- 1Fisheries College, Jimei University, Xiamen, China
- 2Fujian Engineering Research Center of Aquatic Breeding and Healthy Aquaculture, Jimei University, Xiamen, China
- 3Key Laboratory of Healthy Mariculture for the East China Sea, Ministry of Agriculture, Xiamen, China
Light strongly influences the carbon (C) metabolism of seaweed through both algal carbon content and organic carbon release, thereby driving the carbon cycling of coastal oceans. However, the response of seaweed organic carbon release to varying light intensities remains an underexplored area of research. This study aimed to fill this gap by analyzing the effects of four different light intensities (5, 50, 200, and 500 μmol m–2 s–1) on the growth, carbon content, and organic carbon release of two strains (W28–42 and WO15-4) of Pyropia haitanensis. The results showed that as light intensity increased, both strains experienced an initial rise in growth rate followed by a decline, with the highest growth observed at 200 μmol m–2 s–1. Simultaneously, tissue C content increased with light intensity, whereas the nitrogen (N) and phosphorus (P) contents exhibited decreasing trends. This led to increases in the C:N and C:P ratios, indicating that high light intensity may enhance C fixation and suppress the absorption of N and P. Of particular interest was the difference in organic carbon release between the two strains. The W28–42 strain’s rate of dissolved organic carbon (DOC) release increased significantly with light intensity, whereas the WO15–4 strain’s DOC release rate remained unaffected by variations in light intensity. The particulate organic carbon (POC) release rates of both strains increased under higher light intensity, with the W28–42 strain showing a more substantial increase than the WO15–4 strain. This study demonstrates that the release of DOC by P. haitanensis exhibits distinct strain-specific responses to variations in light intensity, a result that may be attributed to differences in photosynthetic physiology and genetic makeup. These insights provide a foundation for enhancing the efficiency of fishery carbon sinks through the manipulation of light intensity.
Introduction
Seaweeds, as important photosynthetic organisms, play a crucial role in various ecological processes. Seaweeds are involved in climate regulation, carbon sequestration, oxygen production, water purification, and environmental restoration (Hurd et al., 2014; Raven and Hurd, 2012; Falkowski and Raven, 2013). The growth and production of seaweeds are influenced by a combination of factors, including the utilization of inorganic nitrogen (Harrison and Hurd, 2001), light intensity, temperature (Wernberg et al., 2010), salinity (Conitz et al., 2001), CO2 concentration, and pH (Lise Middelboe and Juel Hansen, 2007; Britton et al., 2016). Living in the intertidal zone, seaweeds experience significant fluctuations in light intensity due to tidal changes, mixed layer depths, seawater transparency, weather conditions, and climate change. This environmental variability has prompted seaweeds to evolve complex adaptation mechanisms to cope with changes in light conditions and meet their requirements for growth (Hurd et al., 2014). Light is an essential factor for the growth and development of all photosynthetic organisms. However, excessive light can lead to photoinhibition, a process that causes stress to plants and algae and negatively affects their growth. Excessive light energy may damage the photosynthetic system, leading to a decrease in photosynthetic capacity and impacting many important metabolic processes related to growth and development (Li et al., 2009; Raven, 2011; Falkowski and Raven, 2013). Low-light stress also has significant effects on phototrophs. For example, in low-light environments, single-celled algae tend to be smaller to more effectively absorb light energy and support their growth and development, a phenomenon that may be attributed to the fact that smaller cells have a higher surface-to-volume ratio and thus a greater light absorption capacity (Littler and Littler, 1980; Finkel et al., 2010; Beardall et al., 2009). These findings suggest that algae can adjust their physiological and morphological characteristics to adapt to different lighting conditions, thereby ensuring their survival.
The carbon metabolism of algae can be roughly divided into three stages: the absorption of inorganic carbon, the conversion of inorganic carbon to organic carbon, and the distribution of organic carbon within and outside the algae (Paine et al., 2021; Dorte et al., 2018). Through photosynthesis and other metabolic processes, the absorbed carbon and other nutrients are used to synthesize various macromolecules, including carbohydrates, proteins, and fats. Some of these organic substances constitute part of the algae and are involved in various physiological and metabolic functions, while others are released into the environment in the form of dissolved organic carbon (DOC) and particulate organic carbon (POC) (Paine et al., 2021). The seaweed industry effectively removes a significant amount of carbon, nitrogen, and phosphorus from the coastal environment through periodic harvesting, thus playing a key role in controlling eutrophication and preventing harmful algal blooms (Yang et al., 2015). DOC accounts for 20% of all organic carbon on Earth and is considered an essential component of the global biogeochemical carbon cycle (Barrón and Duarte, 2016; Shen and Benner, 2018). In the oceans, both DOC and POC can be converted into refractory dissolved organic carbon (RDOC) by microbial activity. RDOC is a stable form of carbon that functions in long-term storage and thus has potential for development in carbon sequestration technology (Shen and Benner, 2018; Yi et al., 2022; Wang et al., 2022). Therefore, it has been proposed that seaweed aquaculture may have significant applications in oceanic carbon sequestration (Raven, 2018; Ortega et al., 2019).
Based on a laboratory culture experiment using 11 seaweed species, a previous study identified a linear correlation between growth rate and the release rate of DOC (Chen et al., 2020). However, a recent study reported that under sufficient nutrient conditions, the DOC release rate of the seaweed Sargassum horneri was negatively correlated with net primary productivity (NPP), whereas under nutrient-limited conditions, there was no clear relationship between DOC release and NPP (Sun et al., 2024). In addition, several environmental factors, including light, temperature, nutrients, and salinity, can influence the release of DOC from seaweeds (Sun et al., 2024; Chen et al., 2023; Xu et al., 2022; Ni et al., 2022; Xu et al., 2021). These studies indicated that the rate of DOC release from seaweeds is closely related to environmental conditions, even though the precise nature of the relationship remains unclear. Since light intensity is a major environmental factor in the photosynthetic physiology of seaweed and strongly influences many other metabolic processes, it may have a strong effect on DOC release. Given that DOC and POC are derived from photosynthetically fixed carbon, light could indirectly alter the release rates of DOC and POC via photosynthesis. This is the core assumption of the “overflow hypothesis”, which suggests that DOC is released from algal cells when the photosynthetic rate exceeds the rate necessary for growth (Paine et al., 2021).
Light also affects the elemental ratios of algae (Finkel et al., 2006). For example, increased light intensity significantly elevated the cellular C:N and C:P ratios of the marine diatom Skeletonema marinoi (Norici et al., 2011). Similarly, researchers found that increased light intensity could significantly enhance the C:P ratio of Selenastrum capricornutum (Hessen et al., 2008). Furthermore, a recent study found that changes in light intensity altered the C:N ratio by influencing the growth rate, nitrogen consumption, and carbon allocation of Coccomyxa subellipsoidea (Liu and Wei, 2023).Under light limitation, the growth rate and the C:N ratio of the seaweed Gracilaria tikvahiae decreased as the contents of N and protein increased (Lapointe and Duke, 1984). These findings illustrate the impact of light intensity on the elemental ratios of algae; i.e., there are uneven effects on the metabolism of carbon and other elements.
In summary, it is clear that light conditions have a significant impact on the growth and carbon metabolism of seaweeds, but there is still a lack of in-depth studies on the physio-ecological responses of seaweeds to variations in light intensity in terms of the release of organic carbon and elemental composition. For this reason, we selected an economically important seaweed, P. haitanensis, as a research organism, aiming to investigate its responses to light intensity in terms of growth, biochemical composition, carbon content, elemental composition, and organic carbon release. Through this study, we hope to gain a better understanding of the contribution of seaweeds to the coastal ecosystem and provide a basis for efficient seaweed cultivation. This research will help determine the regulatory role of light intensity in the carbon metabolism of seaweeds, and the findings will have important implications for developing reasonable cultivation strategies, optimizing the use of marine resources, and protecting the marine environment.
Materials and methods
Cultures and growth conditions
In this study, two strains of P. haitanensis, W28-42 (a narrow strain) and WO15-4 (a wide strain), were obtained from the Laboratory of Germplasm Improvements and Applications of Pyropia in Fujian Province. The sporophytic filaments (the conchocelis stage) were cultured at 29°C under a light intensity of 10–20 µmol m−2 s−1 with a photoperiod of 8 h light/16 h dark until maturity. The mature conchocelis were then cultured at 21°C with aeration to promote the release of conchospores capable of developing into gametophytic blades (the thallus stage). Healthy, intact thalli with a length of 5 ± 1 cm were randomly selected, with three thalli placed into each 1 L sterilized glass flask for cultivation.
The thalli were cultured at 21 ± 1°C in nutrient-enriched natural seawater prepared according to Provasoli’s seawater medium (Starr and Zeikus, 1993). The media were aerated during cultivation, and the photoperiod was 12 h light/12 h dark. In our preliminary experiments, P. haitanensis thalli were cultured at six gradient light intensity conditions (5, 50, 200, 500, 700 and 1000 μmol m–2 s–1). However, thalli failed to survive under light intensities of 700 and 1000 μmol m–2 s–1. Therefore, only four light intensity gradients (5, 50, 200, and 500 μmol m–2 s–1) were selected for the formal experiments. Each light treatment contained three biological replicates. Samples were harvested after seven days of cultivation.
Experimental design and sample collection
After seven days of cultivation, 200 mL of the medium was collected from each glass flask and filtered using pre-combusted Whatman GF/F filters (diameter: 25 mm, pore size: 0.7 µm) under a low vacuum. The filter membranes were dried before the analysis of particulate organic carbon (POC) and stored at –20°C to prevent microbial decomposition of the POC. The filtrate (50 mL) was collected into pre-treated brown glass bottles and immediately stored at –20°C for the determination of dissolved organic carbon (DOC). All glassware was combusted in a muffle furnace at 450°C for four hours, soaked in 0.1 mol/L HCl solution for over 24 h, and finally rinsed with ultrapure water.
On the final day of cultivation, the thalli were harvested from the culture bottles, and surface water was removed with absorbent gauze. The fresh weight of the thalli was measured using an analytical balance. The thalli were then dried in an oven at 60°C to a constant weight, recorded as the dry weight. The dried algae were stored at –20°C for the determination of elemental contents in the tissue.
Measurements
Growth rate
The length (L), width (W), fresh weight (FW), and dry weight (DW) of the thalli were measured before and after seven days of cultivation. The formulas for the specific growth rate of fresh weight (FW-SGR), the daily growth rate of fresh weight (FW-DGR), and the length (L-DGR) and width (W-DGR) were as follows:
where the lowercase numbers 0 and 7 indicate that the parameters were measured on days 0 and 7, respectively.
Release of DOC and POC
The concentration of DOC was determined with a Shimadzu TOC-VCPH analyzer (TOC5000A; Shimadzu, Kyoto, Japan) through high-temperature catalytic oxidation at 720°C. The POC samples were dried at a constant temperature of 60°C before analysis and were measured using a Costech ECS CHNSO elemental analyzer. The net release rates (mg·g–1 d–1 DW) of OC (as DOC and POC) by P. haitanensis were calculated as
where δOC represents the increase in organic carbon concentration (mg/L) over seven days of culture, and V and DW are the volume of seawater (L) and the initial dry weight of the algal tissue (g), respectively.
Tissue C, N, and P contents
The algal samples were dried at a constant temperature of 60°C and then ground into a powdered form. The C and N contents of the algal tissue were obtained by combustion and were measured using a Costech ECS CHNSO elemental analyzer (Costech, USA). The tissue P content was measured by acid-persulfate digestion and subsequent soluble reactive phosphate analysis using an Automatic Discrete Analyzer (CleverChem380, DeChem-Tech, Germany). The obtained C, N, and P contents were converted into molar concentrations, and the molar ratios of C:N, C:P, and N:P for different treatment groups were calculated.
Statistical analysis
All values represent the mean of three replicates for each treatment. Error bars in the figures depict standard deviations. The differences between treatment groups were analyzed using one-way analysis of variance (ANOVA). All statistical analyses were carried out using SPSS 24.0 (International Business Machines Corporation, USA).
Results
Growth rate
Light intensity had a significant effect on the growth rates of strains W28–42 and WO15-4 (P < 0.05, Figures 1, 2). All four treatments displayed initial increases in growth rate followed by decreases with increasing light intensity, reaching a maximum at 200 μmol m–2 s–1. The specific growth rates of fresh weight (FW-SGR) of W28–42 were always higher than those of WO15-4, but the difference was only significant at a light intensity of 200 μmol m–2 s–1 (P < 0.05, Figure 2A). Interestingly, the daily changes in fresh weight (FW-DGR) showed a different trend compared with FW-SGR; the FW-DGR of W28–42 was always lower than that of WO15-4, except for the lowest light treatment (P < 0.01, Figure 2B). Similarly, the daily changes in length (L-DGR) and width (W-DGR) showed an opposite trend. The L-DGR of W28–42 was significantly higher than that of WO15-4 (P < 0.001, Figure 2C) under exposure to light intensities of 50–500 μmol m–2 s–1, but the W-DGR of W28–42 was significantly lower than that of WO15-4 (P < 0.001, Figure 2D).
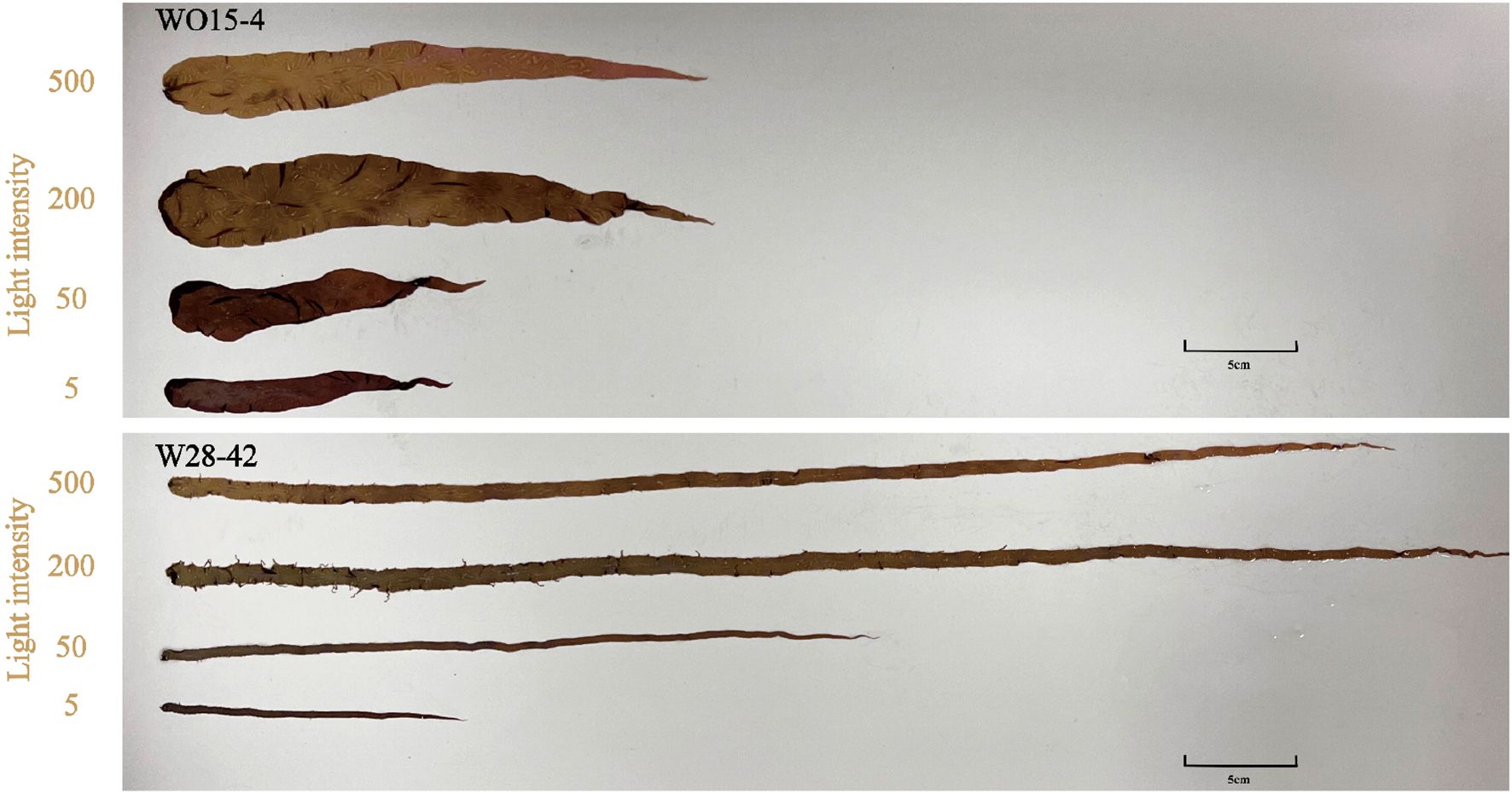
Figure 1. Typical thalli of strains W28-42 (a narrow strain) and WO15-4 (a wide strain) grown under four light intensities (μmol m–2 s–1).
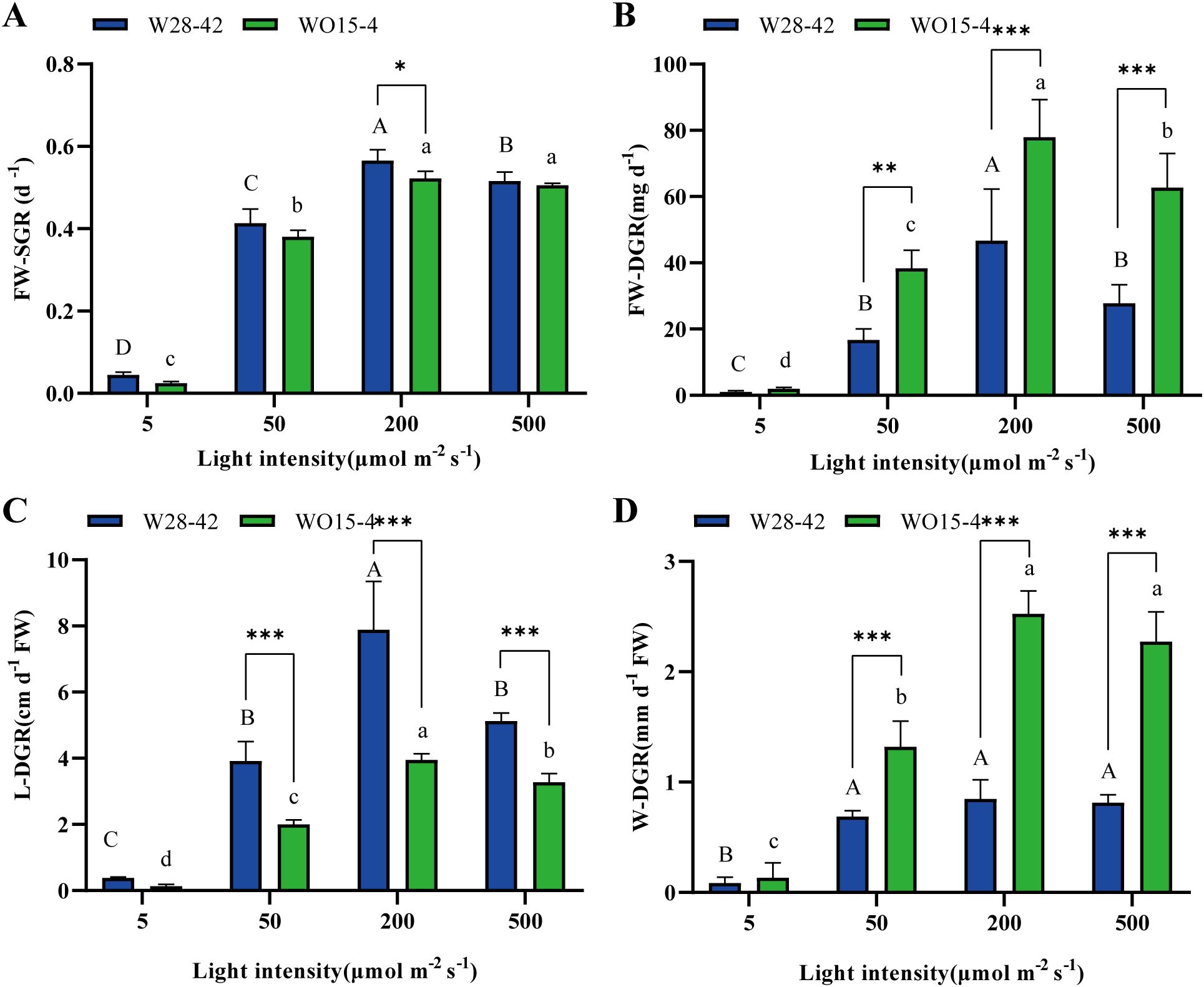
Figure 2. Specific growth rates of fresh weight (FW-SGR, A); daily growth rate of fresh weight (FW-DGR, B); length (L-DGR, C); and width (W-DGR, D) of strains W28-42 (blue bars) and WO15-4 (green bars) under four different light intensities. Different letters indicate significant differences between different light treatments. The symbols “*”, “**”, and “***”, respectively, indicate P < 0.05, P < 0.01, and P < 0.001. The values are expressed as mean ± SD (n=3).
DOC, POC, and tissue C
The carbon accumulation rates of algal tissue and the release rates of DOC and POC of the two strains under four light intensities were measured to investigate the distribution of photosynthetically fixed carbon by the metabolic activity of P. haitanensis. The tissue C accumulation rates significantly increased with light intensity (P < 0.05, Figure 3A), and no significant differences were found between the two strains concerning the rates of tissue C accumulation and DOC release (P > 0.05, Figures 3A, B). The DOC release rates of the two strains showed different responses to light intensity, with the rate of strain W28–42 increasing with light intensity, while strain WO15–4 was not affected by the light intensity (Figure 3B). The POC release rates of both strains increased with light intensity (P < 0.05, Figure 3C). In addition, except for the light intensity of 5 μmol m–2 s–1, the POC release rates of strain W28–42 were much higher than those of strain WO15-4 (P < 0.001, Figure 3C).
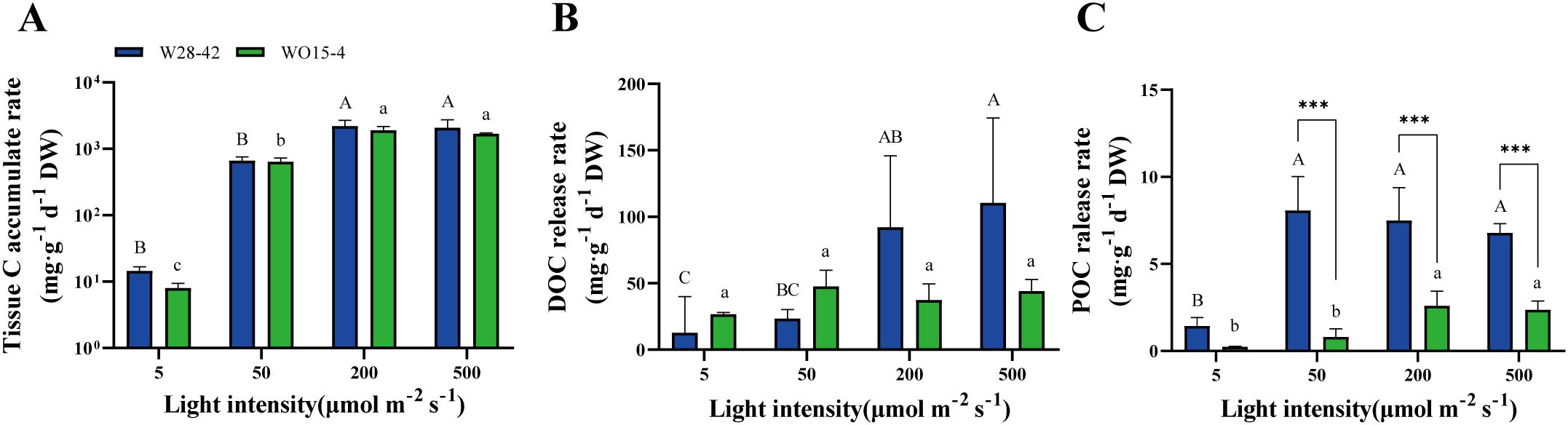
Figure 3. The rates of tissue C accumulation (A), DOC release (B), and POC release (C) of strains W28-42 (blue bars) and WO15-4 (green bars) under four different light intensities during seven days of incubation. Different letters indicate significant differences between treatments. The symbols “*”, “**”, and “***”, respectively, indicate P < 0.05, P < 0.01, and P < 0.001. Values are expressed as mean ± SD (n=3). DW, dry weight.
The production rates of the three types of organic C were positively correlated with the specific growth rates of the algae (Figure 4). In particular, the coefficient of determination (R2) between tissue C accumulation and specific growth rate ranged from 0.7169 to 0.8364, with the high R2 values indicating a relatively close relationship between these two parameters (Figure 4A). Meanwhile, R2 values between DOC release and specific growth rate were relatively lower, ranging only from 0.2824 to 0.3665 (Figure 4B). R2 values between the POC release rate and specific growth rate exceeded 0.6 (Figure 4C), indicating a significant positive correlation between these two parameters.
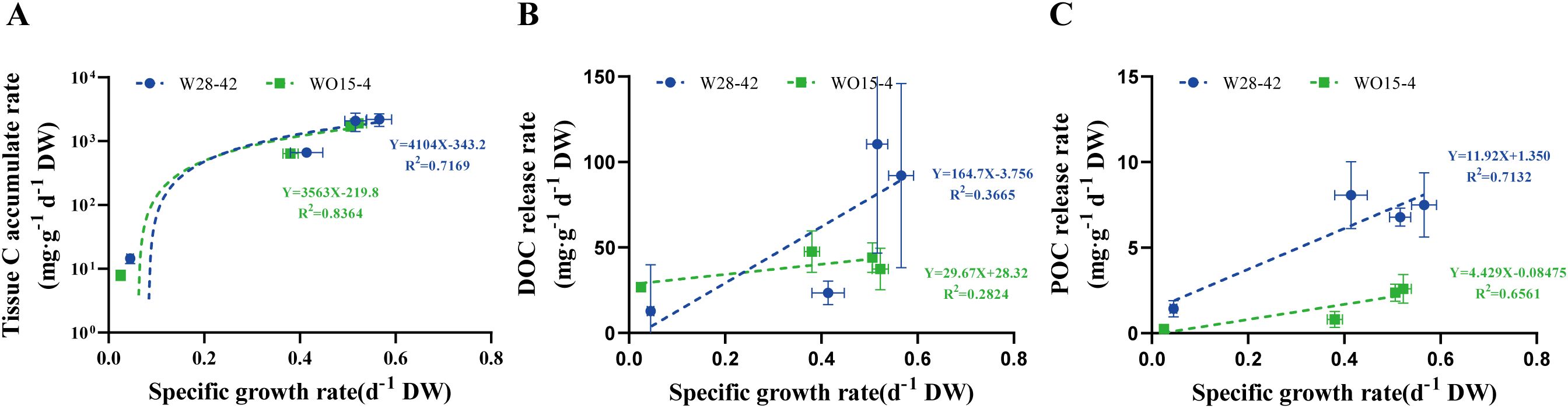
Figure 4. The relationships between the specific growth rate and three types of C variation in two strains of P. haitanensis, W28-42 (blue lines) and WO15-4 (green lines): tissue C accumulation rate (A), DOC release rate (B), and POC release rate (C).
Tissue C, N, and P contents
To further explore the effects of light intensity on the nutrient elemental composition in the tissue of P. haitanensis, we measured the C, N, and P contents in the algal tissue. The results showed that significant differences in the contents of the three nutrient elements under different light intensities. Specifically, the tissue C content of both strains significantly increased with the enhancement of light intensity (P < 0.05). The C content of the W28–42 strain increased by 29.3–34.5%, while the C content of the WO15–4 strain increased by 31.2–34.6% (Figure 5A). However, the tissue N and P contents were either negatively (P < 0.05) affected or unaffected (P > 0.05) by increasing light intensity (Figures 5B, C). There were significant differences between the two strains in the contents of C, N, and P at the two lower light intensities, with no differences under the two higher light intensities (Figures 5A–C).
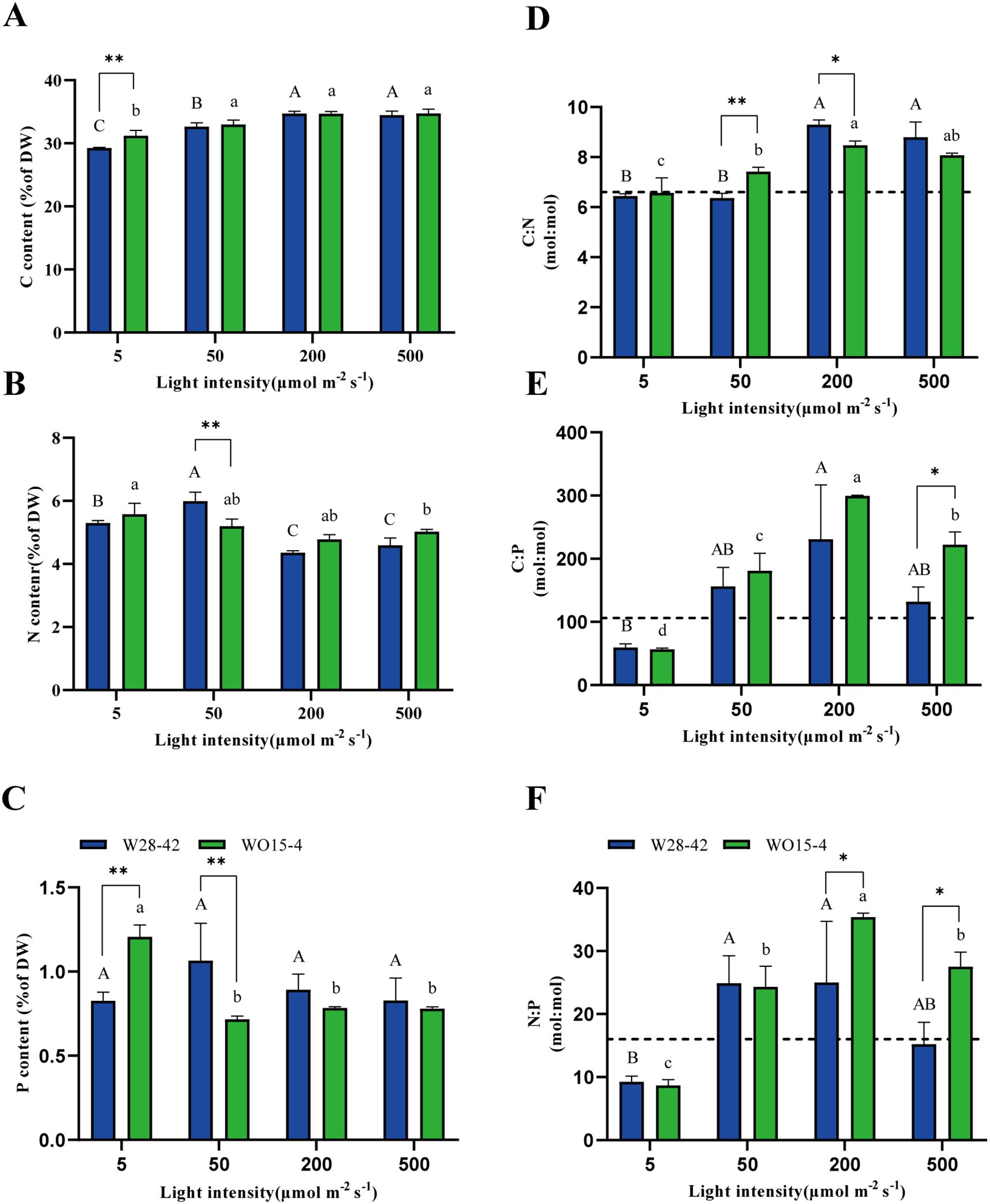
Figure 5. Tissue elemental contents and ratios of the W28-42 (blue bars) and WO15-4 (green bars) strains exposed to the four light treatments. C (carbon, A),N (nitrogen, B), and P (phosphorus, C) contents are expressed as a percentage of the sample dry weight (DW). Three elemental ratios: C:N (D), C:P (E), and N:P (F) in the four light treatments. The horizontal dotted line indicates the Redfield ratio (C:N:P = 106:16:1). All data are expressed as mean ± SD (n = 3), and different letters represent significant differences among the treatments. The symbols “*”and “**”, respectively, indicate P < 0.05 and P < 0.01.
Elemental stoichiometry
We calculated the ecological stoichiometric ratios based on the elemental contents. The results showed significant differences in the stoichiometric ratios of P. haitanensis under different light intensities. Specifically, the C:N ratios were significantly higher under high-light conditions than under low-light conditions (P < 0.05, Figure 5D). In addition, the tissue C:P ratio (Figure 5E) and tissue N:P ratio (Figure 5F) of both strains showed a trend of initially increasing and then decreasing with the increase in light intensity (P < 0.05). At the lowest light intensity (5 μmol m–2 s–1), the differences between the two strains in the C:N, C:P, and N:P ratios were not significant. Under 50 μmol m–2 s–1, only the C:N ratio showed a significant difference between the two strains (P < 0.01, Figure 5D). Under the two higher light intensities (200 and 500 μmol m–2 s–1), the C:N ratio of strain W28–42 tended to be higher than that of strain WO15-4, while the C:P and N:P ratios showed opposite trends between the strains (Figures 5D–F).
At the lowest light intensity of 5 μmol m–2 s–1, the C:P and N:P ratios of both strains of P. haitanensis were much lower than the Redfield ratio (106:16:1), and growth under the other light intensities tended to be higher than the Redfield ratio (Figures 5E, F).
The C:N ratio of algal tissue increased linearly with increasing N content (R2 = 0.8610, Figure 6A), but not with increasing P content (R2 = 0.1859, Figure 6B). The C:P ratio showed a relatively weak negative correlation with N content (R2 = 0.3459) (Figure 6C) and a relatively stronger correlation with P content (R2 = 0.5005) (Figure 6D), indicating the complex regulatory role of light intensity on the elemental stoichiometric ratios of P. haitanensis and its ecological significance.
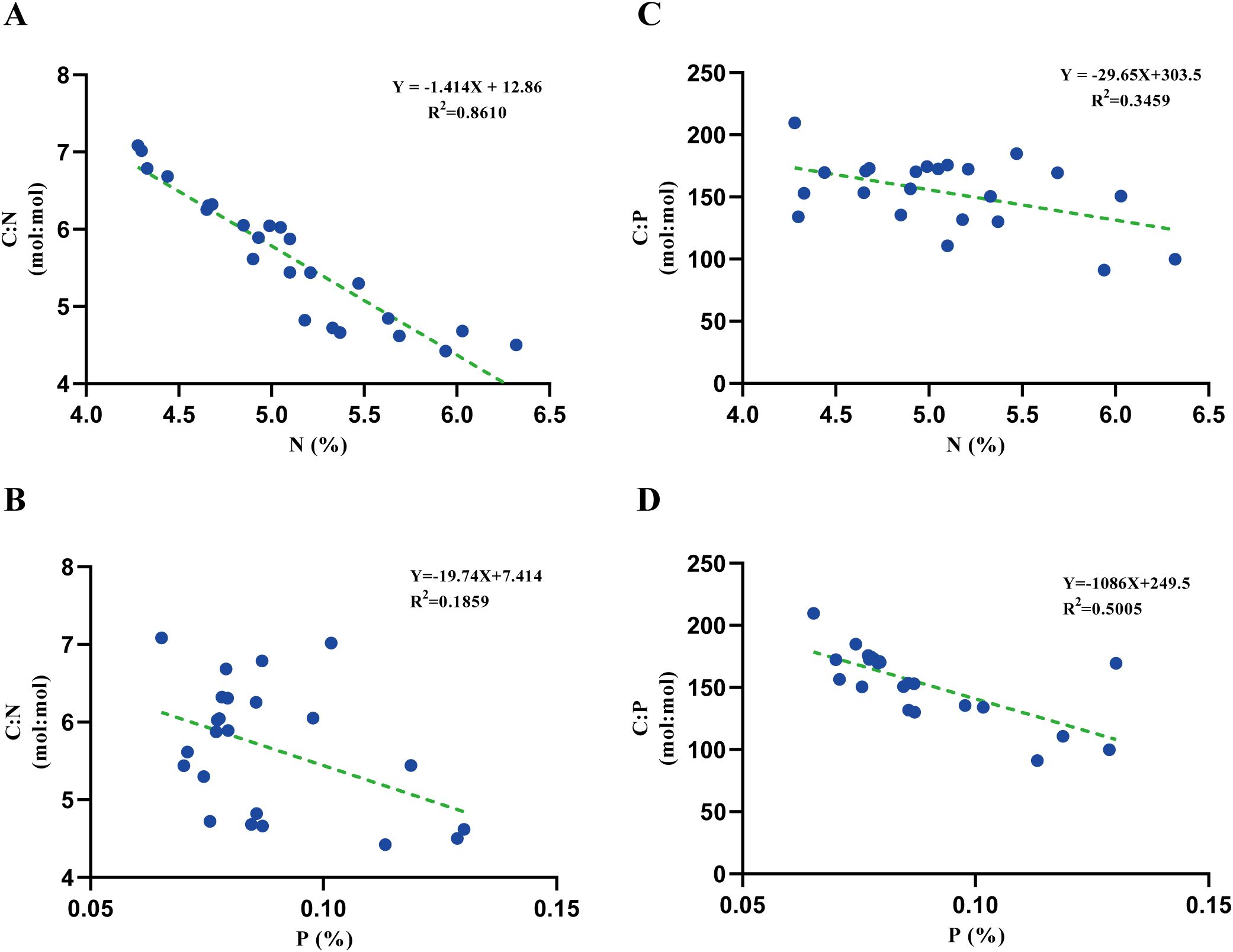
Figure 6. Correlations between N and C:N ratio (A), P and C:N (B), N and C:P (C), and P and C:P (D) of P. haitanensis tissues.
Correlation analysis
The results of the correlation analysis indicated that the overall trends for the two strains were similar (Figures 7A, B). There were significant positive correlations between light intensity and the four measures of growth rate, tissue C content, C:N ratio, and tissue C accumulation rate. However, light intensity was negatively correlated with the N and P contents of the algal tissue. The production of DOC, POC, and tissue C was positively correlated with the four measures of growth rate and tissue C content. There were significant differences between the two strains regarding the correlations among tissue P content. For strain WO15-4, tissue P content was negatively correlated with the four measures of growth rate and tissue C content (Figure 7B); however, strain W28–42 showed weak positive correlations (Figure 7A). The tissue P content of WO15–4 exhibited negative correlations with the C:N, C:P, and N:P ratios as well as the production of DOC, POC, and tissue C, but strain W28–42 displayed opposite or weak correlations. These results reveal a strain-specific difference in phosphorus metabolism under different light intensities.
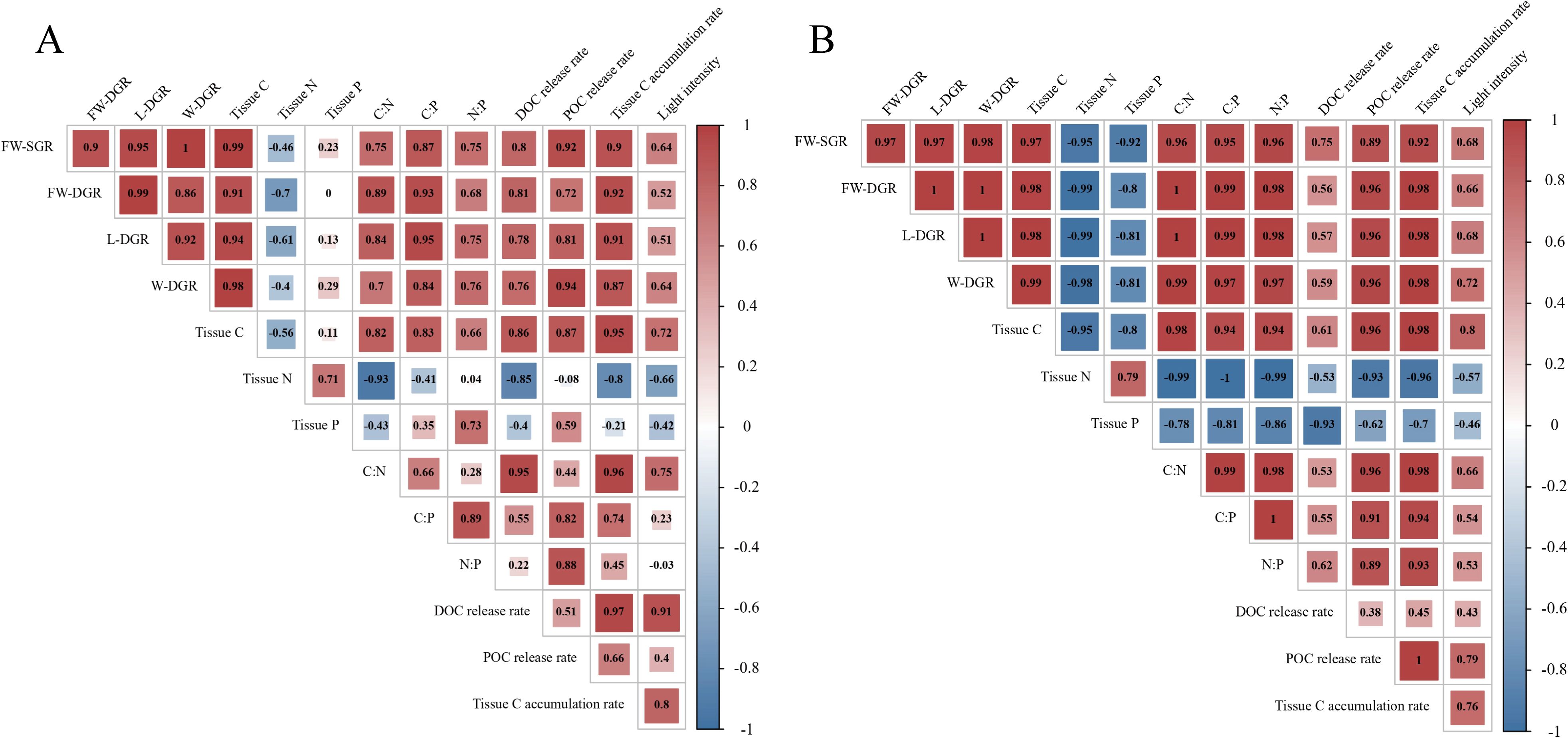
Figure 7. Correlation analysis between light intensity and growth parameters, production rates of three carbon types, and tissue C, N, and P contents with elemental ratios in strains W28-42 (A) and WO15-4 (B). Red signifies a positive correlation, with larger numbers indicating a stronger positive relationship. Conversely, blue signifies a negative correlation, where smaller numbers denote a stronger negative relationship.
Discussion
Growth
It is generally believed that light, as a key environmental factor, plays a crucial role in regulating the photosynthetic physiology and growth rate of seaweed (Lichtenthaler and Wellburn, 1983). The impact of light on the growth rate of marine algae has been extensively studied in various species. Despite the large differences in research subjects and study periods, the overall trends and mechanisms have been fairly consistent. In this study, there were significant correlations between the growth rate of P. haitanensis and light intensity. As light intensity increased, the growth rate of P. haitanensis showed an initially increased and then decreased trend (Figure 1), a pattern that aligns with findings from studies on other seaweed species, such as Sargassum fusiforme (Endo et al., 2023). This phenomenon indicates that light intensity is a key environmental factor affecting seaweed growth. Specifically, under a light intensity of 200 μmol m–2 s–1, the fresh weight growth rate of P. haitanensis significantly exceeded the values observed under the lowest and highest light intensities, regardless of strain (Figures 1, 2). These results suggested that there exists an optimal light intensity range for P. haitanensis.
Particularly, under the light intensity of 200 μmol m–2 s–1, although the relative growth rate (specific growth rate) of W28–42 was higher than that of WO15-4, the absolute growth rate (daily growth rate) showed the opposite trend. This could be attributed to the difference in morphology between the two strains; the initial fresh weight of the narrow strain W28–42 was much lower than that of the wide strain WO15-4. Interestingly, the daily growth rates in length and width showed strain-specific responses to changing light intensity. Under exposure to light intensities of 50–500 μmol m–2 s–1, the growth in length of W28–42 was greater than that of WO15-4, while the opposite trend was observed for width (Figure 2). Such a phenomenon indicates an allometric relationship and has been intensively studied (Brown et al., 2004; West et al., 2001).
Release of organic carbon and implications
Macroalgae release a substantial amount of DOC into the environment during growth, meaning that they have significant carbon sequestration potential (Ortega et al., 2019; Raven, 2018). Meanwhile, the DOC released by autotrophs is an important allochthonous carbon source for heterotrophic bacteria, enhancing the detrital food chain within ecosystems (Ho et al., 2024). In this way, the extracellular release of DOC supports the functional health of coastal ecosystems by delivering photosynthetically fixed carbon to higher trophic levels through the microbial loop, thereby maintaining ecosystem function (Hall et al., 2024). Numerous studies have demonstrated a significant influence of environmental factors on the release of DOC from seaweeds (Paine et al., 2021); this poses challenges for assessing their potential for carbon sequestration. Among the relevant environmental factors, light can be considered the most important for seaweeds since it influences many important metabolic pathways and regulates growth, development, and photosynthetic processes. Given that almost all of the organic carbon released from seaweeds originates from photosynthetically fixed carbon, the release of DOC by seaweeds is considered highly regulated by light intensity (Haas et al., 2010).
Recent studies have reported that the DOC release by seaweed Sargassum thunbergii and marine macrophyte communities is highly light-dependent, in another words, rely on photosynthetic activity (Jiménez-Ramos et al., 2023; Zhao et al., 2023). However, in this study, we observed that the DOC release rate of P. haitanensis strain W28–42 was significantly greater under higher light intensities than under low light conditions, but light intensity did not have a significant effect on the DOC release of strain WO15-4 (Figure 3). Moreover, a recent study found that different life stages (thallus vs conchocelis) of P. haitanensis showed varied responses in DOC release to changing light conditions (Xu et al., 2022). In addition, considering the complex interactions between environmental factors, the present and previous studies highlight the challenges in quantifying the effects of environmental factors on DOC release and the coastal carbon cycle mediated by diverse seaweed species and strains.
In the present study, we attempted to explain the mechanisms resulting in the strain-specific DOC release rates of P. haitanensis in response to changes in light intensity. First, differences in photosynthetic physiological characteristics among strains could be the primary factor contributing to the different DOC release rates under varying light intensity. This is because the carbon in DOC originates from the carbon fixed through photosynthesis. According to the “overflow hypothesis”, DOC is released from algae when the photosynthetic rate exceeds the rate necessary for growth (Paine et al., 2021). However, this hypothesis applies only to strain W28-42, as a linear relationship between growth rate and DOC release was observed in this strain (Figure 4B). Different strains of seaweed may have different photosynthetic efficiencies, pigment contents, and antioxidant systems, all of which influence the metabolism and reallocation of organic carbon within and outside the tissue. Second, significant differences in P metabolism between the two strains (Figure 7) may also contribute to the variation in DOC release. Nutrient supply is a key factor in the release of DOC from seaweed (Paine et al., 2021), and this could influence the effect of light intensity (Mueller et al., 2016). In addition, a recent study found variation in the C, N, and P composition of seaweed tissue and the released organic matter, indicating that elemental metabolism may be directly related to the release of organic matter from seaweed (Chen et al., 2020).
C, N, and P contents and ratios
The coupling between the biogeochemical cycling of elements and the service functions of ecosystems is one of the core issues in ecological research (Schröter et al., 2005). In 1934, Redfield discovered that the ratio of carbon (C), nitrogen (N), and phosphorus (P) in planktonic organisms in the ocean was roughly 106:16:1, closely mirroring the C:N:P ratio found in seawater (Redfield, 1934). This finding suggested that these three elements played a pivotal role in the biogeochemical cycles within oceanic ecosystems. By altering external environmental conditions, it is possible to influence the absorption rates of C, N, and P by marine organisms, thereby affecting the stoichiometric ratios of chemical elements. Both a recent study (Xu et al., 2022) and the present study found that an increase in light intensity significantly enhanced the C:N and C:P ratios of P. haitanensis thalli. This could be attributed to that the increase in light intensity enhancing the tissue C content but tending to decrease the tissue N and P contents (Figure 5). Changing the light intensity also altered the C:N ratio of the microalga Coccomyxa subellipsoidea by influencing the cell growth rate, nitrogen consumption, and carbon allocation (Liu and Wei, 2023). Similarly, the C:N and C:P ratios of phytoplankton communities increased with light intensity (Dickman et al., 2006). These results suggest that there are unequal effects of light intensity on the metabolism of C versus other elements of P. haitanensis and other algae. Further studies are needed to explore the underlying mechanisms.
In addition, significant differences were observed in the C:N, N:P and C:P ratios between the two strains of P. haitanensis (Figure 5). These strain-specific responses of elemental ratios to changing light intensity may be useful for the selection of new varieties. The altered elemental ratio can subsequently affects algal growth and carbon sequestration capacity, as well as play a pivotal role in ecosystem biogeochemical cycling (Hessen et al., 2013).
Conclusion
This study revealed that the growth rates of both strains of P. haitanensis initially increased under rising light intensity, peaking at 200 μmol m–2 s–1 before declining. The tissue C content increased with light intensity, while N and P contents decreased, leading to higher C:N and C:P ratios. The results indicate that elevated light intensity enhances C fixation while simultaneously suppressing N and P uptake. Notably, increasing light intensity significantly increased the DOC release rate of the W28–42 strain, unlike the WO15–4 strain, which showed no change. The POC release rates of both strains increased under higher light intensity, with W28–42 showing a greater increase. These strain-specific responses in organic carbon release to changing light intensity highlight the potential for improving carbon sequestration in aquaculture through tailored light management and breeding.
Data availability statement
The raw data supporting the conclusions of this article will be made available by the authors, without undue reservation.
Author contributions
ZZ: Formal Analysis, Methodology, Validation, Data curation, Supervision, Writing – original draft, Conceptualization, Software, Writing – review & editing, Investigation. WW: Methodology, Supervision, Writing – review & editing. YX: Supervision, Writing – review & editing, Investigation, Resources. DJ: Project administration, Resources, Writing – review & editing, Funding acquisition. CX: Resources, Funding acquisition, Methodology, Writing – review & editing, Project administration, Supervision. KX: Supervision, Methodology, Validation, Writing – review & editing, Funding acquisition, Writing – original draft, Resources.
Funding
The author(s) declare that financial support was received for the research and/or publication of this article. The authors gratefully acknowledge funding from the National Natural Science Foundation of China (42376109 and U21A20265), the Natural Science Foundation of Fujian Province (2022J01801), the China Agriculture Research System of MOF and MARA (CARS-50), the National Key Research and Development Plan for Key and Special Projects (2023YFD2400103), and the Regional Development Project of Fujian Science and Technology Program (2023N3002).
Acknowledgments
We thank LetPub (www.letpub.com.cn) for its linguistic assistance during the preparation of this manuscript.
Conflict of interest
The authors declare that the research was conducted in the absence of any commercial or financial relationships that could be construed as a potential conflict of interest.
Generative AI statement
The author(s) declare that no Generative AI was used in the creation of this manuscript.
Publisher’s note
All claims expressed in this article are solely those of the authors and do not necessarily represent those of their affiliated organizations, or those of the publisher, the editors and the reviewers. Any product that may be evaluated in this article, or claim that may be made by its manufacturer, is not guaranteed or endorsed by the publisher.
References
Barrón C., Duarte C. M. (2016). Dissolved organic carbon pools and export from the coastal ocean. GBC 29, 1725–1738. doi: 10.1002/2014GB005056
Beardall J., Ihnken S. S., Quigg A. (2009). Gross and net primary production: closing the gap between concepts and measurements. Aquat. Microb. Ecol. 56, 113–122. doi: 10.3354/ame01305
Britton D., Cornwall C., Revill A., Hurd C., Johnson C. (2016). Ocean acidification reverses the positive effects of seawater pH fluctuations on growth and photosynthesis of the habitat-forming kelp, Ecklonia radiata. Sci. Rep. 6, 26036. doi: 10.1038/srep26036
Brown J. H., Gillooly J. F., Allen A. P., Savage V. M., West G. B. (2004). Toward a metabolic theory of ecology. Ecology 85, 1771–1789. doi: 10.1890/03-9000
Chen J., Ji D., Xu Y., Chen C., Wang W., Xie C., et al. (2023). Effect of hyposaline stress on the release of dissolved organic carbon from five common macroalgal species. Front. Mar. Sci. 9, 1106703. doi: 10.3389/fmars.2022.1106703
Chen S., Xu K., Ji D., Wang W., Xu Y., Chen C., et al. (2020). Release of dissolved and particulate organic matter by marine macroalgae and its biogeochemical implications. Algal. Res. 52, 102096. doi: 10.1016/j.algal.2020.102096
Conitz J. M., Fagen R., Lindstrom S. C., Gerald Plumley F., Stekoll M. S. (2001). Growth and pigmentation of juvenile Porphyra torta (Rhodophyta) gametophytes in response to nitrate, salinity and inorganic carbon. J. Appl. Phys. 13, 423–431. doi: 10.1023/A:1011976431508
Dickman E. M., Vanni M. J., Horgan M. J. (2006). Interactive effects of light and nutrients on phytoplankton stoichiometry. Oecologia 149, 676–689. doi: 10.1007/s00442-006-0473-5
Dorte K. J., Paul L., Oscar S., Núria M., Pere M., Duarte C. M. (2018). Sequestration of macroalgal carbon: The elephant in the Blue Carbon room. Biol. Lett. 14, 20180236. doi: 10.1098/rsbl.2018.0236
Endo H., Moriyama H., Okumura Y. (2023). Photoinhibition and photoprotective responses of a brown marine macroalga acclimated to different light and nutrient regimes. Antioxidants 12, 357. doi: 10.3390/antiox12020357
Falkowski P. G., Raven J. A. (2013). Aquatic photosynthesis. (Princeton, NJ: Princeton University Press).
Finkel Z. V., Beardall J., Flynn K. J., Quigg A., Rees T. A. V., Raven J. A. (2010). Phytoplankton in a changing world: cell size and elemental stoichiometry. J. Plank. Res. 32, 119–137. doi: 10.1093/plankt/fbp098
Finkel Z. V., Quigg A., Raven J. A., Reinfelder J. R., Schofield O., Falkowski P. G. (2006). Irradiance and the elemental stoichiometry of marine phytoplankton. L&O 51, 2690–2701. doi: 10.4319/lo.2006.51.6.2690
Haas A. F., Naumann M. S., Struck U., Mayr C., El-Zibdah M., Wild C. (2010). Organic matter release by coral reef associated benthic algae in the Northern Red Sea. J. Exp. Mar. Biol. Ecol. 389, 53–60. doi: 10.1016/j.jembe.2010.03.018
Hall J. R., Herkül K., Baltar F., Hepburn C. D., Martin G. (2024). Shifts in macroalgae composition alters carbon flow in Coastal Baltic Sea ecosystems: implications for dissolved organic carbon bioavailability and flux. Front. Mar. Sci. 11, 1384165. doi: 10.3389/fmars.2024.1384165
Harrison P. J., Hurd C. L. (2001). Nutrient physiology of seaweeds: application of concepts to aquaculture. Cah Biol. Mar. 42, 71–82. doi: 10.1186/1750-1326-8-S1-O5
Hessen D. O., Elser J. J., Sterner R. W., Urabe J. (2013). Ecological stoichiometry: an elementary approach using basic principles. L&O 58, 2219–2236. doi: 10.4319/lo.2013.58.6.2219
Hessen D. O., Leu E., Færøvig P. J., Petersen S. F. (2008). Light and spectral properties as determinants of C: N: P-ratios in phytoplankton. Deep Sea Res. Pt II 55, 2169–2175. doi: 10.1016/j.dsr2.2008.05.013
Ho P. C., Nakajima S., Urabe J. (2024). Species-specific effects of leaf litter leachate on the aquatic microbial community and the ratio of heterotrophic to autotrophic biomass. FWB 69, 1727–1737. doi: 10.1111/fwb.v69.11
Hurd C. L., Harrison P. J., Bischof K., Lobban C. S. (2014). Seaweed Ecology and Physiology (Cambridge: Cambridge University Press).
Jiménez-Ramos R., Brun F. G., Pérez-Lloréns J. L., Vergara J. J., Delgado-Cabezas F., Sena-Soria N., et al. (2023). Resistance and recovery of benthic marine macrophyte communities to light reduction: insights from carbon metabolism and dissolved organic carbon (DOC) fluxes, and implications for resilience. Mar. Pollut. Bull. 188, 114630. doi: 10.1016/j.marpolbul.2023.114630
Lapointe B. E., Duke C. S. (1984). Biochemical strategies for growth of Gracilaria tikvahiae (Rhodophyta) in relation to light intensity and nitrogen availability. J. Phycol. 20, 488–495. doi: 10.1111/j.0022-3646.1984.00488.x
Li Z., Wakao S., Fischer B. B., Niyogi K. K. (2009). Sensing and responding to excess light. Annu. Rev. Plant Biol. 60, 239–260. doi: 10.1146/annurev.arplant.58.032806.103844
Lichtenthaler H. K., Wellburn A. R. (1983). Determinations of total carotenoids and chlorophylls a and b of leaf extracts in different solvents. Analysis 11, 591–592. doi: 10.1042/bst0110591
Lise Middelboe A., Juel Hansen P. (2007). Direct effects of pH and inorganic carbon on macroalgal photosynthesis and growth. Mar. Bio Res. 3, 134–144. doi: 10.1080/17451000701320556
Littler M. M., Littler D. S. (1980). The evolution of thallus form and survival strategies in benthic marine macroalgae: field and laboratory tests of a functional form model. Am. Nat. 116, 25–44. doi: 10.1086/283610
Liu Y., Wei D. (2023). Enhancing carbon dioxide fixation and co-production of protein and lutein in oleaginous coccomyxa subellipsoidea by a stepwise light intensity and nutrients feeding strategy. Bioresour. Technol. 376, 128885. doi: 10.1016/j.biortech.2023.128885
Mueller B., Den Haan J., Visser P. M., Vermeij M. J., Van Duyl F. C. (2016). Effect of light and nutrient availability on the release of dissolved organic carbon (DOC) by Caribbean turf algae. Sci. Rep. 6, 23248. doi: 10.1038/srep23248
Ni Z.-J., Zhang Y.-S., Chu H.-Y., Li B., Zhang M.-L., Sun Y.-Q., et al. (2022). Synergetic effect of light and nutrients on the release of dissolved organic carbon from juveniles of Saccharina japonica. Acta Hydrobiol. Sinica 46, 1909–1915. doi: 10.7541/2022.2021.0307
Norici A., Bazzoni A. M., Pugnetti A., Raven J. A., Giordano M. (2011). Impact of irradiance on the C allocation in the coastal marine diatom Skeletonema marinoi Sarno and Zingone. Plant Cell Environ. 34, 1666–1677. doi: 10.1111/j.1365-3040.2011.02362.x
Ortega A., Geraldi N. R., Alam I., Kamau A. A., Acinas S. G., Logares R., et al. (2019). Important contribution of macroalgae to oceanic carbon sequestration. Nat. Geosci. 12, 748–754. doi: 10.1038/s41561-019-0421-8
Paine E. R., Schmid M., Boyd P. W., Diaz-Pulido G., Hurd C. L. (2021). Rate and fate of dissolved organic carbon release by seaweeds: a missing link in the coastal ocean carbon cycle. J. Phycol. 57, 1375–1391. doi: 10.1111/jpy.13198
Raven J. A. (2011). The cost of photoinhibition. Physiol. plant. 142, 87–104. doi: 10.1111/j.1399-3054.2011.01465.x
Raven J. (2018). Blue carbon: past, present and future, with emphasis on macroalgae. Biol. Lett. 14, 20180336. doi: 10.1098/rsbl.2018.0336
Raven J. A., Hurd C. L. (2012). Ecophysiology of photosynthesis in macroalgae. Photosynth. Res. 113, 105–125. doi: 10.1007/s11120-012-9768-z
Redfield A. C. (1934). On the proportions of organic derivatives in sea water and their relation to the composition of plankton (Liverpool: University press of Liverpool).
Schröter D., Cramer W., Leemans R., Prentice I. C., Araújo M. B., Arnell N. W., et al. (2005). Ecosystem service supply and vulnerability to global change in Europe. Science 310, 1333–1337. doi: 10.1126/science.1115233
Shen Y., Benner R. (2018). Mixing it up in the ocean carbon cycle and the removal of refractory dissolved organic carbon. Sci. Rep. 8, 2542. doi: 10.1038/s41598-018-20857-5
Starr R. C., Zeikus J. A. (1993). Utex–the culture collection of algae at the University of Texas At Austin 1993 list of cultures. J. Phycol. 29, 1–106. doi: 10.1111j.0022-3646.1993.00001.x
Sun W., Wu W. G., Liu Y. (2024). The effects of temperature, light intensity, and photoperiod on the organic carbon release rate of Sargassum horneri seaweed. Prog. Fish. Sci. 45, 46–54. doi: 10.19663/j.issn2095-9869.20230309002
Wang W., Li S.-L., Zhong J., Slowinski S., Li S., Li C., et al. (2022). Carbonate mineral dissolution and photosynthesis-induced precipitation regulate inorganic carbon cycling along the karst river-reservoir continuum, SW China. J. Hydrol. 615, 128621. doi: 10.1016/j.jhydrol.2022.128621
Wernberg T., Thomsen M. S., Tuya F., Kendrick G. A., Staehr P. A., Toohey B. D. (2010). Decreasing resilience of kelp beds along a latitudinal temperature gradient: potential implications for a warmer future. Ecol. Lett. 13, 685–694. doi: 10.1111/j.1461-0248.2010.01466.x
West G. B., Brown J. H., Enquist B. J. (2001). A general model for ontogenetic growth. Nature 413, 628–631. doi: 10.1038/35098076
Xu K., Li M., Wang W., Xu Y., Ji D., Chen C., et al. (2022). Differences in organic carbon release between conchocelis and thalli of Pyropia haitanensis and responses to changes in light intensity and pH. Algal. Res. 61, 102574. doi: 10.1016/j.algal.2021.102574
Xu N., Wang W., Xu Y., Ji D., Chen C., Xie C., et al. (2021). Effects of nutrient availability on the release of dissolved and particulate organic carbon by Pyropia haitanensis and its implications. Front. Mar. Sci. 8, 696938. doi: 10.3389/fmars.2021.696938
Yang Y., Chai Z., Wang Q., Chen W., He Z., Jiang S. (2015). Cultivation of seaweed Gracilaria in Chinese coastal waters and its contribution to environmental improvements. Algal. Res. 9, 236–244. doi: 10.1016/j.algal.2015.03.017
Yi Y., Li S.-L., Zhong J., Wang W., Chen S., Bao H., et al. (2022). The influence of the deep subtropical reservoir on the karstic riverine carbon cycle and its regulatory factors: Insights from the seasonal and hydrological changes. Water Res. 226, 119267. doi: 10.1016/j.watres.2022.119267
Keywords: carbon metabolism, dissolved organic carbon, growth, light intensity, particulate organic carbon, Pyropia haitanensis
Citation: Zhang Z, Wang W, Xu Y, Ji D, Xie C and Xu K (2025) Strain-specific responses of Pyropia haitanensis to light intensity in growth, carbon content, and organic carbon release. Front. Mar. Sci. 12:1596003. doi: 10.3389/fmars.2025.1596003
Received: 19 March 2025; Accepted: 14 April 2025;
Published: 09 May 2025.
Edited by:
Fabio Carneiro Sterzelecki, Federal Rural University of the Amazon, BrazilCopyright © 2025 Zhang, Wang, Xu, Ji, Xie and Xu. This is an open-access article distributed under the terms of the Creative Commons Attribution License (CC BY). The use, distribution or reproduction in other forums is permitted, provided the original author(s) and the copyright owner(s) are credited and that the original publication in this journal is cited, in accordance with accepted academic practice. No use, distribution or reproduction is permitted which does not comply with these terms.
*Correspondence: Chaotian Xie, Y3R4aWVAam11LmVkdS5jbg==; Kai Xu, a2FpeHVAam11LmVkdS5jbg==