- 1Department of Microbiology, School of Medicine, Yangzhou University, Yangzhou, China
- 2Jiangsu Key Laboratory of Zoonosis/Jiangsu Co-Innovation Center for Prevention and Control of Important Animal Infectious Diseases and Zoonoses, Yangzhou University, Yangzhou, China
- 3Jiangsu Key Laboratory of Integrated Traditional Chinese and Western Medicine for Prevention and Treatment of Senile Diseases, Yangzhou, China
- 4Department of Pharmacy, Suzhou Hospital of Integrated Traditional Chinese and Western Medicine, Suzhou, China
Acinetobacter baumannii is an important, opportunistic nosocomial pathogen that causes a variety of nosocomial infections, and whose drug resistance rate has increased in recent years. The CRISPR-Cas system exists in several bacteria, providing adaptive immunity to foreign nucleic acid invasion. This study explores whether CRISPR-Cas is related to drug resistance. Antibiotics were used to treat strains ATCC19606 and AB43, and the expression of CRISPR-related genes was found to be changed. The Csy proteins (Csy1–4) were previously detected to promote target recognition; however, the potential function of csy1 gene is still unknown. Thus, the RecAb homologous recombination system was utilized to knock out the csy1 gene from A. baumannii AB43, which carries the Type I-Fb CRISPR-Cas system, and to observe the drug resistance changes in wild-type and csy1-deleted strains. The AB43Δcsy1 mutant strain was found to become resistant to antibiotics, while the wild-type strain was sensitive to antibiotics. Moreover, transcriptome analysis revealed that the csy1 gene regulates genes encoding CRISPR-Cas-related proteins, drug-resistant efflux pumps, membrane proteins, and oxidative phosphorylation-related proteins, inhibiting antimicrobial resistance in A. baumannii. The in vitro resistance development assay revealed that the complete CRISPR-Cas system could inhibit the development of bacterial resistance. Our findings expand our understanding of the role of CRISPR-Cas csy1 gene in A. baumannii and link the CRISPR-Cas system to the biogenesis of bacterial drug-resistant structures.
Introduction
Acinetobacter baumannii, a non-fermented Gram-negative bacterium, is one of the primary causes of nosocomial infections worldwide. It mainly causes ventilator-associated pneumonia and blood, urinary tract, skin, and soft tissue infections, particularly in critically ill patients in the intensive care unit (1). Over the past few years, the drug resistance rate of A. baumannii has gradually increased (2). Due to the treatment challenges posed by emerging and increasing drug resistance, multi-drug resistant (MDR) A. baumannii poses a global threat to human health (3).
The CRISPR-Cas system is an immune system used in prokaryotes resisting invasion of foreign genetic elements. It generally consists of three parts: a CRISPR array, a leader sequence, and Cas-related proteins (4). In general, three different stages have been described in the CRISPR-Cas immune response: (i) adaptation, (ii) CRISPR (Cr) RNA expression and maturation, and (iii) interference (5). The CRISPR-Cas system directs sequence-specific cleavage of phage and plasmid nucleic acids using nucleases programmed by small RNAs (6). The CRISPR-Cas system also inhibits conjugation and transformation, thereby limiting horizontal gene transfer. As the latter significantly affects bacterial evolution, the spread of antibiotic resistance and virulence determinants is the most pronounced (7). Several studies have demonstrated that the CRISPR-Cas system is associated with bacterial drug resistance. A previous study has demonstrated that a variety of genes are regulated by the Campylobacter jejuni II CRISPR-Cas9 system to promote bacterial drug resistance (8). The CRISPR/Cas9 system can mediate MDR Escherichia coli to restore antibiotic sensitivity (9). The Cas9-dependent CRISPR-Cas system of the intracellular bacterial pathogen Francisella novicida enhances antibiotic resistance by strengthening envelope integrity (10). In the I-F CRISPR-Cas system, multiple Cas proteins (Csy1–4) and CRISPR RNA (CrRNA) form a surveillance complex (Csy complex) for target recognition (11). Studies have shown that Csy proteins (Csy1–4) promote target recognition by enhancing sequence-specific hybridization between CRISPR RNA and complementary target sequences (12).
The Type I CRISPR-Cas system is the most widely distributed in nature (13). The unique feature of Type I-F CRISPR-Cas is the fusion of Cas2 and Cas3 (Cas2/3), together with Cas1 mediating the integration of the spacer into the CRISPR site (14, 15). There are two primary subtypes of the I-F CRISPR-Cas system known in A. baumannii, namely, Type I-Fa and Type I-Fb (16–18). The composition of Type I-Fa and Type I-Fb is illustrated in Figure 1. The Csy1 protein is missing in the Type I-Fa CRISPR-Cas system; however, the other components contain additional domains that can compensate for the role of Csy1 protein (19). Moreover, the role of csy1 gene in antimicrobial resistance is still unknown.
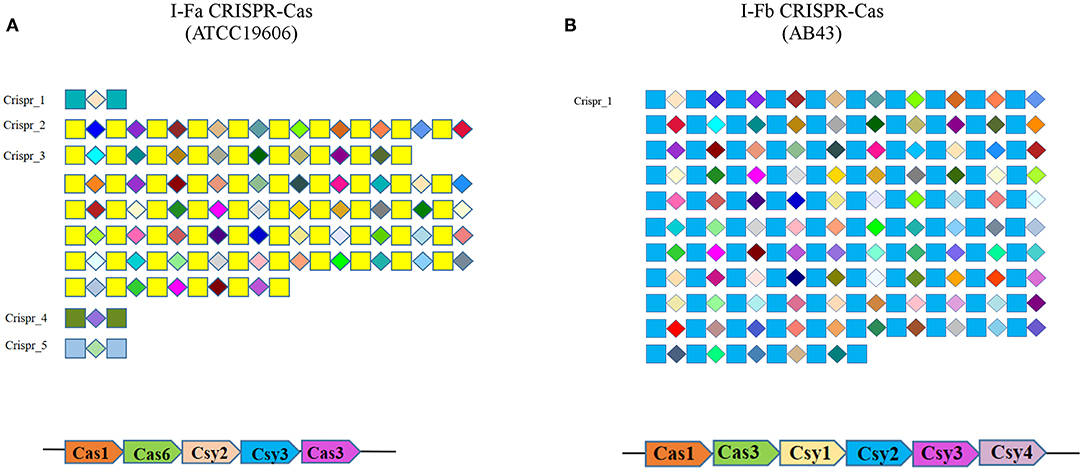
Figure 1. Repeat (squares), spacer (diamonds), and the composition of different types of CRISPR-cas system of A. baumannii. (A) ATCC19606; (B) AB43.
Our study aimed to research whether antibiotics affect the expression of CRISPR-Cas-related genes in A. baumannii to determine the relationship of csy1 gene with antimicrobial resistance.
Materials and Methods
Bacterial Strains and Plasmids
This study included standard strain A. baumannii ATCC19606 (GenBank: CP045108.1, https://www.ncbi.nlm.nih.gov/nuccore/CP045108.1), which carries the complete CRISPR-Cas subtype I-Fa system, and clinical isolates of A. baumannii AB43 (GenBank: CP083182.1, https://www.ncbi.nlm.nih.gov/nuccore/2095284784), carrying the complete CRISPR-Cas subtype I-Fb system. Moreover, AB133, ABF7, and ABE5 are clinical isolates containing an incomplete I-Fa system, an incomplete I-Fb system, or no CRISPR-Cas system, respectively (Table 1). The clinical isolates used in this study were isolated from the Affiliated Hospital of Yangzhou University and identified in our laboratory. The bacterial strains and plasmids used in this study are listed in Table 1.
CRISPR-Cas Finder (https://crisprcas.i2bc.paris-saclay.fr/CrisprCasFinder/Index) was used to determine the existence of the CRISPR-Cas system and spacers in the genomes of strains ATCC19606 and AB43. The existence of clinical strains (AB133, ABF7, and ABE5) with CRISPR-Cas genes was determined by PCR (primers are shown in Supplementary Table 1).
Antimicrobial Susceptibility Assessment
Strains with different CRISPR-Cas systems were subjected to determine the minimum inhibitory concentrations (MICs) of doxycycline, minocycline, tigecycline, ceftriaxone, imipenem, gentamicin, kanamycin, ciprofloxacin, polymyxin B, colistin, erythromycin, and rifampin to detect the relationship of CRISPR-Cas system integrity with drug resistance. The MICs of antibiotics were measured using the standard broth microdilution method, according to the Clinical and Laboratory Standard Institute 2020 guideline.
Real-Time Quantitative Reverse Transcription-PCR (qRT-PCR) to Detect CRISPR Gene Expression After Exposure to Antimicrobials
Strains ATCC19606 and AB43 were grown to the early-exponential phase and incubated with the MICs of antibiotics for 4 h. Total RNA was extracted from the bacteria using a total RNA extraction kit (TIANGEN, Beijing, PR China). The HISCRIPT 1st strand cDNA synthesis kit (Vazyme, Nanjing, China) was used to synthesize cDNA. A housekeeping gene (16S rRNA gene) was used as an internal control for each sample. qRT-PCR was performed on an ABI 7,500 RT-PCR system (Applied Biosystems, CA, USA), and SYBR Green was the dye (Vazyme, Nanjing, China) utilized. Primers used for qRT-PCR are illustrated in Supplementary Table 2. The relative gene expression levels were compared with those of 16S rRNA by the 2−ΔΔCt method.
Construction of the csy1 Gene Deletion Mutant
To delete the csy1 gene from A. baumannii AB43, pKD4 was used as a template to amplify the complete kanamycin cassette gene fragments. The PCR amplification products were identified by 1% agarose gel electrophoresis and purified using the FastPure Gel DNA Extraction Mini Kit (Vazyme). A. baumannii carrying RecAb on pMMB67EH (pAT04) was inoculated into liquid Luria Bertani (LB) medium containing carbenicillin to preserve the plasmid. IPTG was added to bacteria to the mid-log phase with a final concentration of 2 mM, incubated at 37°C for 3 h. Then bacteria were collected, washed thrice with 10% ice-cold glycerol, 1,000-fold concentrated. One microgram recombinant engineering PCR products were electrotransformed into A. baumannii AB43 competent cells (100 μL, ~1010 bacteria) in 2 mm cuvette at 1.8 kV. The bacteria are grown in a 4 mL rich medium containing 2 mM IPTG for 4 h, centrifuged at 4,000 rpm for 10 min, and removed the supernatant, added in 100 μL LB. Then, positive clones were selected from LB agar medium containing 50 μg/mL kanamycin incubated overnight at 37°C. Screening primers outside the homology region were used to confirm the insertion of the kanamycin cassette (20). Then the following steps were made to make the kanamycin cassette lost in the AB43 Δcsy1::kan mutant strains. First, pAT03 (pMMB67EH with flippase recombinase) was electrotransformed into AB43 Δcsy1::kan mutant strains. Then, the bacteria were resuscitated and cultured using LB with 2 mM IPTG for 1 h. Positive clones were then selected on LB agar plates containing carbenicillin (75 μg/mL) incubated overnight at 37°C. The csy1 gene knock-out mutation was confirmed by PCR and DNA sequencing (Tsingke Biotechnology Co., China).
Complementation of Δcsy1 Mutant Strain
The pMMB67EH vector was utilized to generate a csy1 gene complementation vector. An 1,110 bp fragment containing an open reading frame of csy1 gene from the genome was amplified and ligated to the pMMB67EH vector, then the recombinant plasmid was electrotransformed into the AB43Δcsy1. Complementation vector-transformed Δcsy1 mutants were selected on LB agar plates containing 50 μg/mL kanamycin and 10 μg/mL tetracycline. Positive clones were verified by colony PCR using primers and DNA sequencing (Tsingke Biotechnology Co, China). The expression of the csy1 gene in AB43, complemented mutant, and Δcsy1 mutant strains was determined by qRT-PCR as described above.
Antimicrobial Susceptibility Testing of Mutant Strains and Resistance Development Studies
For in vitro resistance development of AB43, AB43Δcsy1/pcsy1, and AB43Δcsy1, antibiotics sensitive to all three strains were selected, and then resistance development experiments were conducted. Strains were exposed separately to polymyxin B and rifampin for step-wise selection. Strains at the exponential phase were diluted 1: 1,000 into fresh MHB medium supplement with 0.5 × MIC or 0.25 × MIC of polymyxin B and rifampin. After being cultured at 37°C for 24 h at 200 rpm, the MIC of each drug was determined by broth microdilution as mentioned before. The process was repeated for 10 generations. Moreover, the ratio of the MIC obtained from every generation to the MIC at the first generation (first contact) was determined. The data are expressed as the relative increase of MIC per generation (21).
Transcriptomic Analysis
The RNA-Seq analysis of AB43 and AB43Δcsy1 was performed as proposed by Kesavan et al. (22). Total RNAs of AB43 and AB43Δcsy1 were extracted from cell cultures at the log phase using an RNA extraction kit (TIANGEN, Beijing, PR China) and quantified using a Nanodrop spectrophotometer (Thermo Scientific, Waltham, MA, USA) by the ratio of absorbance (260 nm/280 nm). cDNA synthesis, library generation, and data analysis were performed by Shanghai Sheng gong Bioengineering Company, and transcriptome sequencing was conducted using Illumina HiSeqTM.
Results
Spacer Identification and CRISPR Analysis of A. baumannii
CRISPR-Cas Finder was used to determine the number and sequence of spacers in the CRISPR-Cas repeat array of ATCC19606 and AB43. The results are illustrated in Figure 1. The results demonstrate that ATCC19606 carries five CRISPRs (Crispr_1, Crispr_2, Crispr_3, Crispr_4, and Crispr_5), where the number of spacers in the CRISPR locus of Crispr_1, Crispr_2, Crispr_3, Crispr_4, and Crispr_5 is 1, 18, 45, 1, and 1, respectively. The Type I-Fa Cas cluster consists of Cas1, Cas6, Csy3, Csy2, and Cas3-Cas2 (Cas3). Strain AB43 carries one confirmed CRISPR, which contains 105 spacers, and the Type I-Fb Cas cluster contains Cas1, Cas3-Cas2 (Cas3), Csy1, Csy2, Csy3, and Csy4.
Antimicrobial Susceptibility of Selected Strains
The status of the CRISPR-Cas system in selected A. baumannii strains is demonstrated in Table 2. To investigate the relationship between csy1 gene and drug resistance, the relationship between the CRISPR-Cas carrier rate and drug resistance was first investigated. The strains with a complete CRISPR-Cas system (ATCC19606 and AB43) were sensitive to 12 antibiotics tested. However, strains with an incomplete set of CRISPR-Cas-related genes (A133 and F7) or without the CRISPR-Cas system (E5) were resistant to most of the antibiotics tested. The results are shown in Table 3. Thus, the CRISPR-Cas system was speculated to be related to the drug resistance of A. baumannii.
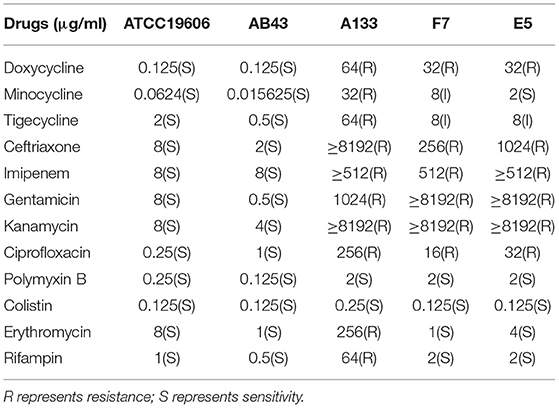
Table 3. Minimum inhibitory concentrations (MICs) of strains with different types of CRISPR-Cas system.
CRISPR-Cas Gene Expression in ATCC19606 and AB43 Exposed to Antibiotics
qRT-PCR analysis of CRISPR-Cas-related cas and csy genes was performed to further study the relationship between the CRISPR-Cas system and antimicrobial resistance. The expression of the CRISPR-Cas-related genes cas and csy changed under antibiotic pressure (Figures 2A,B). Downregulation of cas1, cas3, cas6, csy2, csy3, and csy4 was detected in ATCC19606 and AB43 when treated with most antibiotics. However, csy1 gene in AB43 was upregulated when treated with most antibiotics, and only downregulated when treated with doxycycline and kanamycin.
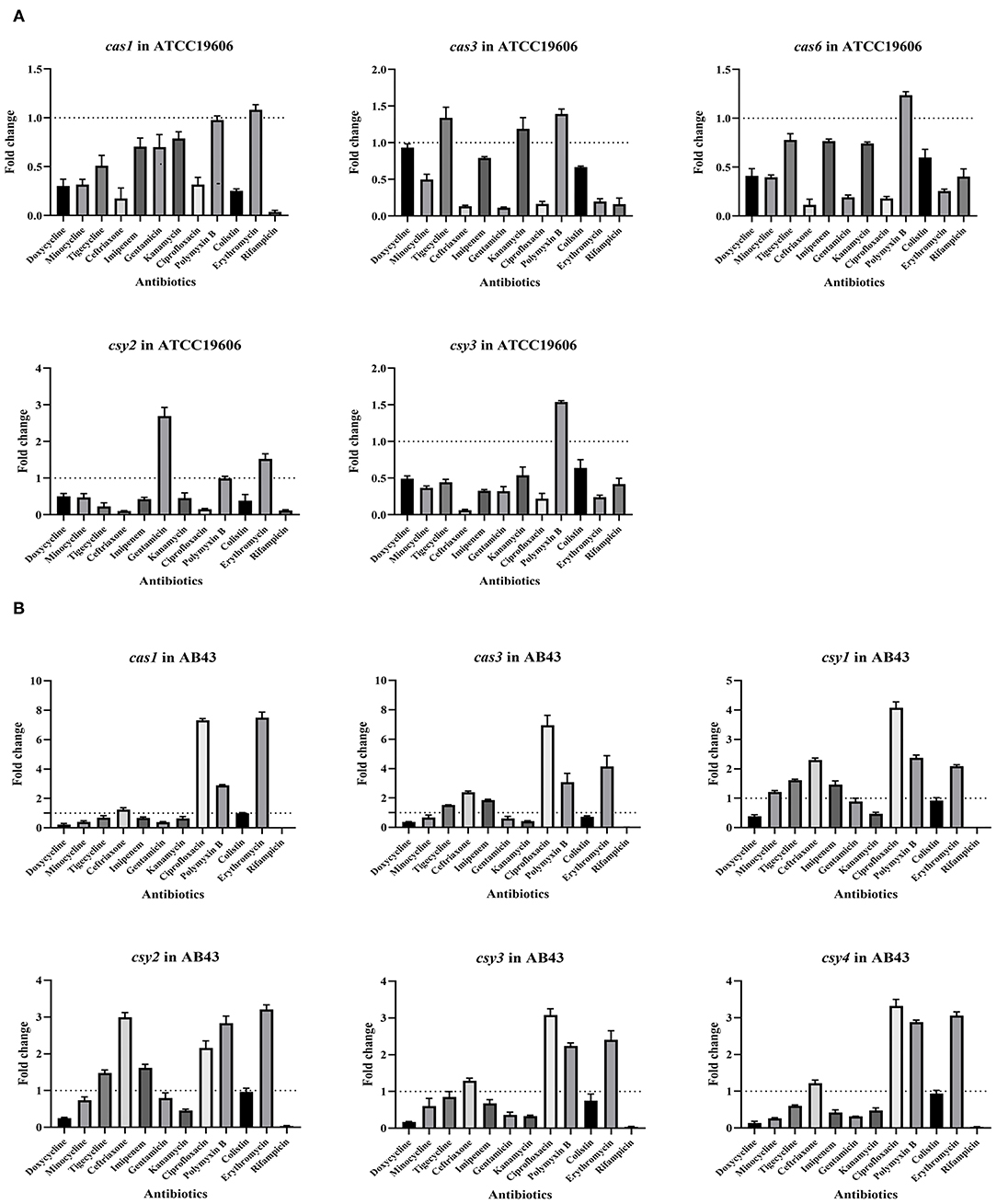
Figure 2. CRISPR-cas gene expression in strains after exposure to antibiotics. (A) CRISPR-related genes (cas1, cas3, cas6, csy2, and csy3) expression in ATCC19606 against antimicrobials; (B) CRISPR-related genes (cas1, cas3, csy1, csy2, csy3, and csy4) expression against antimicrobials in AB43.
The Role of csy1 Gene in Antimicrobial Susceptibility
The above studies demonstrated that an integrated CRISPR-Cas system might inhibit the drug resistance of A. baumannii. Further, when A. baumannii was exposed to tested antibiotics, only csy1 gene was upregulated. However, little is known regarding the role of csy1 gene in the drug resistance of A. baumannii. A csy1 gene knock-out strain was constructed. The expressions of csy1 gene RNA in the wild type (AB43), csy1 gene deletion (AB43Δcsy1), and csy1 gene complementation (AB43Δcsy1/pcsy1) strains were determined by qRT-PCR. The expression of csy1 gene RNA was significantly decreased by deletion in the csy1 gene deletion mutant, and restored to wild-type levels in the csy1 gene complementation strain (Figure 3). The antimicrobial susceptibility of AB43, the complemented mutant, and Δcsy1 mutant strains to 12 drugs was evaluated. The results are shown in Table 4. A 16- to 512-fold (doxycycline, tigecycline, ceftriaxone, imipenem, ciprofloxacin, erythromycin) increase in susceptibility was observed in the Δcsy1 mutant strain compared with its wild (AB43) strain. Moreover, a >1,024-fold increase of MIC (minocycline, gentamicin, and kanamycin) was observed in the Δcsy1 mutant strain compared with AB43. The MICs of polymyxin B, colistin, and rifampin were similar in the three strains.
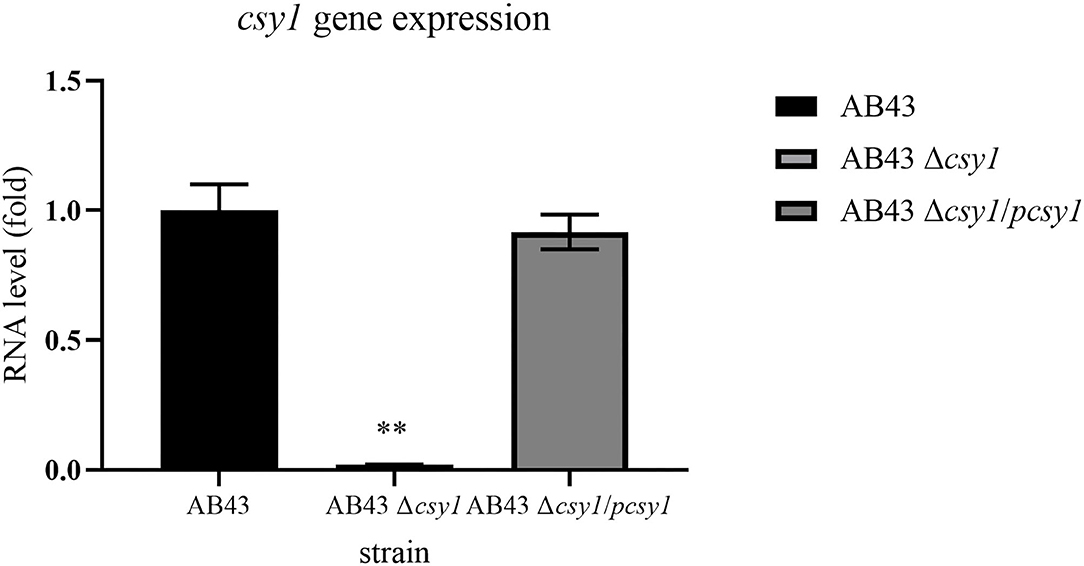
Figure 3. The RNA level of csy1 gene in wild type (AB43), csy1 gene deletion (AB43Δcsy1), csy1gene complementation (AB43Δcsy1/pcsy1) strains. The RNA level in AB43 was set as 1, and those in strains AB43Δcsy1 and AB43Δcsy1/pcsy1 were calculated accordingly. Data are presented as means ± SEM from three independent experiments. **p < 0.01.
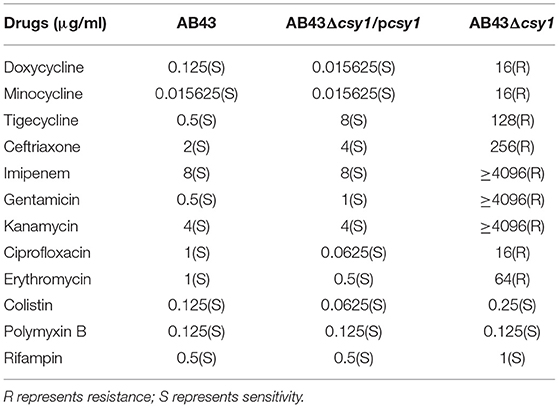
Table 4. Minimum inhibitory concentrations (MICs) of AB43, complemented mutant, and Δcsy1 mutant strains to different antibiotics.
The Role of csy1 Gene in Resistance Development
Based on the results that csy1 gene can regulate the drug resistance of A. baumannii, a drug resistance development assay was done to further elucidate its role in antibiotic resistance. No significant differences were found between AB43, AB43Δcsy1/pcsy1, and AB43Δcsy1 in resistance to polymyxin B and rifampin (Table 4); thus, both were chosen for the resistance induction experiment. There was no significant difference among AB43, AB43Δcsy1/pcsy1, and AB43Δcsy1 in resistance to polymyxin B until the sixth step of selection (2 μg/mL). At 2 μg/mL polymyxin B, only AB43Δcsy1 exhibited growth (Figure 4). The drug resistance of rifampicin showed a difference at the third selection step and a 16-fold increase at the eighth selection step (Figure 4). The results suggested that the csy1 gene mutant strain drug resistance developed significantly faster than that of the wild strain.
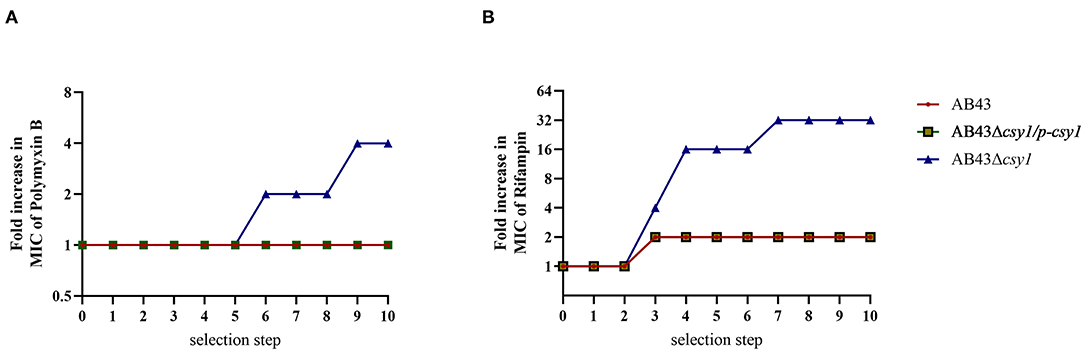
Figure 4. Polymyxin B and rifampicin resistance gains during continuous passaging. (A) Polymyxin B; (B) Rifampicin.
Transcriptomic Analysis of the csy1 Gene Knock-Out Mutant
Transcriptome analyses of AB43 and AB43Δcsy1 were performed, which demonstrated that genes encoding efflux protein, the 50S/30S ribosomal proteins, proteins involved in energy metabolism, and cytochrome C were upregulated in AB43Δcsy1 (Figure 5). The upregulation of the gene encoding the efflux pump was reported to be associated with increased antimicrobial resistance (23). Additionally, the increased synthesis of ATP could promote drug efflux. Several downregulated genes in the mutant strain were also detected. After the csy1 gene was knocked out, the expression of CRISPR-Cas-related genes was downregulated. The related proteins of the CRISPR-Cas system were speculated to influence each other as a whole. The decreased expression of membrane-related proteins indicated that the CRISPR-Cas system might regulate cell membrane formation.
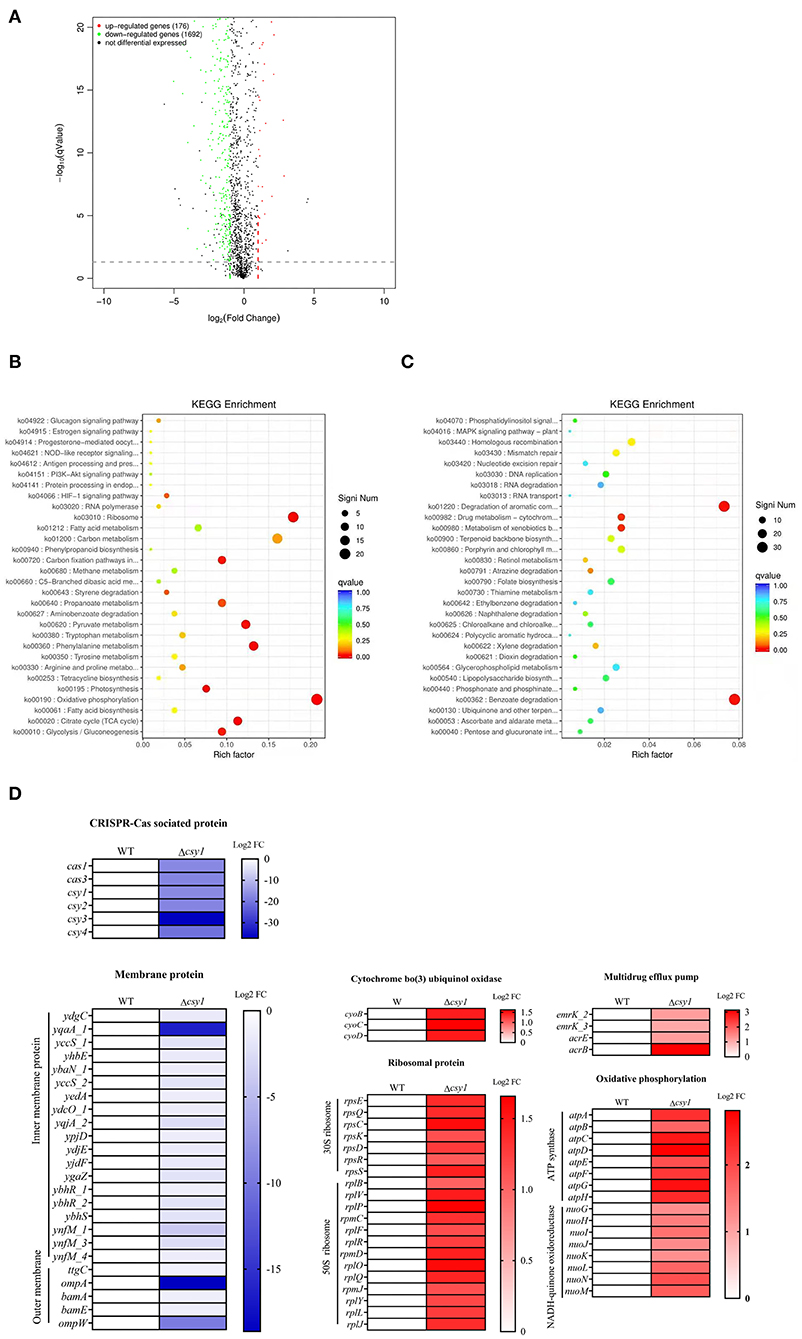
Figure 5. Transcriptome analysis of AB43 and AB43Δcsy1. (A) Differential gene volcano map; (B) Kyoto Encyclopedia of Genes and Genomes (KEGG) enrichment analysis of up-regulation genes; (C) KEGG enrichment analysis of down-regulation genes; the color of the dot represents the size of the Q-value, and the size of the dot represents the number of differential genes; (D) Selected differential expression genes involved in CRISPR-Cas related protein, ribosome protein, oxidative phosphorylation related protein, a membrane-associated related protein, and multidrug efflux pump protein.
Discussion
The primary role of the CRISPR-Cas system is bacterial defense against phages, plasmids, and other foreign DNA. Some recent studies showed that the CRISPR-Cas system might play a role in antibiotic resistance of bacteria (24). The effect of the CRISPR-Cas system on antibiotic resistance varies in different bacteria. The CRISPR-Cas system promotes resistance in C. jejuni and inhibits resistance in E. coli and E. fecium (8, 9, 25). However, the relationship of the CRISPR-Cas system with antibiotic resistance in A. baumannii is not fully elucidated. By investigating the carrying situation of the CRISPR-Cas system and the drug resistance level of selected strains, we assessed whether the CRISPR-Cas system plays a role in A. baumannii antibiotic resistance. The results showed that the complete CRISPR-Cas system might inhibit bacterial drug resistance. qRT-PCR analysis was conducted to measure the expression levels of Type I-Fa and I-Fb CRISPR-Cas system-related genes after antibiotic treatment. The results demonstrated that the expressions of cas and csy genes were modified.
With the excessive use of antibiotics, the bacterial resistance rate has continued to increase. The infection rate of MDR pathogens is increasing. Thus, the development of different strategies to combat antimicrobial resistance is critical (4). Moreover, research on the relationship between CRISPR-Cas and bacterial resistance is primarily focused on the cas3 and cas9 genes (26). The cas3 gene in Streptococcus mutants can regulate biofilm formation and the ability to resist fluoride (27). Sampson et al. proved that the CRISPR-Cas endonuclease gene cas9 together with tracrRNA and ScaRNA is essential for enhancing the stability of the bacterial envelope and promoting antibiotic resistance (10). However, the relationship between the csy1 gene and drug resistance in bacteria has not been previously reported. To the best of our knowledge, this is the first study regarding the relationship between csy1 gene and drug resistance in A. baumannii. Preliminary studies have demonstrated that csy1 gene can regulate the drug resistance of A. baumannii and maintain its sensitivity to antibiotics. After knocking out the csy1 gene, the bacterial resistance level increased. Simultaneously, the drug resistance experiment showed that csy1 gene could inhibit the drug resistance development of bacteria. The complete CRISPR-Cas system may inhibit its drug resistance. The results are similar to studies on Enterococcus fecalis, which found that the lack of CRISPR genes is related to species, multidrug resistance, and major drug resistance-related genes (28).
The study by Aydin et al. suggested that the CRISPR-Cas system may interfere with the acquisition of resistant plasmids, thereby maintaining the sensitivity of these strains (9). Klebsiella pneumoniae strains detected that the spacers in the CRISPR array matched the genome of the plasmid or phage, some containing resistance genes (29). In Francis bacteria, CRISPR-Cas can enhance the integrity of its envelope, leading to resistance to several membrane stressors, including antibiotics, and increasing antibiotic resistance (10). Transcriptome analysis was conducted to assess the mechanism of csy1 gene inhibiting the drug resistance of A. baumannii. The csy1 gene mutant strain has a higher expression of drug efflux pump, ATP synthesis-related, and ribosomal genes, as well as a lower expression of CRISPR-Cas-related and membrane-related genes.
Thus, with the steady increase in MDR bacterial infections, the innovation of novel therapies to combat MDR bacteria is critical. Existing studies have demonstrated that the CRISPR-Cas system delivered by phages can sequentially eliminate Staphylococcus aureus (30). CRISPR-encoded presentation plasmids or CRISPR-Cas antibacterial drugs can reduce the occurrence of antibiotic resistance in Enterococci (31). The delivery of CRISPR-Cas9 effectively removes antibiotic resistance in vitro by targeting plasmid-borne resistance genes (32). Our work main revealed that the csy1 gene participates in the regulation of drug resistance in A. baumannii. The present study has several limitations. It is unknown how csy1 gene, regulates drug resistance in A. baumannii. Moreover, we only performed csy1 gene knock-out experiments in AB43 (I-Fb CRISPR-Cas), and more strains are needed to generalize our results. Thus, future studies should focus on assessing the mechanism by which CRISPR-Cas regulates drug resistance. Moreover, deploying the Type I CRISPR-Cas system as an antimicrobial to treat drug-resistant A. baumannii infection is an attractive strategy compared with conventional antibiotic therapy. It is also necessary to explore the effect of other CRISPR-Cas components in modulating aspects of bacterial physiology such as virulence and drug resistance.
Conclusions
In conclusion, we studied the relationship of the CRISPR-Cas system with antibiotic resistance in A. baumannii. Our results revealed that the expression of CRISPR-Cas related genes was changed under antibiotic pressure and that the presence of the csy1 gene has an inhibitory effect on the drug resistance of A. baumannii. Deletion of the csy1 gene in A. baumannii strain AB43 made it resistant to most of the antibiotics tested. In addition, this study extends our understanding of resistance regulation of A. baumannii and provides a new direction for studying the functions of CRISPR-Cas systems in drug resistance. Future work can be focused on their functions that extend beyond the general disruption of invading foreign DNA and may clarify how CRISPR-Cas systems contribute to bacterial resistance and virulence. This knowledge will be useful in the exploration of new antibacterial strategies.
Data Availability Statement
The datasets presented in this study can be found in online repositories. The names of the repository/repositories and accession number(s) can be found at: NCBI SRA; SRR17253291 and SRR17253292.
Author Contributions
GL and TG contributed to the design of the study. TG, XS, ML, and YW contributed to the acquisition of the data. GL, TG, and XS contributed to the analysis of the data. All authors contributed to data interpretation, drafting the manuscript, critically revising the manuscript for important intellectual content, and approved the final version of the manuscript.
Funding
This work was supported by the National Natural Science Foundation of China (82073611 and 82002186), the Natural Science Foundation of the Higher Education Institutions of Jiangsu Province (Grant Number: 19KJB310002).
Conflict of Interest
The authors declare that the research was conducted in the absence of any commercial or financial relationships that could be construed as a potential conflict of interest.
Publisher's Note
All claims expressed in this article are solely those of the authors and do not necessarily represent those of their affiliated organizations, or those of the publisher, the editors and the reviewers. Any product that may be evaluated in this article, or claim that may be made by its manufacturer, is not guaranteed or endorsed by the publisher.
Acknowledgments
The authors are grateful for the manuscript correction by Dr. Benjamin Chen from Yale University School of Medicine PET center.
Supplementary Material
The Supplementary Material for this article can be found online at: https://www.frontiersin.org/articles/10.3389/fmed.2022.797104/full#supplementary-material
References
1. Sarshar M, Behzadi P, Scribano D, Palamara AT, Ambrosi C. Acinetobacter baumannii: an ancient commensal with weapons of a pathogen. Pathogens. (2021) 10:387. doi: 10.3390/pathogens10040387
2. Liang C, Zhang X, Zhou L, Meng G, Zhong L, Peng P. Trends and correlation between antibacterial consumption and carbapenem resistance in gram-negative bacteria in a tertiary hospital in China from 2012 to 2019. BMC Infect Dis. (2021) 21:444. doi: 10.1186/s12879-021-06140-5
3. Kyriakidis I, Vasileiou E, Pana ZD, Tragiannidis A. Acinetobacter baumannii antibiotic resistance mechanisms. Pathogens. (2021) 10:373. doi: 10.3390/pathogens10030373
4. Shabbir MAB, Shabbir MZ, Wu Q, Mahmood S, Sajid A, Maan MK, et al. CRISPR-cas system: biological function in microbes and its use to treat antimicrobial resistant pathogens. Ann Clin Microbiol Antimicrob. (2019) 18:21. doi: 10.1186/s12941-019-0317-x
5. Shmakov SA, Makarova KS, Wolf YI, Severinov KV, Koonin EV. Systematic prediction of genes functionally linked to CRISPR-Cas systems by gene neighborhood analysis. Proc Natl Acad Sci USA. (2018) 115:E5307–16. doi: 10.1073/pnas.1803440115
6. Price VJ, McBride SW, Hullahalli K, Chatterjee A, Duerkop BA, Palmer KL. Enterococcus faecalis CRISPR-Cas is a robust barrier to conjugative antibiotic resistance dissemination in the murine intestine. mSphere. (2019) 4:e00464–19. doi: 10.1128/mSphere.00464-19
7. Watson BNJ, Staals RHJ, Fineran PC. CRISPR-cas-mediated phage resistance enhances horizontal gene transfer by transduction. mBio. (2018) 9:e02406–17. doi: 10.1128/mBio.02406-17
8. Shabbir MA, Wu Q, Shabbir MZ, Sajid A, Ahmed S, Sattar A, et al. The CRISPR-cas system promotes antimicrobial resistance in campylobacter jejuni. Future Microbiol. (2018) 13:1757–74. doi: 10.2217/fmb-2018-0234
9. Aydin S, Personne Y, Newire E, Laverick R, Russell O, Roberts AP, et al. Presence of Type I-F CRISPR/Cas systems is associated with antimicrobial susceptibility in Escherichia coli. J Antimicrob Chemother. (2017) 72:2213–8. doi: 10.1093/jac/dkx137
10. Sampson TR, Napier BA, Schroeder MR, Louwen R, Zhao J, Chin CY, et al. A CRISPR-Cas system enhances envelope integrity mediating antibiotic resistance and inflammasome evasion. Proc Natl Acad Sci USA. (2014) 111:11163–8. doi: 10.1073/pnas.1323025111
11. Hong S, Ka D, Yoon SJ, Suh N, Jeong M, Suh JY, et al. CRISPR RNA and anti-CRISPR protein binding to the xanthomonas albilineans Csy1-Csy2 heterodimer in the type I-F CRISPR-Cas system. J Biol Chem. (2018) 293:2744–54. doi: 10.1074/jbc.RA117.001611
12. Wiedenheft B, van Duijn E, Bultema JB, Waghmare SP, Zhou K, Barendregt A, et al. RNA-guided complex from a bacterial immune system enhances target recognition through seed sequence interactions. Proc Natl Acad Sci USA. (2011) 108:10092–7. doi: 10.1073/pnas.1102716108
13. Makarova KS, Wolf YI, Iranzo J, Shmakov SA, Alkhnbashi OS, Brouns SJJ, et al. Evolutionary classification of CRISPR-Cas systems: a burst of class 2 and derived variants. Nat Rev Microbiol. (2020) 18:67–83. doi: 10.1038/s41579-019-0299-x
14. Fagerlund RD, Wilkinson ME, Klykov O, Barendregt A, Pearce FG, Kieper SN, et al. Spacer capture and integration by a type I-F Cas1-Cas2-3 CRISPR adaptation complex. Proc Natl Acad Sci USA. (2017) 114:E5122–8. doi: 10.1073/pnas.1618421114
15. Pausch P, Müller-Esparza H, Gleditzsch D, Altegoer F, Randau L, Bange G. Structural variation of type I-F CRISPR RNA guided DNA surveillance. Mol Cell. (2017) 67:622–32.e624. doi: 10.1016/j.molcel.2017.06.036
16. Karah N, Samuelsen O, Zarrilli R, Sahl JW, Wai SN, Uhlin BE. CRISPR-cas subtype I-Fb in acinetobacter baumannii: evolution and utilization for strain subtyping. PLoS ONE. (2015) 10:e0118205. doi: 10.1371/journal.pone.0118205
17. Touchon M, Cury J, Yoon EJ, Krizova L, Cerqueira GC, Murphy C, et al. The genomic diversification of the whole acinetobacter genus: origins, mechanisms, and consequences. Genome Biol Evol. (2014) 6:2866–82. doi: 10.1093/gbe/evu225
18. Tyumentseva M, Mikhaylova Y, Prelovskaya A, Tyumentsev A, Petrova L, Fomina V, et al. Genomic and phenotypic analysis of multidrug-resistant acinetobacter baumannii clinical isolates carrying different types of CRISPR/Cas systems. Pathogens. (2021) 10:205. doi: 10.3390/pathogens10020205
19. Zheng Y, Li J, Wang B, Han J, Hao Y, Wang S, et al. Endogenous type I CRISPR-cas: from foreign DNA defense to prokaryotic engineering. Front Bioeng Biotechnol. (2020) 8:62. doi: 10.3389/fbioe.2020.00062
20. Tucker AT, Nowicki EM, Boll JM, Knauf GA, Burdis NC, Trent MS, et al. Defining gene-phenotype relationships in acinetobacter baumannii through one-step chromosomal gene inactivation. mBio. (2014) 5:e01313–4. doi: 10.1128/mBio.01313-14
21. Maisuria VB, Okshevsky M, Déziel E, Tufenkji N. Proanthocyanidin interferes with intrinsic antibiotic resistance mechanisms of gram-negative bacteria. Adv Sci. (2019) 6:1802333. doi: 10.1002/advs.201802333
22. Kesavan D, Vasudevan A, Wu L, Chen J, Su Z, Wang S, et al. Integrative analysis of outer membrane vesicles proteomics and whole-cell transcriptome analysis of eravacycline induced acinetobacter baumannii strains. BMC Microbiol. (2020) 20:31. doi: 10.1186/s12866-020-1722-1
23. Pontes DS, de Araujo RSA, Dantas N, Scotti L, Scotti MT, de Moura RO, et al. Genetic mechanisms of antibiotic resistance and the role of antibiotic adjuvants. Curr Top Med Chem. (2018) 18:42–74. doi: 10.2174/1568026618666180206095224
24. Roy S, Naha S, Rao A, Basu S. CRISPR-Cas system, antibiotic resistance and virulence in bacteria: through a common lens. Prog Mol Biol Transl Sci. (2021) 178:123–74. doi: 10.1016/bs.pmbts.2020.12.005
25. Sanderson H, Ortega-Polo R, Zaheer R, Goji N, Amoako KK, Brown RS, et al. Comparative genomics of multidrug-resistant enterococcus spp. isolated from wastewater treatment plants. BMC Microbiol. (2020) 20:20. doi: 10.1186/s12866-019-1683-4
26. Vrancianu CO, Gheorghe I, Czobor IB, Chifiriuc MC. Antibiotic resistance profiles, molecular mechanisms and innovative treatment strategies of acinetobacter baumannii. Microorganisms. (2020) 8:935. doi: 10.3390/microorganisms8060935
27. Tang B, Gong T, Zhou X, Lu M, Zeng J, Peng X, et al. Deletion of cas3 gene in streptococcus mutans affects biofilm formation and increases fluoride sensitivity. Arch Oral Biol. (2019) 99:190–7. doi: 10.1016/j.archoralbio.2019.01.016
28. Dos Santos BA, de Oliveira JDS, Parmanhani-da-Silva BM, Ribeiro RL, Teixeira LM, Neves FPG. CRISPR elements and their association with antimicrobial resistance and virulence genes among vancomycin-resistant and vancomycin-susceptible enterococci recovered from human and food sources. Infect Genet Evol. (2020) 80:104183. doi: 10.1016/j.meegid.2020.104183
29. Wang G, Song G, Xu Y. Association of CRISPR/Cas system with the drug resistance in klebsiella pneumoniae. Infect Drug Resist. (2020) 13:1929–35. doi: 10.2147/IDR.S253380
30. Bikard D, Euler CW, Jiang W, Nussenzweig PM, Goldberg GW, Duportet X, et al. Exploiting CRISPR-Cas nucleases to produce sequence-specific antimicrobials. Nat Biotechnol. (2014) 32:1146–50. doi: 10.1038/nbt.3043
31. Rodrigues M, McBride SW, Hullahalli K, Palmer KL, Duerkop BA. Conjugative delivery of CRISPR-Cas9 for the selective depletion of antibiotic-resistant enterococci. Antimicrob Agents Chemother. (2019) 63:e01454–19. doi: 10.1128/AAC.01454-19
32. Tagliaferri TL, Guimarães NR, Pereira MPM, Vilela LFF, Horz HP, Dos Santos SG, et al. Exploring the potential of CRISPR-Cas9 under challenging conditions: facing high-copy plasmids and counteracting beta-lactam resistance in clinical strains of enterobacteriaceae. Front Microbiol. (2020) 11:578. doi: 10.3389/fmicb.2020.00578
Keywords: Acinetobacter baumannii, nosocomial pathogen, CRISPR-Cas system, antimicrobial resistance, csy1 gene
Citation: Guo T, Sun X, Li M, Wang Y, Jiao H and Li G (2022) The Involvement of the csy1 Gene in the Antimicrobial Resistance of Acinetobacter baumannii. Front. Med. 9:797104. doi: 10.3389/fmed.2022.797104
Received: 18 October 2021; Accepted: 03 January 2022;
Published: 26 January 2022.
Edited by:
Jessica L. Jones, United States Food and Drug Administration, United StatesReviewed by:
Prasanth Manohar, Zhejiang University-University of Edinburgh Institute, ChinaTingting Xu, Jinan University, China
Copyright © 2022 Guo, Sun, Li, Wang, Jiao and Li. This is an open-access article distributed under the terms of the Creative Commons Attribution License (CC BY). The use, distribution or reproduction in other forums is permitted, provided the original author(s) and the copyright owner(s) are credited and that the original publication in this journal is cited, in accordance with accepted academic practice. No use, distribution or reproduction is permitted which does not comply with these terms.
*Correspondence: Guocai Li, Z2NsaUB5enUuZWR1LmNu