Experimental study on the effect of sound stimulation on hearing and behavior of juvenile black rockfish (Sebastes schlegelii)
- 1Laboratory of Marine Fisheries Technology, Fisheries College, Ocean University of China, Qingdao, China
- 2College of Fisheries and Life Science, Dalian Ocean University, Dalian, China
Assessing the potential impacts of wind farm noise on fish is a crucial aspect of Environmental Impact Assessment (EIA) studies. There is increasing evidence of disturbances and effects on hearing and behavior in animals. The black rockfish (Sebastes schlegelii) is a commercially valuable rocky reef fish native to East Asia. However, empirical studies that measure the actual consequences are lacking. In this study, we used auditory evoked potentials (AEP) to assess the effects of dominant frequency noise emitted by offshore wind farms on the auditory sensitivity, hearing threshold, swimming, and feeding behavior of juvenile black rockfish. The experimental findings revealed that the most sensitive sound frequency was 200 Hz, with the lowest hearing threshold recorded at 86.4 ± 3.4 dB re 1 μPa. Following 3 and 7 days of exposure to 200 Hz noise at 110 dB, threshold shifts in black rockfish reached 19.0 dB and 13.3 dB, respectively. During the subsequent recovery phase, these shifts decreased to approximately 9.8 dB after 3 days, respectively. The noise-exposed group exhibited higher swimming duration, moving distance, and caudal fin swing frequency compared to the control group without noise exposure. Furthermore, noise prolonged the feeding rate of black rockfish. Our findings provide the first evidence of noise-induced temporary threshold shift and behavioral disturbances in juvenile black rockfish, implying potential fitness consequences associated with noise pollutant.
1 Introduction
With the increasing energy demand and the pursuit of sustainable development in society, the offshore wind industry has experienced significant growth worldwide in recent decades (Slabbekoorn et al., 2010). Many countries are now focusing on utilizing offshore wind farm (OWF) facilities to operate aquaculture systems, aiming to enhance ocean space utilization and reduce costs. The concept of combining OWFs as fixation points for aquaculture or co-using OWF sites by installing aquaculture farms between wind turbines has garnered considerable attention in recent years (Lindell, 2003; Wever et al., 2015; Tullio et al, 2018). However, the noise generated by OWFs primarily arises during the construction and operation stages (Nedwell et al., 2003; De Jong et al., 2011). Based on extensive measurements reviewed by Madsen et al. (2006), the underwater noise produced by operating wind turbines is predominantly limited to low frequencies (below 1 kHz) and low intensity. For instance, the frequency peak observed at the Vindeby OWF in Denmark and the Gotland OWF in Sweden was 25 Hz and 160 Hz, respectively (Nedwell, Langworthy and Howell, 2004). Similarly, the Horns Rev OWF in Denmark exhibited frequency peaks at 150 Hz and 300 Hz (Betke et al., 2005). Additionally, measurements conducted at three different types of OWFs in Denmark and Gotland (Middelgrunden, Vindeby, and Bockstigen-Valar) revealed a noise frequency range below 500 Hz (Tougaard et al., 2009). Blew et al. (2008) summarized the frequency peaks of four OWFs in Denmark, which were 176 Hz, 150 Hz, 135 Hz, and 134 Hz, respectively, with corresponding sound pressure levels (SPLs) of 114 dB, 117 dB, 110 dB, and 122 dB. In the case of the East China Sea Bridge OWF, the noise frequency range was below 400 Hz, and the SPLs ranged from 81 dB to 99 dB (Zhang et al., 2016). Tougaard et al. (2020) compiled data from 46 measured values obtained from 14 wind farms. Although the distances and wind speeds of the measurements varied, the dominant frequencies were found to be in the range of 10-400 Hz, with equivalent continuous sound levels (LAeq) ranging from 80 dB to 135 dB re 1 μPa.
Previous studies have demonstrated the influence of disturbances on fish behavior, including responses to noise (Stevens and Don, 1979; Russell et al., 2001; Sara et al., 2007; Ladich and Fay, 2013; Andrew et al., 2016; Velasquez et al., 2020; Jones et al., 2020). Andersson et al. (2007) conducted a study on two different fish species, Rutilus rutilus L and Gasterosteus aculeatus, exposed to single-tone frequencies and sound generated by offshore wind turbines. They observed various swimming patterns in sticklebacks (Gasterosteus aculeatus), including forward swimming, twitching, backing, and freezing. Previous studies have indicated that prolonged exposure to noise can lead to damage or loss of hair cells in the fish’s inner ear. Severe auditory insults can result in temporary threshold shifts (TTS) or permanent threshold shifts (PTS). In a study by Popper et al (1976), goldfish were exposed to pure tones, and sound pressure levels (SPLs) of 149 dB re 1 μPa caused threshold shifts of approximately 7-9 dB at 500 Hz and 18-27 dB at 800 Hz. Amoser and Ladich (2003) exposed goldfish to white noise at 158 dB re 1 μPa for 24 hours and found the greatest hearing loss at 800 Hz and 1000 Hz. Smith et al. (2004) reported significant threshold shifts (up to 28 dB) at all frequencies in goldfish due to noise exposure, with larger shifts occurring at frequencies where their hearing sensitivity is highest. The negative impact of OWF (OWF) noise on fish has become a matter of concern. Understanding the auditory threshold shift and recovery of fish is crucial (Amoser and Ladich, 2003). Striking a balance between energy development and the preservation of aquatic life is imperative (Lacroix and Sylvain, 2011; Thompson et al., 2020).
In this study, we focused on the black rockfish (Sebastes schlegelii) as our research subject. The black rockfish belongs to the Scorpaenidae family and is an economically important marine ovoviviparous teleost species. It is widely distributed and cultured in Japan, Korea, and the northeast coast of China (Likang et al., 2018). The objectives of our study were as follows: (i) To determine the sensitive sound frequency and hearing threshold of the black rockfish using the auditory evoked potential (AEP) method. (ii) To assess the degree of hearing loss (temporary threshold shift or TTS) in the black rockfish. (iii) To investigate the impact of noise from OWFs at prominent frequency ranges on fish behavior. The findings of this study are expected to provide valuable insights and serve as a reference for the development of OWFs, taking into consideration the effects on black rockfish and other similar species.
2 Methods
2.1 Study species
Juvenile black rockfish (n=80; standard length: 6.99 ± 0.51 cm; wet mass: 19.47 ± 2.93 g) were studied at the Fish Behavior Laboratory at the Dalian Ocean University. Before the experiment, the fish were farmed in a polypropylene water bucket (diameter 52 cm, water depth 46 cm) for 5 days. Water temperature was 21.4-22.8°C and dissolved oxygen was7.89 ± 0.03 mg/L. Fish were fed once daily at noon and 50% of the water was changed after 2 days to clean out excrement. We present the feeding amount based on the experience in the rearing phase. The total quantity fed daily was 1.1% fish body weight (1.7 g/day).
2.2 Auditory evoked potential recordings of black rockfish
The auditory evoked potential (AEP) test conducted in this study was based on the method described by Kenyon et al. (1998). The experimental setup is illustrated in Figure 1. To minimize external noise interference, all equipment was placed inside a soundproof room with an internal sound pressure level measuring 35.2 dB. The testing was carried out in a cylindrical Polyvinyl Chloride (PVC) water bucket with an inner diameter of 0.4 m and a height of 0.4 m. The bucket was positioned on a shockproof table, and an aluminum radiation-proof cloth was applied to the outer wall to prevent noise disturbances. Juvenile black rockfish were first anesthetized and then securely positioned at the center of the tank. The water level was maintained at approximately 3 mm below the fish’s skull. Sound stimuli were generated using an underwater speaker (UW-30, China). The generated sound was pre-amplified with a Crown amplifier (D-75A, China) and analyzed in real-time using a Tucker-Davis Technologies (TDT) system (RZ6, USA) equipped with a hydrophone probe mounted near the fish’s head. The hydrophone probe was connected to an acoustic acquisition system (Aquafeeler IV, Roland, Japan) used for online measurements. The hydrophone probe had a sensitivity of -190 dB re 1 μPa and a bandwidth of 20 Hz to 200 kHz. Ambient noise and stimulus noise were recorded during the experiments. AEP waveforms were generated using the SigGenRZ software.
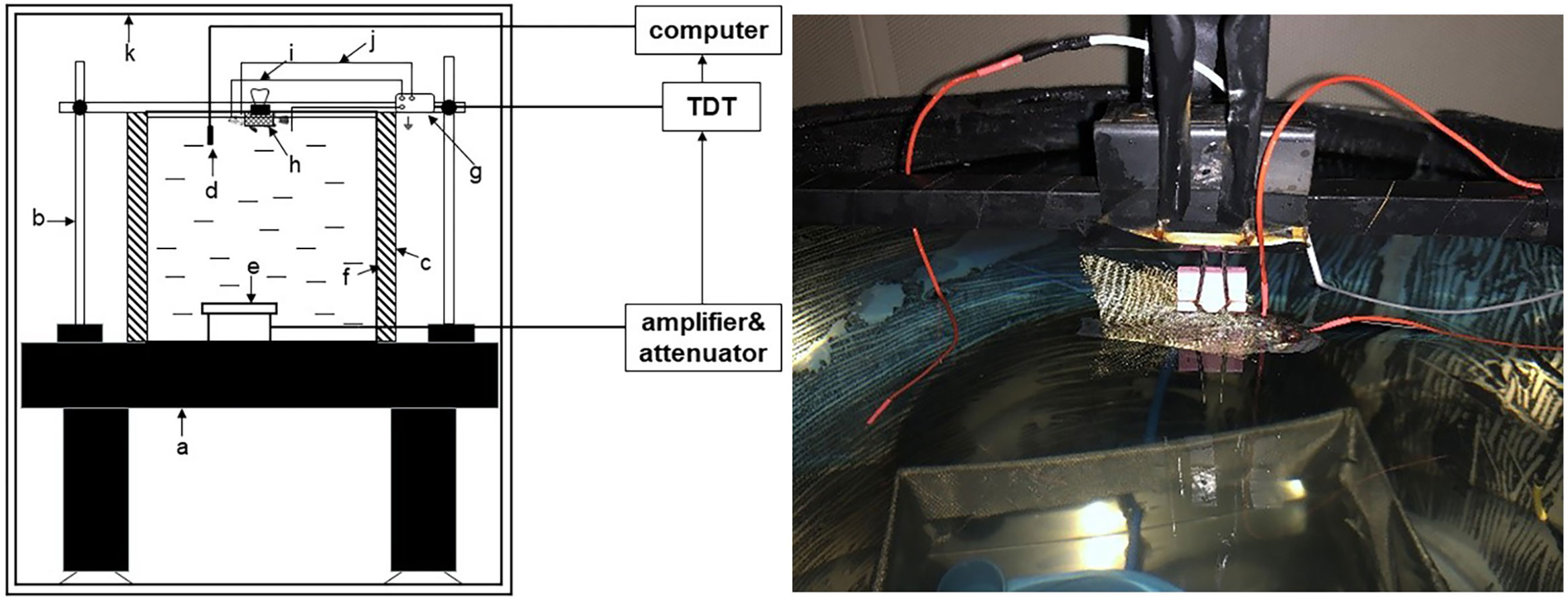
Figure 1 Test installation diagram of AEP. a, holographic-table; b, fixed frame; c, acoustic insulation material foam; d, hydrophone; e, underwater speaker; f, water tank; g, preamplifier; h, fish; i, reference electrode; j, recording electrode; k, sound-proof chamber.
Each experimental case in this study involved the use of a single live fish and lasted for a duration of 2 hours. A total of 8 cases were conducted, but unfortunately, one of them resulted in the death of the fish. Prior to any manipulation, the fish were anesthetized by immersing them in water containing 1:14000 tricaine methanesulfonate (MS-222) until their movements ceased, which typically took around 50 seconds. To minimize myogenic noise levels, the fish were temporarily immobilized by injecting gallamine triiodide (Sigma-Hefei Bomeranian Biology) at a dosage of 1.2-1.4 μg/g per body weight. The anesthesia lasted for 4 to 5 hours. For the AEP recordings, two silver needles with a length of 5 mm and a diameter of 0.25 mm were used as electrodes. One needle was inserted through the fish’s skull at the medulla region and served as the recording electrode, while a reference electrode was placed subcutaneously between the fish’s eyes Codarin and Wysocki (2009). Two additional electrodes were inserted subcutaneously at a depth of 2.5 mm. Shielded electrode leads were connected to the preamplifier’s input, and the other end of the shielded electrode was positioned posterior to the fish’s body. The reference, ground, and recording electrodes were connected to the amplifier. The sound stimuli used in the experiment were short alternating tone bursts with frequencies of 100 Hz, 200 Hz, 300 Hz, and 500 Hz, which were selected based on the dominant frequency of offshore wind turbine noise. The tone bursts were presented at a repetition rate of 10/s and alternated between 90° and 270° phases. The duration of the sound stimuli ranged from 2 cycles at 100 Hz and 200 Hz to 5 cycles at 300 Hz and 500 Hz. The rise and fall times of the stimuli increased from 1 cycle at 100 Hz and 200 Hz to 2 cycles at 300 Hz and 500 Hz. A total of 1000 stimuli were presented for each polarity. The lowest sound pressure level (SPL) at which a repeatable AEP trace could be obtained was considered the threshold. The SPLs were attenuated in 5-dB steps initially. As the threshold was approached, the SPLs were further attenuated in 1-2 dB steps until the threshold level was determined
2.3 Audiometry test under noise exposure
Sixteen juvenile black rockfish were selected for this study and divided into two groups: a noise-exposed group and a controlled and sheltered group, as depicted in Figure 2. Based on previous research on the dominant noise of single-pile foundation of OWFs, the stimuli used for noise exposure in this study consisted of 200 Hz pure tones at a sound pressure level of 110 dB (Betke et al., 2005; Tougaard et al., 2009; Marmo et al., 2014). The duration of noise exposure for each treatment group was set at 7 days. AEP images were collected separately at two time points: 3 days and 7 days after the initiation of noise exposure for both groups. Additionally, images were recorded 1 and 3 days after the recovery period.
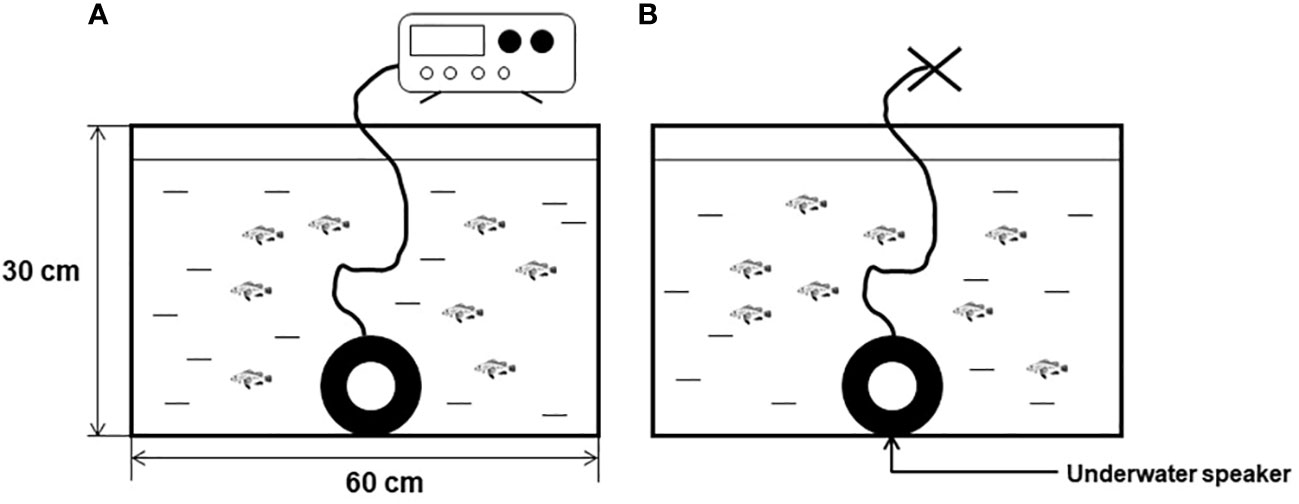
Figure 2 Acoustic exposure behavior test diagram (A) for the acoustic exposure group, (B) for the control group).
2.4 Behavior test under noise exposure
The noise exposure group of black rockfish was subjected to continuous exposure to a pure tone of 200 Hz at an intensity of 110 dB for a period of 7 days. Throughout this period, video recordings capturing the behavior of the fish from a bird’s-eye view were conducted at three specific time points each day: 7:00, 13:00, and 21:00. The duration of each video recording was 5 minutes, and the middle 3 minutes were selected for behavior analysis. Specifically, after the swimming video recording at 13:00 each day, additional videos capturing the feeding behavior of both the noise exposure group and the controlled group were recorded. To minimize anthropogenic disturbances, baits were introduced into the tank using a tube rather than directly by hand. To analyze the potential interaction between tailbeat frequencies (TBF) and swimming time (ST), the analysis of locomotor behavior of the fish was conducted from a side view perspective. Additionally, the feeding time and feed rate of the bottom bait were recorded from each feeding behavior video. The quantity of food provided during the test phase was the same as that during the rearing phase. All the criteria used for recording and analysis are summarized in Table 1.
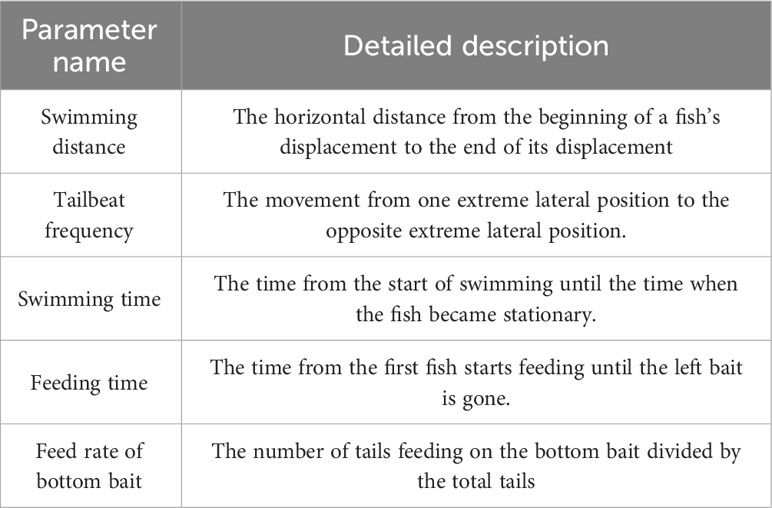
Table 1 Parameter definition used in the collection of behavioral data from black rockfish exposed or not to sound.
2.5 Correlation parameter and data processing
The behavioral data collected were analyzed to determine their normal distribution using the Shapiro-Wilk normality test, which was conducted using SPSS Statistics (Version 17.0). Since the data did not follow a normal distribution, non-parametric tests, specifically the Mann-Whitney test, were performed to compare the differences between the control group and the noise group. The results of the analysis were expressed as the median values with the interquartile range (IQR). To visualize the findings, plots were generated using GraphPad Prism 8 software. The activity behaviors of the black rockfish were tracked and recorded using video footage. The motion trajectory of each individual fish was determined, and the distances traveled were measured using the Digimizer software system. This software facilitated the analysis of the recorded videos to extract relevant behavioral parameters.
2.6 Ethical note
All experimental procedures conducted in this study adhered to the guidelines set forth by the Institutional Animal Care and Use Committee (IACUC). The study protocol, including the ethical considerations and animal welfare practices, was reviewed and approved by the Animal Welfare and Ethical Review Committee of Ocean University of China (IACUC—2105003). Throughout the study, all relevant international, national, and institutional guidelines and regulations regarding the care and use of animals were strictly followed to ensure the ethical treatment and well-being of the black rockfish used in the experiment. These measures were implemented to guarantee the humane and responsible conduct of the research.
3 Results
3.1 Sensitive sound frequency and hearing threshold
AEP (auditory evoked potential) waveforms were successfully obtained from 7 black rockfish that were tested in the study. Figure 3 provides an overview of the AEP waveforms specifically generated in response to a 100 Hz sound stimulus. It can be observed that as the sound intensity decreased, the magnitude of the AEP response also declined. Through repeated testing, it was determined that the average number of wave peaks in the AEP waveforms between sound pressure levels (SPLs) of 105 dB and 93 dB was approximately 8. However, when the SPL was further reduced to 92 dB, recognizable and repeatable waveforms could no longer be produced. This suggests that the AEP threshold for the black rockfish at a frequency of 100 Hz is 92 dB, indicating that the fish’s auditory response becomes undetectable below this sound pressure level.
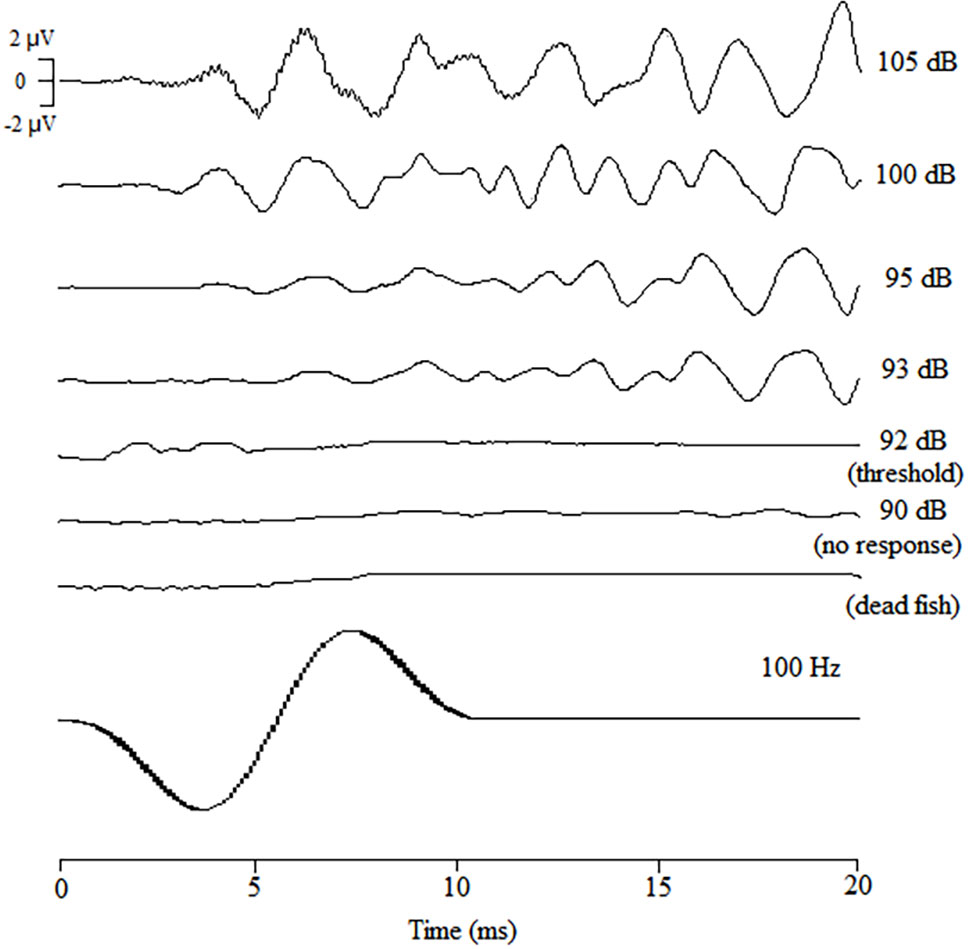
Figure 3 AEP waveforms of black rockfish obtained in response to tone bursts of 200 Hz attenuated from 85 to 100 dB (1000 times repeated).
Audiograms were recorded using the AEP instrument, and the results are presented in Figure 4. It is important to note that the minimum threshold values obtained from the audiograms were considerably higher than the measured background noise level. This indicates that the audiogram data were not influenced by the background noise. The audiograms reveal a decreasing trend in auditory thresholds within the frequency range of 100-200 Hz, indicating that the black rockfish exhibited relatively lower thresholds for sound stimuli in this frequency range. However, beyond 200 Hz and up to 500 Hz, the thresholds showed a tendency to rise, implying that the fish had higher auditory thresholds in this frequency range. Of particular interest is the lowest threshold observed at 200 Hz, which was measured to be 86.4 ± 3.4 dB.
3.2 Effect of 200 Hz noise on the hearing threshold
The degree of hearing loss was quantified by measuring noise-induced threshold shifts during sound stimulation at 110 dB SPL and 200 Hz, as shown in Figure 5. After 3 days of noise exposure, the average auditory thresholds increased by 19.0 ± 8.4 dB. The maximum threshold shift observed was 27.4 dB at 100 Hz. After 7 days of noise exposure, the average threshold shift was 13.3 ± 8.3 dB, with a maximum shift of 21.6 dB at 200 Hz. During the recovery period, the auditory thresholds gradually improved. After the third day of recovery, the decrease was 9.8 ± 5.6 dB. The maximum threshold shifts during recovery was 18.6 dB at 200 Hz, respectively. Overall, the degree of threshold shift decreased with increasing frequency and increased exposure time. However, except for the 3-day exposure group (p=0.019), there were no significant differences between the control group and the noise-exposed group (p>0.05). The greatest threshold shifts were observed at 100 Hz (27.4 dB) and 200 Hz (26.1 dB) after 3 days of noise exposure. However, hearing thresholds for all frequencies were reduced after 7 days of noise exposure compared to 3 days of exposure. Furthermore, even after 3 days of recovery, the auditory thresholds of the black rockfish did not return to the control levels. These findings suggest that noise exposure can lead to significant threshold shifts in the black rockfish, with the extent of the shifts depending on the frequency and duration of exposure. After 3 days, 7 days of noise exposure, and 3 days of recovery, the best frequency of black rockfish changed from 200 to 300 Hz.
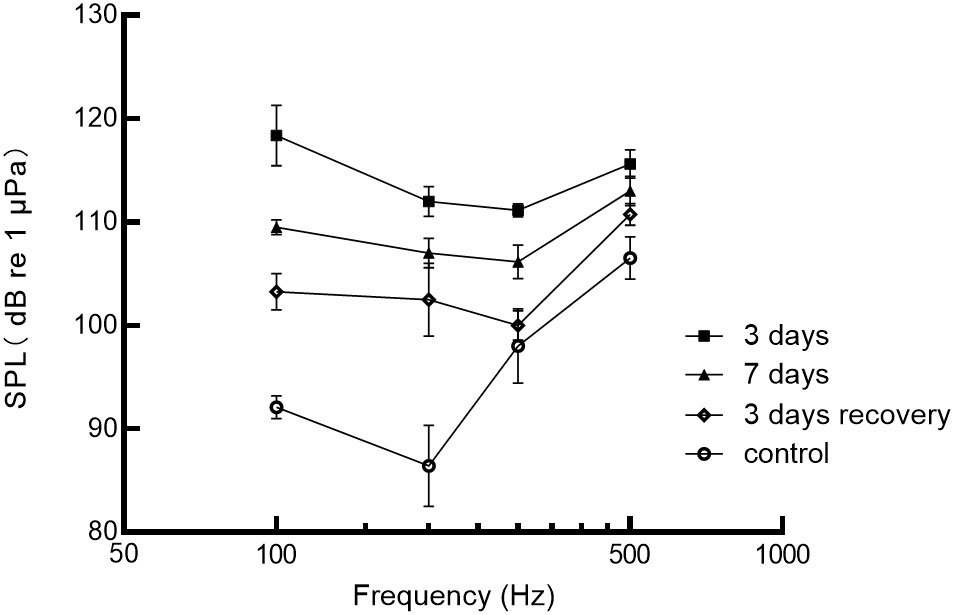
Figure 5 Auditory sensitivity of the black rockfish before (control) and after exposure to 200 Hz noise for 3 or 7 days at 110 dB and 3 days of recovery.
3.3 Effects of 200 Hz noise on behavior
3.3.1 Swimming distance
The effect of noise stress on the swimming behavior of black rockfish was examined, and the results are presented in Figure 6. It was observed that the fish in the test group exhibited startle responses and even jumped out of the water during the initial noise stress. The motion tracks of the black rockfish during a 3-minute observation period are depicted. The distribution of the fish was mainly on the sidewalls of the tank, as shown in the figures. The average horizontal movement distance for the test group on day 1 was 2.38 ± 1.00 m, while for the control group it was 1.42 ± 0.16 m. On day 3, the distance for the test group increased to 3.04 ± 0.54 m, and for the control group, it was 2.56 ± 1.18 m. On day 7, the distance for the test group further increased to 3.38 ± 0.53 m, and for the control group, it was 3.56 ± 0.58 m. Initially, the distance moved by the test group was greater than that of the control group, indicating a more active swimming response to the noise stress. However, as the exposure time increased, the gap between the two groups gradually closed. By day 7, the test group’s distance reached a constant level similar to that of the control group, and there was no significant difference between the two groups (p>0.05). Based on the visualization of the swimming trend, it can be concluded that black rockfish are capable of habituating to noise over a relatively short period, at least in terms of routine swimming behavior. The average distance moved by both the control and experimental groups became similar after 7 days of noise exposure, suggesting a habituation response to the noise stress.
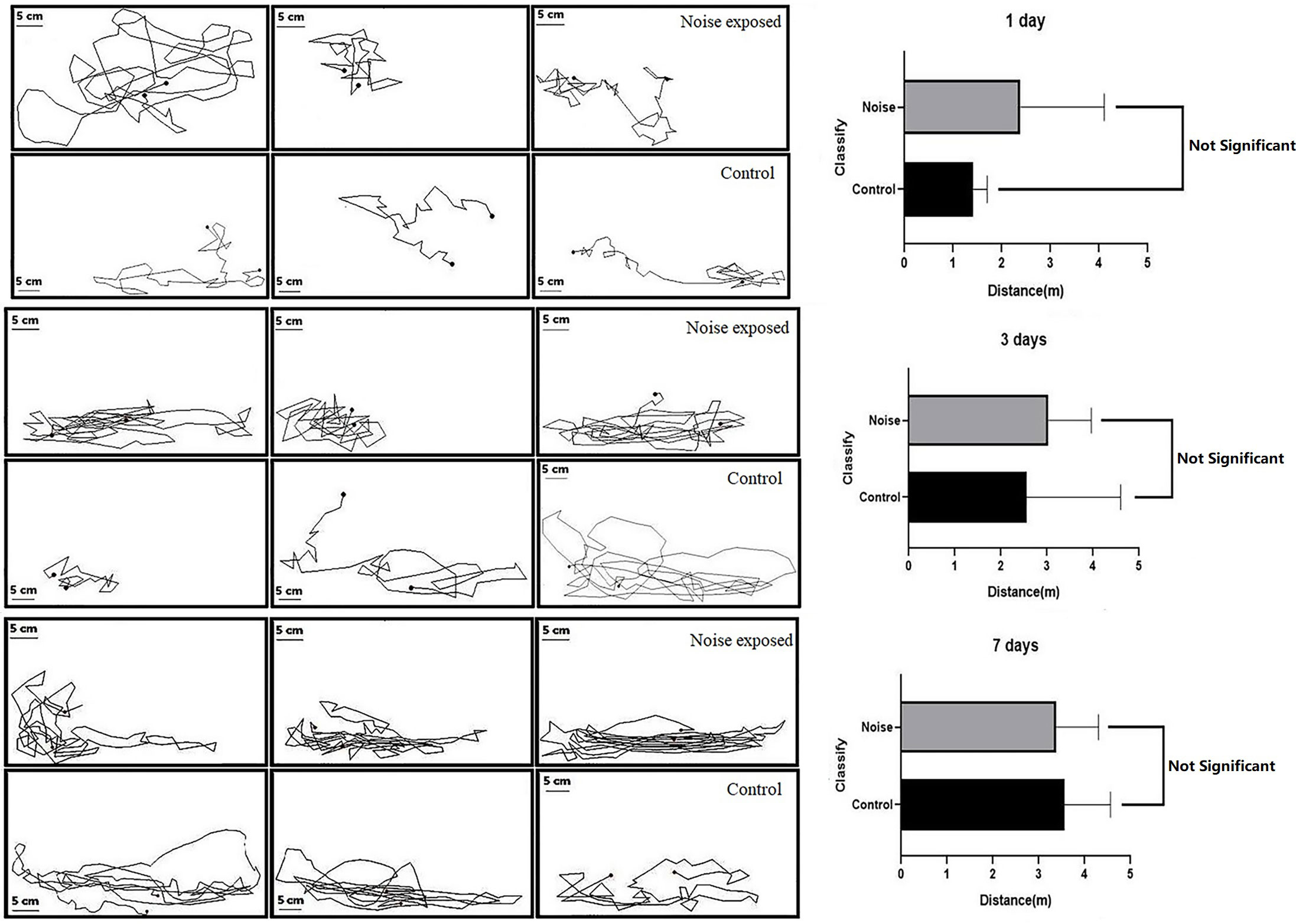
Figure 6 Trajectory diagram (left) and movement distance (right) of black rockfish after exposure to 200 Hz noise (110 dB) for 1, 3, and 7 days, 3 min each time (p>0.05).
3.3.2 Tailbeat frequencies and swimming time
The average tail beat frequency and swimming duration of the test group and control group are presented in Figure 7 and Table 2. The interquartile range of the tail beat frequency in the test group were 246 times, while in the control group, they were 159 times. The average tail beat frequency in the test group was significantly higher than that in the control group (82 times/min vs 53 times/min, p=0.001). There was a significant difference observed between day 4 (p=0.007) and day 7 (p=0.015) in both the control and test groups. Additionally, the interquartile range of the swimming time in the test group were 60.6 s, while in the control group, they were 45.9 s. The average swimming duration of the test group was higher than that of the control group (33.67% vs 25.50%, p<0.05). This difference was found to be significant on day 1, day 2, and day 5 compared to the control group (p<0.05). These results indicate that the fish in the test group exhibited increased tail beat frequency and longer swimming durations compared to the control group, indicating a heightened level of activity and possibly stress response to the noise exposure.
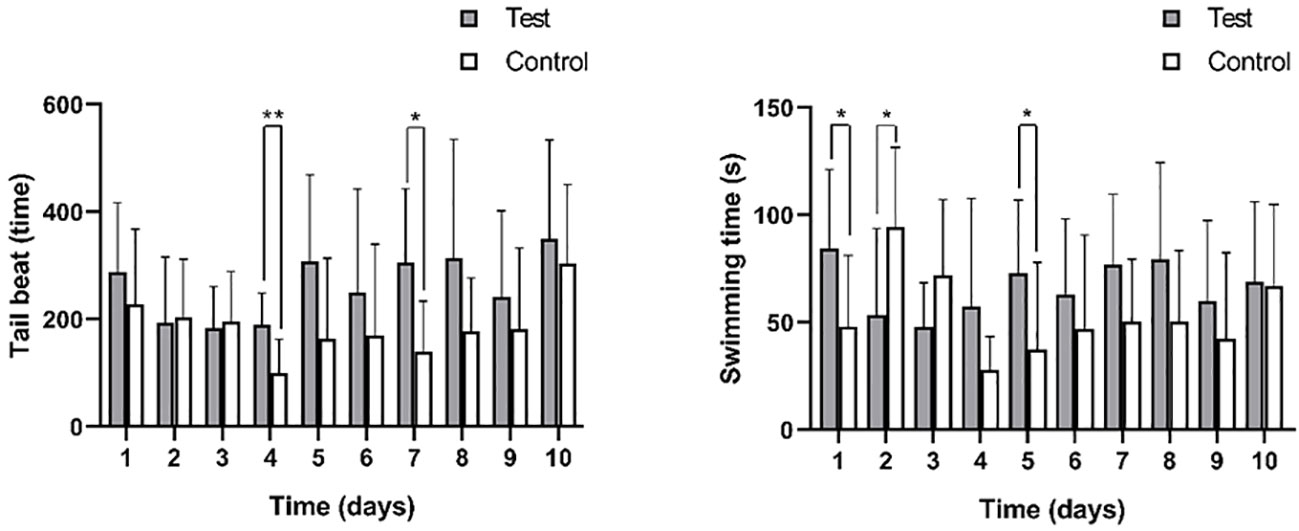
Figure 7 The tail beat times (left) and swimming time (right) of black rockfish were measured 3 min each time after exposure to 200 Hz noise (110 dB) for 11 days (7 days acoustic stress+3 days recovery). * p value < 0.05, ** p value < 0.01.
3.3.3 Feeding behavior
Based on video observation, it was observed that the time taken for the first fish to locate the bait after feeding was approximately 1-2 seconds in both the control and test groups. However, except for the feeding duration on day 1 and day 7 of noise exposure, the control group exhibited shorter feeding times compared to the test group (Figure 8). The total feeding time in the test group was longer than that in the control group (62 seconds/day vs 43 seconds/day). The noise stimulation had a specific impact on the feeding time of the black rockfish, although the difference was not statistically significant (p > 0.05). In the test group, as the bait substrates were not completely consumed, it extended the feeding time significantly. During the recovery stage, only one fish in the experimental group was able to locate the bottom bait on the first day, and from the second day onwards, none of the fish in the experimental group were able to see the bait at the bottom of the tank, resulting in the feeding time being unable to be recorded. In contrast, the control group was able to consume all the bait within 3 days, and there was a significant difference between the control and test groups (p < 0.01). These findings suggest that the noise exposure affected the feeding behavior of the black rockfish, leading to prolonged feeding time and difficulties in locating the bait during the recovery period.
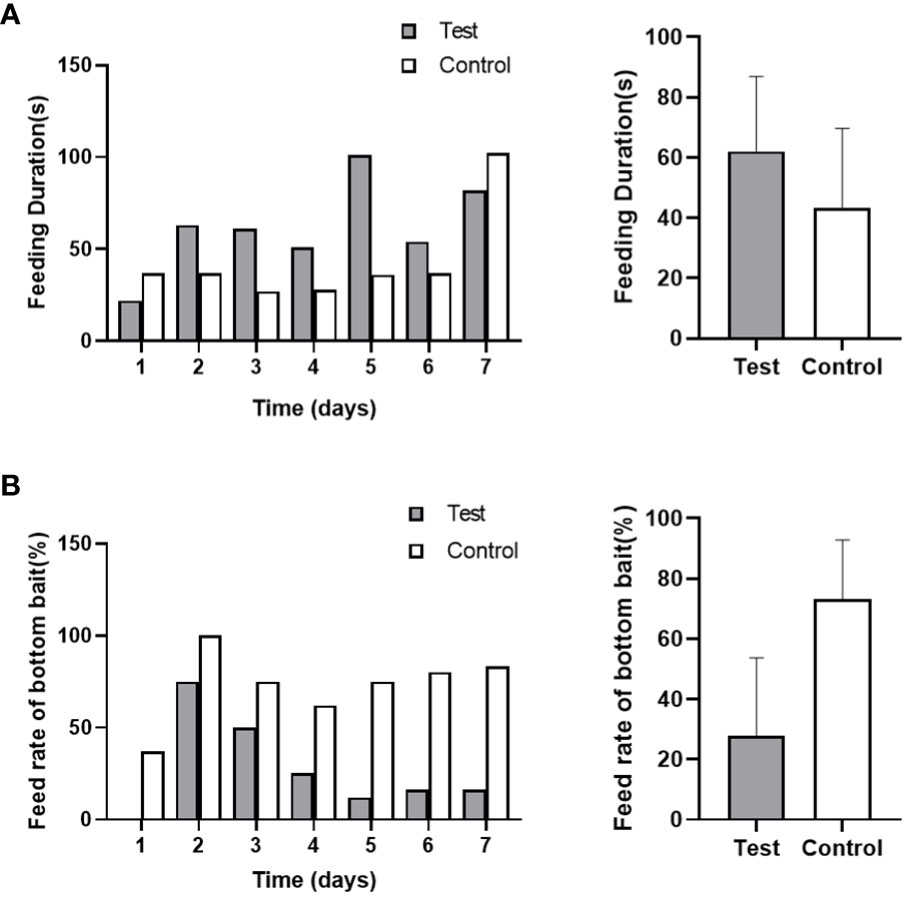
Figure 8 The feed duration (A) and feeding rate of black rockfish on bottom bait (B) after exposure to 200 Hz noise (110 dB) for 7 days (A, p>0.05; B, p<0.01).
4 Discussion
In this study, the best hearing sensitivity of black rockfish was observed at 200 Hz, with a mean auditory threshold of 86.4 ± 3.4 dB re 1μPa. Figure 9 compares three different approaches (behavior, electrocardiogram ECG, auditory evoked potentials AEP) for determining auditory thresholds of black rockfish (Ishizaki et al., 1992; Keiichiro, 1997). The general trends of the three methods were found to be similar. At 100 Hz, the auditory threshold was 92.1 dB, which was 0.1 dB higher than the ECG method and 1.5 dB higher than the behavioral method. At 200 Hz, the auditory threshold was 86.4 dB, which was 4.1 dB lower than the ECG method and 6 dB lower than the behavioral method. At 300 Hz, the auditory threshold was 98 dB, which was 4 dB lower than the ECG method and 8.4 dB lower than the behavioral method. At 500 Hz, the auditory threshold was 106 dB, which was 14.3 dB lower than the ECG method and 10.6 dB lower than the behavioral method. AEP detects synchronous neural activity in the 8th cranial nerve and brainstem auditory nuclei elicited by sound at the surface of the skull. One advantage of the AEP method over behavioral or ECG methods is that it is not affected by the condition or feeding motivation of the fish. The speed and ease with which AEP audiometry can be performed and the fact that there is no need for lengthy and repetitive subject conditioning, make it applicable to studies of hearing sensitivity in fish (Kojima et al, 2005; Ladich and Fay, 2013).
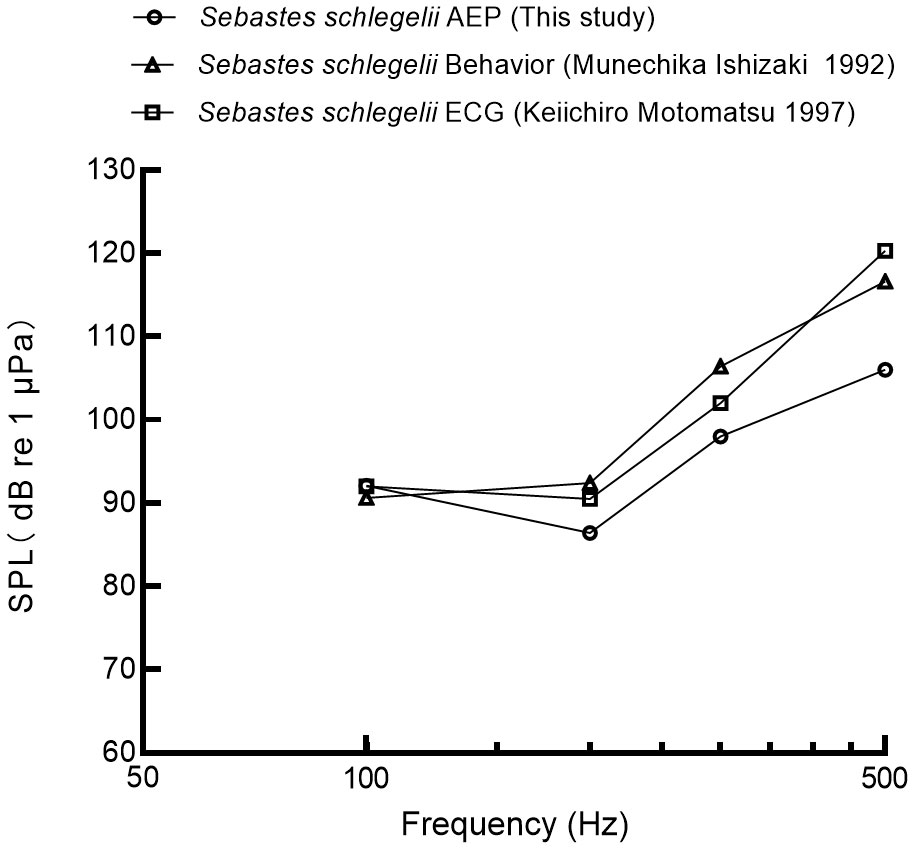
Figure 9 The auditory threshold of black rockfish was measured by different publications (Ishizaki et al., 1992; Motomatsu et al., 1997).
This study provides the first evidence of Noise-Induced Hearing Loss in black rockfish based on changes in auditory sensitivity. After 3 days of noise exposure, the black rockfish exhibited a significant increase in TTS, indicating a temporary shift in their hearing thresholds. However, after 7 days, this shift started to decrease, suggesting that as the duration of noise exposure extends, black rockfish exhibit a potential gradual adaptation to the deleterious effects of noise. After 3 days of noise exposure, the best frequency of black rockfish changed from 200 to 300 Hz. Similar findings have been reported in other studies. For example, Scholik and Yan (2002) examined the effects of white noise and boat noise on Pimephales promelas and found a change in the best frequency from 1000 to 300 Hz. Liu et al. (2013) exposed Myxocyprinus asiaticus to ship noise and observed a change in the best frequency from 800 to 200 Hz. These differences in findings may be attributed to variations in noise SPL, bandwidth, and species-specific characteristics. In general, fish species with limited auditory sensitivity (without Weberian apparatus) are less affected by noise. The auditory threshold tends to decrease as the duration of the recovery period increases. Breitzler et al. (2020) measured zebrafish exposed to white noise at different SPLs and found that recovery function occurred within 7 days for fish exposed to 130 dB and 140 dB noise levels, while fish subject to 150 dB only returned to baseline thresholds after 14 days. Further research is needed to determine the time required for the auditory threshold of black rockfish to recover to baseline levels.
Fish behavior research plays a crucial role in noise studies, as fish exhibit various behavioral responses to anthropogenic disturbances in aquatic environments. Changes in swimming activities, tail movements, and foraging behavior have been used as indicators of stress in fish exposed to human-generated disturbances (Mickle and Higgs, 2017; Weilgart, 2018; Faria et al., 2019). Based on the trajectories of black rockfish observed in this study, it was concluded that the fish spent more time irregularly swimming on the sidewalls of the tank, both with and without exposure to 200 Hz noise. Black rockfish are demersal marine fish that typically live in groups on rocky reefs, and their behavior may be influenced by their natural habitat. The onset of underwater sound propagation elicits startled reactions in some juvenile black rockfish, consistent with the findings reported by Spiga et al. (2017). The tail beat frequency (TBF) and swimming time (ST) of the control group were higher than those of the treatment group on days 2 and 3. From the video observations, it appeared that black rockfish tended to remain still and seek cover for extended periods when under constant threat. We hypothesize that fish may undergo a transition from an initial phase to an adaptive phase. During the initial phase, it is plausible that fish would employ strategies to minimize their interactions with noise stimulation, potentially resulting in a decrease in TBF and ST. These strategies may include seeking refuge or escaping from the noise source. However, from the fourth day onwards, the TBF and ST of the treatment group surpassed those of the control group. Significant differences were observed between the treatment and control groups (*p<0.05, **p<0.01). Following prolonged exposure to noise stimulation, it is conceivable that fish could enter an over-adaptation phase. Within this phase, fish may gradually readjust their swimming behavior, leading to an increase in TBF and ST. This phenomenon may arise from the fish attaining a state of equilibrium in their adaptation to the noise or undergoing behavioral modifications to enhance resource utilization or cope with environmental pressures. These behaviors, such as increased tail beat frequency and swimming time, were likely selected as escape strategies. It is worth noting that tail beat consumes energy in fish and can potentially affect their growth rate. Furthermore, in quiet tanks, black rockfish exhibited more frequent and variable gaping behavior compared to fish in loud tanks. During the 7-day experimental period, the number of gaping behavior in the noise-exposed group was recorded as 60, whereas the control group exhibited 74. Both groups displayed a peak occurrence of gaping behavior during the nighttime period, followed by the morning period, and the lowest frequency was observed during the midday interval. Limited observations on gaping behavior in fish in the scientific literature suggest that gaping is associated with low activity levels (Rasa, 1971), which aligns somewhat with the results of this study, indicating less frequent adjustments in animals in quiet tanks (Andersson, 2011). Overall, these findings demonstrate the impact of noise on black rockfish behavior, highlighting the manifestation of stress and anxiety-related responses. The altered swimming distance, increased tail beat frequency, and modified gaping behavior indicate the adaptive strategies employed by the fish in response to the noise stress.
Stressors appear to cause shifts, lapses and narrowing of attention, and can also influence decision speed, which may explain the increase in foraging errors observed in the test group of black rockfish (Mendl, 1999; De Kloet et al., 1999). Interestingly, the black rockfish in the test group displayed rapid reactions to the baits but exhibited specific behaviors such as swimming in the vertical direction and not consuming the baits that fell to the bottom of the tank, especially during the recovery phase. These observations indicate altered feeding behavior and increased avoidance responses in black rockfish under noise conditions. The longer duration of feeding and lower feeding rate observed in the noise-exposed black rockfish align with the findings reported by Mickle and Higgs (2017) and the conclusions drawn by Sabet et al. (2016). Exposure to elevated noise levels can impair foraging behavior through various mechanisms, which are not mutually exclusive. Firstly, noise can trigger stress or fear-related responses in the fish, affecting their feeding behavior. Secondly, noise can act as a distraction, diverting the fish’s attention away from the feeding task. Lastly, noise can mask important acoustic information that the fish relies on for successful foraging (Voellmy et al., 2014). The reduced foraging strikes and increased feeding duration observed in black rockfish are consistent with the concept of a defense cascade, where ongoing activities, such as foraging, are interrupted. Such responses are typically associated with stressors and fear-inducing stimuli (Metcalfe, Huntingford, & Thorpe, 1987). These behavioral changes indicate that the black rockfish perceive the noise as a threat and exhibit altered feeding strategies as a defensive response. In summary, the impaired foraging behavior, increased feeding duration, and avoidance responses observed in black rockfish exposed to noise are in line with previous research. The effects of noise on feeding behavior can be attributed to stress-related responses, distraction, and the masking of acoustic cues necessary for successful foraging.
Indeed, the research period of this experiment was relatively short, and long-term subsequent studies will provide further insights into the auditory recovery of black rockfish in response to noise. The dominant frequency range of OWF noise falls within the auditory sensitivity range of black rockfish, but it’s crucial to recognize that noise levels can vary significantly depending on the turbine power. Therefore, comprehensive field studies are needed to assess the actual impact of OWF noise on fish behavior and its implications for their fitness (Madsen et al., 2006). This research should include realistic noise simulations, considering key factors such as sound pressure levels and particle motion. By conducting such studies and accumulating more data, researchers will be better equipped to provide evidence-based recommendations and guidelines for mitigating the potential impacts of OWF noise on fish.
5 Conclusion
The results of this study emphasize the impact of anthropogenic noise on juvenile black rockfish, particularly in relation to their auditory threshold, swimming distance, and foraging behavior. The findings indicate that the minimum auditory threshold of black rockfish is 200 Hz, with a sound intensity of 86.4 ± 3.4 dB re 1μPa. Following exposure to 200 Hz noise, the most sensitive frequency for black rockfish shifted from 200 Hz to 300 Hz. Furthermore, we observed that continuous noise stimulation increased the swimming distance, swimming time, and tail beat frequency of juvenile black rockfish. Concurrently, the noise also had detrimental effects on their foraging behavior, potentially impacting their ability to effectively search for and capture food. These findings provide mounting evidence that anthropogenic noise can have wide-ranging effects on marine species, including fish.
Funding
The author(s) declare financial support was received for the research, authorship, and/or publication of this article. This work was funded by National Key Research and Development Program of China (Project No. 2019YFD0901003) and Fundamental Research Funds for the Central Universities (Project No. 202161063).
Acknowledgments
We thank Shengcong Liu for the collection of black rockfish at Dalian Tianzheng Industrial Co., Ltd and Chenxu Zhang and Ankang Xu for assistance with the data acquisition.
Conflict of interest
The authors declare that the research was conducted in the absence of any commercial or financial relationships that could be construed as a potential conflict of interest.
Publisher’s note
All claims expressed in this article are solely those of the authors and do not necessarily represent those of their affiliated organizations, or those of the publisher, the editors and the reviewers. Any product that may be evaluated in this article, or claim that may be made by its manufacturer, is not guaranteed or endorsed by the publisher.
References
Amoser S., Ladich F. (2003). Diversity in noise-induced temporary hearing loss in otophysine fishes. J. Acoustical Soc. America 113, 2170–2179. doi: 10.1121/1.1557212
Andersson M. H. (2011). “OWFs-ecological effects of noise and habitat alteration on fish,” (Doctoral dissertation, Department of Zoology, Stockholm University).
Andersson M. H., Dockåkerman E., Ubralhedenberg R., Ohman R., Marcus C., Sigray P. (2007). Swimming Behaviour of Roach (Rutilus rutilus) and Three-spined Stickleback (Gasterosteus aculeatus) in Response to Wind Power Noise and Single-tone Frequencies. Ambio 36, 636–638. doi: 10.1579/0044-7447(2007)36[636:SBORRR]2.0.CO
Andrew T., Francis E., Charles M., Naigaga I., Jessica N., Micheal O., et al. (2016). Mercury concentration in muscle, bellyfat and liver from Oreochromis niloticus and Lates niloticus consumed in Lake Albert fishing communities in Uganda. Cogent Food Agric. 2 (1), 1214996. doi: 10.1080/23311932.2016.1214996
Betke K., Glahn S. V., Matuschek R. (2005). Underwater noise emissions from offshore wind turbines. Pro CFA/DAGA 18, 25–30. Available at: https://pub.dega-akustik.de/DAGA_1999-2008/data/articles/001773.pdf (Accessed March, 2004).
Blew J., Hoffmann M., Nehls G., Hennig V. (2008). BioConsult SH report: Investigations of the bird collision risk and the responses of harbour porpoises in the offshore wind farms Horns Rev, North Sea, and Nysted, Baltic Sea. Available at: https://tethys.pnnl.gov/sites/default/files/publications/Bird_Collision_Risk_and_the_Responses_of_Harbour_Porpoi.pdf (Accessed 2008).
Breitzler L., Ieng H. L., Fonseca P. J., Vasconcelos R. (2020). Noise-induced hearing loss in zebrafish: investigating structural and functional inner ear damage and recovery. Hearing Res. 391, 107972. doi: 10.1016/j.heares.2020.107952
Codarin A., Wysocki L. E., Ladich F., Picciulin M., Lidia E. (2009). Effects of ambient and boat noise on hearing and communication in three fish species living in a marine protected area (Miramare, Italy). Mar. Pollut. Bull. 58, 1880–1887. doi: 10.1016/j.marpolbul.2009.07.011
De Jong C. A. F., Ainslie M. A., Blacquière G. (2011). TNO report: Standard for measurement and monitoring of underwater noise, Part II: procedures for measuring underwater noise in connection with offshore wind farm licensing. Available at: https://users.ece.utexas.edu/~ling/2A_EU1.pdf (Accessed September, 2011).
De Kloet E., Melly R., Oitzl S., Joëls M. (1999). Stress and cognition: are corticosteroids good or bad guys? Trends Neurosci. 22, 422–426. doi: 10.1016/S0166-2236(99)01438-1
Faria M., Vall A., Prats E., Bedrossiantz J., Orozco M., Porta J., et al. (2019). Further characterization of the zebrafish model of acrylamide acute neurotoxicity: gait abnormalities and oxidative stressSci. Rep. 9(1), 7075. doi: 10.1038/s41598-019-43647-z
Ishizaki M., Hiraishi T., Yamamoto K., Nashimoto K. (1992). Auditory threshold of black rockfish. Nsugaf 58, 55–61. doi: 10.2331/suisan.58.55
Jones I. T., Stanley J. A., Aran Mooney T. (2020). Impulsive pile driving noise elicits alarm responses in squid (Doryteuthis pealeii). Mar. Pollut. Bull. 150, 110792. doi: 10.1016/j.marpolbul.2019.110792
Keiichiro M. (1997). Auditory threshold and masking in black rockfish sebastes schlegeli. Nippon Suisan Gakkaishi 63, 110–111. doi: 10.2331/suisan.63.110
Kenyon T. N., Ladich F., Yan H. Y. (1998). A comparative study of hearing ability in fishes: the auditory brainstem response approach. J. Comp. Physiol. A-neuroethology Sensory Neural Behav. Physiol. 182, 307–318. doi: 10.1007/s003590050181
Kojima T., Ito H., Komada T., Taniuchi T., Akamatsu T. (2005). Measurements of auditory sensitivity in common carp Cyprinus carpio by the auditory brainstem response technique and cardiac conditioning method. Fisheries Sci. 71, 95–100. doi: 10.1111/j.1444-2906.2005.00935x
Lacroix D., Sylvain P. (2011). The multi-use in wind farm projects: more conflicts or a win-win opportunity? Aquat. Living Resources. 24, 129–135. doi: 10.1051/alr/2011135
Ladich F., Fay R. (2013). Auditory evoked potential audiometry in fish. Springer Open Choice 23, 317–364. doi: 10.1007/s11160-012-9297-z
Likang L., Wen H., Yun L., Jifang L., Simin Z., Min S., et al. (2018). Deep transcriptomic analysis of black rockfish (Sebastes schlegelii) provides new insights on responses to acute temperature stress. Sci. Rep. 8, 9113. doi: 10.1038/s41598-018-27013-z
Lindell H. (2003). Ingemansson report: Utgrunden off-shore wind farm- Measurements of underwater noise. Available at: https://mhk.pnnl.gov/sites/default/files/publications/Utgrunden_Underwater_Noise_2003.pdf (Accessed June 17, 2003).
Liu M., Wei Q. W., Du H., Fu Z. Y., Chen Q. C. (2013). Ship noise-induced temporary hearing threshold shift in the Chinese sucker Myxocyprinus asiaticus (Bleeke). J. Appl. Ichthyology 29, 1416–1422. doi: 10.1111/jai.12345
Madsen P. T., Wahlberg M., Tougaard J., Lucke K., Tyack P. L. (2006). Wind turbine underwater noise and marine mammals: Implications of current knowledge and data needs. Mar. Ecol. Prog. Ser. 309, 279–295. doi: 10.3354/meps309279
Marmo B., Roberts I., Buckingham M. P., King S., Booth C. (2013). Scottish Marine and Freshwater Science report: Modelling of Noise Effects of Operational Offshore Wind Turbines including noise transmission through various foundation types. Available at: https://data.marine.gov.scot/dataset/modelling-noise-effects-operational-offshore-wind-turbines-including-noise-transmission-0 (Accessed November 17, 2014).
Mendl M. (1999). Performing under pressure: stress and cognitive function. Appl. Anim. Behav. Sci. 65, 221–244. doi: 10.1016/S0168-1591(99)00088-X
Metcalfe N. B., Huntingford F. A., Thorpe J. E. (1987). The influence of predation risk on the feeding motivation and foraging strategy of juvenile Atlantic salmon. Anim. Behav. 35 (3), 901–911. doi: 10.1016/S0003-3472(87)80125-2
Mickle M. F., Higgs D. M. (2017). Integrating Techniques: a review of the effects of anthropogenic noise on freshwater fish. Can. J. Fisheries Aquat. Science. 75, 217–245. doi: 10.1139/cjfas-2017-0245
Motomatsu K., Hiraishi T., Yamamoto K., Naishimoto K. (1997). Auditory Threshold and Masking in Black Rockfish Sebastes schlegeli. Nihon Suisan Gakkaishi 63 (1), 110–111. doi: 10.2331/suisan.64.792
Nedwell J., Langworthy J., Howell D. (2003) COWRIE report: Assessment of sub-sea acoustic noise and vibration from offshore wind turbines and its impact on marine wildlife; initial measurements of underwater noise during construction of offshore windfarms, and comparison with background noise. Available at: https://users.ece.utexas.edu/~ling/2A_EU1.pdf (Accessed May, 2003).
Nedwell J., Langworthy J., Howell D. (2004). COWRIE report: Mesurement of underwater noise during construction of offshore wind farm, and comparaison with background noise. Available at: https://tethys.pnnl.gov/sites/default/files/publications/COWRIE_Noise_Report_Draft.pdf (Accessed 2004).
Netherlands Minstry of Infrastructure and the Environment. (2011). TNO report: Standard for measurement and monitoring of underwater noise, Part II. procedures for measuring underwater noise in connection with OWF licensing. Available at: https://tethys.pnnl.gov/sites/default/files/publications/TNO-Report-2011.pdf (Accessed September 25, 2011).
Popper A. N., Nancy L., Clarke (1976). The auditory system of the goldfish (Carassius auratus): effects of intense acoustic stimulation. Comp. Biochem. Physiol. Part A: Physiol. 53, 11–18. doi: 10.1016/S0300-9629(76)80003-5
Rasa (1971). The causal factors and function of ‘Yawning’ in Microspathodon chrysurus (Pisces: Pomacentridae). Behaviour 39, 39–57. doi: 10.1163/156853971X00168
Russell D. F., Tucker A., Wettring B. A., Neiman A. B., Wilkens L., Moss F. (2001). Noise effects on the electrosense-mediated feeding behavior of small paddlefish. Fluctuation Noise Lett. 1 (02), L71–L86. doi: 10.1142/S021947750100024X
Sabet S. S., Wesdorp K., Campbell J., Snelderwaard P., Slabbekoorn H. (2016). Behavioural responses to sound exposure in captivity by two fish species with different hearing ability. Anim. Behav. 116, 1–11. doi: 10.1016/j.anbehav.2016.03.027
Sara G., Dean J. M., D’Amato D., Buscaino G., Oliveri A., Genovese S., et al. (2007). Effect of boat noise on the behaviour of bluefin tuna Thunnus thynnus in the Mediterranean Sea. Mar. Ecol. Prog. Ser. 331, 243–253. doi: 10.3354/meps331243
Scholik A. R., Yan H. Y. (2002). Effects of boat engine noise on the auditory sensitivity of the fathead minnow, pimephales promelas. Environ. Biol. Fishes 63, 203–209. doi: 10.1023/A:1014266531390
Slabbekoorn H., Bouton N., Opzeeland I. V., Coers A., Cate C., Popper A. N. (2010). A noisy spring: the impact of globally rising underwater sound levels on fish. Trends Ecol. Evol. 25, 419–427. doi: 10.1016/j.tree.2010.04.005
Smith M. E., Kane A. S., Popper A. N. (2004). Acoustical stress and hearing sensitivity in fishes: does the linear threshold shift hypothesis hold water? J. Exp. Biol. 207, 3591–3602. doi: 10.1242/jeb.01188
Spiga I., Aldred N., Gary S., Caldwell (2017). Anthropogenic noise compromises the anti-predator behaviour of the European seabass, Dicentrarchus labrax (L.). Mar. Pollut. Bull. 122, 297–305. doi: 10.1016/j.marpolbul.2017.06.067
Stevens E., Don (1979). The effect of temperature on tail beat frequency of fish swimming at constant velocity. Can. J. Zoology 57, 1628–1635. doi: 10.1139/z79-214
Subacoustech Ltd. (2003). COWRIE report: Assessment of sub-sea acoustic noise and vibration from offshore wind turbines and its impact on marine wildlife. Available at: https://www.mendeley.com/catalogue/a7794cec-bdf8-3574-a332-67d20afe4ef3/ (Accessed Accessed: May 3, 2003).
Subacoustech Ltd. (2004). COWRIE report: A review of offshore windfarm related underwater noise sources. Available at: https://tethys.pnnl.gov/publications/review-offshore-windfarm-related-underwater-noise-sources (Accessed October 1, 2004).
Thompson P. M., Graham I. M., Cheney B., Barton T. R., Merchant N. D. (2020). Balancing risks of injury and disturbance to marine mammals when pile driving at OWFs. Ecol. Solutions Evidence 1, e12034. doi: 10.1002/2688-8319.12034
Tougaard J., Henriksen O. D., Miller L. A. (2009). Underwater noise from three types of offshore wind turbines: estimation of impact zones for harbor porpoises and harbor seals. J. Acoustical Soc. America 125, 3766–3773. doi: 10.1121/1.3117444
Tougaard J., Hermannsen L., Madsen P. T. (2020). How loud is the underwater noise from operating offshore wind turbines? J. Acoustical Soc. America 148, 2885–2893. doi: 10.1121/10.0002453
Tullio G., Mariani P., Benassai G., Luccio D. D., Grieco L. (2018). Sustainable use of marine resources through offshore wind and mussel farm co-location. Ecol. Model. 367, 34–41. doi: 10.1016/j.ecolmodel.2017.10.012
Velasquez J., Laura E., Mark I. (2020). Vessel noise affects routine swimming and escape response of a coral reef fish. PloS One 15, e0235742. doi: 10.1371/journal.pone.0235742
Voellmy I. K., Purser J., Flynn D., Kennedy P., Simpson S. D., Radford A. N. (2014). Acoustic noise reduces foraging success in two sympatric fish species via different mechanisms. Anim. Behav. 89, 191–198. doi: 10.1016/j.anbehav.2013.12.029
Weilgart L. (2018). OceanCare report: The impact of ocean noise pollution on fish and invertebrates. 2018. In: Report for oceancare. Available at: https://thegreentimes.co.za/wp-content/uploads/2022/01/impact-of-ocean-noise-pollution-on-fish-and-invertebrates.pdf (Accessed May 1, 2018).
Wever L., Krause G., Buck B. H. (2015). Lessons from stakeholder dialogues on marine aquaculture in OWFs: Perceived potentials, constraints and research gaps. Mar. Policy 51, 251–259. doi: 10.1016/J.MARPOL.2014.08.015
Keywords: hearing sensitivity, auditory evoked potential, temporary threshold shift, behavior, fish welfare
Citation: Wang Y, Huang L and Xing B (2023) Experimental study on the effect of sound stimulation on hearing and behavior of juvenile black rockfish (Sebastes schlegelii). Front. Mar. Sci. 10:1257473. doi: 10.3389/fmars.2023.1257473
Received: 12 July 2023; Accepted: 10 November 2023;
Published: 05 December 2023.
Edited by:
Pablo Almazan Rueda, National Council of Science and Technology (CONACYT), MexicoReviewed by:
Zonghang Zhang, Shantou University, ChinaAna C. Puello Cruz, National Council of Science and Technology (CONACYT), Mexico
Copyright © 2023 Wang, Huang and Xing. This is an open-access article distributed under the terms of the Creative Commons Attribution License (CC BY). The use, distribution or reproduction in other forums is permitted, provided the original author(s) and the copyright owner(s) are credited and that the original publication in this journal is cited, in accordance with accepted academic practice. No use, distribution or reproduction is permitted which does not comply with these terms.
*Correspondence: Liuyi Huang, huangly@ouc.edu.cn