- 1The University of the South Pacific, Suva, Fiji
- 2Department of Science, The University of Fiji, Lautoka, Fiji
- 3Geoscience, Energy and Maritime (GEM) Division, Pacific Community (SPC), Suva, Fiji
The Pacific Island Countries (PICs) are exposed to extreme wave conditions which are projected to be exacerbated by rising sea levels due to climate change, prompting the need for strategic planning of coastal communities and assets. Nature-based protection has been proposed as a sustainable solution to promote the resilience of coastal areas from physical impacts such as wave-induced erosion. In this study, we investigate the potential coastal protection service of shallow sub-tidal low-canopy seagrass beds, dominated by Halodule uninervis, on the rate of wave height and wave energy reduction on a barrier and fringing reefs. The data was collected using bottom-mounted pressure sensors to measure wave height and energy reduction as waves moved toward the shoreline across the seagrass beds. The results show that on average, the seagrass beds were able to reduce wave height by 30% and energy by 47% in both reef environments. These reduction rates are strongly influenced by water depth, seagrass characteristics and local reef conditions. Based on these results, seagrasses can strengthen the resilience of coastal shorelines to wave erosion, thus conserving healthy low-canopy seagrass habitats has measurable benefits for shoreline protection in Fiji and other PICs.
Introduction
Seagrasses are unique marine flowering plants that form diverse, extensive beds in shallow coastal waters, with nearly half of the global seagrass coverage occurring in the Indo-Pacific (Waycott et al., 2011; McKenzie et al., 2021). Seagrass beds can be made up of a single species or exist as a mixed bed with several species (Vonk et al., 2008) and are found along the coastline of all continents except Antarctica (Short et al., 2007). Many ecosystem functions and economic services are provided by seagrass beds but some of these like coastal protection are indirect and quite often overlooked (Brodie et al., 2020a). These services include pathogen reduction (Lamb et al., 2017), nutrient cycling and particle trapping, stabilization of sediments (Cullen-Unsworth et al., 2014), provision of food for associated faunal species (Nordlund et al., 2016; Nordlund et al., 2018), and habitat and nursery grounds for commercial and recreational fish (York et al., 2017). It is increasingly recognised that a service provided by seagrass beds is carbon sequestration. Seagrass beds are classified as blue carbon ecosystems alongside mangroves and marshes, and recently, these ecosystems have recently gained attention for their potential to aid in climate change mitigation (Bedulli et al., 2020). Furthermore, seagrass ecosystems are among the most efficient natural carbon sinks on the earth, sequestering CO2 through photosynthesis and storing organic carbon beneath their soils for decades to millennia (Macreadie et al., 2021). These services make seagrasses one of the most valuable ecosystems on earth considering the numerous services provided to the marine environment, the climate, and human societies.
Seagrass beds are also known for their ability to alter local hydrodynamics such as reducing current magnitudes (Fonseca et al., 1982; Fonseca and Koehl, 2006; Lowe et al., 2007), wave velocity, and turbulence (Luhar et al., 2010; Ma et al., 2013) wave setup (van Rooijen et al., 2016a) as well as reducing wave energy (John et al., 2015; Van Rooijen et al., 2015; Serrano Ureña, 2016; James et al., 2021). These interactions allow seagrasses to play an important role as a natural barrier in coastal environments (van Rooijen et al., 2016b) thereby playing an important role in mitigating the impacts of coastal hazards such as wave energy and coastal erosion (Ondiviela et al., 2014; Guannel et al., 2016; Arkema et al., 2017; James et al., 2021). Thus the presence of nearshore seagrass beds places them as natural coastal protection and coastal managers and engineers are becoming increasingly aware of this nature-based service (James et al., 2021).
Annually, billions of dollars are lost due to the damage caused by natural coastal hazards such as coastal erosion and flooding (Hinkel et al., 2013; Hinkel et al., 2014). The damage inflicted by these events is projected to be greater future with increasing sea levels and cyclones intensity (Woodruff et al., 2013; Neumann et al., 2015) placing the livelihoods of coastal communities in low-lying countries such as those in the Pacific at greater risk (McInnes et al., 2014; Quataert et al., 2015; Vitousek et al., 2017; Storlazzi et al., 2018; Vousdoukas et al., 2018). To mitigate the risk of significant damages, effective strategies need to be implemented and at the moment, engineered solutions such as seawalls are widely adopted. But the engineered mitigation approaches are expensive to construct and maintain, thereby shifting the attention to the option of natural ecosystems to build resilience by integrating ecosystem services into adaptation strategies (Arkema et al., 2017).
Globally, utilising natural ecosystem services as a solution for climate adaptation and mitigation is increasingly recognized (Gattuso et al., 2018; Bindoff et al., 2019). The IPCC AR6 report on climate change mitigation stated that the protection of coastal ecosystems is a mitigation option and should be advocated as a climate solution on a national scale with multiple benefits (Lecocq et al., 2022). However, such benefits cannot be fully utilized due to a lack of available information which is often the case in the Pacific Island Countries (PICs). Hence, it is important to fully understand how natural ecosystems like seagrass beds can play a role in climate change mitigation and adaptation and to work actively to fill gaps in our knowledge as seagrasses are found in eleven of the fourteen Pacific Small Island Developing States (PSIDS) (Brodie et al., 2020b).
The Nordlund et al. (2016) study on ecosystem services provided by seagrass genera looked at six bioregions where they found information gaps on coastal protection service for “low canopy” (relatively small blade size) seagrass species such as Halodule uninervis in the Tropical-Indo Pacific. The current study, therefore, addresses this information gap by measuring if H. uninervis dominated seagrass beds, in two different shallow coastal environments in Fiji, provide measurable wave energy reduction using in-situ field data.
In Fiji, seagrass habitat is significant in nearshore areas and has ecosystem services that underpin local livelihoods (McKenzie and Yoshida, 2020). The current status and condition of seagrass resources in Fiji are not considered to be in decline however only about 16% of Fiji’s seagrass resources are estimated to occur within marine protected areas (McKenzie et al., 2021). To date, the majority of seagrass research published for Fiji focuses on intertidal rather than sub-tidal areas.
Materials and methods
Study locations
The study was conducted in Fiji, an island group in the South Pacific Ocean (Figure 1A). The study locations (1) Suva Lagoon and (2) Maui Bay are on the southern coast of Fiji’s main island of Viti Levu (Figure 1B). These locations were selected because (1) they were found within two different nearshore reef system types, a barrier reef, and a fringing reef as discussed by Guannel et al. (2016), (2) they both had continuous shallow sub-tidal Halodule uninervis dominated seagrass beds for which no information is available on coastal the protection service offered in the tropical pacific region (Nordlund et al., 2016), and (3) because they were both located close to existing semi-permanent tide recording data loggers that could be utilised to validate the accuracy of the pressure sensors used for collecting wave data.
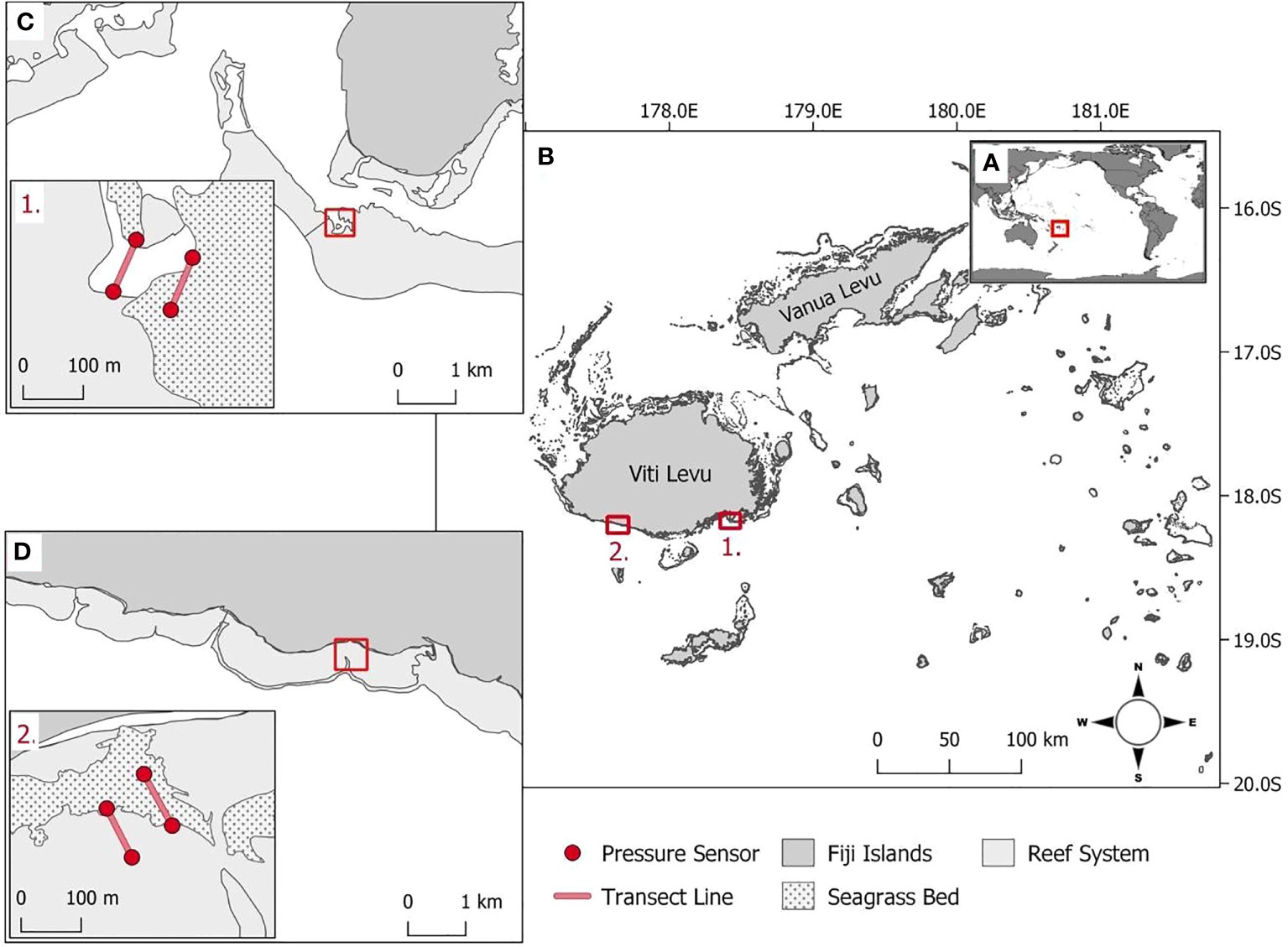
Figure 1 (A) Fiji’s position within the South Pacific Ocean. (B1) Suva Lagoon and (B2) Maui Bay are the two study locations on the island of Viti Levu. (C) Within Suva Lagoon and (D) Maui Bay, pressure sensors (red dot markers, inserts C1, D2) were deployed 100m apart on either end of the seagrass bed and non-seagrass (control) bed denoted with transects (red line, inserts C1, D2).
Suva Lagoon is sheltered by barrier reefs including Suva’s main Sosoikula barrier reef where fieldwork was conducted (Figure 1C). The fieldwork at Sosoikula was undertaken sub-tidally at the back edge of the barrier reef (Figure 1C. inset 1.). Separating the barrier reefs are deep channels and passages that allow currents to be driven by both tidal cycles and local wind conditions (Singh and Aung, 2008). The tidal cycle in Suva Lagoon as in most other places is semidiurnal. The average annual high and low tide records for Suva Lagoon are 2.11m and 0.26m. A nearshore wave hindcast for Suva (over 30 years between 1979 and 2013) estimated the annual mean wave height and wave period to be 1.30m and 13.33s respectively (Bosserelle et al., 2015a).
Maui Bay to the west of Suva Lagoon is sheltered by a 650m wide fringing reef with a relatively shallow reef flat (Figure 1D). The fieldwork in Maui Bay was undertaken sub-tidally at a jetty area straight off the shore (Figure 1D. inset 2.). Although jetties have been found to have influence some on local hydrodynamics such as increased wave set-up (e.g. António et al., 2020) which may also be the case for Maui Bay, the current study does not capture this information but can be recommended for future studies. A nearshore wave hindcast for Maui Bay (over 30 years between 1979 and 2013) show the annual mean wave height to be 1.07m with a mean wave period of 13.89s (Bosserelle et al., 2015a). The water levels on the reef flat are mostly controlled by waves which are modulated by a semidiurnal tide spring and neap cycles (Wandres et al., 2020). The average annual high and low tide records for Maui Bay are 2.12m respectively, and depth-averaged currents are modulated by infragravity waves and oriented toward the shore (Bosserelle et al., 2015b). In both study locations, H. uninervis dominated seagrass beds are present (Chand, 2019; Singh et al., 2019).
Seagrass bed canopy characteristics
Seagrass bed canopy characteristics were collected to establish the effect of seagrasses on wave energy reduction in the sub-tidal coastal zones. In both locations, a 100m transect was laid out perpendicular to the shoreline from the shoreward edge to the ocean-facing edge of the seagrass beds before data collection commenced. Following the method of Duarte and Kirkman (2001), blade height, blade width, and shoot density were recorded in a 50 x 50 cm quadrat every 10 m along the 100 m transect to determine the above ground physical characteristics of the seagrass beds. Five blade widths and blade height measurements were made per quadrat and averaged. The average shoot density was calculated using smaller squares of 25cm x 25cm, where the total values per square were multiplied by 16 to calculate shoot density for 1 square meter. Then using Excel, the confidence intervals of each seagrass characteristic were calculated.
In situ wave parameters
In situ wave data were collected sub-tidally using four RBRsolo3 pressure sensors (accuracy ± 0.05% FS and resolution< 0.001% FS). The pressure sensors were deployed at each location with two being placed 100 meters apart (end of transect) on the seaward and shoreward side of the seagrass bed and two in equivalent positions in an adjacent non-seagrass bed (Figures 1C, D, insets 1. and 2.). The positioning of the sensors was made to provide two adjacent transects for which environmental parameters were as close as possible in all regards except for the presence or absence of seagrass (the latter being the control). This included making sure the angle of transects to the approaching waves was equivalent. The pressure sensors were preprogrammed to record pressure readings continuously at one 1Hz for 6 days, and the spectral data was stored internally and downloaded upon retrieval. The data collection in Suva Lagoon was conducted in mid-December 2018, while the data collection in Maui Bay was collected in mid-February 2019.
The deployment periods fall during the wet season in Fiji (November to April) when cyclones usually occur, bringing swells to Fiji’s coastal waters. Such energetic wave conditions were observed to have an impact on seagrasses such as defoliating the leaves and even uprooting the plants thereby affecting their wave reduction ability (e.g. Oprandi et al., 2020; James et al., 2021). The deployments were therefore timed to capture the most energetic wave conditions possible. However, during the field study only calm conditions were recorded.
Wave analysis
Raw data collected by the pressure sensors were analysed and compared to data obtained from nearby semi-permanent tidal gauges (previously deployed by the Pacific Community (SPC) close to the study locations) to verify sensor performance during the six-day deployment periods. Then, using MATLAB2018b software, a spectral analysis was performed on the raw pressure time series data using the Fourier transformation method. Following Wandres et al. (2020) the significant wave heights were calculated from the partially integrated power spectrum using Equation (1).
Where f is the frequency and E is the energy spectral density of the wave spectrum. The cutoff frequencies for shortwave were set to fmin = 0.02 and fmax = 0.6 Hz (wave period between 1.6 and 50 seconds). Using the method proposed by Denny (2014), the resulting significant wave heights were consequently converted into wave energy using the equation for kinetic energy per area for linear waves (Equation 2).
Where E is wave energy, ρ is the density of seawater = 1025 kg/L, g is the acceleration due to gravity = 9.81 m/s2 and is the hourly significant wave heights raised to the power of two.
Wave reduction calculations
Overall wave reduction was calculated by subtracting the wave heights and wave energy on the shoreward side from wave heights and wave energy at the seaward side of the seagrass bed and non-seagrass bed using Equation 3 and Equation 4.
Furthermore, wave height and wave energy reduction were expressed as percentages to distinguish the rate of wave reduction at different water depths using Equation 5 and Equation 6.
Statistical analysis
Statistical analysis was conducted to test the null hypothesis that wave height and energy reduction were no different in the seagrass and non-seagrass areas at the study sites using Excel and IBM SPSS Statistical software. Linear regressions were conducted to determine the association between wave height reduction and wave energy reduction, as well as water depth vs. wave height reduction. Mann-Whitney U Tests compared the wave height reduction and wave energy reduction between the seagrass and non-seagrass areas. The non-parametric Mann-Whitney U Test was used as data were not normally distributed.
Results
Seagrass bed canopy characteristics
The Suva Lagoon seagrass bed was found to have an average shoot density of 2,500 ± 20 shoots per meter square and an average leaf blade width of 0.36 ± 0.05cm and a leaf blade length of 15.17 ± 4cm (Table 1). Maui Bay on the other hand had a seagrass bed with an average shoot density of 1,200 ± 15 shoots per meter square, a leaf blade width of 0.34cm ± 0.03, and a leaf length of 12.51 ± 3cm. The Halodule uninervis dominated seagrass bed in Suva Lagoon was therefore significantly denser and had a longer leaf blade length.

Table 1 Comparison of average Seagrass bed canopy characteristics (shoot density, blade size, and length) for Suva Lagoon and Maui Bay locations with 95% Confidence Interval (±).
Wave characteristics
The six-day raw time series water depth data showed a close fit with data obtained from the nearby tide gauges at both locations for the same deployment period (Figure 2), thereby confirming the suitability of the data for further analysis. In both study locations, waves are primarily wind-driven, with average significant wave heights noted during the deployment period ranging from 0.07m within the seagrass bed to 0.1m at the non-seagrass area in Suva Lagoon (Figures 3A, B), and 0.13m in the seagrass bed to 0.12m at the non-seagrass area in Maui Bay.
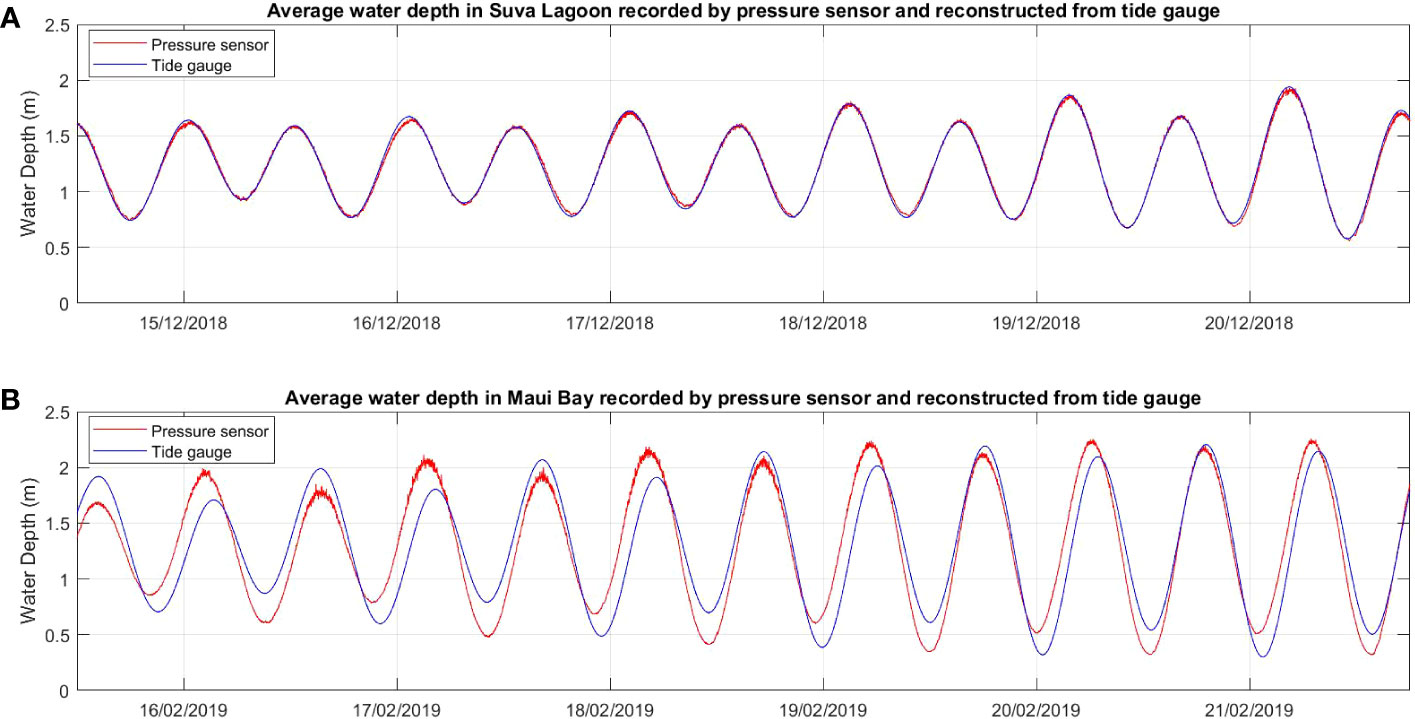
Figure 2 Comparison of time series water depth data recorded by the pressure sensors deployed in the current study with water depth data over the same time period measured by existing semi-permanent tide gauges in the two study locations (A) Suva Lagoon and (B) Maui Bay.
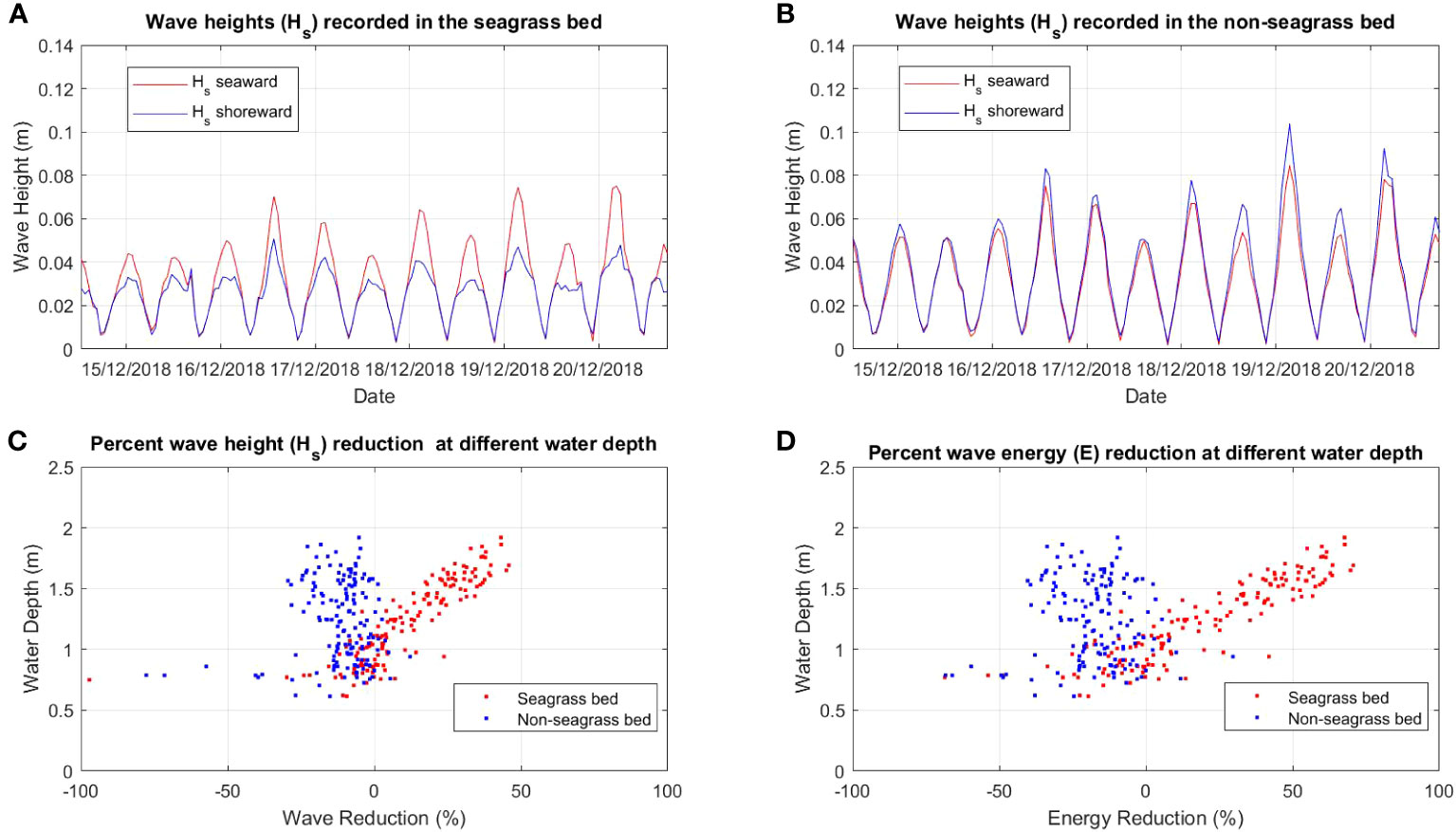
Figure 3 Comparison of wave height (Hs) between (A) seagrass bed and (B) non-seagrass (control) bed and the percent of wave (Hs) reduction (C) and wave energy (E) reduction (D) at different water depths in the seagrass bed area and non seagrass area in Suva Lagoon.
The wave analysis performed for both locations showed a clear reduction of wave heights in the seagrass beds compared to the non-seagrass areas (Figures 3A, B, 4A, B). In Suva Lagoon, a maximum wave height of 0.075m recorded at the seaward side of the seagrass bed decreases to 0.04m by the time it reaches the shoreward side (Figure 3A), resulting in a reduction of 0.035m. In the non-seagrass area however, there was a 0.02m increase in wave height from the maximum wave height of 0.082m that was recorded at the seaward side of the area. This resulted in a maximum wave height of 0.1m observed at the shoreward side of the non-seagrass area (Figure 3B). In Maui Bay, a maximum wave height of 0.13m recorded at the seaward of the seagrass bed experienced 0.02m reduction resulting in maximum wave height of 0.11m at the shoreward side (Figure 4A). In contrast, waves in the non-seagrass area showed little reduction in height (Figure 4B).
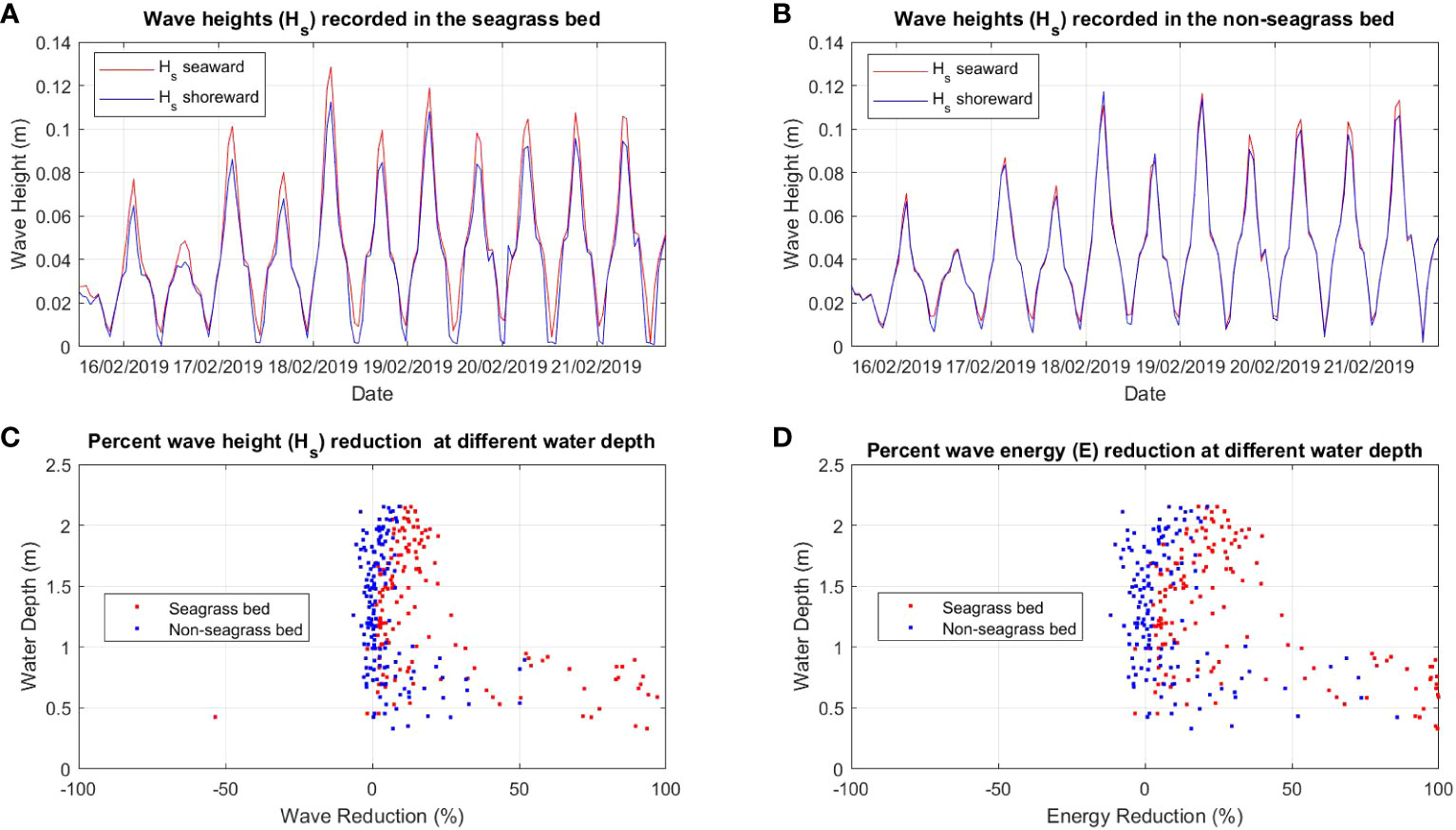
Figure 4 Comparison of wave height (Hs) between (A) seagrass bed and (B) non-seagrass bed (control) and the percent of wave (Hs) reduction (C) and wave energy (E) reduction (D) at different water depths in the seagrass bed area and non seagrass area in Maui Bay.
Further analysis was performed for wave reduction at different water depths to determine the reduction for different submergence ratios between seagrass leaf blade length and the water column, and the influence of water depth on the rate of reduction. Wave height and wave energy reduction at water depths between 0.5m - 2m were observed (Figures 3C, D, 4C, D). Waves travelling through the seagrass bed in Suva Lagoon experienced up to 45% reduction in wave heights and a 70% reduction in wave energy (Figure 3C) as compared to the non-seagrass area where maximum wave height and a wave energy reduction of 15% and 23% were observed (Figure 3D). The opposite trend was observed for the Maui Bay seagrass bed where the maximum wave height reduction is 96% in the seagrass bed and 52% in the non-seagrass area at water depths less than 1m (Figure 4C). However, wave height reduction in seagrass bed dropped to 30% and 10% in the non-seagrass area respectively as the water depth increases beyond 1m. In terms of wave energy, the reduction was also noticeable in the 0.5m to 1m water depth range. There was a 100% wave energy reduction in the seagrass bed and a 76% reduction in the non-seagrass area at water depths less than 1 m (Figure 4D). In contrast, for water depth over 1m, the seagrass bed was able to reduce wave energy by up to 50%, whereas only 25% reduction in energy was recorded at non-seagrass areas.
The rate of wave height and energy reduction imposed by the seagrass bed in Suva Lagoon increases with the water depth (Figures 3C, D). The opposite trend is observed for the seagrass bed in Maui Bay where the rate of water height and energy reduction decreases with decreasing water depth (Figures 4C, D). This suggests that the water depth may affect the way seagrass beds reduce wave energy in different sub-tidal reef environments, but further study is needed to ascertain this. The overall pattern deduced from the results is that seagrass beds in the study location always reduce wave height and energy up to 2m water depth. In Suva Lagoon, the reduction of wave energy increases with depth, whereas in Maui the reduction decreases with depth.
Statistical outputs
The linear regressions identified associations between wave height reduction, wave energy reduction and water depth, and showed an overall significant association in wave reduction between the seagrass bed and the non-seagrass bed in Suva Lagoon and Maui Bay.
For the study site in Suva lagoon, there is a strong positive relationship between wave height reduction and wave energy reduction in the seagrass bed (r2 = 0.94, F = 2410, p<0.001) as well as the non-seagrass area (r2 = 0.91, F = 1451, p<0.001). When looking at the effect of water depth on wave height reduction and wave energy reduction, there is a weak positive relationship in the seagrass bed for water depth vs. wave height reduction (r2 = 0.78, F = 525, p<0.001) and water depth vs. wave energy reduction (r2 = 0.66, F = 289, p<0.001). On the other hand, in the non-seagrass area, there is a negative relationship between water depth and wave height reduction (r2 = 0.41, F = 103, p<0.001) and water depth and wave energy reduction (r2 = 0.43, F = 112, p<0.001).
For the study site in Maui Bay, there is a positive relationship between wave height reduction and wave energy reduction in the seagrass bed (r2 = 0.47, F = 132, p<0.001) as well as the non-seagrass area (r2 = 0.75, F = 433, p<0.001). In terms of the effect of water depth on wave height reduction and wave energy reduction, there is a weak positive relationship in the seagrass bed for water depth vs. wave height reduction (r2 = 0.04, F = 5, p>0.001) and water depth vs. wave energy reduction (r2 = 0.36, F = 82, p<0.001). A similar weak positive relationship is also observed in the non-seagrass area for water depth vs. wave height reduction (r2 = 0.02, F = 2, p>0.001) and water depth vs. wave energy reduction (r2 = 0.14, F = 23, p<0.001).
The Mann-Whitney tests show that there is a significant difference in wave height reduction and wave energy reduction between the seagrass bed and non-seagrass area at the study locations (p-values< 0.0001 at α = 0.05) (Table 2). The overall effect observed is that wave height and energy reduction were greater in seagrass beds compared to non-seagrass areas in both Suva Lagoon and Maui Bay.
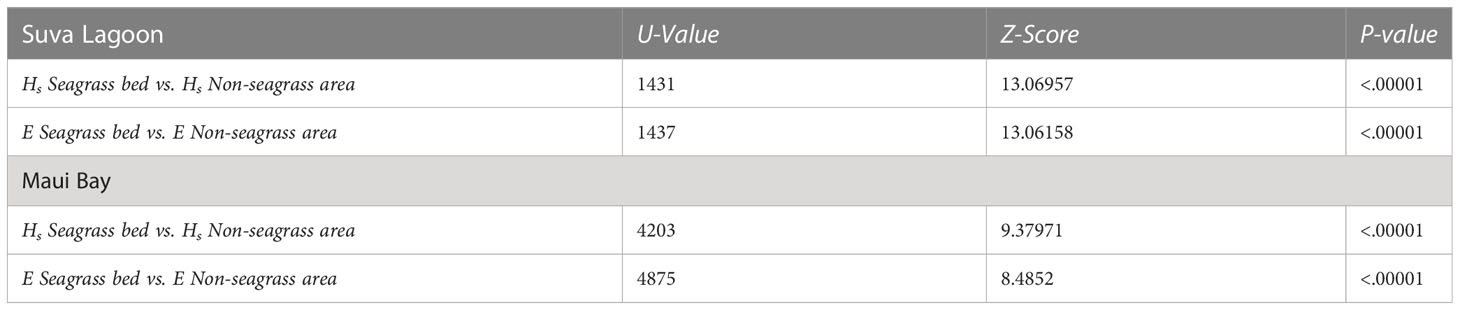
Table 2 Outputs of the Mann-Whitney U Test (α = 0.05, n = 150) assessing the wave height (Hs) reduction and wave energy (E) reduction between the seagrass and non-seagrass transects at the study locations.
Discussion
The rate of wave energy reduction in seagrass beds has been studied primarily on high canopy seagrass genera, in both laboratory and field settings elsewhere (e.g. Fonseca and Cahalan, 1992; Bradley and Houser, 2009; John et al., 2015; John et al., 2016; James et al., 2021), but not much in the Pacific Islands where there is lack of research and information. In the present study, we investigated wave reduction in low-canopy sub-tidal seagrass habitats in two different types of coastal reef systems using in situ field observations. Our results indicate that the presence of seagrass beds makes a significant difference to the overall wave height (30% reduction) and wave energy (47% reduction) moving toward the shoreline, resulting in a gradual release of wave energy which lessens the strength of wave energy impacting the shoreline.
Considering that H. uninervis is a tropical Indo-Pacific occurring species (Short et al., 2007), a comparison was made with Enhalus acoroides, the only species from the same region used in similar studies that were conducted in a laboratory setting (John et al., 2015). Both studies showed that E. acoroides wave height reduction of approximately 50% is higher than that of H. uninervis that we observed in Suva Lagoon (by 5%), but lower than the maximum wave height reduction observed in Maui Bay (by 46%) (Figures 3C, 4C; Tables 3, 4). Additionally, a study conducted by Reidenbach and Thomas (2018) showed that Zostera marina with a canopy height of 53cm was able to reduce wave height by 25–49%. This finding coincides with Ondiviela et al. (2014) who stated that seagrass with large canopy height such as E. acoroides might provide higher wave reduction than the smaller seagrass species. However, H. uninervis despite having a relatively small blade length (12-15cm) compared to E. acoroides (30-150cm) and Zostera marina, managed to impose considerable wave height reduction during the study period, thus confirming its effectiveness in reducing wave height.
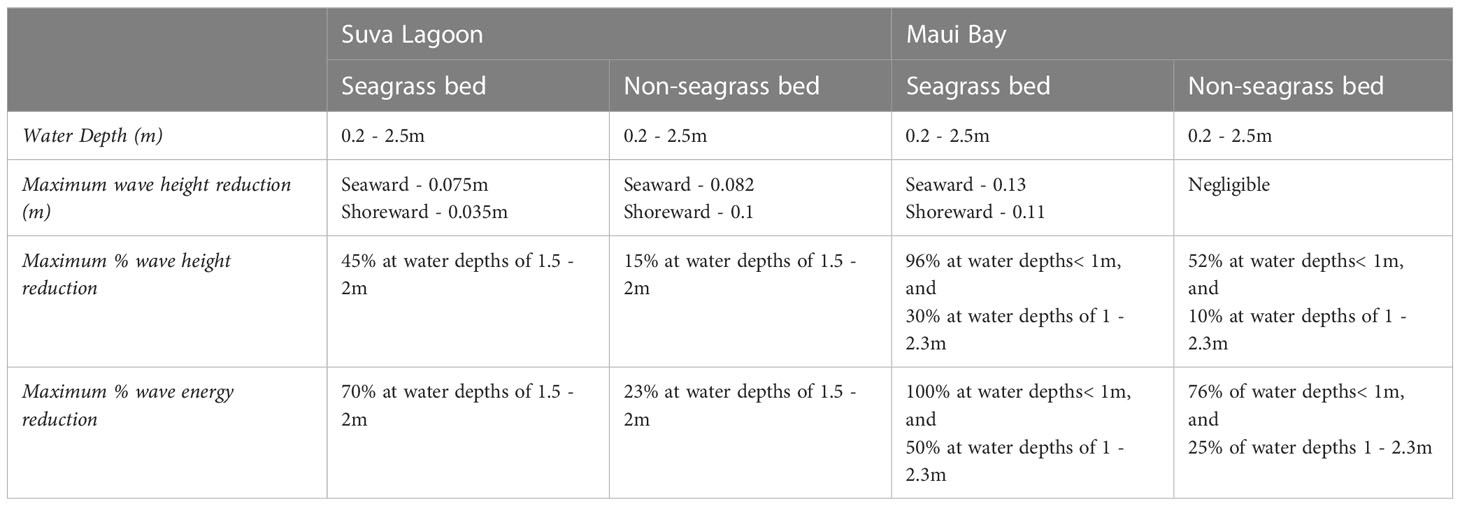
Table 3 The maximum recorded wave height (in meters and percentage) and wave energy reduction (in percentage) for the study locations.

Table 4 Percent wave height reduction due to water depth as observed in the current and prior studies.
In terms of wave energy reduction, H. uninervis was found to impose reduction rates comparable to high canopy seagrass species like Halodule wrightii, Syringodium filiforme, Thalassia testudinum, all of which occur primarily in the tropical Atlantic region and Zostera marina a seagrass species found in the temperate Pacific and Atlantic region”. These seagrasses have blade lengths of 20cm, 65cm, 30 cm and 100 cm respectively (Short et al., 2007). A preliminary evaluation of wave reduction by these four seagrasses conducted by Fonseca and Cahalan (1992) showed approximately 40% percent wave energy reduction per meter of each seagrass bed. Our results showed that H. uninervis has an energy reduction rate between 30% - 47% in Suva Lagoon (Figure 3D) and up to 100% in Maui Bay (Figure 4D) depending on local conditions (Table 3). This suggests the effective role that H. uninervis beds may have in reducing wave energy.
Our study also showed that water depth has a significant influence on wave reduction which is consistent with the findings of Brander et al. (2004); Manca (2010); Prinos et al. (2010); Koftis and Prinos (2011); Stratigaki et al. (2011); John et al. (2015); Reidenbach and Thomas (2018) (Table 4). Water depth affects reduction through the submergence ratio (seagrass canopy height/leaf length to water depth). In Suva Lagoon, where the water depth ranges from 0.5m at low tide to 2.5m during high tide, H. uninervis reduced wave height and energy with increasing water depth (Figures 3C, D). This result contradicted Fonseca and Cahalan (1992), who stated that wave energy reduction is expected to be higher in lower water depths. It is suspected that the dense Suva Lagoon seagrass bed may have forced a raised flow level above the top canopy of the seagrass creating a different flow regime thus a different energy reduction profile. This also suggests that the structural characteristics of seagrass canopies may be important in determining microscale hydrodynamics, but additional study is required to confirm this. On the other hand, the results from Maui Bay (fringing reef) agree with Fonseca and Cahalan (1992) finding, showing a decrease in wave height and energy reduction as the water depth increases (Figures 4C, D). This observation suggests that even though water depth has a strong influence on wave reduction in seagrass beds, the effectiveness of H. uninervis in reducing wave height and energy is not the same for different locations and may also depend on the location of the seagrass bed in the reef environment.
For Suva Lagoon results, it appears that the seagrass bed does not effectively reduce wave energy in very shallow water, but as water depth increases (0.5m or more), the seagrass bed becomes more effective at wave energy reduction (Figure 4D). In very shallow water, it can be assumed the waves are swashing in (i.e. have broken), whereas in shallow water, the waves are rolling in and breaking partly due to increased drag on the seabed caused by seagrass, which causes the wave to topple forwards and break (Figure 5). However, it should be noted that in Suva Lagoon, these shallow depths occur at low tides during the deployment period.
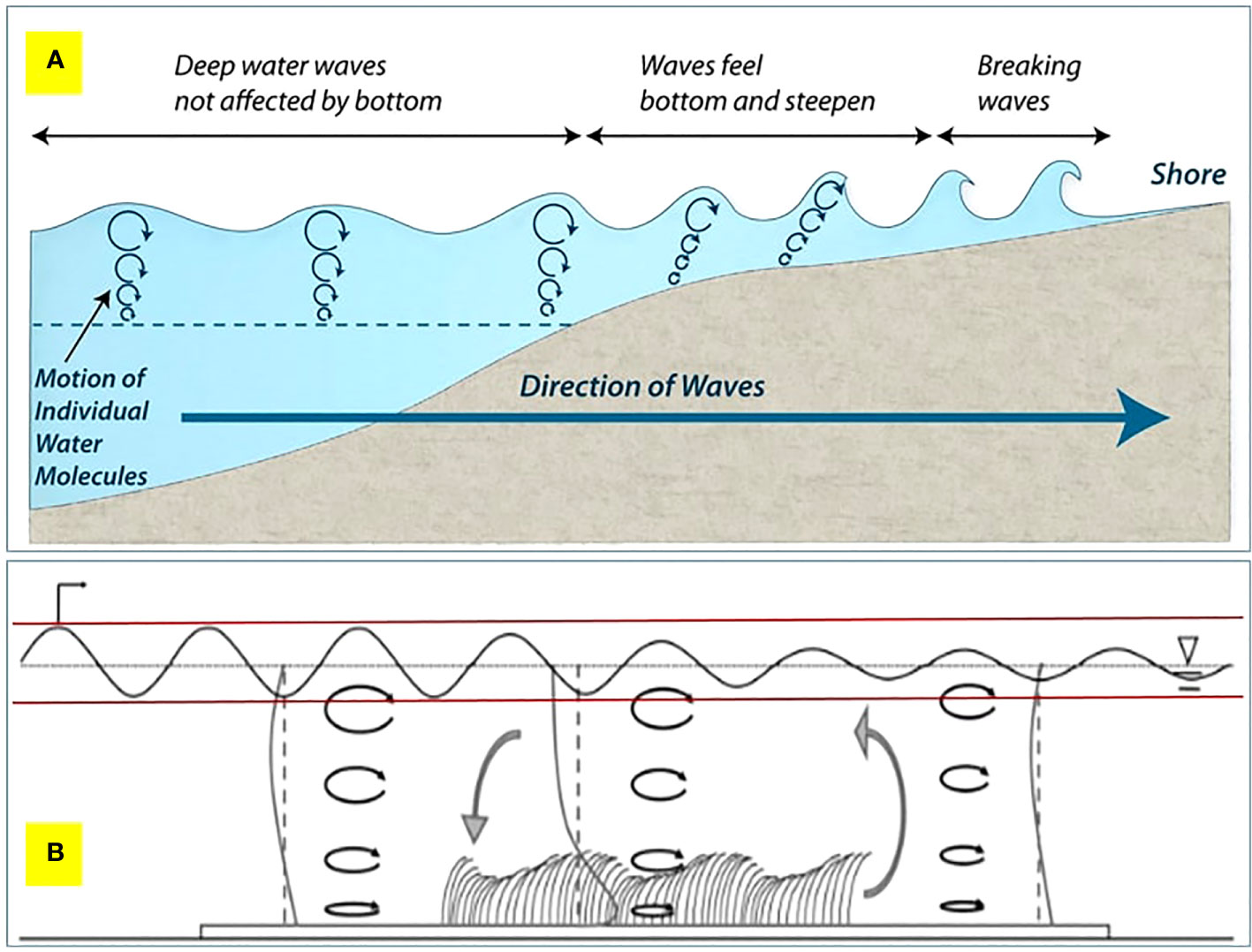
Figure 5 (A) The evolution of waves travelling towards shore from the ocean. (B) A qualitative overview of the hydrodynamic conditions within a conceptual seagrass bed (not to scale). The reduction in wave height is represented by a fine black oscillatory line between the two red horizontal lines along the seagrass bed. The direction of wave propagation is from left to right. The black ellipses with arrows indicate the wave orbitals. Vertical profiles of the mean current (heavy grey lines) are shown upstream, downstream, and in a seagrass bed. At each position, the vertical dashed lines indicate the axis position for the profile. The local circulation pattern, shown by the large grey arrows, results from the difference in the velocity profile within and outside the meadow. Figure 5B is adapted from the work of Luhar et al. (2010).
In management terms, this (possibly) means two things: First, seagrass has an upside-down U-shaped effectiveness, with wave energy being maximally reduced when the wave bottom drags in the seagrass bed (Figure 5). If the water is too deep, the wave system will not be affected, and if the water is too shallow, the wave will be in swash mode, which is less effective at reducing energy. The optimal point of the U-shaped curve is affected by tidal height and seagrass blade length. Secondly, in storm conditions the bigger wave system will move the zone of most effective energy reduction into deeper water as the drag on the bottom of the wave system to make it topple will be deeper. The shifting of the optimal energy reduction zone means that seagrass has to cover various depths (with the possible presence of different species in some places), not just a strip across at a single depth, as wave depth and tidal height will affect the zone of optimal energy reduction. Furthermore, different depth zones of the seagrass beds operate in different conditions, with shallow seagrass beds providing energy reduction to reduce more normal conditions (and reducing incremental erosion), whereas a deeper seagrass bed operates in big wave/storm conditions to break incoming waves (and reduce overtopping, coastal flooding, etc.) (Guannel et al., 2016; James et al., 2021). This highlights the importance of protecting seagrass beds as well as shallow near-shore zones.
Shoot density was also found to significantly affect wave height reduction along a vegetation bed (Manca, 2010; Stratigaki et al., 2011; Manca et al., 2012). Manca et al. (2012) conducted studies on two P. oceanica shoot densities (360 shoots/m2 and 180 shoots/m2) and found wave height reduction between 10% - 35%. Infantes et al. (2012) conducted a study on the same species with a higher canopy density of 600 shoots/m2 and found that the reduction rate was approximately 50%. This study showed similar results when H. uninervis shoot density was taken into account. The results showed that a denser seagrass bed in Suva Lagoon (2,500 shoots/m2) imposed higher wave reduction compared to the less dense seagrass bed (1200 shoots/m2) in Maui Bay (Table 1).
With global warming threatening coral reef health and rising sea levels accelerating coastal erosion and threatening shoreline stability, it is time to pay attention to the coastal protection services provided by natural ecosystems such as seagrass meadows (Guannel et al., 2016). Guannel et al. (2016) and Unsworth et al. (2018) projected that when 90% of coral reef is lost; seagrass meadows will continue to provide important ecosystem services such as coastal protection. But the level of wave height and energy reduction provided by seagrass meadows is dependent on the height of the seagrass canopy relative to the local water level. For situations where the seagrass canopy occupies less of the water column, as is the case expected with increasing sea levels, the effectiveness of seagrass at attenuating waves may be reduced (Barbier et al., 2008). Additionally, storms have been found to impact coastline protection offered by seagrass meadows through defoliation and uprooting of the plants (Guannel et al., 2016; Lebbe et al., 2021). However, a study conducted by James et al. (2021) who investigated tropical Biogeomorphic seagrass landscapes for coastal protection shows that despite losing out on the ability to reduce wave energy, the extensive root system of the meadow still stabilizes coastal sediments thereby preventing erosion.
In the Pacific Islands, the importance of seagrass ecosystems to coastal protection is often overlooked due to the lack of awareness of the importance of seagrass ecosystem services (Pascal et al., 2015; Brodie et al., 2020a; Brodie et al., 2020b). Across Pacific Island countries, a lack of recognition, local capacity, mapping, and explicit policies to protect seagrass ecosystems hinders conservation efforts (McKenzie and Yoshida, 2020). Despite being a preliminary study, the results presented here highlight the importance of seagrass ecosystems in coastal protection and are relevant to other locations in the Pacific.
Conclusions and reccomendations
Seagrass beds of Halodule uninervis were shown to reduce wave height and energy and assist in the gradual release of energy as the wave travels towards the shoreline. It can be cheaper and more sustainable to rely on natural ecosystems to provide resilience to wave-based erosion. By promoting and integrating ecosystem services into adaptation strategies, cost-effective solutions may be developed which obviate the high cost of building and maintaining engineered solutions (Arkema et al., 2017). The coastal protection service provided by the seagrass beds is particularly important with climate change as the projected increase in sea level rise and wave energy continues to cause coastal erosion and flooding. The level of wave energy reduction provided by seagrass meadows is dependent on the height of the seagrass canopy relative to the local water level (Guannel et al., 2016; Lebbe et al., 2021). Therefore healthy seagrass beds should be protected to ensure proper development of the physical characteristics of seagrass (canopy height, width and shoot density) that interact with waves thereby maintaining the effectiveness of seagrass at reducing wave energy.
Based on our results, for PICs, we recommend that: (1) the health, printeractions allow seagrasses to playotection, and conservation of seagrass beds be explicitly included in future coastal restoration or remediation activities, (2) that seagrass beds are more widely acknowledged to provide coastal protection and can contribute to nature-based solutions to climate change resilience, and (3) that future studies should build on the current work to obtain equivalent data under storm or cyclone conditions and that longer-term data collection (preferably a year) is undertaken to capture the seasonal influence of seagrass canopy changes on coastal protection.
Data availability statement
The raw data supporting the conclusions of this article will be made available by the authors, without undue reservation.
Author contributions
LM: Conceptualization, methodology, investigation, and writing. GB: Conceptualization, writing, reviewing and editing. AS: Data analysis, reviewing and editing. JH: Conceptualization, data analysis, reviewing and editing. MW: Conceptualization, data analysis, writing and editing. HD: Conceptualization, data analysis, writing and editing. All authors contributed to the article and approved the submitted version.
Acknowledgments
Our sincere thanks to The University of the South Pacific Research Office for support under their SRT program and the Centre for Environment, Fisheries and Aquaculture Science (Cefas) UK for the top-up scholarship and sensor equipment support (via the Commonwealth Marine Economies Programme - CMEP) which allowed wave data to be collected. The University of the South Pacific (USP) research office also provided the write-up scholarship to put this paper together. Special acknowledgement goes to Mr Jasha Dehm for creating Figure 1. The authors would like to acknowledge the Climate and Ocean Support Programme in the Pacific (COSPPac) for the financial support towards the publication fee.
Conflict of interest
The authors declare that the research was conducted in the absence of any commercial or financial relationships that could be construed as a potential conflict of interest.
Publisher’s note
All claims expressed in this article are solely those of the authors and do not necessarily represent those of their affiliated organizations, or those of the publisher, the editors and the reviewers. Any product that may be evaluated in this article, or claim that may be made by its manufacturer, is not guaranteed or endorsed by the publisher.
References
António M. H. P., Fernandes E. H. L., Muelbert J. H. (2020). Impact of jetty configuration changes on the hydrodynamics of the subtropical patos lagoon estuary, Brazil. Water 12 (11), 3197. doi: 10.3390/w12113197
Arkema K. K., Griffin R., Maldonado S., Silver J., Suckale J., Guerry A. D. (2017). Linking social, ecological, and physical science to advance natural and nature-based protection for coastal communities. Ann. New York Acad. Sci. 1399 (1), 5–26. doi: 10.1111/nyas.13322
Barbier E. B., Koch E. W., Silliman B. R., Hacker S. D., Wolanski E., Primavera J., et al. (2008). Coastal ecosystem-based management with nonlinear ecological functions and values. Science 319 (5861), 321–323. doi: 10.1126/science.1150349
Bedulli C., Lavery P. S., Harvey M., Duarte C. M., Serrano O. (2020). Contribution of seagrass blue carbon toward carbon neutral policies in a touristic and environmentally-friendly island. Front. Mar. Sci. 7, 1. doi: 10.3389/fmars.2020.00001
Bindoff N. L., Cheung W. W. L., Kairo J., Arístegui V. A., Guinder R., Hallberg N., et al. (2019). “Changing ocean, marine ecosystems, and dependent communities,” IPCC special report on the ocean and cryosphere in a changing climateThe Ocean and Cryosphere in a Changing Climate.. Eds. Pörtner H.-O., Roberts D. C., Masson-Delmotte V., Zhai P., Tignor M., Poloczanska E., Mintenbeck K., Alegría A., Nicolai M., Okem A., Petzold J., Rama B., Weyer N. M. (Cambridge, UK and New York, NY, USA: Cambridge University Press), 447–587. doi: 10.1017/9781009157964.007
Bongarts Lebbe T., Rey-Valette H., Chaumillon É., Camus G., Almar R., Cazenave A., et al. (2021). Designing coastal adaptation strategies to tackle sea level rise. Front. Mar. Sci. 8, 740602. doi: 10.3389/fmars.2021.740602
Bosserelle C., Kruger J., Movono M., Reddy S. (2015b). Wave inundation on the coral coast of fiji. australas. Coasts Ports Conf. 2015, 96–101.
Bosserelle C., Reddy S., Lal D. (2015a). WACOP wave climate reports (Fiji, Fiji Wave Buoy: Secretariat of the Pacific Community). Available at: http://gsd.spc.int/wacop/.
Bradley K., Houser C. (2009). Relative velocity of seagrass blades: implications for wave reduction in low-energy environments. J. Geophysical Research: Earth Surface 114 (F1). doi: 10.1029/2007JF000951
Brander R. W., Kench P. S., Hart D. (2004). Spatial and temporal variations in wave characteristics across a reef platform, warraber island, Torres strait, Australia. Mar. Geology 207 (1–4), 169–184. doi: 10.1016/j.margeo.2004.03.014
Brodie G., Brodie J., Maata M., Peter M., Otiawa T., Devlin M. J. (2020a). Seagrass habitat in Tarawa lagoon, Kiribati: service benefits and links to national priority issues. Mar. Pollut. Bull. 155, 111099. doi: 10.1016/j.marpolbul.2020.111099
Brodie G., Holland E., N’Yeurt A., Soapi K., Hills J. (2020b). Seagrasses and seagrass habitats in pacific small island developing states: potential loss of benefits via human disturbance and climate change. Mar. Pollut. Bull. 160, 111573. doi: 10.1016/j.marpolbul.2020.111573
Chand N. (2019). Mapping the spatial distribution of seagrass in Suva lagoon using remote sensing and field studies (School of Marine Sciences, University of the South Pacific). Available at: https://librarycat.usp.ac.fj/client/en_GB/search/asset/5539/0.
Cullen-Unsworth L. C., Nordlund L. M., Paddock J., Baker S., McKenzie L. J., Unsworth R. K. F. (2014). Seagrass meadows globally as a coupled social–ecological system: implications for human wellbeing. Mar. Pollut. Bull. 83 (2), 387–397. doi: 10.1016/j.marpolbul.2013.06.001
Denny M. (1988). Biology and the mechanics of the wave-swept environment (Princeton University Press). Available at: http://www.jstor.org/stable/j.ctt7ztnb7.
Duarte C. M., Kirkman H. (2001). Methods for the measurement of seagrass abundance and depth distribution (Elsevier eBooks), 141–153. doi: 10.1016/b978-044450891-1/50008-6
Elgar S., Holthuijsen L. H. (2007). Review of waves in oceanic and coastal waters, HolthuijsenLeo h. Oceanography 20 (3), 133–135. doi: 10.5670/oceanog.2007.42
Fonseca M. S., Cahalan J. A. (1992). A preliminary evaluation of wave reduction by four species of seagrass. Estuarine Coast. Shelf Sci. 35 (6), 565–576. doi: 10.1016/S0272-7714(05)80039-3
Fonseca M. S., Fisher J. S., Zieman J. C., Thayer G. W. (1982). Influence of the seagrass, zostera marina l., on current flow. Estuarine Coast. Shelf Sci. 15 (4), 351–364. doi: 10.1016/0272-7714(82)90046-4
Fonseca M. S., Koehl M. A. R. (2006). Flow in seagrass canopies: the influence of patch width. Estuarine Coast. Shelf Sci. 67 (1), 1–9. doi: 10.1016/j.ecss.2005.09.018
Gattuso J.-P., Magnan A. K., Bopp L., Cheung W. W. L., Duarte C. M., Hinkel J., et al. (2018). Ocean solutions to address climate change and its effects on marine ecosystems. Front. Mar. Sci. 5. doi: 10.3389/fmars.2018.00337
Guannel G., Arkema K., Ruggiero P., Verutes G. (2016). The power of three: coral reefs, seagrasses and mangroves protect coastal regions and increase their resilience. PloS One 11 (7), e0158094. doi: 10.1371/journal.pone.0158094
Hinkel J., Lincke D., Vafeidis A. T., Perrette M., Nicholls R. J., Tol R. S. J., et al. (2014). Coastal flood damage and adaptation costs under 21st century sea-level rise. Proc. Natl. Acad. Sci. 111 (9), 3292–3297. doi: 10.1073/pnas.1222469111
Hinkel J., Nicholls R. J., Tol R. S. J., Wang Z. B., Hamilton J. M., Boot G., et al. (2013). A global analysis of erosion of sandy beaches and sea-level rise: an application of DIVA. Global Planetary Change 111, 150–158. doi: 10.1016/j.gloplacha.2013.09.002
Infantes E., Orfila A., Simarro G., Terrados J., Luhar M., Nepf H. (2012). Effect of a seagrass (Posidonia oceanica) meadow on wave propagation. Mar. Ecol. Prog. Ser. 456, 63–72. doi: 10.3354/meps09754
James R. K., Lynch A., Herman P. M. J., van Katwijk M. M., van Tussenbroek B. I., Dijkstra H. A., et al. (2021). Tropical biogeomorphic seagrass landscapes for coastal protection: persistence and wave reduction during major storms events. Ecosystems. 24 (2), 301–318. doi: 10.1007/s10021-020-00519-2
John B. M., Shirlal K. G., Rao S. (2015). Effect of artificial sea grass on wave reduction-an experimental investigation. Aquat. Proc. 4, 221–226. doi: 10.1016/j.aqpro.2015.02.030
John B. M., Shirlal K. G., Rao S., Rajasekaran C. (2016). Effect of artificial seagrass on wave reduction and wave run-up. Intern. J. Ocean Clim. Sys. 7 (1), 14–19. doi: 10.1177/1759313115623163
Koftis T. K., Prinos P. (2011). Spectral wave reduction over’Posidonia oceanica’. 935. IAHR. Available at: https://www.researchgate.net/publication/257251751_Spectral_Wave_Attenuation_over_Posidonia_Oceanica#fullTextFileContent.
Lamb J. B., Van De Water J. A., Bourne D. G., Altier C., Hein M. Y., Fiorenza E. A., et al. (2017). Seagrass ecosystems reduce exposure to bacterial pathogens of humans, fishes, and invertebrates. Science 355 (6326), 731–733. doi: 10.1126/science.aal1956
Lecocq F., Winkler H., Daka J. P., Fu S., Gerber J. S., Kartha S., et al. (2022). “Mitigation and development pathways in the near- to mid-term,” in IPCC 2022: climate change 2022: mitigation of climate change. contribution of working group III to the sixth assessment report of the intergovernmental panel on climate change. Eds. Shukla P. R., Skea J., Slade R., Khourdajie A., Diemen R.v., McCollum D., Pathak M., Some S., Vyas P., Fradera R., Belkacemi M., Hasija A., Lisboa G., Luz S., Malley J. (Cambridge, UK and New York, NY, USA: Cambridge University Press). doi: 10.1017/9781009157926.006
Lowe R. J., Falter J. L., Koseff J. R., Monismith S. G., Atkinson M. J. (2007). Spectral wave flow reduction within submerged canopies: implications for wave energy dissipation. J. Geophysical Research: Oceans 112 (C5). doi: 10.1029/2006JC003605
Luhar M., Coutu S., Infantes E., Fox S., Nepf H. (2010). Wave-induced velocities inside a model seagrass bed. J. Geophysical Research: Oceans 115 (C12). doi: 10.1029/2010JC006345
Ma G., Kirby J. T., Su S.-F., Figlus J., Shi F. (2013). Numerical study of turbulence and wave damping induced by vegetation canopies. Coast. Eng. 80, 68–78. doi: 10.1016/j.coastaleng.2013.05.007
Macreadie P. I., Costa M. D., Atwood T. B., Friess D. A., Kelleway J. J., Kennedy H., et al. (2021). Blue carbon as a natural climate solution. Nat. Rev. Earth Environ. 2 (12), 826–839. doi: 10.1038/s43017-021-00224-1
Manca E. (2010). Effects of posidonia oceanica seagrass on nearshore waves and wave-induced flows (Phd, University of Southampton). Available at: https://eprints.soton.ac.uk/195257/.
Manca E., Cáceres I., Alsina J., Stratigaki V., Townend I., Amos C. (2012). Wave energy and wave-induced flow reduction by full-scale model posidonia oceanica seagrass. Continental Shelf Res. 50, 100–116. doi: 10.1016/j.csr.2012.10.008
McInnes K. L., Walsh K. J. E., Hoeke R. K., O’Grady J. G., Colberg F., Hubbert G. D. (2014). Quantifying storm tide risk in Fiji due to climate variability and change. Global Planetary Change 116, 115–129. doi: 10.1016/j.gloplacha.2014.02.004
McKenzie L. J., Yoshida R. L. (2020). Over a decade monitoring fiji’s seagrass condition demonstrates resilience to anthropogenic pressures and extreme climate events. Mar. Pollut. Bull. 160, 111636. doi: 10.1016/j.marpolbul.2020.111636
McKenzie L. J., Yoshida R. L., Aini J. W., Andréfouet S., Colin P. L., Cullen-Unsworth L. C., et al. (2021). Seagrass ecosystems of the pacific island countries and territories: a global bright spot. Mar. Pollut. Bull. 167 (2021), 112308. doi: 10.1016/j.marpolbul.2021.112308
Neumann B., Vafeidis A. T., Zimmermann J., Nicholls R. J. (2015). Future coastal population growth and exposure to Sea-level rise and coastal flooding–a global assessment. PloS One 10 (3), e0118571. doi: 10.1371/journal.pone.0118571
Nordlund L. M., Jackson E. L., Nakaoka M., Samper-Villarreal J., Beca-Carretero P., Creed J. C. (2018). Seagrass ecosystem services – what’s next? Mar. Pollut. Bull. 134, 145–151. doi: 10.1016/j.marpolbul.2017.09.014
Nordlund L. M., Koch E. W., Barbier E. B., Creed J. C. (2016). Seagrass ecosystem services and their variability across genera and geographical regions. PLOS ONE 11 (10), e0163091. doi: 10.1371/journal.pone.0163091
Ondiviela B., Losada I. J., Lara J. L., Maza M., Galván C., Bouma T. J., et al. (2014). The role of seagrasses in coastal protection in a changing climate. Coast. Eng. 87, 158–168. doi: 10.1016/j.coastaleng.2013.11.005
Oprandi A., Mucerino L., De Leo F., Bianchi C. N., Morri C., Azzola A., et al. (2020). Effects of a severe storm on seagrass meadows. Sci. Total Environ. 748, 141373. doi: 10.1016/j.scitotenv.2020.141373
Pascal N., Leport G., Molisa V., Wendt H., Brander L., Fernandes L., et al. (2015). National marine ecosystem service valuation: Vanuatu (Suva, Fiji: MACBIO (GIZ/IUCN/SPREP), 79.
Prinos P., Stratigaki V., Manca E., Losada I., López Lara J., Sclavo M., et al. (2010). Wave propagation over posidonia oceanica: Large scale experiments. (hydralab+) 57–60. Available at: https://www.researchgate.net/publication/220013920_Wave_propagation_over_posidonia_oceanica_large_scale_experiments#fullTextFileContent.
Quataert E., Storlazzi C., Rooijen A.v., Cheriton O., Dongeren A.v. (2015). The influence of coral reefs and climate change on wave-driven flooding of tropical coastlines. Geophysical Res. Lett. 42 (15), 6407–6415. doi: 10.1002/2015GL064861
Reidenbach M. A., Thomas E. L. (2018). Influence of the seagrass, zostera marina, on wave attenuation and bed shear stress within a shallow coastal bay. Front. Mar. Sci. 5, 397. doi: 10.3389/fmars.2018.00397
Serrano Ureña A. M. (2016). Wave energy dissipation due to posidonia oceanica in the Mediterranean Sea. TUDelft. Available at: http://resolver.tudelft.nl/uuid:76b4a299-3dff-48a2-aa1c-64b6dd4c4712.
Serrano O., Gómez-López D. I., Sánchez-Valencia L., Acosta-Chaparro A., Navas-Camacho R., González-Corredor J., et al. (2021). Seagrass blue carbon stocks and sequestration rates in the colombian caribbean. Sci. Rep. 11 (1), 11067. doi: 10.1038/s41598-021-90544-5
Short F., Carruthers T., Dennison W., Waycott M. (2007). Global seagrass distribution and diversity: a bioregional model. J. Exp. Mar. Biol. Ecol. 350 (1), 3–20. doi: 10.1016/j.jembe.2007.06.012
Singh A. M., Aung T. H. (2008). Salinity, temperature and turbidity structure in the Suva lagoon, Fiji. Am. J. Environ. Sci. 4 (4), 266–275. doi: 10.3844/ajessp.2008.266.275
Singh A. A., Maharaj A., Kumar M., Singh P., Singh S., Muller-Karger F. E., et al. (2019). Developing high resolution baseline coast resource maps using world view 2 imagery for a coastal village in Fiji. Front. Mar. Sci. 6. doi: 10.3389/fmars.2019.00207
Storlazzi C. D., Gingerich S. B., Dongeren A., Cheriton O. M., Swarzenski P. W., Quataert E., et al. (2018). Most atolls will be uninhabitable by the mid-21st century because of sea-level rise exacerbating wave-driven flooding. Sci. Adv. 4 (4), eaap9741. doi: 10.1126/sciadv.aap9741
Stratigaki V., Manca E., Prinos P., Losada I. J., Lara J. L., Sclavo M., et al. (2011). Large-Scale experiments on wave propagation over posidonia oceanica. J. Hydraulic Res. 49 (sup1), 31–43. doi: 10.1080/00221686.2011.583388
Unsworth R. K. F., McKenzie L. J., Nordlund L. M., Cullen-Unsworth L. C. (2018). A changing climate for seagrass conservation? Curr. Biol. 28 (21), R1229–R1232. doi: 10.1016/j.cub.2018.09.027
Van Rooijen A., Lowe R. J., Ghisalberti M., Hansen J., McCall R. T., van Dongeren A. R. (2016a). Physical and numerical modelling of wave transformation through a coastal canopy. (20th Australasian Fluid Mechanics Conference) 5. Available at: https://www.researchgate.net/publication/312126229_Physical_and_Numerical_Modelling_of_Wave_Transformation_through_a_Coastal_Canopy.
Van Rooijen A., McCall R. T., Vries J.S.M.v., Dongeren A.R.v., Reniers A. J. H. M., Roelvink J. A. (2016b). Modeling the effect of wave-vegetation interaction on wave setup. J. Geophysical Research: Oceans 121 (6), 4341–4359. doi: 10.1002/2015JC011392
Van Rooijen A., Van Thiel de Vries J., McCall R., Van Dongeren A., Roelvink J., Reniers A. (2015). “Modeling of wave reduction by vegetation with XBeach,” in E-proceedings of the 36th IAHR World Congress. (The Hague, the Netherlands: IAHR). Available at: http://resolver.tudelft.nl/uuid:98a66b95-8fed-421a-9bfe-4ae978375dbe.
Vitousek S., Barnard P. L., Fletcher C. H., Frazer N., Erikson L., Storlazzi C. D. (2017). Doubling of coastal flooding frequency within decades due to sea-level rise. Sci. Rep. 7 (1), 1399. doi: 10.1038/s41598-017-01362-7
Vonk J. A., Christianen M. J. A., Stapel J. (2008). Redefining the trophic importance of seagrasses for fauna in tropical indo-pacific meadows. Estuarine Coast. Shelf Sci. 79 (4), 653–660. doi: 10.1016/j.ecss.2008.06.002
Vousdoukas M. I., Mentaschi L., Voukouvalas E., Verlaan M., Jevrejeva S., Jackson L. P., et al. (2018). Global probabilistic projections of extreme sea levels show intensification of coastal flood hazard. Nat. Commun. 9 (1), 1–12. doi: 10.1038/s41467-018-04692-w
Wandres M., Aucan J., Espejo A., Jackson N., De Ramon N’Yeurt A., Damlamian H. (2020). Distant-source swells cause coastal inundation on fiji’s coral coast. Front. Mar. Sci. 7. doi: 10.3389/fmars.2020.00546
Waycott M., McKenzie L., Mellors J. E., Ellison J. C., Sheaves M. T., Collier C., et al. (2011). “Vulnerability of mangroves, seagrasses and intertidal flats in the tropical pacific to climate change,” in Vulnerability of tropical pacific fisheries and aquaculture to climate change. Eds. Bell J. D., Johnson J. E., Hobday A. J. (). Secretariat of the Pacific Community), 297–368. Available at: http://www.spc.int/climate-change/fisheries/assessment/.
Woodruff J. D., Irish J. L., Camargo S. J. (2013). Coastal flooding by tropical cyclones and sea-level rise. Nature 504 (7478), 44–52. doi: 10.1038/nature12855
Keywords: seagrass, coastal erosion, wave reduction, Halodule uninervis, nature-based protection
Citation: Muna LR, Brodie G, Singh A, Hills J, Wandres M and Damlamian H (2023) Understanding ecosystem services for climate change resilience in coastal environments: a case study of low-canopy sub-tidal seagrass beds in Fiji. Front. Mar. Sci. 10:1184568. doi: 10.3389/fmars.2023.1184568
Received: 12 March 2023; Accepted: 12 June 2023;
Published: 27 June 2023.
Edited by:
Ibon Galparsoro, Technological Center Expert in Marine and Food Innovation (AZTI), SpainReviewed by:
Lillian Aoki, Cornell University, United StatesKathleen Sullivan Sealey, University of Miami, United states
Copyright © 2023 Muna, Brodie, Singh, Hills, Wandres and Damlamian. This is an open-access article distributed under the terms of the Creative Commons Attribution License (CC BY). The use, distribution or reproduction in other forums is permitted, provided the original author(s) and the copyright owner(s) are credited and that the original publication in this journal is cited, in accordance with accepted academic practice. No use, distribution or reproduction is permitted which does not comply with these terms.
*Correspondence: Lency Royce Muna, bGVuY3ltdW5hNzk3QGdtYWlsLmNvbQ==
†ORCID: Lency Royce Muna, orcid.org/0000-0003-1223-2231
Giliane Brodie, orcid.org/0000-0002-6896-4696
Awnesh Singh, orcid.org/0000-0002-5341-0703
Jeremy Hills, orcid.org/0000-0002-9204-2536
Moritz Wandres, orcid.org/0000-0002-3063-4448
Herve Damlamian, orcid.org/0000-0002-2794-8809