ijs.0.01923-0
Fichtel K., Logemann J., Fichtel J., Rullktter J., Cypionka H., Engelen B. (2015). Temperature and pressure adaptation of a sulfate reducer from the deep subsurface. Front. Microbiol. 6, 13. doi: 10.3389/fmicb.2015.01078
Foster P. L. (2007). Stress-induced mutagenesis in bacteria. Crit. Rev. Biochem. Mol. Biol. 42 (5), 373–397. doi: 10.1080/10409230701648494
Franzmann P. D., Wehmeyer U., Stackebrandt E. (1988). Halomonadaceae fam. nov., a new family of the class proteobacteria to accommodate the genera Halomonas and Deleya. Systematic Appl. Microbiol. 11 (1), 16–19. doi: 10.1016/S0723-2020(88)80043-2
Garel M., Bonin P., Martini S., Guasco S., Roumagnac M., Bhairy N., et al. (2019). Pressure-retaining sampler and high-pressure systems to study deep-Sea microbes under in situ conditions. Front. Microbiol. 10, 13. doi: 10.3389/fmicb.2019.00453
Garel M., Izard L., Vienne M., Nerini D., Tamburini C., Martini S. (2022). A ready-to-use logistic verhulst model implemented in r shiny to estimate growth parameters of microorganisms. bioRxiv. 2022.2007.2029.501982. doi: 10.1101/2022.07.29.501982
Garel M., Panagiotopoulos C., Boutrif M., Repeta D., Sempere R., Santinelli C., et al. (2021). Contrasting degradation rates of natural dissolved organic carbon by deep-sea prokaryotes under stratified water masses and deep-water convection conditions in the NW Mediterranean Sea. Mar. Chem. 231, 12. doi: 10.1016/j.marchem.2021.103932
Gayan E., Govers S. K., Aertsen A. (2017). Impact of high hydrostatic pressure on bacterial proteostasis. Biophys. Chem. 231, 3–9. doi: 10.1016/j.bpc.2017.03.005
Golyshin P. N., Dos Santos V., Kaiser O., Ferrer M., Sabirova Y. S., Lunsdorf H., et al. (2003). Genome sequence completed of Alcanivorax borkumensis, a hydrocarbon-degrading bacterium that plays a global role in oil removal from marine systems. J. Biotechnol. 106 (2-3), 215–220. doi: 10.1016/j.jbiotec.2003.07.013
Grognot M., Mittal A., Mah'moud M., Taute K. M. (2021). Vibrio cholerae motility in aquatic and mucus-mimicking environments. Appl. Environ. Microbiol. 87, e01293–e01221. doi: 10.1128/AEM.01293-21
Grossart H. P., Riemann L., Azam F. (2001). Bacterial motility in the sea and its ecological implications. Aquat. Microbial Ecol. 25 (3), 247–258. doi: 10.3354/ame025247
Grossart H. P., Steward G. F., Martinez J., Azam F. (2000). A simple, rapid method for demonstrating bacterial flagella. Appl. Environ. Microbiol. 66 (8), 3632–3636. doi: 10.1128/AEM.66.8.3632-3636.2000
Gutierrez T., Singleton D. R., Berry D., Yang T. T., Aitken M. D., Teske A. (2013). Hydrocarbon-degrading bacteria enriched by the deepwater horizon oil spill identified by cultivation and DNA-SIP. Isme J. 7 (11), 2091–2104. doi: 10.1038/ismej.2013.98
Hazen T., Dubinsky E., Desantis T., Andersen G., Piceno Y., Jansson J., et al. (2010). Deep-Sea oil plume enriches indigenous oil-degrading bacteria. Science 330 (6001), 204–208. doi: 10.1126/science.1195979
Janda J. M., Abbott S. L. (2014). The genus Shewanella: from the briny depths below to human pathogen. Crit. Rev. Microbiol. 40 (4), 293–312. doi: 10.3109/1040841X.2012.726209
Jones P. G., Vanbogelen R. A., Neidhardt F. C. (1987). Induction of proteins in response to low temperature in Escherichia coli. J. Bacteriology 169 (5), 2092–2095. doi: 10.1128/jb.169.5.2092-2095.1987
Joye S. B., Kleindienst S., Gilbert J. A., Handley K. M., Weisenhorn P., Overholt W. A., et al. (2016). Responses of microbial communities to hydrocarbon exposures. Oceanography 29 (3), 136–149. doi: 10.5670/oceanog.2016.78
Joye S., Kleindienst S., Pena-Montenegro T. D. (2018). SnapShot: microbial hydrocarbon bioremediation. Cell 172 (6), 1336–133+. doi: 10.1016/j.cell.2018.02.059
Kallmeyer J., Pockalny R., Adhikari R. R., Smith D. C., D'hondt S. (2012). Global distribution of microbial abundance and biomass in subseafloor sediment. Proc. Natl. Acad. Sci. United States America 109 (40), 16213–16216. doi: 10.1073/pnas.1203849109
Keegstra J. M., Carrara F., Stocker R. (2022). The ecological roles of bacterial chemotaxis (vol 2022, pg , 2022). Nat. Rev. Microbiol. 20 (8), 505–505. doi: 10.1038/s41579-022-00734-9
Kimes N. E., Callaghan a V, Aktas D. F., Smith W. L., Sunner J., Golding B. T., et al. (2013). Metagenomic analysis and metabolite profiling of deep-sea sediments from the gulf of Mexico following the deepwater horizon oil spill. Front. Microbiol. 4, 17. doi: 10.3389/fmicb.2013.00050
Kiørboe T., Grossart H. P., Ploug H., Tang K. (2002). Mechanisms and rates of bacterial colonization of sinking aggregates. Appl. Environ. Microbiol. 68 (8), 3996–4006. doi: 10.1128/AEM.68.8.3996-4006.2002
Knauss J. A., Garfield N. (2017). Introduction to physical oceanography (Long Grove, Illinois: Waveland Press, Inc).
Kostka J. E., Prakash O., Overholt W. A., Green S. J., Freyer G., Canion A., et al. (2011). Hydrocarbon-degrading bacteria and the bacterial community response in gulf of Mexico beach sands impacted by the deepwater horizon oil spill. Appl. Environ. Microbiol. 77 (22), 7962–7974. doi: 10.1128/AEM.05402-11
Kumar S., Stecher G., Li M., Knyaz C., Tamura K. (2018). MEGA X: molecular evolutionary genetics analysis across computing platforms. Mol. Biol. Evol. 35 (6), 1547–1549. doi: 10.1093/molbev/msy096
Lacal J. (2018). Cellular ecophysiology of microbe: hydrocarbon and lipid interactions. Ed. Krell T. (Switzerland: Sprinter International Publishing AG), 241–254.
Lacal J., Reyes-Darias J. A., Garcia-Fontana C., Ramos J. L., Krell T. (2013). Tactic responses to pollutants and their potential to increase biodegradation efficiency. J. Appl. Microbiol. 114 (4), 923–933. doi: 10.1111/jam.12076
Lambert B. S., Fernandez V. I., Stocker R. (2019). Motility drives bacterial encounter with particles responsible for carbon export throughout the ocean. Limnology Oceanography Lett. 4 (5), 113–118. doi: 10.1002/lol2.10113
Lane D. J. (1991). Nucleic acid techniques in bacterial systematics. Eds. Stackebrandt E., Goodfellow M. (New York: John Wiley and Sons).
Lauro F. M., Bartlett D. H. (2008). Prokaryotic lifestyles in deep sea habitats. Extremophiles 12 (1), 15–25. doi: 10.1007/s00792-006-0059-5
Lee C. (2002). Chemistry of marine water and sediments. Eds. Gianguzza A., Pelizzetti E., Sammartano S. (Berlin, Heidelberg: Springer), 125–146.
Lemaire O. N., Mejean V., Iobbi-Nivol C. (2020). The shewanella genus: ubiquitous organisms sustaining and preserving aquatic ecosystems. FEMS Microbiol. Rev. 44 (2), 155–170. doi: 10.1093/femsre/fuz031
Levin S. A. (1992). The problem of pattern and scale in ecology. Ecology 73 (6), 1943–1967. doi: 10.1007/978-1-4615-1769-6_15
Liu R. Y., Ochman H. (2007). Stepwise formation of the bacterial flagellar system. Proc. Natl. Acad. Sci. United States America 104 (17), 7116–7121. doi: 10.1073/pnas.0700266104
Lochte K., Boetius A., Petry C. (1998). Microbial food webs under severe nutrient limitations: life in the deep sea. Eds. Bell C. R., Brylinsky M., Johnson-Green P. (Halifax, Canada: Atlantic Canada Society for Microbial Ecology).
Macnab R. M. (2003). How bacteria assemble flagella. Annu. Rev. Microbiol. 57, 77–100. doi: 10.1146/annurev.micro.57.030502.090832
Macnab R. M. (2004). Type III flagellar protein export and flagellar assembly. Biochim. Et Biophys. Acta-Molecular Cell Res. 1694 (1-3), 207–217. doi: 10.1016/j.bbamcr.2004.04.005
Maeda K., Imae Y., Shioi J. I., Oosawa F. (1976). Effect of temperature on motility and chemotaxis of Escherichia coli. J. Bacteriology 127 (3), 1039–1046. doi: 10.1128/jb.127.3.1039-1046.1976
Manisegaran M., Bornemann S., Kiesel I., Winter R. (2019). Effects of the deep-sea osmolyte TMAO on the temperature and pressure dependent structure and phase behavior of lipid membranes. Phys. Chem. Chem. Phys. 21 (34), 18533–18540. doi: 10.1039/C9CP03812D
Marquis R. E. (1994). Basic and applied high pressure biology. Eds. Bennett P. B., Marquis R. E. (Rochester, NY: University of Rochester Press), 1–14.
Marquis R. E., Bender G. R. (1987). Current perspectives in high pressure biology. Eds. Jannasch H. W., Marquis R. E., Zimmerman A. M. (London: Academic Press), 65–73.
Mason O. U., Hazen T. C., Borglin S., Chain P. S. G., Dubinsky E. A., Fortney J. L., et al. (2012). Metagenome, metatranscriptome and single-cell sequencing reveal microbial response to deepwater horizon oil spill. Isme J. 6 (9), 1715–1727. doi: 10.1038/ismej.2012.59
Matsumura P., Marquis R. E. (1977). Energetics of streptococcal growth inhibition by hydrostatic pressure. Appl. Environ. Microbiol. 33 (4), 885–892. doi: 10.1128/aem.33.4.885-892.1977
Meganathan R., Marquis R. E. (1973). Loss of bacterial motility under pressure. Nature 246 (5434), 525–527. doi: 10.1038/246525a0
Mitchell J. G. (2002). The energetics and scaling of search strategies in bacteria. Am. Nat. 160 (6), 727–740. doi: 10.1086/343874
Mitchell J. G., Kogure K. (2006). Bacterial motility: links to the environment and a driving force for microbial physics. FEMS Microbiol. Ecol. 55 (1), 3–16. doi: 10.1111/j.1574-6941.2005.00003.x
Mueller R. S., Mcdougald D., Cusumano D., Sodhi N., Kjelleberg S., Azam F., et al. (2007). Vibrio cholerae strains possess multiple strategies for abiotic and biotic surface colonization. J. Bacteriology 189 (14), 5348–5360. doi: 10.1128/JB.01867-06
Nishiyama M., Arai Y. (2017). Tracking the movement of a single prokaryotic cell in extreme environmental conditions. Biophys. J. 116 (3), 545A–545A. doi: 10.1007/978-1-4939-6927-2_13
Nishiyama M., Kojima S. (2012). Bacterial motility measured by a miniature chamber for high-pressure microscopy. Int. J. Mol. Sci. 13 (7), 9225–9239. doi: 10.3390/ijms13079225
Nishiyama M., Sowa Y. (2012). Microscopic analysis of bacterial motility at high pressure. Biophys. J. 102 (8), 1872–1880. doi: 10.1016/j.bpj.2012.03.033
Nishiyama M., Sowa Y., Kimura Y., Homma M., Ishijima A., Terazima M. (2013). High hydrostatic pressure induces counterclockwise to clockwise reversals of the Escherichia coli flagellar motor. J. Bacteriology 195 (8), 1809–1814. doi: 10.1128/JB.02139-12
Ortega D. R., Kjaer A., Briegel A. (2020). The chemosensory systems of Vibrio cholerae. Mol. Microbiol. 114 (3), 367–376. doi: 10.1111/mmi.14520
Otter T., Salmon E. D. (1979). Hydrostatic pressure reversibly blocks membrane control of ciliary motility in Paramecium. Science 206 (4416), 358–361. doi: 10.1126/science.482945
Parkes R. J., Cragg B., Roussel E., Webster G., Weightman A., Sass H. (2014). A review of prokaryotic populations and processes in sub-seafloor sediments, including biosphere:geosphere interactions. Mar. Geology 352, 409–425. doi: 10.1016/j.margeo.2014.02.009
Patching J. W., Eardly D. (1997). Bacterial biomass and activity in the deep waters of the eastern Atlantic - evidence of a barophilic community. Deep-Sea Res. Part I-Oceanographic Res. Papers 44 (9-10), 1655–1670. doi: 10.1016/S0967-0637(97)00040-X
Paul K. L., Morita R. Y. (1971). Effects of hydrostatic pressure and temperature on the uptake and respiration of amino acids by a facultatively psychrophilic marine bacterium. J. Bacteriology 108 (2), 835–843. doi: 10.1128/jb.108.2.835-843.1971
Pavlovic M., Hormann S., Vogel R. F., Ehrmann M. A. (2005). Transcriptional response reveals translation machinery as target for high pressure in Lactobacillus sanfranciscensis. Arch. Microbiol. 184 (1), 11–17. doi: 10.1007/s00203-005-0021-4
Peoples L. M., Bartlett D. H. (2017). Microbial ecology of extreme environments. Eds. Chénard C., Lauro F. M. (Switzerland: Springer Nature), 7–50.
Peoples L. M., Donaldson S., Osuntokun O., Xia Q., Nelson A., Blanton J., et al. (2018). Vertically distinct microbial communities in the Mariana and kermadec trenches. PloS One 13 (4), 21. doi: 10.1371/journal.pone.0195102
Pledger R. J., Crump B. C., Baross J. A. (1994). A barophilic response by 2 hyperthermophilic, hydrothermal vent archaea - an upward shift in the optimal temperature and acceleration of growth-rate at supra-optimal temperatures by elevated pressure. FEMS Microbiol. Ecol. 14 (3), 233–241. doi: 10.1111/j.1574-6941.1994.tb00109.x
Pollard E. C., Weller P. K. (1966). The effect of hydrostatic pressure on the synthetic processes in bacteria. Biochim. Biophys. Acta 11, 573–580. doi: 10.1016/0926-6585(66)90261-5
Poremba K. (1994). Impact of pressure on bacterial activity in water columns situated at the European continental margin. Netherlands J. Sea Res. 33 (1), 29–35. doi: 10.1016/0077-7579(94)90048-5
Raina J.-B., Giardina M., Brumley D. R., Clode P. L., Pernice M., Guagliardo P., et al. (2023). Chemotaxis increases metabolic exchanges between marine picophytoplankton and heterotrophic bacteria. Nat. Microbiol. 8, 510–521. doi: 10.1038/s41564-023-01327-9
Regnard P. (1891). Recherches experimentales sur les conditions physique de la vie dans les eaux (Paris: Masson et Cie).
Roche J., Royer C. A. (2018). Lessons from pressure denaturation of proteins. J. R. Soc. Interface 15 (147), 21. doi: 10.1098/rsif.2018.0244
Schindelin J., Arganda-Carreras I., Frise E., Kaynig V., Longair M., Pietzsch T., et al. (2012). Fiji: An open-source platform for biological-image analysis. Nat. Methods 9 (7), 676–682. doi: 10.1038/nmeth.2019
Scoma A. (2021). Functional groups in microbial ecology: updated definitions of piezophiles as suggested by hydrostatic pressure dependence on temperature. Isme J. 15 (7), 1871–1878. doi: 10.1038/s41396-021-00930-0
Smriga S., Fernandez V. I., Mitchell J. G., Stocker R. (2016). Chemotaxis toward phytoplankton drives organic matter partitioning among marine bacteria. Proc. Natl. Acad. Sci. United States America 113 (6), 1576–1581. doi: 10.1073/pnas.1512307113
Son K., Guasto J. S., Stocker R. (2013). Bacteria can exploit a flagellar buckling instability to change direction. Nat. Phys. 9 (8), 494–498. doi: 10.1038/nphys2676
Stocker R. (2012). Marine microbes see a Sea of gradients. Science 338 (6107), 628–633. doi: 10.1126/science.1208929
Stocker R., Seymour J. R. (2012). Ecology and physics of bacterial chemotaxis in the ocean. Microbiol. Mol. Biol. Rev. 76 (4), 792–812. doi: 10.1128/MMBR.00029-12
Stocker R., Seymour J. R., Samadani A., Hunt D. E., Polz M. F. (2008). Rapid chemotactic response enables marine bacteria to exploit ephemeral microscale nutrient patches. Proc. Natl. Acad. Sci. United States America 105 (11), 4209–4214. doi: 10.1073/pnas.0709765105
Strom S. L. (2008). Microbial ecology of ocean biogeochemistry: a community perspective. Science 320 (5879), 1043–1045. doi: 10.1126/science.1153527
Tamburini C., Boutrif M., Garel M., Colwell R. R., Deming J. W. (2013). Prokaryotic responses to hydrostatic pressure in the ocean - a review. Environ. Microbiol. 15 (5), 1262–1274. doi: 10.1111/1462-2920.12084
Tamburini C., Garcin J., Gregori G., Leblanc K., Rimmelin P., Kirchman D. L. (2006). Pressure effects on surface Mediterranean prokaryotes and biogenic silica dissolution during a diatom sinking experiment. Aquat. Microbial Ecol. 43 (3), 267–276. doi: 10.3354/ame043267
Tamburini C., Goutx M., Guigue C., Garel M., Lefevre D., Charriere B., et al. (2009). Effects of hydrostatic pressure on microbial alteration of sinking fecal pellets. Deep-Sea Res. Part Ii-Topical Stud. Oceanography 56 (18), 1533–1546. doi: 10.1016/j.dsr2.2008.12.035
Taylor J. R., Stocker R. (2012). Trade-offs of chemotactic foraging in turbulent water. Science 338 (6107), 675–679. doi: 10.1126/science.1219417
Terashima H., Kojima S., Homma M. (2008). International review of cell and molecular biology Vol. 270. Ed. Jeon K. W. (San Diego: Elsevier Academic Press Inc), 39–85.
Trent J. D., Yayanos a A (1985). Pressure effects on the temperature range for growth and survival of the marine bacterium Vibrio harveyi - implications for bacteria attached to sinking particles. Mar. Biol. 89 (2), 165–172. doi: 10.1007/BF00392887
Turley C. M. (1993). The effect of pressure on leucine and thymidine incorporation by free-living bacteria and by bacteria attached to sinking oceanic particles. Deep-Sea Res. Part I-Oceanographic Res. Papers 40 (11-12), 2193–2206. doi: 10.1016/0967-0637(93)90098-N
Wakeham S. G., Lee C. (1993). Organic geochemistry. Eds. Engel M. H., Macko S. A. (Boston, MA: Springer), 145–169.
Wang F. P., Wang J. B., Jian H. H., Zhang B., Li S. K., Wang F., et al. (2008). Environmental adaptation: genomic analysis of the piezotolerant and psychrotolerant deep-Sea iron reducing bacterium Shewanella piezotolerans WP3. PloS One 3 (4), 12. doi: 10.1371/annotation/744d7c8d-db8a-4ad4-aec5-62549b1dbefe
Weiman S., Joye S. B., Kostka J. E., Halanych K. M., Colwell R. R. (2021). GoMRI insights into microbial genomics and hydrocarbon bioremediation response in marine ecosystems. Oceanography 34 (1), 124–135. doi: 10.5670/oceanog.2021.121
Wouters P. C., Glaasker E., Smelt J. (1998). Effects of high pressure on inactivation kinetics and events related to proton efflux in Lactobacillus plantarum. Appl. Environ. Microbiol. 64 (2), 509–514. doi: 10.1128/AEM.64.2.509-514.1998
Xie L., Altindal T., Chattopadhyay S., Wu X. L. (2011). Bacterial flagellum as a propeller and as a rudder for efficient chemotaxis. Proc. Natl. Acad. Sci. United States America 108 (6), 2246–2251. doi: 10.1073/pnas.1011953108
Yang S., Li M. Q., Lai Q. L., Li G. Z., Shao Z. Z. (2018). Alcanivorax mobilis sp nov., a new hydrocarbon-degrading bacterium isolated from deep-sea sediment. Int. J. Systematic Evolutionary Microbiol. 68 (5), 1639–1643. doi: 10.1099/ijsem.0.002612
Yang T. T., Nigro L. M., Gutierrez T., D'ambrosio L., Joye S. B., Highsmith R., et al. (2016). Pulsed blooms and persistent oil-degrading bacterial populations in the water column during and after the deepwater horizon blowout. Deep-Sea Res. Part Ii-Topical Stud. Oceanography 129, 282–291. doi: 10.1016/j.dsr2.2014.01.014
Yayanos a A (1986). Evolutional and ecological implications of the properties of deep-Sea barophilic bacteria. Proc. Natl. Acad. Sci. United States America 83 (24), 9542–9546. doi: 10.1073/pnas.83.24.9542
Yayanos a A (2001). Deep-sea piezophilic bacteria. Methods Microbiol. 30, 615–637. doi: 10.1016/S0580-9517(01)30065-X
Yayanos a A, Pollard E. C. (1969). A study of the effects of hydrostatic pressure on macromolecular synthesis in Escherichia coli. Biophys. J. 9, 1464–1482. doi: 10.1016/S0006-3495(69)86466-0
Yayanos a A, Vanboxtel R., Dietz a S (1983). Reproduction of bacillus stearothermophilus as a function of temperature and pressure. Appl. Environ. Microbiol. 46 (6), 1357–1363. doi: 10.1128/aem.46.6.1357-1363.1983
Yuan J. H., Berg H. C. (2010). Thermal and solvent-isotope effects on the flagellar rotary motor near zero load. Biophys. J. 98 (10), 2121–2126. doi: 10.1016/j.bpj.2010.01.061
Zhao X., Ford R. M. (2022). Marine bacteria chemotaxis to crude oil components with opposing effects. bioRxiv. 2022.2001.2026.477900. doi: 10.1101/2022.01.26.477900
Zhou M., Liu Z. S., Wang J. Q., Zhao Y. X., Hu B. L. (2022). Sphingomonas relies on chemotaxis to degrade polycyclic aromatic hydrocarbons and maintain dominance in coking sites. Microorganisms 10 (6), 13. doi: 10.3390/microorganisms10061109
Zobell C. E., Cobet a B (1962). Growth, reproduction, and death rates of escherichia coli at increased hydrostatic pressures. J. Bacteriology 84 (6), 1228–&. doi: 10.1128/jb.84.6.1228-1236.1962
Zobell C. E., Johnson F. H. (1949). The influence of hydrostatic pressure on the growth and viability of terrestrial and marine bacteria. J. Bacteriology 57 (2), 179–189. doi: 10.1128/jb.57.2.179-189.1949
Keywords: high hydrostatic pressure, low temperature, Halomonas, Alcanivorax, Shewanella, motility, Deepwater horizon (DWH), deep sea
Citation: Mullane KK, Nishiyama M, Kurihara T and Bartlett DH (2023) Compounding deep sea physical impacts on marine microbial motility. Front. Mar. Sci. 10:1181062. doi: 10.3389/fmars.2023.1181062
Received: 07 March 2023; Accepted: 02 May 2023;
Published: 23 May 2023.
Edited by:
Federico Baltar, University of Vienna, AustriaReviewed by:
Aude Picard, University of Nevada, Las Vegas, United StatesChloé Baumas, UMR7294 Institut Méditerranéen d’océanographie (MIO), France
Uria Alcolombri, ETH Zürich, Switzerland
Copyright © 2023 Mullane, Nishiyama, Kurihara and Bartlett. This is an open-access article distributed under the terms of the Creative Commons Attribution License (CC BY). The use, distribution or reproduction in other forums is permitted, provided the original author(s) and the copyright owner(s) are credited and that the original publication in this journal is cited, in accordance with accepted academic practice. No use, distribution or reproduction is permitted which does not comply with these terms.
*Correspondence: Kelli K. Mullane, kmullane@ucsd.edu