- 1Department of Ecology, Fiskaaling, Hvalvík, Faroe Islands
- 2Section for Aquaculture, The North Sea Research Centre, DTU Aqua, Technical University of Denmark, Hirtshals, Denmark
- 3Section for Coastal Ecology, Danish Shellfish Centre, DTU Aqua, Technical University of Denmark, Nykøbing Mors, Denmark
- 4Blue Economy Research, The Scottish Association for Marine Science (SAMS), Oban, United Kingdom
Commercial salmonid farming is typically performed in open-water net cages where interactions between the environment and production unit might be widespread and not easily predicted or controlled. Integrated multi-trophic aquaculture (IMTA) has been suggested to mitigate some of the environmental impacts. Based on empirical data, the assimilation of particulate waste from a commercial fish farm was modeled by two approaches to salmon/blue mussel IMTA: a system with mussels at the surface next to the fish farm and an alternative setup with mussels submerged under the farm. According to the model, 15% of the feed was defecated and assumed available to the mussels and the submerged mussel farm could assimilate 14.6% of the feces. Sensitivity analysis showed that the current speed, the mussel filtration rate, and the proportion of the material that settles slowly had an impact on this assimilation estimate, which could be significantly higher. However, the model did not include assimilation limitations due to particle size, which may contribute significantly to the submerged farm. The mussel farm at the surface mainly received small slow settling particles, and according to the model, only 0.4% of the fish farm waste was assimilated. The maximum obtainable assimilation was 5.5%. The fraction of slowly settling waste had the most pronounced influence on waste assimilation in the mussel farm at the surface. This is also among the most uncertain parameters, since the relative portion of different settling velocities of fish feces is highly variable, and more information on the size distribution of waste is needed.
1 Introduction
The release of nutrients and organic material to the environment from salmon farms is associated with various environmental concerns, especially in coastal areas with intensive aquaculture production in net cages with free water exchange (Naylor et al., 2000; Beveridge, 2004).
Integrated multi-tropic aquaculture (IMTA) has been promoted as a sustainable and practical alternative to monoculture that could help solve some ecological issues with intensified aquaculture in cage systems (Chopin et al., 2012; Custódio et al., 2020). IMTA involves the cocultivation of trophically linked species. The lower trophic -level species feed on the waste from the upper trophic -level species, treating waste as a valuable resource utilized through natural synergistic interactions between the cultured species (Chopin et al., 2012; Kerrigan and Suckling, 2018).
Thus, the environmental impact is mitigated while economic value is added to the farmer in secondary marketable products, reduced risk through product diversification, and potential allocation for increased production due to positive mitigation effects (Barrington et al., 2009).
Blue mussels (Mytilus edulis) have been promoted for extracting particulate waste (PW) in IMTA systems (Barrington et al., 2009). Some studies find increased growth of mussels in proximity to fish farms (Kerrigan and Suckling, 2018), and especially during winter when other suspended material is scarce, effluents may contribute considerably to the blue mussel diet (Handå et al., 2012).
However, it is debated if significant mitigation can be obtained by direct assimilation of fish farm waste particles (Sanz-Lazaro and Sanchez-Jerez, 2017), and most studies that model the expected mitigation effect by blue mussels in the vicinity of fish farms find it to be minimal (Troell and Norberg, 1998; Cranford et al., 2013; Filgueira et al., 2017).
For open water systems, spatial arrangement of the IMTA system and local environmental factors are highly influential on the performance of IMTA, and there is a need to assess the potential mitigation within the local environmental and spatial constraints (Kerrigan and Suckling, 2018; Zhang et al., 2019; Reid et al., 2020; Zhou et al., 2022). For PW assimilation by blue mussels, the mitigation effect depends on the dispersion of the PW from the fish farm to the mussel farm and the exposure time to the waste, which depend on currents and settling velocity of the waste particles (Reid et al., 2020).
Likewise, ambient environmental conditions substantially influence mussel filtration rates, such as the concentration of natural seston (Riisgård et al., 2003), quality of seston (Ward and Shumway, 2004), and differential clearance rates relative to mussel size (Jacobs et al., 2015).
As vertical transport dominates the PW distribution around fish farms, it has been suggested that higher mitigation could be achieved by locating the extractive species below fish farms (Filgueira et al., 2017), and studies have explored IMTA with extractive species at the seabed (Cubillo et al., 2016; Jansen et al., 2019; Nederlof et al., 2020). However, the nature of fish farming implies that the living conditions at the seabed vary considerably during the course of a production cycle, especially in sheltered areas (á Norði et al., 2011). An alternative approach to extract particles in the vertical waste stream is to suspend the extractive species below the fish farm; a mitigation potential that has received little attention.
Blue mussels as extractive species are also considered on a regional level through a budgetary approach targeting microalga to remove nutrients and organic matter (OM) from the ecosystem instead of direct assimilation of PW from the farm. This allows evaluation of connectivity between the different functional groups at a larger scale than at farm level (Nederlof et al., 2022).
To support the decision basis in the future implementation of blue mussels to mitigate the impact of commercial fish farms, this study models the assimilation of PW by blue mussels at an operational fish farm. The waste production was modeled based on feed data, analysis of the commercial feed, and the spatial arrangement of the net pens (Figure 1B). Dispersion was modeled according the local hydrodynamics. The model does not take into account limitations in waste assimilation by blue mussels due to particle size limitations or reduced filtration rates due to saturation or selective feeding and thus represents the maximum achievable mitigation.
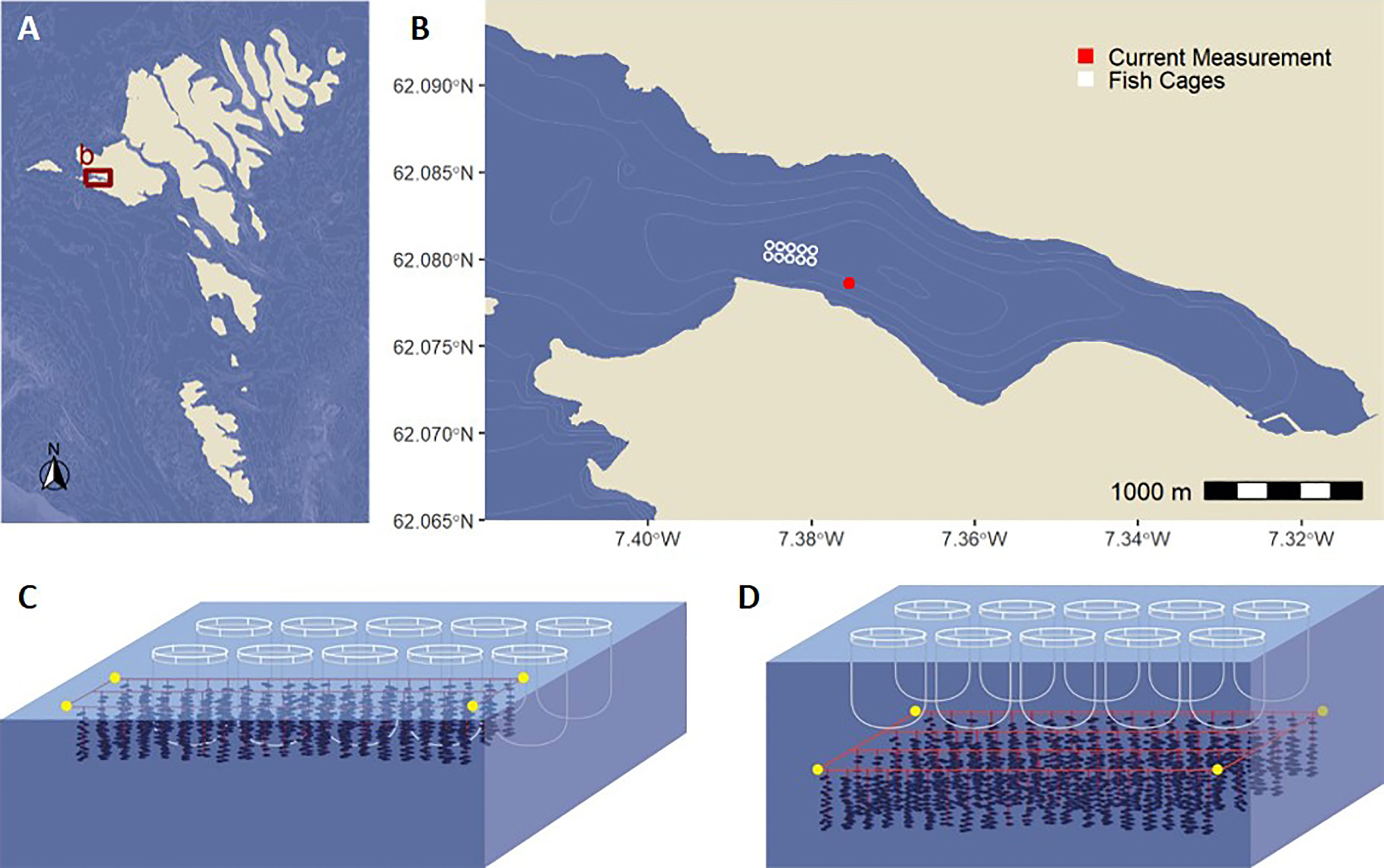
Figure 1 Map of the Faroe Islands (A) and the location of the studied fjord. (B) The study site showing the location of the fish farm and the current measurement. Conceptual drawings of the modeled spatial locations of the Atlantic salmon farm and the blue mussel farm, with blue mussels at the surface next to the fish farm (C) and submerged below the fish farm (D).
Assimilation of PW by blue mussels was modeled according to two spatial blue mussel/salmon farm configurations: the approach with blue mussels at the surface at the long side of the fish farm (Figure 1C) and an alternative approach with the blue mussel farm submerged directly below the net cages (Figure 1D). As the fish farm was the primary economic interest for the company, the modeled blue mussel farms were low-maintenance farms with the primary purpose of mitigating the environmental impact.
The general design of the fish farm is widely used in salmon farming and to investigate if the mitigation potential would be higher at other farms with different hydrodynamic settings, a sensitivity analysis was conducted on the current speed. A sensitivity analysis was likewise performed on the density of blue mussels, as it is variable on passive spat collectors. Size and settling velocities of fish fecal particles are highly variable and depend on feed ingredients, fish size, and hydrodynamics (Reid et al., 2009); and in general, the information on the size fractionations of waste particles is scarce (Nederlof et al., 2022). Thus, a sensitivity analysis was also conducted to investigate how the fraction of slowly settling particles influenced the mitigation performance of the blue mussel farms.
2 Materials and methods
2.1 Study site
The salmon sea cage farm was located in a fjord (Sørvágsfjørður) on the westernmost main island on the Faroe Islands. The fjord is 5 km long and less than 1.5 km wide, and the maximum depth is 55 m (Figures 1A, B). The seawater circulation in the fjord is influenced by tidal forces, with semidiurnal water level changes up to 2 m at spring tide and estuarine circulation driven by water runoff from the 31 km2 catchment area, most of which is uninhabited and uncultivated (Diedericks and á Norði, 2021).
In general, the annual seawater temperature in Faroese fjords ranges from ~6°C to ~11.5°C (á Norði et al., 2011) and vertical stratification is weak, with vertical temperature differences less than 3°C (ICES, 2023). Wind forces easily break down the stratification and nutrients are readily mixed into the euphotic zone. Thus, the primary production in Faroese fjords is high during the entire summer with an annual production of ~330 gC m-2 year-1 (Gaard et al., 2011).
Blue mussels are endemic in the investigated fjord, and mussel larvae are present in the upper water masses at densities up to 4,000 ind. m-3 from May to August (Danielsen and á Norði, 2021). Preliminary investigations with mussels grown directly on spat collectors at 0–15 m depth showed an average blue mussel abundance of 600 mussels per meter after the second summer in water (Danielsen and á Norði, 2021).
2.2 Production data and release of wastes
The discharge of waste in the form of OM, nitrogen (N), and phosphorus (P) from the fish farm was calculated as mass balances for a complete production cycle. Production data for the farming cycle from January 2018 to June 2019 were provided by the fish farming company operating in Sørvágsfjørður. The data consisted of net cage locations, feed types, daily feed use, fish count, and fish biomass. In the Faroe Islands, there is a trend toward larger smolt at deployment, thus reducing the duration of the farming cycles at sea (ICES, 2023). In the investigated farming cycle, the average smolt weight was 360 g at deployment, and the average weight at harvest was 6.7 kg. The biological feed conversion ratio was 1.05.
The commercial fish feed used (FAE Premium 1200 and 2500, Skretting) during the production cycle was analyzed for proximate composition (Table 1). Nitrogen-free extract (NFE) was calculated as dry matter (DM) minus the sum of crude protein, crude lipid, and ash. The formulated diets were homogenized using a Krups Speedy Pro homogenizer and analyzed for DM and ash (Kolar, 1992), crude protein (ISO, 2005) (crude protein = Kjeldahl N × 6.25), crude lipid (Bligh and Dyer, 1959), and total P (ISO, 1998). DM was determined as the weight difference after drying pellets at 105°C for 24 h. Ash was determined after combustion at 550°C for 24 h. The OM content was determined as the feed dry weight minus the ash content.
In the mass balance, the feed was either ingested or lost to the environment. Ingested feed was digested or defecated. Digested matter was incorporated in the biomass or used for metabolism and excreted as dissolved and thus unavailable to the mussels. Variables for the calculations of waste production at the fish farm are listed in Table 2.
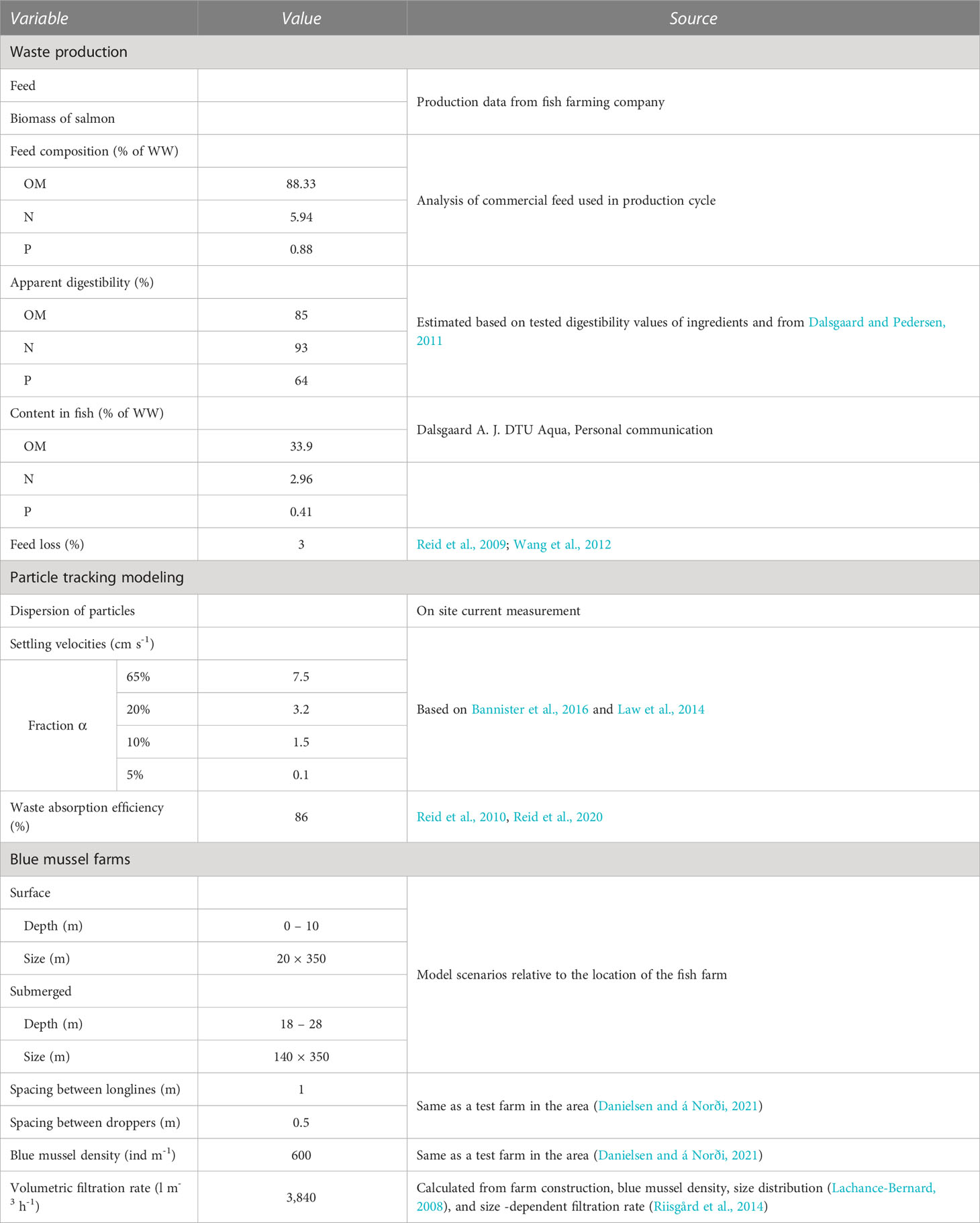
Table 2 Variables used in the calculations of waste production at the fish farm, the dispersion of waste from the fish farm, and the filtration rate at the blue mussel farm.
It is estimated that feed loss is generally below 5% for salmon reared in net pens (Cromey et al., 2002). In this study, the feed loss was estimated to be 3% (Reid et al., 2009; Wang et al., 2012) based on the automatic feeding system monitored by camera and the low feed conversion ratio at the farm.
Energy expenditure depends on the size of fish, species, and temperature, which can vary even within species (Pettersen et al., 2018). In this study, the apparent digestibility coefficients (ADCs) of OM, N, and P was estimated based on tested digestibility values of ingredients and on Dalsgaard and Pedersen (2011), who investigated the production of suspended and solid waste from rainbow trout when fed commercial diets and feces collected every 24 h for 10 days (Table 2). Since leaching of dissolved material typically occurs shortly after defecation (Shomorin et al., 2019), the presented ADC represents the particle portion of the feces after leaching. The ADC in various feed trials on Atlantic salmon is variable and generally similar to or lower than the used digestibility values (Storebakken et al., 1998; Glencross et al., 2004; Kraugerud et al., 2007; Øverland et al., 2009).
The amount of digested material incorporated into the fish biomass was calculated from the wet weight and proximate composition of large rainbow trout obtained from sea cages in Denmark (Dalsgaard A.J, DTU Aqua, personal communication), which is similar to the measured composition of Atlantic salmon (Wang et al., 2013).
It was assumed that fecal particles were the only PW available for the blue mussels. Uneaten feed pellets were assumed unavailable due to their large size and high settling velocity (Cromey et al., 2002; Filgueira et al., 2017).
2.3 Blue mussel farms
Two configurations of salmon/blue mussel IMTA were modeled: a scenario with blue mussels next to the fish cages (Chopin et al., 2012) at 0 – 10 m depth and a more theoretical system with mussels submerged below the cages at 18 – 28 m depth (Figures 1C, D). The mussel farm at the surface was placed at the long side of the farm next to the grid holding the fish cages where most waste particles were available according to initial modeling of particle dispersion by the currents. The dimensions of the modeled blue mussel farms are listed in Table 2.
Since the primary purpose of the mussel farm was to mitigate the impact of fish farming and the long lines were positioned below the fish cages in one of the scenarios, it was assumed that the blue mussels were grown directly on the collectors, which is a method often used in mitigation practices (Petersen et al., 2019).
The mussels alter the vertical particle transport by consuming suspended particles and subsequent production of fecal pellets and possible pseudofeces that exhibit higher settling velocities (McKindsey et al., 2011). Thus, the capture of phytoplankton and zooplankton should be considered when investigating the alteration of particle transport in the vicinity of fish farms to accommodate ambient food supply. However, as this study investigates the potential removal of fish farm waste by the mussels, increased deposition of OM adjacent to the fish farm in the form of mussel feces from phytoplankton and zooplankton capture was not modeled.
2.4 Particle tracking model
Currents were measured close to the farm with a bottom-mounted acoustic Doppler current profiler (ADCP) current meter, Teledyne RDI Workhorse Sentinel 300 kHz (Figure 1B). The bottom depth was 52 m, and vertical current profiles were measured every 5 min in 4 m bins from 7 February to 1 May 2017. The dispersion of PW was modeled according to the Lagrangian particle-tracking module in Cromey et al. (2002). The module assumes uniform currents in the horizontal plane. The model was forced with the time series of the observed currents, with linear interpolation through time and vertically between ADCP bins, i.e., depth.
Particles were released at 2 min intervals, a time step of 0.01 s was used, and the position of the particles was recorded in vertical layers of 1 cm.
Ten identical cylindrical salmon cages with a circumference of 160 m were placed in two rows of five cages distanced 70 m apart center to center. This was the general setup at the inner part of the commercial fish farm (Figure 1), and the cages were cylindrical down to 10 m depth whereafter they cone down to a point at 18 m. The swimming depth of caged salmon varies in response to environmental cues and feeding (Oppedal et al., 2011). This model released PW uniformly from the cages at 5 m depth for the entire modeling period based on an estimated average swimming depth. The cages were gridded to 4 × 4 m2, resulting in about 100 release points per cage.
In dispersion models, the settling velocity of feces is often fixed to a mean value of 3.2 cm s-1 (Cromey et al., 2002; Filgueira et al., 2017). However, the size and settling velocity of the individual fecal particles vary greatly (Chen et al., 2003; Law et al., 2014; Bannister et al., 2016), which has implications for their availability for the mussels.
Four settling velocities were modeled where 65% of the PW settled at 7.5 cm s-1, 20% at 3.2 cm s-1, 10% at 1.5 cm s-1 based on Bannister et al. (2016), and to include PW smaller than 500 µm, 5% of the fecal particles were assumed to settle slowly at 0.1 cm s-1 (Law et al., 2014), see Table 2.
Separate particle tracking models are run for each of the particle settling velocities. The results from each modeled particle track thus represent a random sample of the distribution of all particle tracks when released from the cages at a depth of 5 m. This resulting sample set of approximately 60 million particles is assumed to be representative of the distribution of that settling velocity.
An approximated distribution of the duration a particle is in a mussel farm can then be found from this sample.
As the particle settling velocity is constant throughout the entire modeling period, the duration a particle is in the mussel farm can instead be described as the vertical distance traveled in the mussel farm.
The distribution of vertical distances traveled has a natural resolution of 1 cm as is given by the model parameters and is approximated by:
where describes the vertical distance in cm traveled in the mussel farm, is the probability that a particle of settling velocity is in the mussel farm for a vertical distance of cm, represents the number of modeled particles with settling velocity that are observed times inside the mussel farm, and is the total number of modeled particles with settling velocity .
2.5 Assimilation of fish waste by blue mussels
The results from the particle tracing models were used to calculate the fraction of fish waste assimilated by the blue mussels. The potential production of pseudofeces was not included in the model, and thus the ingestion of the individually modeled waste particles by the blue mussels () can be expressed as:
where is the volumetric filtration rate of the entire mussel population per cubic meter blue mussel farm, and is the mass of the individually modeled fish waste particle.
When modeling the assimilation of fish waste by the blue mussels, uniform mussel distribution within the mussel farm is assumed, and ingestion of particles affects the particle availability deeper in the mussel farm. The change in mass of the modeled particles due to ingestion by the mussels during the total time the waste particles are in the mussel farm () can be expressed as:
where is the mass of the modeled particle at time 0.
When taking into account the constant settling velocity () of the individual particle, can be expressed as a function of the vertical distance the particles have covered in the mussel farm (), and equation 4 can be expressed as:
In the dispersion model, the horizontal position of the particle is logged in predetermined vertical positions of 10 mm as previously discussed. Thereby, the final equation for the fraction of fish waste assimilated by the blue mussels during passage through the farm can be expressed as:
where i describes the vertical distance in cm traveled in the mussel farm, is the probability that a particle of settling velocity is in the mussel farm for a vertical distance of cm, M is the maximum possible number that i can obtain, αv is the fraction of PW with the settling velocity v (Table 2), and z(i) is the vertical distance covered in the farm converted to m.
The blue mussel absorption efficiency (AE) of fish waste was 86% (Reid et al., 2020).
2.6 Sensitivity analysis
The absorption of fish farm waste by blue mussels depends on the site-specific characteristics and the setup of farms that may vary considerably between locations (Reid et al., 2020). To investigate how some of these variations affect the performance of IMTA farms, sensitivity analyses were conducted on the current speed, the filtration capacity of the blue mussel farm, and the portion of waste released as slowly settling particles.
For the current speed sensitivity analysis, the vertical changes in speed were kept. Model runs were conducted with reduced or increased currents by multiplying by factors from 0.1 to 4 to the measured current speed. This resulted in average currents of up to 34 cm s-1 in the upper 10 m of the water column and up to 20 cm s-1 in the area where the submerged blue mussel farm was modeled.
Blue mussel densities at commercial farms vary greatly depending on the distance between lines and mussel densities on individual lines. The sensitivity analysis tested volumetric filtration rates (FRV) from 750 l h-1 m-3 to 16,000 l h-1 m-3. For simplicity, alterations were only assumed in the blue mussel density on the long lines, while the general setup of the farm was fixed. However, the range in filtration rates covers various combinations of farm arrangement and blue mussel density.
The lower limit was set from general observations at commercial Danish blue mussel farms where the blue mussel density typically is approximately 80 ind. m-1 when 5 cm large blue mussels are harvested (Daniel Taylor, DTU Aqua, personal communication). The upper limit is based on empirical observations of the weight density relation at blue mussel farms for mitigation purposes. The blue mussels are not restocked, and hence self-thinning occurs as they grow. The observations are from farms in inner Danish waters, which have a very high settlement.
Mussel density can be described by the power function (Holbach et al., 2020):
where N is the number of blue mussels per meter longline, and W is the tissue dry weight.
From the relation between size, filtration rate (Riisgård et al., 2014), and N, the limit for filtration rate per meter longline is within the range of 6,000 to 8,000 l h-1 m-1.
Size ranges of fish fecal particles are highly variable (Reid et al., 2009), and the relative portion of different size classes and settling velocities remains a challenge in the application of aquaculture models (Reid et al., 2020). Since the particles with low settling velocity disperse away from the fish farm, the sensitivity analysis focused on the portion of the particles settling at 1 mm s-1. The sensitivity analysis tested amounts of slowly settling particles from 0% to 27%, with the higher limit based on measurements by Wong and Piedrahita (2000). The proportions of the fastest settling particles at 7.5 cm s-1 were accordingly adjusted, while the proportions of particles settling at 1.5 and 3.2 cm s-1 were unchanged.
3 Results
3.1 Discharge of particulate waste
Smolt was transferred to the farm from January to April 2018 and harvested for 6 months from January 2019. Thus, the entire farming cycle was 18 months, although the individual fish were at sea for 12 –14 months. During the farming cycle, the total wet weight of feed supply to the farm was 4,599 tonnes and the total biomass increase, including fish that died before the harvest, was 4,400 tonnes. Forty percent of the total feed use was supplied to the farm during the 4 months prior to the onset of harvest (Figure 2).
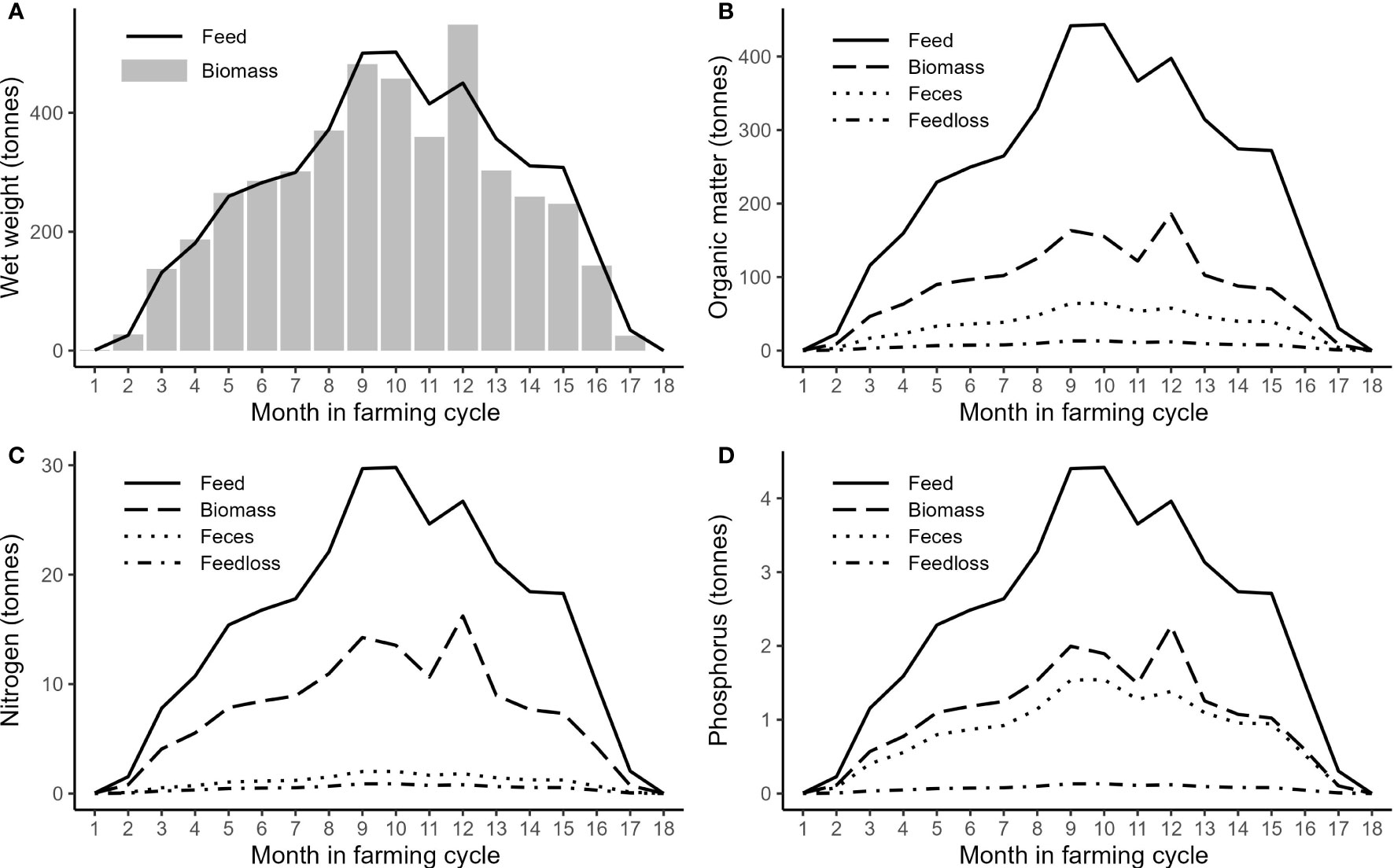
Figure 2 Monthly supply of fish feed and biomass increase (wet weight) (A) and monthly supply of OM (B), N (C), and P (D) with fish feed, incorporation into biomass, and release of particulate matter in feed loss and feces.
According to the analyzed composition of the commercial feed, the total supply of OM was 4,062 tonnes, of which 37% were incorporated into the fish biomass and 15% were released to the environment in the form of feces, according to the calculations (Table 3). The N and P supplied with feed were 273 and 40 tonnes, respectively, and almost half were found in the biomass. Only 7% of the nitrogen was estimated to be released to the environment as feces compared to the estimated 35% of the P. However, the total amount of these elements in feces was quite similar due to the considerably higher ADC for N than P and relative N/P composition of the feed (Table 3).

Table 3 Total supply of OM, N, and P with feed during the production cycle, incorporation in biomass, and discharge to the environment as dissolved, feed loss, and feces. Values in tonnes and percent of total supply with feed in brackets.
The calculated total particulate organic matter (POM) waste from the farm, including both feed and feces, was 712 tonnes, of which the blue mussels potentially can assimilate 83% under the assumption that ambient food particles were unavailable while all the fecal particles were available (PW). The fractions of particulate nitrogen (PN) and particulate phosphorus (PP) available for the mussels were 69% and 92%, respectively.
3.2 Particle dispersion
The current measurements from which the particle dispersion was modeled showed the highest currents to occur in the top 10 m of the water column, where they were influenced by the wind in addition to tidal forces (Figure 3). Current speeds higher than 25 cm s-1 were recorded, and the median current speed was 9.4 cm s-1. From 10 m depth to the seabed, the maximum current speed did not change, while the median current was lowest at 10 m depth (3.4 cm s-1) and increased slightly with depth. In the area below the fish farm, where the submerged mussel farm was modeled, the current speed rarely exceeded 15 cm s-1 (Figure 3).
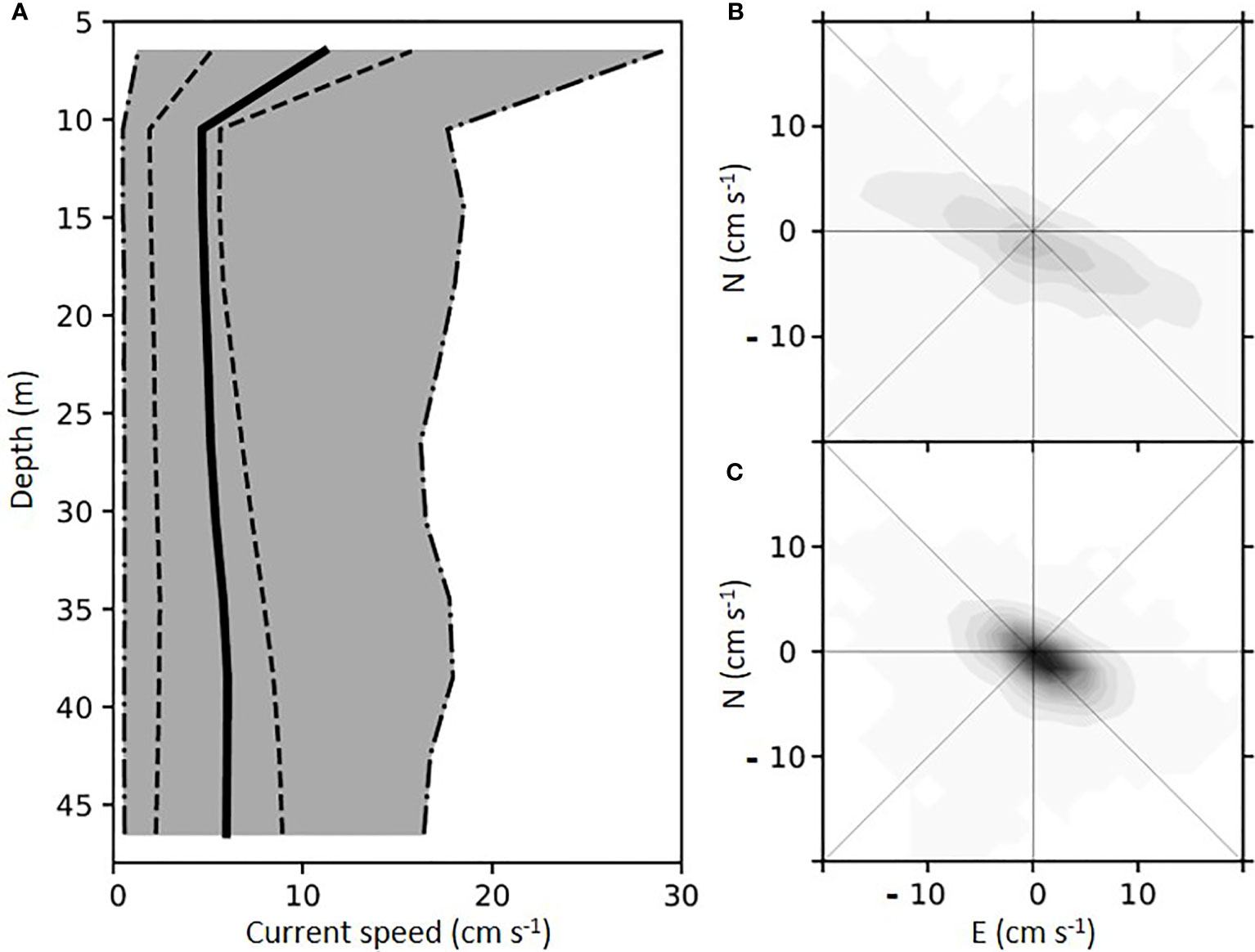
Figure 3 Current speed with depth close to the fish farm (A). The solid line shows the mean value; the dashed lines show the 25 and 75 percentiles, and the gray area covers 95% of the observed current speeds. Distribution of measured velocities at 6.5 m (B) and 22.5 m (C) depths. Positive values are in North (N) and East (E), respectively.
According to the model, only 3.1% of the PW entered the 7,000 m2 area with the mussel farm at the surface next to the wide side of the fish farm (Figure 4A), of which 70% were particles that settled at 1 mm s-1. In addition, 92.5% of the PW entered the submerged mussel farm that covered the 49,000 m2 below the fish farm (Figure 4B). Almost the entire fraction of particles that settled at 7.5 cm s-1 entered the submerged farm, while the slowly settling particles only amounted to 1%.
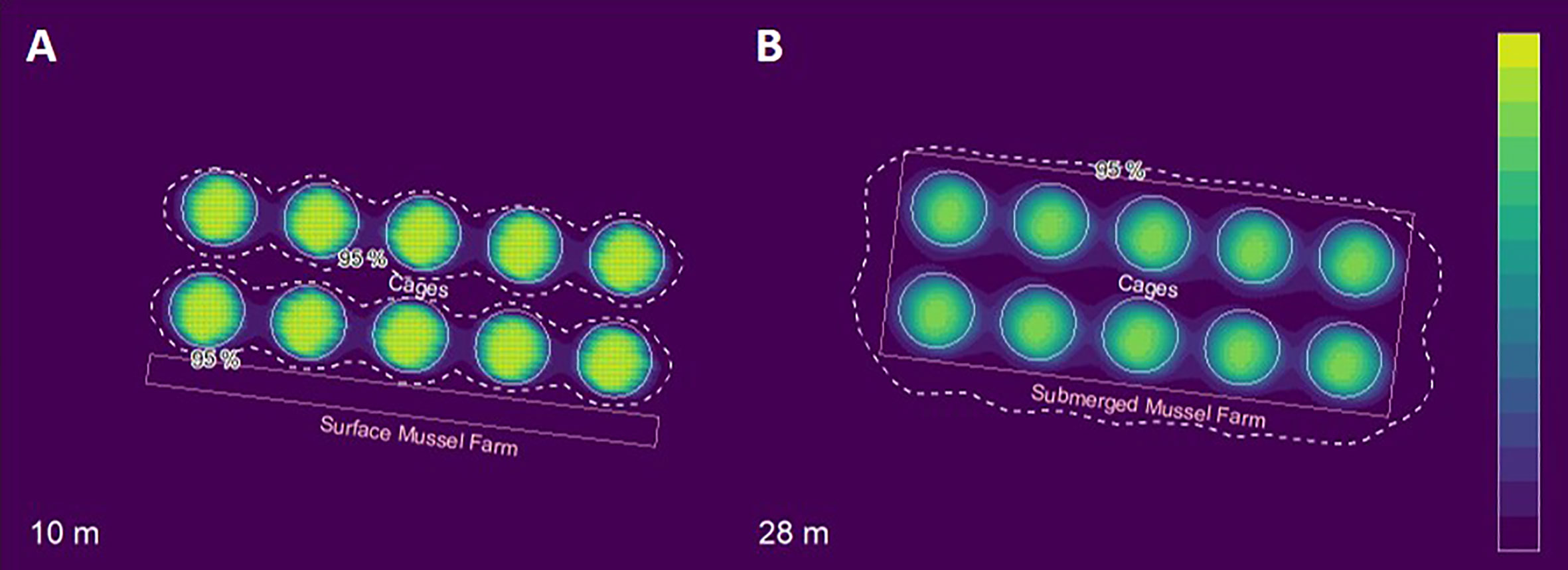
Figure 4 Distribution of waste particles at the base of the surface blue mussel farm (A) and at the base of the submerged mussel farm (B). The 2D Kernel Density Estimate at 95% is also shown (dashed white line). The scale is normalized with highest concentrations shown in yellow.
During October 2018, when the waste production was at its maximum (Figure 2), the average daily influx of fecal POM to the mussel farm at the surface was 18.5 g m-2 d-1, while the inflow of PN and PP amounted to 0.58 and 0.44 g m-2 d-1, respectively. However, the feeding rate and waste production at the fish farm were quite variable over time (Figure 2). Averaged over the entire farming period, the influx of POM was 10.6 g m-2 d-1.
The submerged mussel farm had a higher influx of fecal particles, with a daily POM influx of 39 g m-2 d-1 when the waste production was at its maximum. Averaged over the entire farming cycle, the submerged mussel farm received 22.4 g POM m-2 d-1.
3.3 Assimilation of fish waste in the mussel farms
In the mussel farm at the surface next to the fish farm, 13% of the fecal particles that reached the farm were assimilated according to the model. This amounted to 0.4% of the total PW.
The assimilation of particles in the submerged mussel farm was 16% of the PW that entered the farm. However, since the quantity of particles that entered the mussel farm that covered the entire area below the fish farm was considerably higher than the amount that reached the mussel farm at the surface (Figure 4), the total assimilation of POM during the farming cycle was 86 tonnes, amounting to 15% of the total PW.
The model assumed that the blue mussels could ingest all of the fish feces regardless of particle size or other factors, so it is highly likely that the modeled mitigation is an overestimation for the submerged blue mussel farm.
3.4 Sensitivity analysis
In the blue mussel farm at the surface next to the fish farm, the effect of the current speed on the ingestion of particles was dependent on their settling velocity (Figure 5A). Increased current velocities increased assimilation for denser particles due to increased advection of all particle classes to the surface mussel farm. On the contrary, for the slow-settling particles, maximum assimilation was observed when the current speed was below the measured currents, while increased velocities reduced the assimilation.
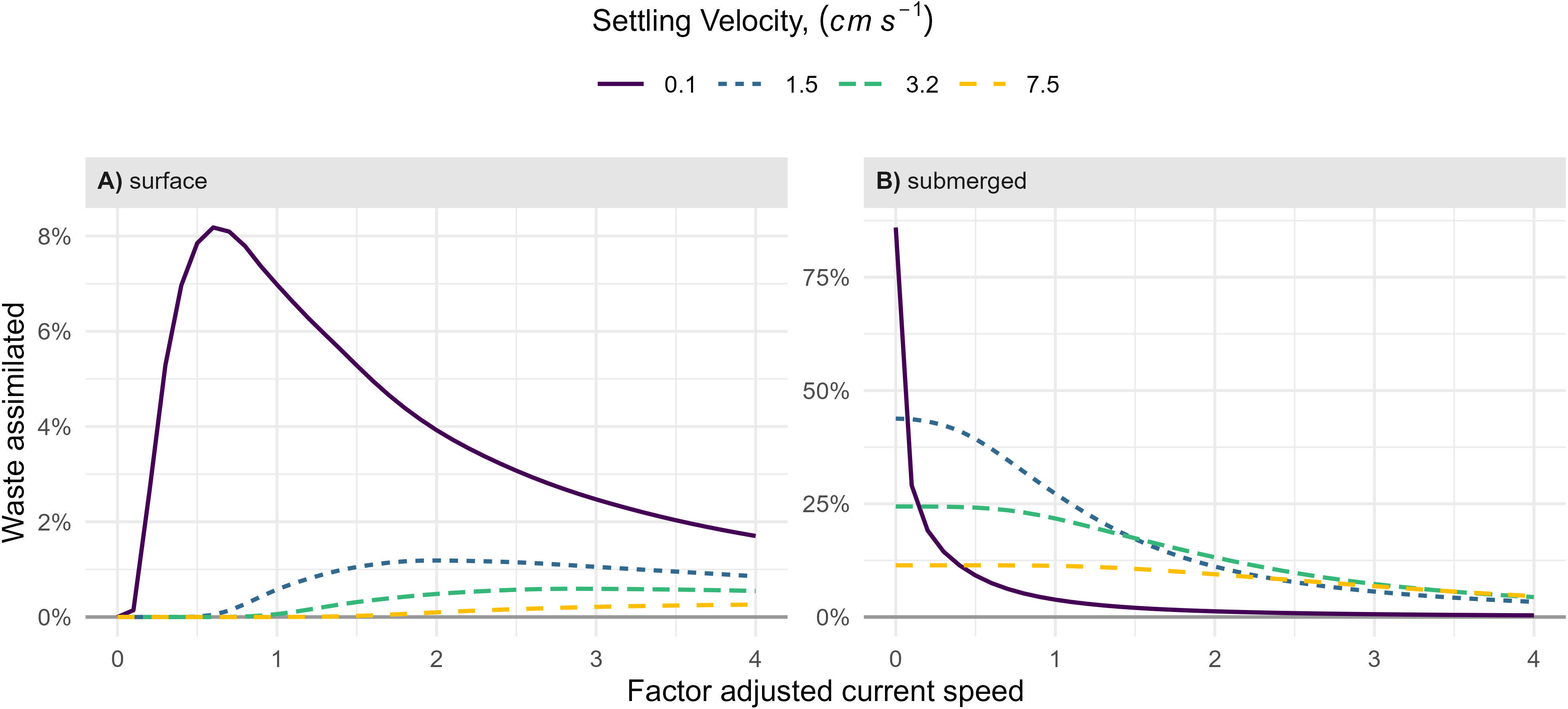
Figure 5 Influence of current speed on particulate waste assimilation calculated for four different settling velocities for the blue mussel farm at the surface (A) and the submerged mussel farm (B). The volumetric filtration rate was 3,840 l m-3 h-1. The factor adjusted current speed at one equals the measured current speed. The mean currents were 8.6 cm s-1 at 0 – 10 m depth and 4.9 cm s-1 in the area of the submerged mussel farm.
For the blue mussel farm below the fish farm, waste assimilation decreased inversely with current speed for all the settling velocities (Figure 5B).
The tested parameters showed a highly different impact on the two blue mussel/fish farm configurations. At the blue mussel farm at the surface, the fraction of slowly settling particles had the most pronounced influence on the total assimilation of fish farm waste (Figures 6A-C). The fraction of slowly settling particles also influenced the impact of current speed on total assimilation. With no slowly settling particles, ingestion increased with the current speed, as more of the faster settling particles reached the farm (Figure 6A), but with high quantities, assimilation decreased with the current speed (Figure 6C). The relation between filtration rate per volume mussel farm and assimilation was linear because assimilation efficiency was fixed in the model. The maximum obtainable waste assimilation at the blue mussel farm at the surface was 5.5% of PW. This was achieved when the fraction of slowly settling particles was 27%, the current speed was three-fourths of the measured currents, and the filtration rate was 16,000 l h-1 m-3.
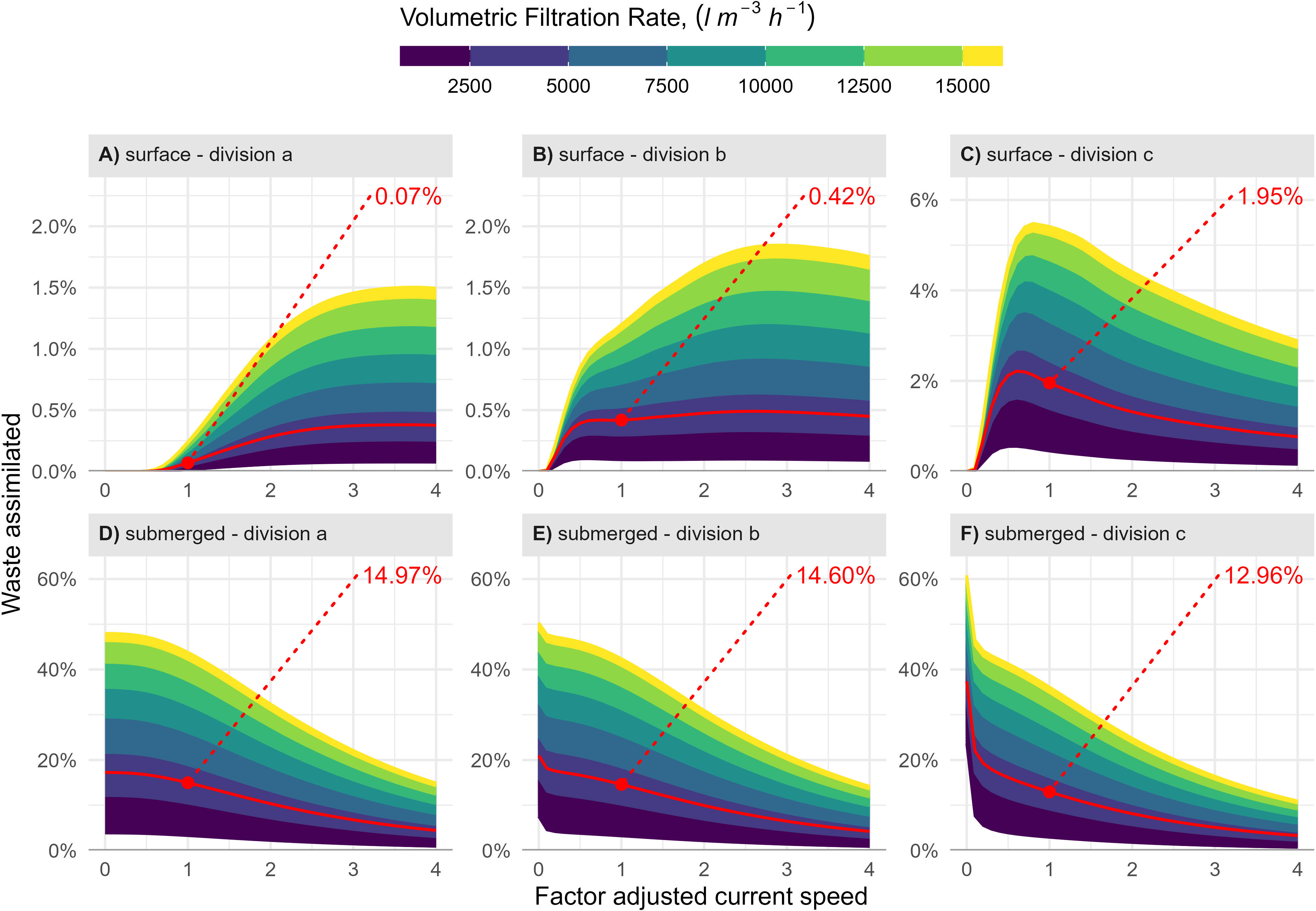
Figure 6 Waste assimilation at the blue mussel farm at the surface (A-C) and the submerged farm (D-F) as influenced by current speed, volumetric filtration rate, and three different combinations of the feces settling velocities. The contributions of particles settling at 1.5 cm s-1 and 3.2 cm s-1 were 10% and 20% in all of the figures. The contributions of particles settling at the lowest and highest velocities 0.1 cm s-1 and 7.5 cm s-1 were 0% and 70%, 5% and 65%, and 27% and 43% for divisions (a–c), respectively. The fish waste assimilated at the measured current speed and volumetric filtration rate set to 3,840 l m-3 h-1 is indicated in red.
The relation between waste assimilation and the tested parameters was more straightforward at the submerged blue mussel farm. The assimilation was highest with low currents, high filtration rate, and low proportions of slowly settling particles (Figure 6).
4 Discussion
This study investigated the removal of PW from a fish farm and did not consider the feeding of natural seston. It was modeled that at a commercial fish farm under the given conditions and assumptions (Table 2), 15% of the OM, 7% of N, and 35% of P were released to the environment in the form of feces, amounting to 591, 19, and 14 tonnes of the respective elements during the course of a farming cycle (Table 3). When the model was run using observed parameters, 3.1% of the feces entered the blue mussel farm when it was modeled at the surface next to the fish farm, and the blue mussels assimilated 0.4% of the total fecal waste. When the model was run with parameters optimized to maximize waste assimilation, the highest obtainable waste assimilation was 5.5% or 32.5 tonnes of OM. Other models have found the highest obtainable removal of fish farm waste by blue mussel farms at the surface near fish farms to be 3.5% or lower (Cranford et al., 2013; Filgueira et al., 2017).
The blue mussel farm modeled below the fish farm received 92.5% of the fecal waste from the fish farm, but due to limited residence time in the mussel farm, assimilation was restricted to 15% of the fecal waste.
4.1 Availability of particulate fish farm waste
The smolt were quite large when they were transferred to the cages (360 g) compared to the 50 – 100 g smolt typically deployed, and in this study, the incorporation of OM, N, and P into biomass was in the higher end of values found in other studies, while the release of PW was in the lower end (Table 3).
In studies from the 1980s and 1990s, only 16% – 22% of the carbon and 19% – 28% of the N were incorporated in fish biomass (Gowen and Bradburry, 1987; Hall et al., 1990; Hall et al., 1992; Ackefors and Enell, 1994). Fish farming and feed quality have evolved considerably since then, which is reflected in the portion of feed incorporated into biomass. More recent studies generally find carbon incorporation of 30% – 38%, somewhat higher N incorporation (32% – 43%), and 20% – 30% of P (Sugiura et al., 2006; á Norði et al., 2011; Wang et al., 2012; Wang et al., 2013).
The discharge of OM and N as PW in this study was somewhat lower than that in other studies where 19% – 71% of the carbon and 11%–28% of the N in the feed were released as feed loss and feces (Gowen and Bradburry, 1987; Hall et al., 1990; Hall et al., 1992; Wang et al., 2012; Wang et al., 2013). The release of PP was within the range of 22% – 54% as previously reported (Holby and Hall, 1991; Sugiura et al., 2006; Wang et al., 2012; Wang et al., 2013).
The daily influx of particulate fish farm waste to the surface mussel farm was 18.5 g m-2 d-1 when the biomass was highest. However, the model assumes a continuous supply of particles during the day and does not account for daily fluctuation, whereby the fish were typically fed during the working day. Hence, the availability of waste particles was variable during the course of a day and the variability in particle flux to the mussel units may influence capture efficiency if particle density exceeds filtration capacity. Resolving temporal fluctuation in waste release could be useful in determining daily variability in assimilation potential.
4.2 Mitigation by blue mussel farm at the surface next to the fish farm
In this study, 13% of the particles that reached the blue mussel farm at the surface were assimilated, comparable to the 10% – 15% assimilation found by Brager et al. (2015). However, this only amounted to 0.4% of the total fecal waste, since only 3.1% of the particulate fish farm waste reached the blue mussel farm. Thus, the mitigation effect by this IMTA approach was nominal when modeled parameters approximated local settings. This highlights the importance of resolving particle transport dynamics in reference to the IMTA configuration to estimate the efficacy of mitigation.
Particles at the outer edge of net cages generally are smaller than 600 µm with low settling velocity (Lander et al., 2013; Law et al., 2014; Brager et al., 2016). The model results are consistent with the observations, as mainly particles with low settling velocity entered the blue mussel farm.
The sensitivity analysis also showed that the fraction of particles with low settling velocity substantially influences the possible mitigation by mussels at the surface next to the fish farm. The maximum mitigation, where 5.5% of the fecal particles were assimilated, was obtained when the portion of slowly settling particles was set to the highest value found in the literature (Wong and Piedrahita, 2000); the mussel density was assumed similar to observations on mitigation farms in inner Danish waters, where settlement tends to be high, and the average current speed was 6.5 cm s-1.
Blue mussel densities depend on natural recruitment rates and management strategies. High densities result in increased size heterogeneity and smaller average sizes, while low densities favor larger sizes and homogeneity, but reduce the total filtration capacity and total biomass at the blue mussel farm (Taylor et al., 2019). The observed density in the area was 600 mussels per meter. With this density at the local hydrodynamic settings and an assumption that 27% of the fecal waste was slowly settling (Wong and Piedrahita, 2000), 2.0% of the waste would be assimilated in the mussel farm at the surface (Figure 6).
The exact portion of slowly settling waste particles remains a challenge to estimate, as the size ranges of fecal particles are highly variable and dependent on feed ingredients, fish size, and local hydrodynamics. In addition, measurements tend to focus on either the small slowly settling or larger faster settling particles (Reid et al., 2009). This study assumed that 5% of the fecal waste settled at 1 mm s-1. Although the exact portion is unknown, most studies assume it to be minor (Cranford et al., 2013; Reid et al., 2020), and the lack of a strong consistent signal of POM around commercial fish farms (Brager et al., 2016) supports this assumption.
Assimilation of slowly settling particles could improve the growth conditions for blue mussels, as observed in some studies (Kerrigan and Suckling, 2018), especially during winter when natural food availability is low (Handå et al., 2012; Lander et al., 2013). IMTA could also benefit blue mussel growth in offshore conditions where food is scarce (Ferreira et al., 2012). Additional benefits of producing blue mussels near fish farms could be immobilizing salmon lice larvae (Bartsch et al., 2013) and disease vectors such as amebic gill disease (Rolin et al., 2016).
However, the effect is minimal when it comes to mitigating the environmental impact of the particulate organic load from fish farming. On the contrary, the ingestion of natural seston by the blue mussels close to the fish farm can even increase the local benthic impact, since digested seston will be defecated by the mussels and psuedofeces will settle close to the farm (Cranford et al., 2013; Hughes and Black, 2016). Ingestion of suspended and slow -settling fish farm waste particles that otherwise would drift away from the farm also potentially increases the local benthic load (Cranford et al., 2013).
4.3 Blue mussels below the fish farm
Locating a mussel farm below a fish farm is a theoretical possibility, although the technical feasibility of fully submerged cultivation units, particularly in exposed waters, is still in commercial development. The blue mussels would not affect access to the fish farm by boat or the flow-through of oxygenated water. However, it might be unpractical unless such mussel farms are an integrated part of the fish farm, where establishment and harvesting of the fish and blue mussels are synchronized, and there might be other practical obstacles. However, from an IMTA perspective, it is interesting to model the potential mitigation as a first step of evaluating the configuration.
In such a setup, more than 90% of the fecal particles reach the mussel farm. In addition, the concentration of phytoplankton is less at 18 – 28 m depth than in the surface waters (Gaard et al., 2011). Thus, fish farm waste would probably comprise a large portion of the mussel’s dietary composition. In total, 15% of the fecal waste from the fish farm was absorbed by the blue mussels in this setup, which is considerably higher than obtainable with blue mussels at the surface (Troell and Norberg, 1998; Cranford et al., 2013; Filgueira et al., 2017). The absorption can even be substantially higher in weaker currents and with higher blue mussel densities (Figure 6). However, contrary to the setup with blue mussels at the surface, where the particles are small slowly settling particles, the assumption that the blue mussels can ingest all of the fecal particles that pass the mussels below the fish farm is questionable. The upper limit for the reported particle size that mussels can filter is 6 mm (Davenport et al., 2000), while the majority of the fecal particles are considerably larger at defecation. According to Bannister et al. (2016), the pellet length of feces from 600, 1,500, and 3,500 g salmon is practically larger than 5 mm for all of the fish weights, while the pellet width from the smallest salmon was less than 5 mm for approximately half of the pellets, a portion that decreased with salmon size. Defragmentation of fecal pellets in the water column during settlement depends on the composition of feed and the physical properties such as particle shear strength and the specific flow conditions at individual sites that modify the particles through fragmentation and aggregation (Schumann and Brinker, 2020).
This modeling study most likely suggests a higher assimilation rate than would be demonstrated in reality, and even the modeled assimilation of 15% of the PW is relatively low, considering that more than 90% of particles intersect the blue mussel farm. This further discounts the efficacy of using mussels as a direct IMTA method by targeting PW.
More efficient mitigation might be obtained by using benthic deposit feeders such as sea cucumbers or polychaetes (Cubillo et al., 2016; Jansen et al., 2019; Nederlof et al., 2020), where particle residence time is not a limitation, as in the case with suspended blue mussels. On the other hand, spatial constraints might be even more limiting with benthic species compared to suspended species. IMTA with benthic species also requires tolerable benthic environmental conditions during the entire farming cycle. This is likely to prove difficult, especially in intensive salmon farms in temperate areas where the feeding activity is highly variable due to biomass and temperature changes (Figure 2).
4.4 Regional level
It has been suggested that IMTA should be considered in the context of integrated coastal area management (i.e., a budgetary approach) rather than defined sites and direct trophic interaction (Chopin, 2017; Small et al., 2019; Sanz-Lazaro and Sanchez-Jerez, 2020; Nederlof et al., 2022). The limited mitigation by direct uptake of particulate fish farm waste also suggests that other mitigation purposes might be more successful, especially when filtration of natural particles and potential increased benthic impact around the fish farm due to enhanced particle immobilization by mussels are taken into consideration (Cranford et al., 2013; Hylén et al., 2021).
Nutrients from fish farms are not incorporated in phytoplankton at the site due to the high flushing rate compared to the time scale for algal growth (Lander et al., 2013). Thus, the potential impact from nutrients will be on the scale of the local ecosystem rather than at the farm. In addition, nutrients from the degradation of particulate fish farm waste are released to the water column and distributed with the currents, and potential resuspension of fish farm waste might spread quite widely (á Norði et al., 2011).
Although the physics and settling velocity of the PW from fish farms limit the direct waste assimilation, the high filtration rate of the blue mussels is useful for mitigating environmental impacts from fish farming and other anthropogenic nutrient sources in the recipient water body, even though there is not necessarily a direct link between the nutrient source and blue mussels (Petersen et al., 2016). Especially in eutrophic coastal areas, positive ecosystem services from blue mussel cultures are likely to occur (Cranford, 2019). An example of the role of bivalves in controlling phytoplankton biomass, although unintended, is from Ringkøbing fjord, where changes in sluice practice caused an increase in salinity and massive recruitment of the clam Mya arenaria. The grazing of the clam caused a shift from a turbid hypereutrophic state with mean annual chl a concentrations of 52 µg l-1 to a clear water state with chl a levels of 9 µg l-1 (Petersen et al., 2019).
The few measurements of chl a concentrations in Faroese fjords during summer have found them to be high and fluctuate between 2 and 17 µg l-1 in the upper water masses (ICES, 2023). This results from weak stratification that is easily broken down by wind forces. Thus, new nutrients are supplied to the upper water masses occasionally during the summer, and they are rarely growth-limiting (Gaard et al., 2011). Chl a measurements in the investigated fjord show a similar range with concentrations up to 12 µg l-1 (Danielsen and á Norði, 2021).
It has been demonstrated that a mussel farm with a potential clearance time of 20% – 35% d-1 of the entire water volume in a region can induce top-down control on phytoplankton biomass under conditions where nutrient concentrations do not limit the primary production. Furthermore, the filtration of large volumes of water reorganizes sedimentation patterns and organic enrichment to a limited area while potentially reducing net sedimentation at the ecosystem scale (Timmermann et al., 2019). Thus, the blue mussels can mitigate the environmental impact by clearing the water column and controlling the spatial deposition of OM.
The ecosystem impacts of blue mussel farming are not simple to predict, however, as mussels can induce both desirable and undesirable feedbacks on the nutrient and organic carbon cycles of the system (Small et al., 2019). Besides the most apparent impacts of nutrient and OM removal from the system in the form of harvestable biomass, undesirable impacts can manifest, such as changes in the plankton community toward smaller plankton due to the size selection of the blue mussels (Cranford, 2019) and excretion of nutrients that may influence the nutrient turnover time and availability in the water column (Petersen et al., 2019).
5 Conclusion
The findings of this study show that the direct assimilation of fish farm waste particles by IMTA with blue mussel farms suspended in the surface waters next to the fish farm does not provide significant mitigation on the total PW load from the farm, which is consistent with other previous findings. However, this study also shows that the assimilation of waste in such IMTA systems is highly dependent on the size and settling velocity of the waste particles. Farming blue mussels below fish cages might increase the mitigation potential provided that the settling particles are within the particle size range the blue mussels can assimilate. This remains unexplored. However, based on the size of fish grown in the present study, feed type and pellet size fed, and estimated feces particle size, it is questionable how much of the waste is within the filtering size range of blue mussels. In order to determine the true mitigation potential of filter feeders in connection to fish farms, there is an emerging need for more information on proportions of waste particle sizes, settling velocities, and defragmentation during settling. This study can help inform further investigation of fish waste particle dynamics and interactions with cultured filter feeders under different configurations.
Data availability statement
The raw data supporting the conclusions of this article will be made available by the authors, without undue reservation. Scripts for the particle tracking model and assimilation by blue mussels are available at the GitHub repository https://github.com/Fiskaaling/modelling_blue_mussels_commercial_fish_farm_imta.
Author contributions
GáN, IL, DT, BJ, BA, TJ, and AH conceived and designed the study, and the modeling was conducted by GáN, TJ, and BA. All authors contributed to the writing of the article and approval of the final version.
Funding
This work was funded by the Nordforsk Nordic Center of Excellence SureAqua, grant no. 82342, and the EU H2020 Research Innovation Programme AquaVitae project, grant agreement no. 818173.
Acknowledgments
The authors thank the fish farming company Hiddenfjord for access to fish farm data and current measurements. We also thank Dagunn H. J. Clementsen for the 3D conceptual drawings.
Conflict of interest
The authors declare that the research was conducted in the absence of any commercial or financial relationships that could be construed as a potential conflict of interest.
Publisher’s note
All claims expressed in this article are solely those of the authors and do not necessarily represent those of their affiliated organizations, or those of the publisher, the editors and the reviewers. Any product that may be evaluated in this article, or claim that may be made by its manufacturer, is not guaranteed or endorsed by the publisher.
References
Ackefors H., Enell M. (1994). The release of nutrients and organic matter from aquaculture systems in Nordic countries. J. Appl. Ichthyol. 10, 225–241. doi: 10.1111/j.1439-0426.1994.tb00163.x
á Norði G., Glud R. N., Gaard E., Simonsen K. (2011). Environmental impacts of coastal fish farming: Carbon and nitrogen budgets for trout farming in Kaldbaksfjørður (Faroe Islands). Mar. Ecol. Prog. Ser. 431, 223–241. doi: 10.3354/meps09113
Bannister R. J., Johnsen I. A., Hansen P. K., Kutti T., Asplin L. (2016). Near- and far-field dispersal modelling of organic waste from Atlantic salmon aquaculture in fjord systems. ICES J. Mar. Sci. 73, 2408–2419. doi: 10.1093/icesjms/fsw027
Barrington K., Chopin T., Robinson S. M. C. (2009). “Integrated multi-trophic aquaculture (IMTA) in marine temperate waters” Integrated mariculture: a global review. FAO Fisheries Aquacult. Tech. Pap. 529, 7–46.
Bartsch A., Robinson S. M. C., Liutkus M., Ang K. P., Webb J., Pearce C. M. (2013). Filtration of sea louse, Lepeophtheirus salmonis , copepodids by the blue mussel, Mytilus edulis, and the Atlantic sea scallop, Placopecten magellanicus, under different flow, light and copepodid-density regimes. J. Fish Dis. 36, 361–370. doi: 10.1111/jfd.12069
Bligh E. G., Dyer W. J. (1959). A rapid method of total lipid extraction and purification. Can. J. Biochem. Physiol. 37, 265–272. doi: 10.1139/o59-099
Brager L. M., Cranford P. J., Grant J., Robinson S. M. C. (2015). Spatial distribution of suspended particulate wastes at open-water Atlantic salmon and sablefish aquaculture farms in Canada. Aquac. Environ. Interact. 6, 135–149. doi: 10.3354/aei00120
Brager L., Cranford P., Jansen H., Strand Ø (2016). Temporal variations in suspended particulate waste concentrations at open-water fish farms in Canada and Norway. Aquac. Environ. Interact. 8, 437–452. doi: 10.3354/aei00190
Chen Y. S., Beveridge M. C. M., Telfer T. C., Roy W. J. (2003). Nutrient leaching and settling rate characteristics of the faeces of Atlantic salmon (Salmo salar L.) and the implications for modelling of solid waste dispersion. J. Appl. Ichthyol. 19, 114–117. doi: 10.1046/j.1439-0426.2003.00449.x
Chopin T. (2017). To enable Integrated Multi-Trophic aquaculture (IMTA) and the Seaweed sector to develop in Canada, regulatory issues will need to be seriously addressed. Bulletin Aquaculture Association Canada 1, 41–44.
Chopin T., Cooper J. A., Reid G., Cross S., Moore C. (2012). Open-water integrated multi-trophic aquaculture: Environmental biomitigation and economic diversification of fed aquaculture by extractive aquaculture. Rev. Aquac. 4, 209–220. doi: 10.1111/j.1753-5131.2012.01074.x
Cranford P. J. (2019). “Magnitutd and extent of water clarification services provided by bivalve suspension feeding,” in Goods and services of marine bivalves. Eds. Small A. C., Ferreira J. G., Grant J., et al (Springer, Cham: Springer Nature Switzerland AG), 119–142.
Cranford P. J., Reid G. K., Robinson S. M. C. (2013). Open water integrated multi-trophic aquaculture: Constraints on the effectiveness of mussels as an organic extractive component. Aquac. Environ. Interact. 4, 163–173. doi: 10.3354/aei00081
Cromey C. J., Nickell T. D., Black K. D. (2002). DEPOMOD-modelling the deposition and biological effects of waste solids from marine cage farms. Aquaculture 214, 211–239. doi: 10.1016/S0044-8486(02)00368-X
Cubillo A. M., Ferreira J. G., Robinson S. M. C., Pearce C. M., Corner R. A., Johansen J. (2016). Role of deposit feeders in integrated multi-trophic aquaculture - A model analysis. Aquaculture 453, 54–66. doi: 10.1016/j.aquaculture.2015.11.031
Custódio M., Villasante S., Calado R., Lillebø A. I. (2020). Valuation of Ecosystem Services to promote sustainable aquaculture practices. Rev. Aquac. 12, 392–405. doi: 10.1111/raq.12324
Dalsgaard J., Pedersen P. B. (2011). Solid and suspended dissolved waste (N, P, O) from rainbow trout (Oncorynchus mykiss). Aquaculture 313, 92–99. doi: 10.1016/j.aquaculture.2011.01.037
Danielsen E., á Norði G. (2021). Blue mussel spat availability and settlement on longlines in a Faroese Fjord (Fiskaaling rit 2021-09). doi: 10.5281/zenodo.6563040
Davenport J., Smith R. J. J. W., Packer M. (2000). Mussels Mytilus edulis: Significant consumers and destroyers of mesozooplankton. Mar. Ecol. Prog. Ser. 198, 131–137. doi: 10.3354/meps198131
Diedericks G., á Norði G. (2021). Modelling of the hydrodynamics in Sørvágsfjørður to support simulation of related fish farming processes. doi: 10.5281/zenodo.7529819
Ferreira J. G., Saurel C., Ferreira J. M. (2012). Cultivation of gilthead bream in monoculture and integrated multi-trophic aquaculture. Analysis of production and environmental effects by means of the FARM model. Aquaculture 358–359, 23–34. doi: 10.1016/j.aquaculture.2012.06.015
Filgueira R., Guyondet T., Reid G. K., Grant J., Cranford P. J. (2017). Vertical particle fluxes dominate integrated multi-trophic aquaculture (IMTA) sites: Implications for shellfish-finfish synergy. Aquac. Environ. Interact. 9, 127–143. doi: 10.3354/aei00218
Gaard E., Norði G., Simonsen K. (2011). Environmental effects on phytoplankton production in a Northeast Atlantic fjord, Faroe Islands. J. Plankton Res. 33, 947–959. doi: 10.1093/plankt/fbq156
Glencross B. D., Carter C. G., Duijster N., Evans D. R., Dods K., McCafferty P., et al. (2004). A comparison of the digestibility of a range of lupin and soybean protein products when fed to either Atlantic salmon (Salmo salar) or rainbow trout (Oncorhynchus mykiss). Aquaculture 237, 333–346. doi: 10.1016/j.aquaculture.2004.03.023
Gowen R. J., Bradburry N. B. (1987). The ecological impact of salmonid farming in coastal waters: a review. Oceanogr. Mar. Biol. 25, 563–575.
Hall P. O. J., Anderson L. G., Holby O., Kollberg S., Samuelsson M. O. (1990). Chemical fluxes and mass balances in a marine fish cage farm. I Carbon Mar. Ecol. ProgSer 61, 61–73. doi: 10.3354/meps061061
Hall P., Holby O., Kollberg S., Samuelsson M. O. (1992). Chemical fluxes and mass balances in a marine fish cage farm. IV Nitrogen Mar. Ecol. Prog. Ser. 89, 81–91. doi: 10.3354/meps070263
Handå A., Min H., Wang X., Broch O. J., Reitan K. I., Reinertsen H., et al. (2012). Incorporation of fish feed and growth of blue mussels (Mytilus edulis) in close proximity to salmon (Salmo salar) aquaculture: Implications for integrated multi-trophic aquaculture in Norwegian coastal waters. Aquaculture 356–357, 328–341. doi: 10.1016/J.AQUACULTURE.2012.04.048
Holbach A., Maar M., Timmermann K., Taylor D. (2020). A spatial model for nutrient mitigation potential of blue mussel farms in the western Baltic Sea. Sci. Total Environ. 736, 139624. doi: 10.1016/j.scitotenv.2020.139624
Holby O., Hall J. (1991). Chemical fluxes and mass balances in a marine fish cage farm. 11. Phosphorus. Mar. Ecol. Prog. Ser. 70, 263–272. doi: 10.3354/meps070263
Hughes A. D., Black K. D. (2016). Going beyond the search for solutions: Understanding trade-offs in European integrated multi-trophic aquaculture development. Aquac. Environ. Interact. 8, 191–199. doi: 10.3354/AEI00174
Hylén A., Taylor D., Kononets M., Lindegarth M., Stedt A., Bonaglia S., et al. (2021). In situ characterization of benthic fluxes and denitrification efficiency in a newly re-established mussel farm. Sci. Total Environ. 782, 146853. doi: 10.1016/j.scitotenv.2021.146853
ICES (2023). Workshop on the faroese ecoregion aquaculture overview (WKFaroesAO). ICES Sci. Rep. 5 (28), 87. doi: 10.17895/ices.pub.21551541
ISO (1998). Animal feeding stuffs — Determination of phosphorus content — Spectrometric method, 16 pp. Available at: https://www.iso.org/standard/12864.html
ISO (2005). Animal feeding stuffs – determination of nitrogen content and calculation of crude protein content – part 2: block digestion/steam distillation method, 14pp. Availble at: https://www.iso.org/standard/52199.html.
Jacobs P., Troost K., Riegman R., van der Meer J. (2015). Length- and weight-dependent clearance rates of juvenile mussels (Mytilus edulis) on various planktonic prey items. Helgol Mar. Res. 69, 101–112. doi: 10.1007/s10152-014-0419-y
Jansen H. M., Hansen P. K., Brennan N., Dahlgren T. G., Fang J., Nederlof M. A. J., et al. (2019). Enhancing opportunistic polychaete communities under fish farms: An alternative concept for integrated aquaculture. Aquac. Environ. Interact. 11, 331–336. doi: 10.3354/AEI00318
Kerrigan D., Suckling C. C. (2018). A meta-analysis of integrated multitrophic aquaculture: extractive species growth is most successful within close proximity to open-water fish farms. Rev. Aquac. 10, 560–572. doi: 10.1111/raq.12186
Kolar K (1992). Gravimetric determination of Moisture and Ash in Meat and Meat Products: NMKL Interlaboratory Study. J AOAC International 75, 1016–1022.
Kraugerud O. F., Penn M., Storebakken T., Refstie S., Krogdahl Å., Svihus B. (2007). Nutrient digestibilities and gut function in Atlantic salmon (Salmo salar) fed diets with cellulose or non-starch polysaccharides from soy. Aquaculture 273, 96–107. doi: 10.1016/j.aquaculture.2007.09.013
Lachance-Bernard M. (2008) Relation biomasse-densité et phénomène d’autoréduction chez la moule bleue (Mytilus edulis) élevée sur collecteur autogéré, Départment de Biologie. Univerité Laval, Québec, 53 pp.
Lander T. R., Robinson S. M. C., MacDonald B. A., Martin J. D. (2013). Characterization of the suspended organic particles released from salmon farms and their potential as a food supply for the suspension feeder, Mytilus edulis in integrated multi-trophic aquaculture (IMTA) systems. Aquaculture 406–407, 160–171. doi: 10.1016/j.aquaculture.2013.05.001
Law B. A., Hill P. S., Maier I., Milligan T. G., Page F. (2014). Size, settling velocity and density of small suspended particles at an active salmon aquaculture site. Aquac. Environ. Interact. 6, 29–42. doi: 10.3354/aei00116
McKindsey C. W., Archambault P., Callier M. D., Olivier F. (2011). Influence of suspended and off-bottom mussel culture on the sea bottom and benthic habitats: A review. Can. J. Zool 89, 622–646. doi: 10.1139/z11-037
Naylor R. L., Goldburg R. J., Primavera J. H., Kautsky N., Beveridge C. M., Clay J., et al. (2000). Effect of aquaculture on world fish supplies. Nature 405, 1017–1024. doi: 10.1038/35016500
Nederlof M. A. J., Fang J., Dahlgren T. G., Rastrick S. P. S., Smaal A. C. (2020). Application of polychaetes in (de)coupled integrated aquaculture: an approach for fish waste bioremediation. Aquac. Environ. Interact. 12, 385–399. doi: 10.3354/aei00371
Nederlof M. A. J., Verdegem M. C. J., Small A. C., Jansen H. M. (2022). Nutrient retention efficiencies in integrated multi-trophic aquaculture. Rev. Aquac. 14, 1194–1212. doi: 10.1111/raq.12645
Oppedal F., Dempster T., Stien L. H. (2011). Environmental drivers of Atlantic salmon behaviour in sea-cages: a review. Aquaculture 311, 1–18. doi: 10.1016/j.aquaculture.2010.11.020
Øverland M., Sørensen M., Storebakken T., Penn M., Krogdahl Å., Skrede A. (2009). Pea protein concentrate substituting fish meal or soybean meal in diets for Atlantic salmon (Salmo salar)-Effect on growth performance, nutrient digestibility, carcass composition, gut health, and physical feed quality. Aquaculture 288, 305–311. doi: 10.1016/j.aquaculture.2008.12.012
Petersen J. K., Holmer M., Termansen M., Hasler B. (2019). “Nutrient extraction through bivalves,” in Goods and services of marine bivalves. Eds. Small A. C., Ferreira J. G., Grant J., et al (Cham: Springer International Publishing), 179–208.
Petersen J. K., Saurel C., Nielsen P., Timmermann K. (2016). The use of shellfish for eutrophication control. Aquacult. Int. 24, 857–878. doi: 10.1007/s10499-015-9953-0
Pettersen A. K., Marshall D. J., White C. R. (2018). Understanding variation in metabolic rate. J. Exp. Biol. 221, jeb166876. doi: 10.1242/jeb.166876
Reid G. K., Lefebvre S., Filgueira R., Robinson S. M. C., Broch A. D., Chopin T. B. R., et al. (2020). Performance measures and models for open-water integrated multi-trophic aquaculture. Rev. Aquac. 12, 47–75. doi: 10.1111/raq.12304
Reid G. K., Liutkus M., Bennett A., Robinson S. M. C., MacDonald B., Page F. (2010). Absorption efficiency of blue mussels (Mytilus edulis and M. trossulus) feeding on Atlantic salmon (Salmo salar) feed and fecal particulates: Implications for integrated multi-trophic aquaculture. Aquaculture 299, 165–169. doi: 10.1016/j.aquaculture.2009.12.002
Reid G. K., Liutkus M., Robinson S. M. C., Chopin T. R., Blair T., Lander T., et al. (2009). A review of the biophysical properties of salmonid faeces: Implications for aquaculture waste dispersal models and integrated multi-trophic aquaculture. Aquac. Res. 40, 257–273. doi: 10.1111/j.1365-2109.2008.02065.x
Riisgård H. U., Kittner C., Seerup D. F. (2003). Regulation of opening state and filtration rate in filter-feeding bivalves (Cardium edule, Mytilus edulis, Mya arenaria) in response to low algal concentration. J. Exp. Mar. Biol. Ecol. 284, 105–127. doi: 10.1016/S0022-0981(02)00496-3
Riisgård H. U., Larsen P. S., Pleissner D. (2014). Allometric equations for maximum filtration rate in blue mussels Mytilus edulis and importance of condition index. Helgol Mar. Res. 68, 193–198. doi: 10.1007/s10152-013-0377-9
Rolin C., Graham J., McCarthy U., Martin S. A. M., Matejusova I. (2016). Interactions between Paramoeba Perurans, the causative agent of amoebic gill disease, and the blue mussel, Mytilus edulis. Aquaculture 456, 1–8. doi: 10.1016/j.aquaculture.2016.01.019
Sanz-Lazaro C., Sanchez-Jerez P. (2017). Mussels do not directly assimilate fish farm wastes: Shifting the rationale of integrated multi-trophic aquaculture to a broader scale. J. Environ. Manage 201, 82–88. doi: 10.1016/j.jenvman.2017.06.029
Sanz-Lazaro C., Sanchez-Jerez P. (2020). Regional Integrated Multi-Trophic Aquaculture (RIMTA): Spatially separated, ecologically linked. J. Environ. Manage 271, 110921. doi: 10.1016/j.jenvman.2020.110921
Schumann M., Brinker A. (2020). Understanding and managing suspended solids in intensive salmonid aquaculture: a review. Rev. Aquac. 12, 2109–2139. doi: 10.1016/j.epidem.2014.09.007
Shomorin G. O., Storebakken T., Kraugerud O. F., Øverland M., Hansen B. R., Hansen J. Ø. (2019). Evaluation of wedge wire screen as a new tool for faeces collection in digestibility assessment in fish: The impact of nutrient leaching on apparent digestibility of nitrogen, carbon and sulphur from fishmeal, soybean meal and rapeseed meal-based diets in. Aquaculture 504, 81–87. doi: 10.1016/j.aquaculture.2019.01.051
Small A. C., Ferreira J. G., Petersen J. K., Strand Ø (2019). Goods and services of marine bivalves (Cham: Springer International Publishing).
Storebakken T., Kvien I. S., Shearer K. D., Grisdale-Helland B., Helland S. J., Berge G. M. (1998). The apparent digestibility of diets containing fish meal, soybean meal or bacterial meal fed to Atlantic salmon (Salmo salar): Evaluation of different faecal collection methods. Aquaculture 169, 195–210. doi: 10.1016/S0044-8486(98)00379-2
Sugiura S. H., Marchant D. D., Kelsey K., Wiggins T., Ferraris R. P. (2006). Effluent profile of commercially used low-phosphorus fish feeds. Environ. pollut. 140, 95–101. doi: 10.1016/j.envpol.2005.06.020
Taylor D., Saurel C., Nielsen P., Petersen J. K. (2019). Production characteristics and optimization of mitigation mussel culture. Front. Mar. Sci. 6. doi: 10.3389/fmars.2019.00698
Timmermann K., Maar M., Bolding K., Larsen J., Windolf J., Nielsen P., et al. (2019). Mussel production as a nutrient mitigation tool for improving marine water quality. Aquac. Environ. Interact. 11, 191–204. doi: 10.3354/aei00306
Troell M., Norberg J. (1998). Modelling output and retention of suspended solids in an integrated salmon-mussel culture. Ecol. Modell 110, 65–77. doi: 10.1016/S0304-3800(98)00042-8
Wang X., Andresen K., Handå A., Jensen B., Reitan J. K., Olsen Y. (2013). Chemical composition and release rate of waste discharge from an Atlantic salmon farm with an evaluation of IMTA feasibility. Aquac. Environ. Interact. 4, 147–162. doi: 10.3354/aei00079
Wang X., Olsen L. M., Reitan K. I., Olsen Y. (2012). Discharge of nutrient wastes from salmon farms: Environmental effects, and potential for integrated multi-trophic aquaculture. Aquac. Environ. Interact. 2, 267–283. doi: 10.3354/aei00044
Ward J. E., Shumway S. E. (2004). Seperating the grain from the chaff: particle selection in suspension- and deposit-feeding bivalves. J. Exp. Mar. Biol. Ecol. 300, 83–130. doi: 10.1016/j.jembe.2004.03.002
Wong K. B., Piedrahita R. H. (2000). Settling velocity characterization of aquacultural solids. Aquac. Eng. 21, 233–246. doi: 10.1016/S0144-8609(99)00033-3
Zhang J., Zhang S., Kitazawa D., Zhou J., Part S., Gao S., et al. (2019). Bio-mitigation based on integrated multi-trophic aquaculture in temperate coastal waters: Practice, assessment, and challenges. Lat Am. J. Aquat Res. 47, 212–223. doi: 10.3856/vol47-issue2-fulltext-1
Keywords: IMTA, particulate waste, blue mussels, Atlantic salmon, modelling, spatial setup, settling velocities
Citation: á Norði G, Lund I, Andreasen B, Taylor D, Johannesen TT, Jacobsen B and Hughes AD (2023) Modeling particulate waste assimilation by blue mussels within the spatial constraints of a commercial fish farm: implications for multitrophic aquaculture. Front. Mar. Sci. 10:1236294. doi: 10.3389/fmars.2023.1236294
Received: 07 June 2023; Accepted: 27 July 2023;
Published: 25 August 2023.
Edited by:
Morten Omholt Alver, Norwegian University of Science and Technology, NorwayReviewed by:
Laura B. Ribeiro, Portuguese Institute for Sea and Atmosphere (IPMA), PortugalNafsika Papageorgiou, National and Kapodistrian University of Athens, Greece
Copyright © 2023 á Norði, Lund, Andreasen, Taylor, Johannesen, Jacobsen and Hughes. This is an open-access article distributed under the terms of the Creative Commons Attribution License (CC BY). The use, distribution or reproduction in other forums is permitted, provided the original author(s) and the copyright owner(s) are credited and that the original publication in this journal is cited, in accordance with accepted academic practice. No use, distribution or reproduction is permitted which does not comply with these terms.
*Correspondence: Gunnvør á Norði, Z3VubnZvckBmaXNrYWFsaW5nLmZv