Sharks and their relatives: can their past help predict their future?
- 1College of Science and Engineering, James Cook University, Townsville, QLD, Australia
- 2Marine Climate Change Unit, Okinawa Institute of Science and Technology (OIST), Onna-Son, Japan
Elasmobranchs (i.e., sharks, skates, and rays) have survived five mass extinction events and changed relatively little throughout their ~450-million-year evolutionary history. Therefore, elasmobranchs may provide critical evolutionary perspectives on how species and populations can elicit phenotypic plasticity and adaptation responses to climate change. Unfortunately, despite their roles as critical apex- and meso-predators, most elasmobranch species are considered to be highly vulnerable to the impacts of fisheries exploitation and climate change, which is compounded by their K-selected life history strategies. Furthermore, the future of elasmobranchs is uncertain at best in the face of anthropogenic climate change because there have only been a handful of studies that have directly investigated the effects of climate change related stressors. Phenotypic plasticity in response to climate change, specifically ocean warming, may be a species’ best chance of resilience given the expedited rate of environmental change. However, despite extensive research on plasticity within and across generations in teleost fishes, there remains a knowledge gap for elasmobranch species, owing to their extended life spans and delayed sexual maturity. Here, we present four case studies on different elasmobranch species to lend perspectives on the capacity for phenotypic plasticity within the context of ocean warming. Furthermore, we discuss potential research avenues and modern technologies that may enable future investigations to empirically explore the capacity for phenotypic plasticity in elasmobranchs.
1 Introduction
Redistribution to optimal thermal habitats and phenotypic plasticity and/or adaptation to maintain performance and fitness have been documented as the primary means to circumvent climate change (Donelson et al., 2019; Vilmar and Di Santo, 2022). The ability of a species to employ these processes is often considered in relation to their ecology, with movement and connectivity playing a substantial role. For example, some level of mobility will be required for species to take advantage of redistribution and range shifts (Bates et al., 2014). Furthermore, movement of individuals may allow rapid response to environmental change and even maintain stable internal conditions (i.e., behavioral thermoregulation; Papastamatiou et al., 2015). For site-attached species, the capacity for physiological plasticity is key to responding to environmental change (Fox et al., 2019). Whereas phenotypic plasticity is the ability of individual genotypes to produce various phenotypes (Ghalambor et al., 2007), adaptation is a population level process by which a species increases its fitness and is the result of natural selection acting upon heritable variation over two or more generations (Fraser et al., 2011). How adaptation has occurred in the past can help predict future responses. For example, whether local adaptation to historical conditions has occurred, as determined by selective forces and connectivity between diverse habitats, may influence the capacity for future adaptation.
Elasmobranch fishes (i.e., sharks, skates, and rays) have changed relatively little throughout their ~450-million-year evolutionary history and have survived the world’s five mass extinction events (Kriwet et al., 2008); consequently, using the past to predict the future may be highly relevant for these species. Unfortunately, there have been only a few studies on elasmobranchs within a climate change context (Rummer et al., 2022), and even fewer have directly assessed phenotypic plasticity of physiological traits within these species (Tullis and Baillie, 2005; Rytkönen et al., 2012; Devaux et al., 2019). This paucity of information highlights critical knowledge gaps in understanding how elasmobranchs will respond to future climate change. This is particularly concerning, considering the vital roles that elasmobranchs fulfill as apex- and meso-predators within the world’s marine ecosystems. To lend perspective, we first lay the theoretical foundation for phenotypic plasticity as a response to environmental change, outline types of plasticity, and discuss conditions under which they are expected. We then explore how the expectations of plasticity types relate to elasmobranch ecology, utilizing a series of case studies to highlight species that may fit various plasticity expectations under ocean warming. Following this, we utilize the knowledge base of physiological plasticity from teleost fish studies to lend perspective on how physiological plasticity may occur within elasmobranchs and suggest future research initiatives for this taxon.
2 Phenotypic plasticity
For many species, plasticity is expected to play a significant role in persistence, because the rate of environmental change is likely to surpass the rate at which many species may be able to adapt (Visser, 2008). The nature of environmental heterogeneity and predictability influences whether and what type of plasticity occurs, including the magnitude, duration, and thresholds of environmental cues that elicit plastic responses. There are three primary forms of phenotypic plasticity: reversible plasticity (RP), developmental plasticity (DP), and transgenerational plasticity (TGP) (Leimar and McNamara, 2015; Donelson et al., 2019); however, these forms are not mutually exclusive. Here, we define TGP as an inclusive term (including carryover effects and parental effects) that does not require the interaction between past and current generation conditions (Bonduriansky et al., 2012). RP is a shift of performance within a relatively rapid temporal period in response to environmental variability (Beaman et al., 2016). Most RP responses reflect acclimation processes that occur from seasonal and/or diel variation and indicate the organism’s capacity to revert to previously experienced environmental conditions (Angilletta, 2009). RP is likely to be important in longer-lived species where environmental cues in early life or past generations may not be relevant for current environmental conditions (Munday et al., 2013). DP generally occurs in response to environmental conditions experienced during prezygotic to early juvenile developmental periods (Beaman et al., 2016). Moreover, DP is often expected when thermal environments are heterogenous, intergenerationally (Angilletta, 2009). TGP is expected to occur when conditions also vary intergenerationally but requires environmental predictability between generations. Methods of transfer can include nutrients, hormones, and mRNA (Angilletta, 2009), and TGP can occur in response to conditions experienced in the parent and previous generations. This need for predictability between generations can result in TGP being highly sensitive to the timing, duration, and magnitude of environmental influences experienced by past generations to establish beneficial phenotypes for the offspring’s environment (Munday et al., 2013; Donelson et al., 2018). Two crucial ontogenetic stages to facilitate TGP are the periods from fertilization to early development and the period centered around reproduction, because embryonic cells are more sensitive to environmental factors and have higher rates of epigenetic changes during these time periods (Burton and Metcalfe, 2014; Fawcett and Frankenhuis, 2015).
3 Plasticity for elasmobranchs
Previous studies on the potential for phenotypic plasticity in elasmobranchs have mainly focused on small, benthic species (e.g., Hemiscyllium ocellatum), particularly in relation to hypoxia/anoxia (Dowd et al., 2010; Hickey et al., 2012; Devaux et al., 2019); however, studies related to how these species compensate physiological traits to thermal changes in the context of climate change context are lacking. The thermal sensitivity of elasmobranchs and, consequently, the potential need for plasticity were reviewed by Pereira-Santos and colleagues (2021) and provided an overview of responses and knowledge gaps. This synthesis suggests that ocean warming will negatively affect elasmobranchs, regardless of climatic region, taxonomic order, lifestyle, or reproductive mode (Pereira-Santos et al., 2021). Some traits exhibit consistent patterns with warming, such as reductions in development time or increases in feeding, digestion, and metabolic rates (Papastamatiou et al., 2015; Wheeler et al., 2021). However, the eclectic range of effect size responses indicates that substantial diversity in thermal sensitivity exists (Pereira-Santos et al., 2021), and, for many species, plasticity will be critical.
Elasmobranchs are generally K-selected life history strategists and thus long-lived, slow-growing, sexually mature at a late age; have long reproductive cycles; and produce few, high-quality offspring (Conrath and Musick, 2012). As the oldest living vertebrates on Earth (Edwards et al., 2019), RP would seemingly be beneficial as individuals are likely to experience environmental change within their lifetime (Beaman et al., 2016). In addition, many species exhibit large home-ranges and migrations resulting in high potential for behavioral plasticity to optimize performance (Payne et al., 2018). This can arise in the form of behavioral thermoregulation, such as for whale sharks Rhincodon typus partitioning their time between the surface and deep water to allow behavioral warming at the surface (Thums et al., 2013). Alternatively, frequent movements can coincide with physiological capacity to thrive across a range of environmental conditions (e.g., bull shark Carcharhinus leucas; Huepel and Simpfendorfer, 2008). For species that exhibit site fidelity, environmental variation (i.e., range and stochasticity) relative to thermal performance will dictate whether physiological plasticity is necessary. Although most elasmobranchs are long-lived, some species have a maximum life expectancy of <20 years, similar to teleosts in which DP and TGP occur (Mejía-Falla et al., 2014). The key consideration is whether intergenerational shifts in environmental conditions are predictable to yield TGP or DP, but high levels of environmental stochasticity could instead result in bet-hedging (Shama, 2015). In the following, we outline four examples of species’ characteristics and expected plasticity outcomes in response to ocean warming.
3.1 Expected reversible behavioral plasticity
The tiger shark (Galeocerdo cuvier; Figure 1A) is a highly mobile species that is globally distributed throughout tropical and temperate latitudes (Papastamatiou et al., 2013). As such, temperature drives their movement patterns and abundance (Ferreira et al., 2015; Dicken et al., 2016). Indeed, mechanistic distribution models based on abundance, performance and movement data suggest 22°C as a persistent thermal optimum (Payne et al., 2018). Data from two satellite-tagged individuals lends further evidence of long-term behavioral thermoregulation in this species, given that they maintained 22°C year-round across 14° of latitude (Holmes et al., 2014; Payne et al., 2018). G. cuvier occasionally inhabit temperatures outside this thermal optimum in exchange for increased access to preferred prey, such as green sea turtles (Fitzpatrick et al., 2012). As the oceans warm, G. cuvier represents an example of behavioral plasticity, given that movements coincide with thermal gradients that optimize performance. Many elasmobranchs behaviorally thermoregulate to similar extents, and future expectation for such species would be poleward shifts and exploitation of thermal depth or current refuges (Papastamatiou et al., 2015). The risk for these species is if the environment shifts beyond their capacity to use behavior to maintain optimum body temperatures, as they likely lack capacity for physiological plasticity (Logan et al., 2019).
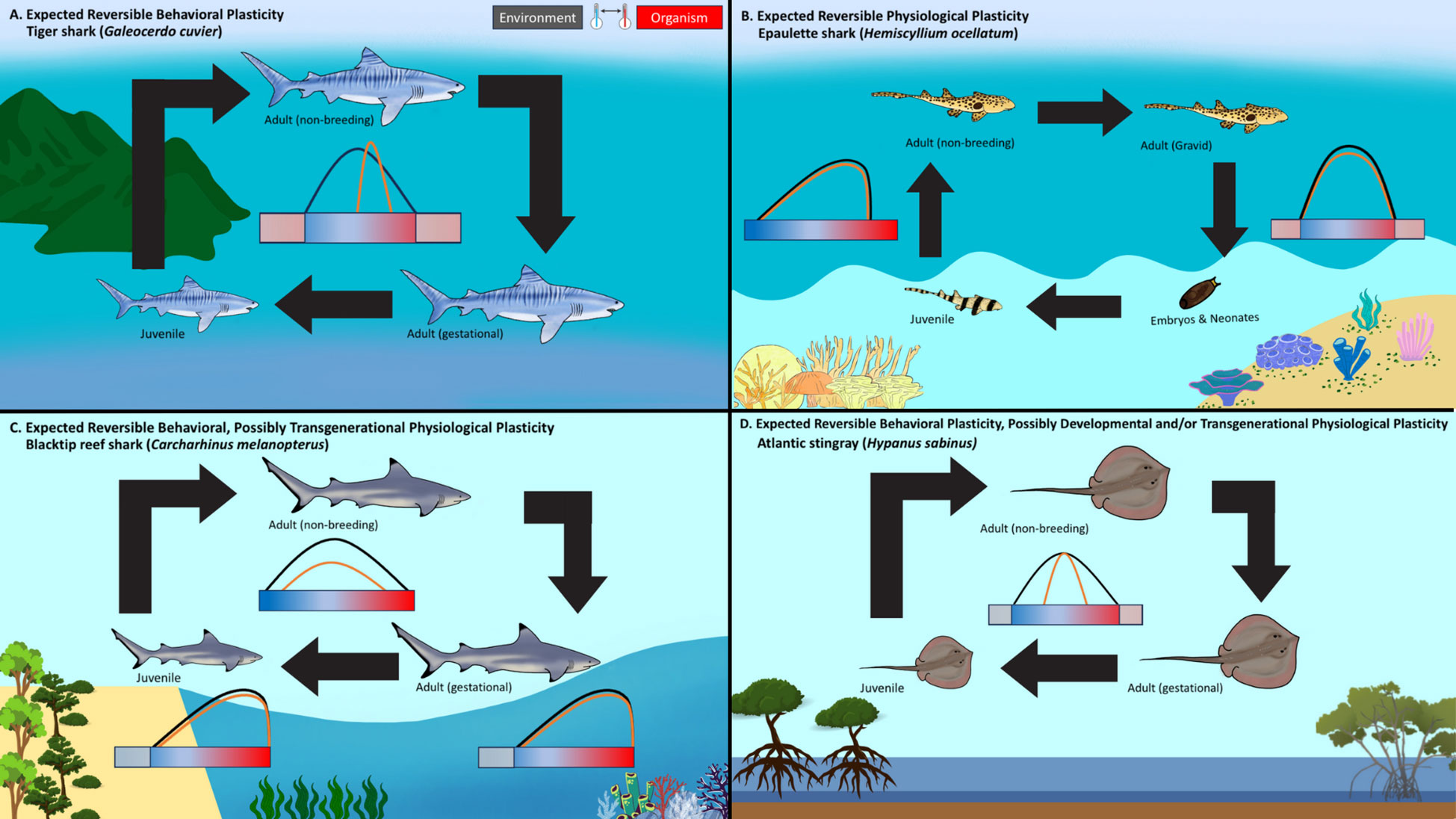
Figure 1 Expected pathways of phenotypic plasticity within four elasmobranch species [(A) Tiger Shark – Galeocerdo cuvier, (B) Epaulette shark – Hemiscyllium ocellatum, (C) Blacktip reef shark – Carcharhinus melanopterus, (D) Atlantic stingray – Hypanus sabinus across life history stages. The horizontal color gradient bar (i.e., blue and red) represents the thermal environmental variability that depends on the associated habitat. The black distribution curve indicates the frequency of environmental temperatures for each life stage. The orange distribution curve indicates the frequency of organismal body temperatures for each life stage, with a difference between the environment (i.e., black) indicating behavioral thermoregulation. (A) Galeocerdo cuvier represents a highly mobile, behavioral thermoregulation specialist that follows distinct temperature gradients around 22°C throughout its ontogeny to optimize performance within tropical and temperate waters. (B) Hemiscyllium ocellatum exhibits reversible physiological plasticity or tolerance, with non-breeding adults and juveniles encountering daily, drastic temperature fluctuations on the reef flats. Gravid adults presumably seek out more thermally stable, deeper waters to deposit their eggs within the reef structure, to conceal them from predators, and to protect them from the high temperatures present in the reef flat environments. (C) Carcharhinus melanopterus can utilize behavioral plasticity for much of their life; however, when females shift into shallow reef lagoons during gestational periods, they and their litter are exposed to highly variable temperatures. High site fidelity creates environmental homogeneity between parent and offspring that is conducive to TGP. (D) Hypanus sabinus elicits the capacity to behaviorally thermoregulate across life stages but also exhibits physiological tolerance to variable temperatures within inshore estuaries. Because this species shows both site fidelity and longer-range movements, there it also potential for both DP and TGP.
3.2 Expected reversible physiological plasticity
The epaulette shark (Hemiscyllium ocellatum; Figure 1B) is endemic to the Great Barrier Reef and coastal waters of northern Australia (Wise et al., 1998). This species is physiologically robust to low oxygen conditions (1.55 mg O2 L−1; Wise et al., 1998), elevated carbon dioxide (880 µatm; Heinrich et al., 2014; Heinrich et al., 2016), and thermal variation (Gervais et al., 2018; Wheeler et al., 2022), likely because of the environmental fluctuations that it routinely experiences in the shallow reef flats it inhabits. H. ocellatum encounters dramatic daily temperature fluctuations on a diurnal and seasonal basis (12°C; Potts and Swart, 1984). The lack of long-distance locomotor capabilities to pursue optimal thermal habitats and the relatively extreme environmental variability experienced would suggest high thermal tolerance and capacity for physiological plasticity as adults, which can be utilized in response to ocean warming (Nay et al., 2021). Some of these plastic responses may include compensation of metabolic traits, blood-oxygen carrying capacity, and mitigation of reactive oxygen species. Indeed, H. ocellatum has previously been observed to exhibit RP outcomes through compensatory mitochondrial and metabolic adjustments in response to hypoxic and/or anoxic stress (Dowd et al., 2010; Hickey et al., 2012; Rytkönen et al., 2012). The potential for DP within this species seems less likely, as acute warming conditions of 31°C during embryonic development resulted in reduced embryonic growth, decreased metabolic performance, and reduced size and body mass post-hatching (Wheeler et al., 2021). However, this sensitivity might also indicate that embryonic development occurs under more stable thermal conditions (e.g., reef crest, deeper depths) during the austral summer months (Huepel and Bennett, 1998; Wheeler et al., 2021).
3.3 Expected reversible behavioral plasticity and/or transgenerational plasticity
The blacktip reef shark (Carcharhinus melanopterus; Figure 1C) inhabits shallow coral reefs, sand flats of atolls, and high islands, and, occasionally, non-reef environments throughout the western Indo-Pacific (Mourier et al., 2013). Whereas C. melanopterus regularly exhibits site fidelity (8–12 km2; Papastamatiou et al., 2010), some coastal populations undergo larger movements to adjacent coastal habitats including offshore reefs (Chin et al., 2013; Mourier et al., 2013). This level of mobility allows some behavioral thermoregulation (Papastamatiou et al., 2015) but is not expected to result in perfect thermoregulation due to relatively high thermal safety margin in neonates (~6°C) above the summer average conditions (Bouyoucos et al., 2018; Bouyoucos et al., 2021). Species that entirely rely on behavioral thermoregulation are expected to maintain a relatively narrow thermal window; consequently, such species would likely be living close to their upper thermal limits.
Ontogenetic movements of C. melanopterus generally consist of heavily localized males and females mating during the summer and early autumn months, presumably within deeper fore reef environments (Chin et al., 2013) after which adult females shift to lagoons and remain for the 8-to 11-month gestation period (Mourier and Planes, 2013; Mourier et al., 2013). Upon parturition, neonates remain in the warmer, thermally variable lagoon nursery areas for the first years of development before moving to cooler, deeper fore reefs (Mourier et al., 2013). Shifts due to ontogeny or environmental conditions make DP less likely. Neonates and juveniles endure challenging habitat conditions in exchange for protection from predators (Knip et al., 2010; Bouyoucos et al., 2018; Bouyoucos et al., 2020; Bouyoucos et al., 2022). However, the high site fidelity and extended exposure of mother, embryos, and neonates to the same environmental conditions could create predictability between generations, thus promoting potential for TGP (Mourier and Planes, 2013; Beaman et al., 2016).
3.4 Expected reversible behavioral plasticity, possibly developmental, and/or transgenerational physiological plasticity
The Atlantic stingray (Hypanus sabinus; Figure 1D) is a euryhaline, viviparous species that resides predominately within western Atlantic inshore estuaries (Snelson et al., 1988). Adults and juveniles can inhabit inshore estuaries year-round and have profound physiological thermal tolerance, enduring temperatures from 0.7°C to 43°C (Fangue and Bennett, 2003; Wallman and Bennett, 2006). Pregnant females behaviorally thermoregulate during gestation to expedite embryonic development, preferring warmer temperatures (~26.1°C) than their non-pregnant counterparts that prefer 0.8°C cooler (Wallman and Bennett, 2006). Mating occurs during the spring, and parturition occurs during late summer (Snelson et al., 1988). Occasionally, H. sabinus may seasonally migrate to deeper, offshore waters during winter months (Snelson et al., 1988), likely indicating sub-optimal coastal temperatures during these time frames. Although H. sabinus has some capacity for behavioral thermal plasticity, it is unlikely sufficient to keep pace with the rate of climate change, especially given these highly variable shallow habitats can fluctuate ~10°C daily. Because H. sabinus can either reside in the same estuaries, creating predictability between generations, or migrate offshore, creating possible variation between generations if not returning to the same estuaries, TGP and DP may be possible as thermal conditions shift beyond optimal.
4 Mechanisms of physiological plasticity
Behavioral thermal plasticity influences environmental conditions experienced by individuals and thus acts upon lower levels of biological organization. Under future circumstances where behavior can no longer keep pace with environmental change or for less mobile species, physiological plasticity becomes critical. For elasmobranchs that employ physiological plasticity, preliminary studies investigating phenotypic plasticity in response to other environmental stressors as well as knowledge accumulated on potential mechanisms from teleost fishes can direct future research. At the organismal level, metabolic rates represent all processes encompassing the acquisition of energetic resources from the environment, assimilation, and utilization for fitness-enhancing processes, ultimately forming the mechanistic link between an organism’s fitness and environmental resources (Brown et al., 2004). Despite intrinsic links to the functional energetic capacity of organisms, high degrees of intraspecific phenotypic flexibility of metabolic rates in response to the environment remain (Norin and Metcalfe, 2019). For example, when exposed to warming, barramundi (Lates calcarifer) individuals with originally depressed metabolic attributes at control temperatures elicited greater reversible thermal plasticity at elevated temperature when compared to individuals initially exhibiting elevated metabolic attributes (Norin et al., 2016). Multi-generational research on a coral reef damselfish, Acanthochromis polyacanthus with limited dispersal ability, and thus possibly high environmental predictability between generations, suggests high capacity for TGP and mild DP of aerobic metabolism (Donelson and Munday, 2012). It is worth noting that the capacity for plasticity can differ between populations of the same species, likely due to differences in environmental heterogeneity (Donelson and Munday, 2012). Multi-generational experiments are exceptionally challenging for elasmobranchs, given their prolonged life histories, and TGP and DP are not likely if strong RP outcomes exist. Metabolic performance in elasmobranchs is expected to be thermally sensitive, as ATP production and blood-oxygen carrying capacity becomes inefficient (Bouyoucos et al., 2019).
The metabolic responses at the cellular level are largely influenced by the thermal sensitivities of mitochondria and the kinetic properties of metabolic enzymatic reactions (Pörtner, 2001; Pörtner, 2002; Pörtner et al., 2017; Chung and Schulte, 2020; Illing et al., 2020). Mitochondrial plasticity in response to temperature stress has been well documented, with studies identifying modified morphology, densities, and feedback functions of critical enzymes involved in cellular respiration (Tyler and Sidell, 1984; Blier et al., 2013; Pichaud et al., 2019). Conserved performance through compensation of cytochrome-c-oxidase and citrate synthase activities occurred when zebrafish (Danio rerio) embryos were warm-acclimated and then subsequently exposed to this same temperature as adults, indicating beneficial DP (Schnurr et al., 2014). RP of mitochondrial function was demonstrated in the tilapia (Oreochromis mossambicus), where fish acclimated to warmer conditions exhibited increased oxidative capacity (Schnell and Seebacher, 2008). Citrate synthase enzyme activities, which suggest a degree of biochemical compensation through RP, have been observed in white spotted bamboo sharks (Chiloscyllium plagiosum) acclimated to 30°C (Tullis and Baillie, 2005). In response to hypoxia, mitochondrial plasticity in H. ocellatum has been shown to reduce free radical production by decreasing succinate metabolism (Devaux et al., 2019). This could potentially serve to prevent reactive oxygen species production at elevated temperatures. Such ex situ techniques offer a means to explore thermal tolerance in species that are challenging to maintain in aquaria and/or for whole-organism testing. However, some enzymatic and mitochondrial function results may overestimate thermal tolerance in cases were the cardiovascular system fails (Pörtner et al., 2017).
Gene regulation has been shown to be a primary mechanism underpinning phenotypic plasticity (Bonduriansky et al., 2012; Duncan et al., 2014; Ryu et al., 2020). Elucidating the epigenetic states that drive resultant phenotypes can reveal the critical mechanistic nexus for understanding how ocean warming may contribute to the emergence of novel physiological phenotypes (Salinas et al., 2013; Fanter et al., 2022). For example, in A. polyacanthus, Veilleux et al. (2015) observed upregulation of genes related to metabolic function, stress, and immune responses with thermal DP and TGP, with the same genes differentially expressed. Further investigation determined that genes affiliated with metabolism, development, cardiovascular function, and heat shock responses also exhibited differentially methylated regions (Ryu et al., 2020). In H. ocellatum, exposure to hypoxia or anoxia leads to compensatory changes in the proteome, involving both structural and metabolic reorganization (Dowd et al., 2010). In addition, transcriptional responses have been observed (Rytkönen et al., 2012), implying a level of phenotypic flexibility that allows for compensation in the face of environmental stressors. Linking gene expression changes to levels of higher biological function is crucial for understanding phenotypic plasticity, as not all changes in gene expression necessarily lead to significant phenotypic changes. Currently, there are no molecular investigations into thermal plasticity in elasmobranchs, highlighting for future research in this area.
5 Future directions
Despite the vast knowledge gap regarding the necessity and capacity for thermal plasticity in elasmobranchs, given their long-life histories and logistical constraints for field and captive studies technologies are emerging such that theoretical predictions for this taxon can be tested. A candidate species approach to represent the diverse life histories, movement ecology, ontogeny, and reproductive modes would be ideal moving forward. Determining the level at which species rely on either behavior and physiology within a generation is a critical first step. This is especially important as behavior and physiology are intrinsically linked, and the reliance on one or the other influences the need for other forms of plasticity and the sensitivity to future climate change (Table 1). Species such as the Atlantic stingray, epaulette shark, and blacktip reef shark might be ideal candidates for experimental ideas outlined in Table 1, as they are amenable to captivity and ecologically, differing capacities and types of plasticity would be expected (Figure 1). Ultimately, evolutionary insights require empowering future studies through integrative, multidisciplinary approaches that utilize modern technological advances in genetics to bridge this gap (Donelson et al., 2023). It should be noted that this perspective has been written largely from the viewpoint of beneficial plasticity to respond adaptively to environmental change, focusing on warming. As climate change threatens ecosystems worldwide, it is critical to identify and understand the capacity for elasmobranchs to acclimate and adapt to shifting environmental conditions.
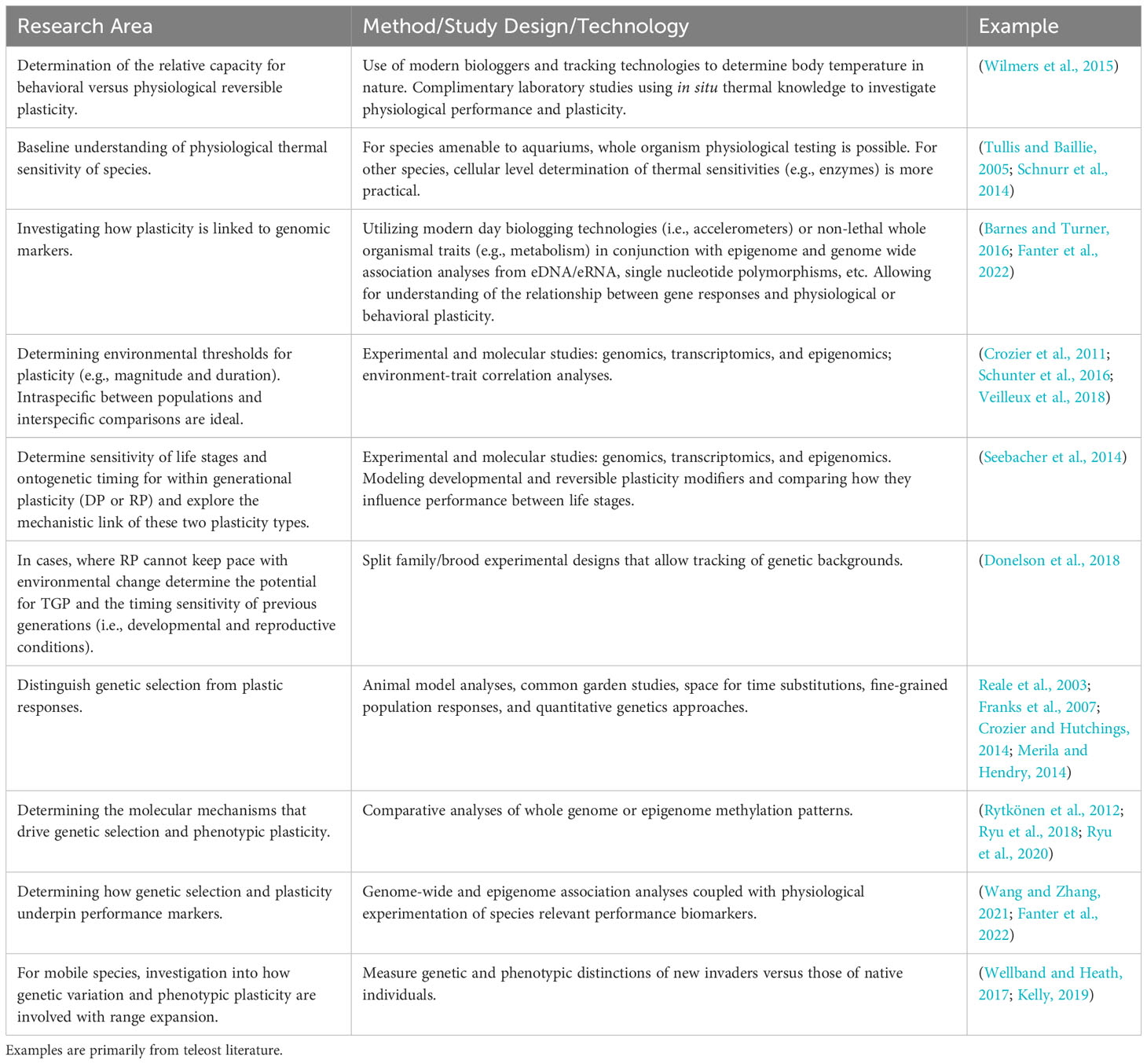
Table 1 Future research initiatives and technological advances that may help fill knowledge gaps regarding elasmobranchs’ potential for phenotypic plasticity and adaptation to environmental change.
Data availability statement
The original contributions presented in the study are included in the article/supplementary material. Further inquiries can be directed to the corresponding author.
Author contributions
AH: Conceptualization, Writing – original draft, Writing – review & editing. JD: Conceptualization, Writing – original draft, Writing – review & editing. JR: Conceptualization, Writing – original draft, Writing – review & editing. TR: Conceptualization, Writing – review & editing.
Funding
The author(s) declare financial support was received for the research, authorship, and/or publication of this article. AH is supported through a James Cook University post-graduate research scholarship and American Australian Association scholarship. JD is supported by an ARC Future Fellowship (FT190100015). TR is supported by the Okinawa Institute of Science and Technology (OIST).
Acknowledgments
The authors would like to thank Zainab Zulfikar Saria for her major contribution toward illustrating and constructing Figure 1 of this manuscript. The authors would like to thank the two reviewers for their constructive feedback, which greatly enhanced the quality of this manuscript.
Conflict of interest
The authors declare that the research was conducted in the absence of any commercial or financial relationships that could be construed as a potential conflict of interest.
Publisher’s note
All claims expressed in this article are solely those of the authors and do not necessarily represent those of their affiliated organizations, or those of the publisher, the editors and the reviewers. Any product that may be evaluated in this article, or claim that may be made by its manufacturer, is not guaranteed or endorsed by the publisher.
References
Angilletta M. J. Jr. (2009). “Chapter 5 thermal acclimation,” in Thermal adaptation: A theoretical and empirical synthesis (Oxford, United Kingdom: Oxford Academic), 126–156.
Barnes M. A., Turner C. R. (2016). The ecology of environmental DNA and implications for conservation genetics. Conserv. Genet. 17, 1–17. doi: 10.1007/s10592-015-0775-4
Bates A. E., Barrett N. S., Stuart-Smith R. D., Holbrook N. J., Thompson P. A., Edgar G. J. (2014). Resilience and signatures of tropicalization in protected reef fish communities. Nature. Climate Change 4, 62–67. doi: 10.1038/nclimate2062
Beaman J. E., White C. R., Seebacher F. (2016). Evolution of plasticity: mechanistic link between development and reversible acclimation. Trends Ecol. Evol. 31 (3), 237–249. doi: 10.1016/j.tree.2016.01.004
Blier P. U., Lemieux H., Pichaud N. (2013). Holding our breath in our modern world: will mitochondria keep the pace with climate changes? Can. J. Zoology 92 (7), 591–601. doi: 10.1139/cjz-2013-0183
Bonduriansky R., Crean A. J., Day T. (2012). The implications of nongenetic inheritance for evolution in changing environments. Evolutionary Appl. 5, 192–201. doi: 10.1111/j.1752-4571.2011.00213.x
Bouyoucos I. A., Morrison P. R., Weideli O. C., Jacquesson E., Planes S., Simpfendorfer C. A., et al. (2020). Thermal tolerance and hypoxia tolerance are associated in blacktip reef shark (Carcharhinus melanopterus) neonates. J. Exp. Biol. 223 (Pt 14). doi: 10.1242/jeb.221937
Bouyoucos I. A., Simpfendorfer C. A., Planes S., Schwieterman G. D., Weideli O. C., Rummer J. L. (2022). Thermally insensitive physiological performance allows neonatal sharks to use coastal habitats as nursery areas. Mar. Ecol. Prog. Ser. 682, 137–152. doi: 10.3354/meps13941
Bouyoucos I. A., Simpfendorfer C. A., Rummer J. L. (2019). Estimating oxygen uptake rates to understand stress in sharks and rays. Rev. Fish Biol. Fisheries 29, 297–231. doi: 10.1007/s11160-019-09553-3(0123456789
Bouyoucos I. A., Trujillo J. E., Weideli O. C., Nakamura N., Mourier J., Planes S., et al. (2021). Investigating links between thermal tolerance and oxygen supply capacity in shark neonates from a hyperoxic tropical environment. Sci. Total Environ. 782, 146854. doi: 10.1016/j.scitotenv.2021.146854
Bouyoucos I. A., Weideli O. C., Planes S., Simpfendorfer C. A., Rummer J. L. (2018). Dead tired: evaluating the physiological status and survival of neonatal reef sharks under stress. Conserv. Physiol. 6 (1), coy053. doi: 10.1093/conphys/coy053
Brown J. H., Gillooly J. F., Allen A. P., Savage V. M., West G. B. (2004). Toward a metabolic theory of ecology. Ecology 85 (7), 1771–1789. doi: 10.1890/03-9000
Burton T., Metcalfe N. B. (2014). Can environmental conditions experienced in early life influence future generations? Proc. R. Soc. B 281, 20140311. doi: 10.1098/rspb.2014.0311
Chin A., Tobin A. J., Huepel M. R., Simpfendorfer C. A. (2013). Population structure and residency patterns of the blacktip reef shark Carcharhinus melanopterus in turbid coastal environments. J. Fish Biol. 82 (4), 1192–1210. doi: 10.1111/jfb.12057
Chung D. J., Schulte P. M. (2020). Mitochondria and the thermal limits of ectotherms. J. Exp. Biol. Pt 20, jeb227801. doi: 10.1242/jeb.227801
Conrath C. L., Musick J. A. (2012). “Reproductive biology of elasmobranchs,” in Biology of sharks and their relatives, 2nd edition (Boca Raton, FL: CRC Press), 291–312.
Crozier L. G., Hutchings J. A. (2014). Plastic and evolutionary responses to climate change in fish. Evolutionary Appl. 7, 68–87. doi: 10.1111/eva.12135
Crozier L. G., Scheuerell M. D., Zabel. R. W. (2011). Using time series analysis to characterize evolutionary and plastic responses to environmental change: a case study of a shift toward earlier migration date in sockeye salmon. Am. Nat. 178, 755–773. doi: 10.1086/662669
Devaux J. B., Hickey A. J., Renshaw G. M. (2019). Mitochondrial plasticity in the cerebellum of two anoxia-tolerant sharks: contrasting responses to anoxia/re-oxygenation. J. Exp. Biol. 222 (6), jeb191353. doi: 10.1242/jeb.191353
Dicken M., Cliff G., Winker H. (2016). Sharks caught in the KwaZulu- Natal bather protection programme, South Africa. 13. The tiger shark Galeocerdo cuvier. Afr. J. Mar. Sci. 38, 285–301. doi: 10.2989/1814232X.2016.1198276
Donelson J. M., Gaitan-Espitia J. D., Hobday A. J., Mokany K., Andrew S. C., Boulter S., et al. (2023). Putting plasticity into practice for effective conservation actions under climate change. Nat. Climate Change 13, 632–647. doi: 10.1038/s41558-023-01706-4
Donelson J. M., Munday P. L. (2012). Thermal sensitivity does not determine acclimation capacity for a tropical reef fish. J. Anim. Ecol. 81 (5), 1126–1131. doi: 10.1111/j.1365-2656.2012.01982.x
Donelson J. M., Salinas S., Munday P. L., Shama L. N. S. (2018). Transgenerational plasticity and climate change experiments: Where do we go from here? Global Change Biol. 24 (1), 13–34. doi: 10.1111/gcb.13903
Donelson J. M., Sunday J. M., Figueira W. F., Gaitan-Espitia J. D., Hobday A. J., Johnson C. R., et al. (2019). Understanding interactions between plasticity, adaptation and range shifts in response to marine environmental change. Philos. Trans. R. Soc. B 374 (1768), 20180186. doi: 10.1098/rstb.2018.0186
Dowd W. W., Renshaw G. M., Cech J. J., Kültz D. (2010). Compensatory proteome adjustments imply tissue-specific structural and metabolic reorganization following episodic hypoxia or anoxia in the epaulette shark (Hemiscyllium ocellatum). Physiol. Genomics 42 (1), 93–114. doi: 10.1152/physiolgenomics.00176.2009
Duncan E. J., Gluckman P. D., Dearden P. K. (2014). Epigenetics, plasticity, and evolution: how do we link epigenetic change to phenotype? J. Exp. Zoology 322, 208–220. doi: 10.1002/jez.b.22571
Edwards J. E., Hiltz E., Broell F., Bushnell P. G., Campana S. E., Christiansen J. S., et al. (2019). Advancing research for the management of long-lived species: A case study on the Greenland shark. Front. Mar. Sci. 6. doi: 10.3389/fmars.2019.00087
Fangue N. A., Bennett W. A. (2003). Thermal tolerance responses of laboratory-acclimated and seasonally acclimatized atlantic stingray, dasyatis sabina. Copeia 2, 315–325. doi: 10.1643/0045-8511(2003)003[0315:TTROLA]2.0.CO;2
Fanter C., Madelaire C., Genereux D. P., Breukelen F., Levesque D., Hindle A. (2022). Epigenomics as a paradigm to understand the nuances of phenotypes. J. Exp. Biol. 225, jeb243411. doi: 10.1242/jeb.243411
Fawcett T. W., Frankenhuis W. E. (2015). Adaptive explanations for sensitive windows in development. Front. Zoology 12, S3. doi: 10.1186/1742-9994-12-S1-S3
Ferreira L. C., Thums M., Meeuwig J. J., Vianna G. M., Stevens J., McAuley R., et al. (2015). Crossing latitudes—long-distance tracking of an apex predator. PloS One 10 (2), e0116916. doi: 10.1371/journal.pone.0116916
Fitzpatrick R., Thums M., Bell I., Meekan M. G., Stevens J. D., Barnett A. (2012). A comparison of the seasonal movements of tiger sharks and green turtles provides insight into their predator-prey relationship. PloS One 7 (12), e51927. doi: 10.1371/journal.pone.0051927
Fox R. J., Donelson J. M., Schunter C., Ravasi T., Gaitan-Espitia J. D. (2019). Beyond buying time: the role of plasticity in phenotypic adaptation to rapid environmental change. Philos. Trans. R. Soc. B 374 (1768), 20180174. doi: 10.1098/rstb.2018.0174
Franks S. J., Sim S., Weis. A. E. (2007). Rapid evolution of flowering time by an annual plant in response to a climate fluctuation. Proc. Natl. Acad. Sci. U. S. A. 104, 1278–1282. doi: 10.1073/pnas.0608379104
Fraser D. J., Weir L. K., Bernatchez L., Hansen M. M., Taylor E. B. (2011). Extent and scale of local adaptation in salmonid fishes: review and meta-analysis. Heredity 106, 404–420. doi: 10.1038/hdy.2010.167
Gervais C. R., Nay T. J., Renshaw G., Johansen J. L., Steffensen J. F., Rummer J. L. (2018). Too hot to handle? Using movement to alleviate effects of elevated temperatures in a benthic elasmobranch, Hemiscyllium ocellatum. Mar. Biol. 165 (11). doi: 10.1007/s00227-018-3427-7
Ghalambor C. K., McKay J. K., Carroll S. P., Reznick D. N. (2007). Adaptive versus non-adaptive phenotypic plasticity and the potential for contemporary adaptation in new environments. Funct. Ecol. 21 (3), 394–407. doi: 10.1111/j.1365-2435.2007.01283.x
Heinrich D. D., Rummer J. L., Morash A. J., Watson S. A., Simpfendorfer C. A., Heupel M. R., et al. (2014). A product of its environment: the epaulette shark (Hemiscyllium ocellatum) exhibits physiological tolerance to elevated environmental CO2. Conserv. Physiol. 2 (1), cou047. doi: 10.1093/conphys/cou047
Heinrich D. D., Watson S., Rummer J. L., Brandl S. J., Simpfendorfer C. A., Heupel M. R., et al. (2016). Foraging behaviour of the epaulette shark Hemiscyllium ocellatum is not affected by elevated CO2. ICES J. Mar. Sci. 73 (3), 633–640. doi: 10.1093/icesjms/fsv085
Hickey A. J., Renshaw G. M., Speers-Roesch B., Richards J. G., Wang Y., Farrell A. P., et al. (2012). A radical approach to beating hypoxia: depressed free radical release from heart fibres of the hypoxia-tolerant epaulette shark (Hemiscyllum ocellatum). J. Comp. Physiol. B 182, 91–100. doi: 10.1007/s00360-011-0599-6
Holmes B. J., Pepperell J. G., Griffiths S. P., Jaine F. R., Tibbetts I. R., Bennett M. B. (2014). Tiger shark (Galeocerdo cuvier) movement patterns and habitat use determined by satellite tagging in eastern Australian waters. Mar. Biol. 161 (11), 2645–2658. doi: 10.1007/s00227-014-2536-1
Huepel M. R., Bennett M. B. (1998). Observations on the diet and feeding habits of the epaulette shark, Hemiscyllium ocellatum (Bonnaterre), on Heron Island Reef, Great Barrier Reef, Australia. Mar. Freshw. Res. 49, 753–756. doi: 10.1071/MF97026
Huepel M. R., Simpfendorfer C. A. (2008). Movement and distribution of young bull sharks Carcharhinus leucas in a variable estuarine environment. Aquat. Biol. 1, 277–289. doi: 10.3354/ab00030
Illing B., Downie A. T., Beghin M., Rummer J. L. (2020). Critical thermal maxima of early life stages of three tropical fishes: Effects of rearing temperature and experimental heating rate. J. Thermal Biol. 90, 102582. doi: 10.1016/j.jtherbio.2020.102582
Kelly M. (2019). Adaptation to climate change through genetic accommodation and assimilation of plastic phenotypes. Philos. Trans. R. Soc. B 374, 20180176. doi: 10.1098/rstb.2018.0176
Knip D., Heupel M., Simpfendorfer C. (2010). Sharks in nearshore environments: models, importance, and consequences. Mar. Ecol. Prog. Ser. 402, 1–11. doi: 10.3354/meps08498
Kriwet J., Witzmann F., Klug S., Heidtke U. H. J. (2008). First direct evidence of a vertebrate three-level trophic chain in the fossil record. Proc. R. Soc. B 275, 181–186. doi: 10.1098/rspb.2007.1170
Leimar O., McNamara J. M. (2015). The evolution of transgenerational integration of information in heterogeneous environments. Am. Nat. 185, E55–E69. doi: 10.1086/679575
Logan M. L., van Berkel J., Clusella-Trullas S. (2019). The Bogert Effect and environmental heterogeneity. Oecologia 191, 817–827. doi: 10.1007/s00442-019-04541-7
Mejía-Falla P. A., Cortés E., Navia A. F., Zapata F. A. (2014). Age and growth of the round stingray urotrygon rogersi, a particularly fast-growing and short-lived elasmobranch. PloS One 9 (4), e96077. doi: 10.1371/journal.pone.0096077
Merila J., Hendry A. P. (2014). Climate change, adaptation, and phenotypic plasticity: the problem and the evidence. Evolutionary Appl. 7 (1), 1–14. doi: 10.1111/eva.12137
Mourier J., Mills S. C., Planes S. (2013). Population structure, spatial distribution and life-history traits of blacktip reef sharks. Carcharhinus melanopterus J. Fish Biol. 82 (3), 979–993. doi: 10.1111/jfb.12039
Mourier J., Planes S. (2013). Direct genetic evidence for reproductive philopatry and associated fine-scale migrations in female blacktip reef sharks (Carcharhinus melanopterus) in French Polynesia. Mol. Ecol. 22, 201–214. doi: 10.1111/mec.12103
Munday P. L., Warner R. R., Monro K., Pandolfi J. M., Marshall D. J. (2013). Predicting evolutionary responses to climate change in the sea. Ecol. Lett. 16 (12), 1488–1500. doi: 10.1111/ele.12185
Nay T. J., Longbottom R. J., Gervais C. R., Johansen J. L., Steffensen J. F., Rummer J. L., et al. (2021). Regulate or tolerate: Thermal strategy of a coral reef flat resident, the epaulette shark, Hemiscyllium ocellatum. J. Fish Biol. 98 (3), 723–732. doi: 10.1111/jfb.14616
Norin T., Malte H., Clark T. D., Konarzewski M. (2016). Differential plasticity of metabolic rate phenotypes in a tropical fish facing environmental change. Funct. Ecol. 30 (3), 369–378. doi: 10.1111/1365-2435.12503
Norin T., Metcalfe N. B. (2019). Ecological and evolutionary consequences of metabolic rate plasticity in response to environmental change. Philos. Trans. R. Soc Lond. B. Biol. Sci. 374 (1768), 20180180. doi: 10.1098/rstb.2018.0180
Papastamatiou Y. P., Friedlander A. M., Caselle J. E., Lowe C. G. (2010). Long-term movement patterns and trophic ecology of blacktip reef sharks (Carcharhinus melanopterus) at Palmyra Atoll. J. Exp. Mar. Biol. Ecol. 386, 94–102. doi: 10.1016/j.jembe.2010.02.009
Papastamatiou Y. P., Meyer C. G., Carvalho F., Dale J. J., Hutchinson M. R., Holland K. N. (2013). Telemetry and random-walk models reveal complex patterns of partial migration in a large marine predator. Ecology 94 (11), 2595–2606. doi: 10.1890/12-2014.1
Papastamatiou Y. P., Watanabe Y. Y., Bradley D., Dee L. E., Weng K., Lowe C. G., et al. (2015). Drivers of daily routines in an ectothermic marine predator: hunt warm, rest warmer? PloS One 10 (6), e0127807. doi: 10.1371/journal.pone.0127807
Payne N. L., Meyer C. G., Smith J. A., Houghton J. R., Barnett A., Holmes B. J., et al. (2018). Combining abundance and performance data reveals how temperature regulates coastal occurrences and activity of a roaming apex predator. Global Change Biol. 24, 1884–1893. doi: 10.1111/gcb.14088
Pereira-Santos C. P., Sampaio E., Pereira B. P., Pegado M. R., Borges F. O., Wheeler C. R., et al. (2021). Elasmobranch responses to experimental warming, acidification, and oxygen loss—A meta-analysis. Front. Mar. Sci. 8, 735377. doi: 10.3389/fmars.2021.735377
Pichaud N., Ekström A., Breton S., Sundström F., Rowinski P., Blier P. U., et al. (2019). Cardiac mitochondrial plasticity and thermal sensitivity in a fish inhabiting an artificially heated ecosystem. Sci. Rep. 9 (1), 17832. doi: 10.1038/s41598-019-54165-3
Pörtner H. O. (2001). Climate change and temperature-dependent biogeography: oxygen limitation of thermal tolerance in animals. Naturwissenschaften 88 (4), 137–146. doi: 10.1007/s001140100216
Pörtner H. O. (2002). Climate variations and the physiological basis of temperature dependent biogeography: systemic to molecular hierarchy of thermal tolerance in animals. Comp. Biochem. Physiol. 132 (4), 739–761. doi: 10.1016/S1095-6433(02)00045-4
Pörtner H. O., Bock C., Mark F. C. (2017). Oxygen- and capacity-limited thermal tolerance: bridging ecology and physiology. J. Exp. Biol. 220 (15), 2686–2695. doi: 10.1242/jeb.134585
Potts D., Swart P. (1984). Water temperature as an indicator of environmental variability on a coral reef. Limnology Oceanography 29, 504–516. doi: 10.4319/lo.1984.29.3.0504
Reale D., McAdam A. G., Boutin S., Berteaux. D. (2003). Genetic and plastic responses of a northern mammal to climate change. Proc. R. Soc. B: Biol. Sci. 270, 591–596. doi: 10.1098/rspb.2002.2224
Rummer J. L., Bouyoucos I. A., Wheeler C. W., Santos C., Rosa R. (2022). “Climate change and sharks,” in Biology of sharks and their relatives, 3rd edition (Boca Raton, FL: CRC Press), 767–793.
Rytkönen K. T., Renshaw G. M., Vainio P. P., Ashton K. J., Williams-Pritchard G., Leder E. H., et al. (2012). Transcriptional responses to hypoxia are enhanced by recurrent hypoxia (hypoxic preconditioning) in the epaulette shark. Physiol. Genomics 44 (22), 1090–1097. doi: 10.1152/physiolgenomics.00081.2012
Ryu T., Veilleux H. D., Donelson J. M., Munday P. L., Ravasi T. (2018). The epigenetic landscape of transgenerational acclimation to ocean warming. Nat. Climate Change 8, 504–509. doi: 10.1038/s41558-018-0159-0
Ryu T., Veilleux H. D., Munday P. L., Jung I., Donelson J. M., Ravasi T. (2020). An epigenetic signature for within-generational plasticity of a reef fish to ocean warming. Front. Mar. Sci. 7, 284. doi: 10.3389/fmars.2020.00284
Salinas S., Brown S. C., Mangel M., Munch S. B. (2013). Non-genetic inheritance and changing environments. Non-Genetic Inheritance 1, 38–50. doi: 10.2478/ngi-2013-0005
Schnell A. K., Seebacher F. (2008). Can phenotypic plasticity facilitate the geographic expansion of the tilapia Oreochromis mossambicus? Physiol. Biochem. Zoology 81 (6), 733–742. doi: 10.1086/592027
Schnurr M. E., Yin Y., Scott G. R. (2014). Temperature during embryonic development has persistent effects on metabolic enzymes in the muscle of zebrafish. J. Exp. Biol. 217 (8), 1370–1380. doi: 10.1242/jeb.094037
Schunter C., Welch M. J., Ryu T., Zhang H., Berumen M. L., Nilsson G. E., et al. (2016). Molecular signatures of transgenerational response to ocean acidification in a species of reef fish. Nat. Climate Change 6, 1014–1018. doi: 10.1038/nclimate3087
Seebacher F., White C. R., Franklin C. E. (2014). Physiological plasticity increases resilience of ectothermic animals to climate change. Nat. Climate Change 5, 61–66. doi: 10.1038/nclimate2457
Shama L. S. (2015). Bet hedging in a warming ocean: predictability of maternal environment shapes offspring size variation in marine sticklebacks. Global Change Biol. 21 (12), 4387–4400. doi: 10.1111/gcb.13041
Snelson F. F., Williams-Hooper S. E., Schmid T. H. (1988). Reproduction and ecology of the atlantic stingray, dasyatis sabina, in florida coastal lagoons. Copeia 3, 729–739. doi: 10.2307/1445395
Thums M., Meekan M., Stevens J., Wilson S., Polovina J. (2013). Evidence for behavioural thermoregulation by the world’s largest fish. J. R. Soc. Interface 10, 20120477. doi: 10.1098/rsif.2012.0477
Tullis A., Baillie M. (2005). The metabolic and biochemical responses of tropical white spotted bamboo shark Chiloscyllium plagiosum to alterations in environmental temperature. J. Fish Biol. 67, 950–968. doi: 10.1111/j.0022-1112.2005.00795.x
Tyler S., Sidell B. D. (1984). Changes in mitochondrial distribution and diffusion distances in muscle of goldfish upon acclimation to warm and cold temperatures. J. Experimental. Zoology 232, 1–9. doi: 10.1002/jez.1402320102
Veilleux H. D., Ryu T., Donelson J. M., Munday P., Ravasi T. (2018). Molecular response to extreme summer temperatures differs between two genetically differentiated populations of a coral reef fish. Front. Mar. Sci. 5, 349. doi: 10.3389/fmars.2018.00349
Veilleux H. D., Ryu T., Donelson J. M., Van Herwerden L., Seridi L., Ghosheh Y., et al. (2015). Molecular processes of transgenerational acclimation to a warming ocean. Nat. Climate Change 5 (12), 1074–1078. doi: 10.1038/nclimate2724
Vilmar M., Di Santo V. (2022). Swimming performance of sharks and rays under climate change. Rev. Fish Biol. Fisheries 32, 765–781. doi: 10.1007/s11160-022-09706-x
Visser M. E. (2008). Keeping up with a warming world: assessing the rate of adaptation to climate change. Proc. R. Soc. B 275, 649–659. doi: 10.1098/rspb.2007.0997
Wallman H. L., Bennett W. A. (2006). Effects of parturition and feeding on thermal preference of Atlantic stingray, Dasyatis sabina (Lesueur). Environ. Biol. Fishes 75, 259–267. doi: 10.1007/s10641-006-0025-1
Wang J., Zhang Z. (2021). GAPIT Version 3: boosting power and accuracy for genomic association and prediction. Genomics Proteomics Bioinf. 19 (4), 629–640. doi: 10.1016/j.gpb.2021.08.005
Wellband K. W., Heath D. D. (2017). Plasticity in gene transcription explains the differential performance of two invasive fish species. Evolutionary Appl. 10 (6), 563–576. doi: 10.1111/eva.12463
Wheeler C. R., Lang B. J., Mandelman J. W., Rummer J. L. (2022). The upper thermal limit of epaulette sharks (Hemiscyllium ocellatum) is conserved across three life history stages, sex, and body size. Conserv. Physiol. 10 (1), coac074. doi: 10.1093/conphys/coac074
Wheeler C. R., Rummer J. L., Bailey B., Lockwood J., Vance S., Mandelman J. W. (2021). Future thermal regimes for epaulette sharks (Hemiscyllium ocellatum): growth and metabolic performance cease to be optimal. Sci. Rep. 11 (1), 454. doi: 10.1038/s41598-020-79953-0
Wilmers C. C., Nickel B., Bryce C. M., Smith J. A., Wheat R. E., Yovovich V. (2015). The golden age of bio-logging: how animal-borne sensors are advancing the frontiers of ecology. Ecology 96 (7), 1741–1753. doi: 10.1890/14-1401.1
Keywords: plasticity, adaptation, elasmobranch, climate change, physiology
Citation: Hasenei A, Donelson JM, Ravasi T and Rummer JL (2023) Sharks and their relatives: can their past help predict their future? Front. Mar. Sci. 10:1268532. doi: 10.3389/fmars.2023.1268532
Received: 28 July 2023; Accepted: 12 October 2023;
Published: 01 November 2023.
Edited by:
Anthony (Tony) John Hickey, The University of Auckland, New ZealandReviewed by:
Lene H. Petersen, Texas A&M University at Galveston, United StatesGillian Renshaw, Griffith University, Australia
Copyright © 2023 Hasenei, Donelson, Ravasi and Rummer. This is an open-access article distributed under the terms of the Creative Commons Attribution License (CC BY). The use, distribution or reproduction in other forums is permitted, provided the original author(s) and the copyright owner(s) are credited and that the original publication in this journal is cited, in accordance with accepted academic practice. No use, distribution or reproduction is permitted which does not comply with these terms.
*Correspondence: Aaron Hasenei, aaron.hasenei@my.jcu.edu.au