- 1 Department of Biology, Utah State University, Logan, UT, USA
- 2 Program in Physical Biology, Eunice Kennedy Shriver National Institute of Child Health and Human Development, National Institutes of Health, Bethesda, MD, USA
The plant-associated bacterium Pseudomonas syringae pv. syringae simultaneously produces two classes of metabolites: the small cyclic lipodepsinonapeptides such as the syringomycins and the larger cyclic lipodepsipeptide syringopeptins SP22 or SP25. The syringomycins inhibit a broad spectrum of fungi (but particularly yeasts) by lipid-dependent membrane interaction. The syringopeptins are phytotoxic and inhibitory to Gram-positive bacteria. In this study, the fungicidal activities of two major syringopeptins, SP22A and SP25A, and their mechanisms of action were investigated and compared to those of syringomycin E. SP22A and SP25A were observed to inhibit the fungal yeasts Saccharomyces cerevisiae and Candida albicans although less effectively than syringomycin E. S. cerevisiae mutants defective in ergosterol and sphingolipid biosyntheses were less susceptible to SP22A and SP25A but the relative inhibitory capabilities of SRE vs. SP22A and SP25A were maintained. Similar differences were observed for capabilities to cause cellular K+ and Ca2+ fluxes in S. cerevisiae. Interestingly, in phospholipid bilayers the syringopeptins are found to induce larger macroscopic ionic conductances than syringomycin E but form single channels with similar properties. These findings suggest that the syringopeptins target the yeast plasma membrane, and, like syringomycin E, employ a lipid-dependent channel-forming mechanism of action. The differing degrees of growth inhibition by these lipodepsipeptides may be explained by differences in their hydrophobicities. The more hydrophobic SP22A and SP25A might interact more strongly with the yeast cell wall that would create a selective barrier for their incorporation into the plasma membrane.
Introduction
In addition to well-characterized small cyclic lipodepsinonapeptides, Pseudomonas syringae pv. syringae produces larger cyclic lipodepsipeptides known as the syringopeptins. These compounds are composed of 22 or 25 amino acids (SP22 and SP25, respectively) with an octadepsipeptide ring structure and a 3-hydroxy fatty acyl chain. Isoforms of SP22 and SP25 possessing fatty acyl chains composed of 10 and 12 carbons are designated A and B homologs, respectively. They or close structural variants are produced by other P. syringae pathovars (Vassilev et al., 1996; Grgurina et al., 2005). The syringopeptins are phytotoxic, and also have antimicrobial activities against Gram-positive bacteria (Lavermicocca et al., 1997; Grgurina et al., 2005; Bensaci and Takemoto, 2007). However, little is known about the antifungal properties of the syringopeptins although inhibitory activities against yeasts including the pathogen Candida albicans are briefly mentioned in reports (Iacobellis et al., 1992; Lavermicocca et al., 1997; Grgurina et al., 2005).
The small cyclic lipodepsinonapeptide syringomycin E (SRE) acts on yeast plasma membranes to cause increases in cellular K+ efflux and transient Ca2+ fluxes (Zhang and Takemoto, 1989; Takemoto et al., 1991). The effects are consistent with SRE’s ability to form ion-conducting voltage sensitive channels in planar lipid bilayers (Feigin et al., 1996; Kaulin et al., 1998; Malev et al., 2002). Studies with yeast lipid mutants revealed that sphingolipids and sterols (lipids that predominate in the plasma membrane) modulate SRE’s fungicidal activity (Cliften et al., 1996; Grilley et al., 1998; Stock et al., 2000). Lipid-modulated clustering of single channels each composed of six SRE molecules has been proposed as the structural basis for SRE’s mechanism of action on membranes (Kaulin et al., 1998, 2005; Malev et al., 2002).
Less is known about the mechanisms of action of the syringopeptins. A few studies have shown that the syringopeptins are capable of forming ion-conducting membrane channels (Hutchison and Gross, 1997; Dalla Serra et al., 1999; Agner et al., 2000). In lipid bilayers and erythrocytes, SP22A channels do not thermally inactivate as do SRE channels – a phenomenon related to SP22A’s greater effectiveness in ordering membrane lipids (Szabo et al., 2004). In plant systems, the syringopeptins have been shown to cause electrolyte leakage in leaf tissues (Iacobellis et al., 1992; Lavermicocca et al., 1997), to increase the permeability of tonoplasts (Carpaneto et al., 2002), close stomata (Di Giorgio et al., 1996a), and modify H+ fluxes across mitochondria and plasma membranes (Di Giorgio et al., 1994, 1996b). Finally, syringopeptins interact with bacterial cell surface teichoic acids (Bensaci and Takemoto, 2007), but whether and how they act on bacterial membranes is still unknown. Despite recognition that the syringopeptins are antifungal, there are no published studies devoted to the fungicidal mechanisms of action of syringopeptins.
In this study, we addressed the relationship between fungicidal activity and the mechanism of action of the syringopeptins. Despite structural differences between the syringopeptins and SRE, we show that the physiological responses and the membrane lipid requirements for growth inhibition are similar but the degrees of effectiveness differ in the order: SRE > SP22A > SP25A. To examine the basis for these differences, the channel-forming properties of the syringopeptins in lipid bilayers in comparison with those of SRE were investigated. It was found that in planar lipid bilayers SP22A and SP25A induce larger macroscopic conductances than SRE but form single channels of very similar properties.
Materials and Methods
Yeast Strains
Saccharomyces cerevisiae SRE-sensitive strains KZ1-1C, 8A-1B, W303C, and BY4741 and SRE-resistant and lipid biosynthesis mutant strains Δerg3, Δsyr2, Δipt1, Δelo2, Δelo3, and Δfah1 were described previously (Taguchi et al., 1994; Hama et al., 2000; Stock et al., 2000). SRE-resistant strains Δerg3 (formerly Δsyr1, Taguchi et al., 1994) and Δfah1 are single-gene disruptants and isogenic to parental strains 8A-1B and BY4741, respectively. All other SRE-resistant mutants are single-gene disruptants and isogenic to parental strain W303C. C. albicans ATCC10231 was obtained from the American Type Culture Collection (Manassas, VA, USA). All yeast strains were grown at 28°C and maintained (at 5°C) with yeast extract–peptone dextrose (YPD) broth or agar medium (Hama et al., 2000).
Purification of SRE, SP22A, and SP25A
SRE (Mr 1224) was purified from P. syringae pv. syringae strains B301D or M1 by previously described methods of Bidwai et al. (1987), (Adetuyi et al. 1995). SP22A (Mr 2142) and SP25A (Mr 2397) were purified from extracts of strains P. syringae pv. syringae B301D and M1 respectively, using methods described earlier (Bensaci and Takemoto, 2007).
Growth Inhibition
For measuring growth inhibition in liquid batch cultures, S. cerevisiae strain KZ1-1C was first grown in YPD broth medium at 28°C in 125 mL capacity Erlenmeyer flasks with rotary shaking for 48 h to a density of ~8 × 108 cells mL−1. The cells were centrifuged and the sedimented cells suspended in YPD broth medium to give 2.4 × 107 cells mL−1. SRE, SP22A, or SP25A were added at designated concentrations (between 0.8 and 9.3 μM). The suspensions were incubated with rotary shaking at 28°C and samples removed hourly for direct cell counts using a light microscope and hemocytometer. Minimal inhibitory concentrations (MICs) were determined by the microbroth dilution assay according to methods of the National Committee of Clinical Laboratory Standards (NCCLS, 2002). Yeast strains were grown to a final concentration of 108 colony-forming units (CFU) mL−1 and suspended at a final concentration of 5 × 105 CFU mL−1. Cell suspensions (25 μL) were added to 25 μL aliquots of twofold serial dilutions of SRE, SP22A, and SP25B. YPD broth media were dispensed (100 μL total volume) in wells of 96-well polystyrene microtiter plates. The plates were incubated for 24 h at 28°C. The MICs were determined by visual inspection of the plate. For determination of cell viability, a suspension of 4 × 105 CFU mL−1 was treated with different concentrations of SRE, SP22A, and SP25A and the cell suspension was twofold serially diluted. Aliquots (100 μL) were spread-plated onto YPD agar, the agar plates incubated for 24–48 h at 28°C, and the numbers of colony-forming units per milliliter of undiluted cell suspension determined. All growth inhibitory experiments were performed in triplicate and the results reported as mean values.
K+ Efflux
Whole cell K+ efflux rates were determined as changes in extracellular K+ concentrations. Yeast strain KZ1-1C was grown in YPD broth medium with rotary shaking at 28°C to a density of 108 cells mL−1. Cells were suspended in 2 mM Tris/MES buffer, pH 6.5, and 0.1 M-glucose with or without SRE, SP22A, or SP25A (at concentrations of 1, 10, or 100 μM) to A600 nm of 1 in 125 mL capacity Erlenmeyer flasks with rotary shaking (200 rpm) at 28°C (Takemoto et al., 1991). At 5 min after suspension, 1 mL samples were withdrawn, centrifuged in an Eppendorf microcentrifuge for 30 s, and the supernatant fractions were recovered. K+ concentrations were determined by atomic absorption spectroscopy (AA/AE spectrophotometer 457, Instrumentation Laboratories).
Ca2+ Uptake
The net cellular uptake of 45Ca2+ was measured using strain KZ1-1C as described previously (Takemoto et al., 1991). Cells were grown in YPD broth medium to a density of 1 × 107 cells mL−1, harvested by centrifugation, and then suspended in YPD broth medium to a density of 4 × 107 cells mL−1). Ten milliliter aliquots were dispensed in 125 mL capacity Erlenmeyer flasks with radioactive 45CaCl2 (Amersham Radiochemicals) and incubated with rotary shaking (200 rpm) at 28°C. The specific radioactivity of 45Ca2+ was adjusted to 4 mCi (148 MBq) per millimole of CaCl2 (Takemoto et al., 1991). SRE, SP22A, or SP25A were added 5 min following addition of 45CaCl2. At designated times, cell samples (200 μL) were collected on glass fiber filters (0.45 μM pore size), the filters were quickly washed twice with ice-cold water, and the radioactivity on the filters determined using a liquid scintillation counter.
Channel Formation in Planar Lipid Bilayers
Lipid bilayer electrophysiological experiments were performed using 1,2-dioleoyl-sn-glycero-3-phosphorylcholine (DOPC; Avanti Polar Lipids) as previously described (Blasko et al., 1998; Malev et al., 2002). Solutions of 0.1 M NaCl were buffered with 5 mM MOPS (Sigma) to pH 6. Bilayer lipid membranes were prepared by a monolayer-opposition technique (Montal and Mueller, 1972) on a 50- to 100-μm diameter aperture in the 15-μm thick Teflon film separating two (cis and trans) compartments of a Teflon chamber. SP22A, SP25A, and SRE were added to the aqueous phase of the cis-side compartment. A pair of Ag/AgCl electrodes with agarose/2 M KCl bridges was used to apply transmembrane voltages and to measure single-channel currents. All experiments were performed at room temperature (22 ± 2°C). Current measurements were carried out using an Axopatch 200B amplifier (Axon Instruments) in a voltage clamp mode. The data were filtered with a low-pass 8-pole Model 9002 Bessel filter (Frequency Devices) at 1 kHz and directly recorded into computer memory with a sampling frequency of 5 kHz. Data were analyzed using pClamp 9.2 (Axon Instruments) and Origin 7.0 (Origin Lab). Current transition histograms were generated for each tested voltage. Histogram peaks were fitted with the normal distribution function. Single-channel conductance was calculated as the mean single-channel current divided by the applied transmembrane voltage.
Results
Growth Inhibitory Activities against Yeasts
SRE and syringopeptins SP22A and SP25A inhibited the growth of different strains of S. cerevisiae and C. albicans ATCC 10231 (Table 1). In all cases, SP22A was ~2-fold more active than SP25A and both were less inhibitory than SRE. The same relative inhibitory capabilities (i.e., SRE > SP22A > SP25A) were observed when measuring effects on cell numbers in batch growth cultures (Table 2) and on cell viability (Figure 1). In microbroth dilution inhibition assays, growth did not resume at MIC levels of SRE, SP22A, or SP22B after 48 h incubation indicating that these compounds were fungicidal (data not shown).
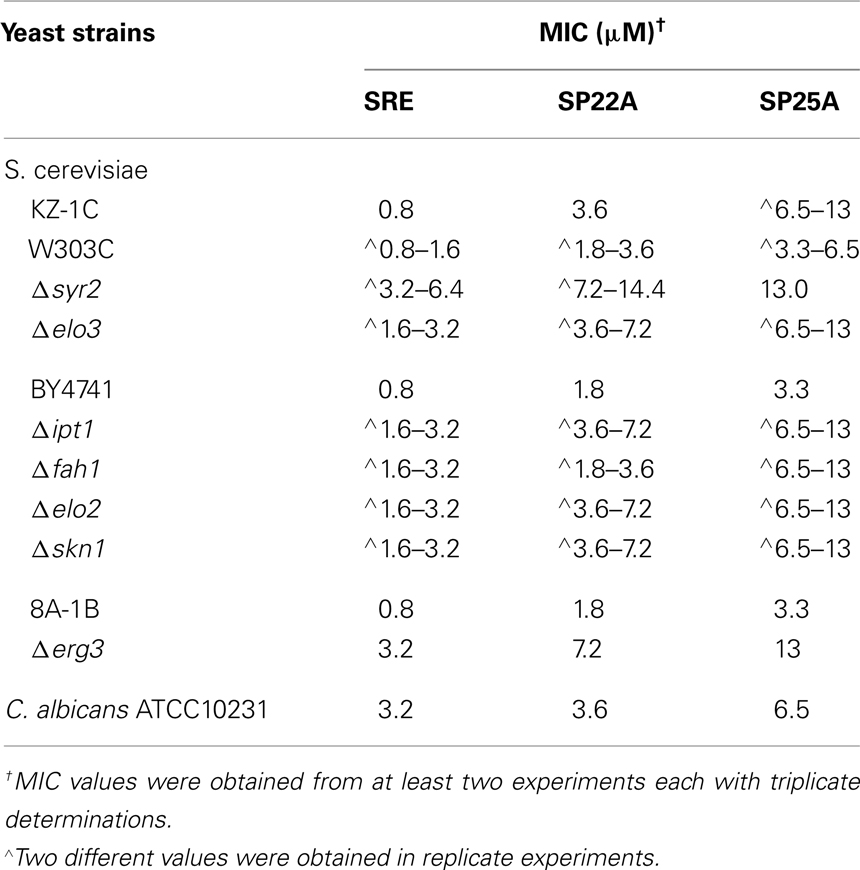
Table 1. Minimal inhibitory concentrations (MICs) of SRE, SP22A, and SP25A against S. cerevisiae strains, lipid biosynthetic mutants, and C. albicans ATCC10231.
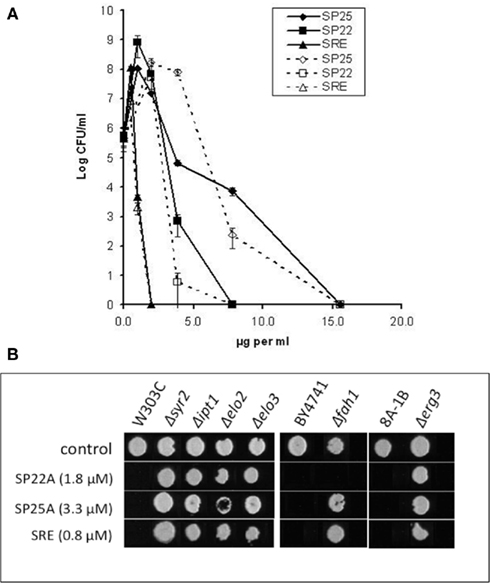
Figure 1. Inhibition of yeasts and lipid mutants by syringopeptins and SRE. (A) Effects of SP25A (♦), SP22A (■), and SRE (▲) at the designated concentrations on the viability of C. albicans (solid line) and S. cerevisiae strain KZ-1C (dashed lines). Error bars indicate SDs from average values from three separate experiments. (B) Effects on sphingolipid and ergosterol biosynthesis mutants and isogenic parental strains replica plated onto YPD agar with or without: SP22A (1.8 μM), SP25A (3.3 μM) or SRE (0.8 μM). The plates were incubated at 28°C for 48 h.
Susceptibility of Lipid Biosynthetic Mutants
Previous studies showed that certain S. cerevisiae mutants with defects in sphingolipid or sterol biosynthesis are more resistant to SRE than isogenic wild type strains (Grilley et al., 1998; Hama et al., 2000; Stock et al., 2000). In the present study, mutant strains with genes deleted for dihydrosphingosine C-4 hydroxylase (Δsyr2), mannosyl-diinositolphosphoryl-ceramide synthase (Δipt1), sphingolipid very long chain elongases (Δelo2 and Δelo3), and sterol C5,6 desaturase (Δerg3) were each less susceptible to inhibition by SP22A and SP25A at MICs for their respective isogenic wild type parental strains (Table 1; Figure 1B). Mutant strain Δfah1 defective in sphingolipid fatty acid α-hydroxylase was more susceptible to SP22A than to SRE or SP25A (Figure 1B) but nevertheless more resistant to SP22A concentrations below 2 μM that were inhibitory to isogenic wild type strain BY4741 (Table 1). The inhibitory patterns suggest influences of target membrane lipids on the fungicidal action of SP22A and SP25A similar to those for SRE (Grilley et al., 1998; Hama et al., 2000; Stock et al., 2000; Kaulin et al., 2005). Although needed at higher concentrations with the mutants, the same relative inhibitory capabilities of SRE > SP22A > SP25A were observed regardless of the lipid biosynthesis defect.
Effects on Cellular K+ and Ca2+ Fluxes
As previously observed for SRE (Takemoto et al., 1991), SP22A, and SP25A caused net K+ effluxes and Ca2+ influxes in whole cells of S. cerevisiae strain KZ1-1C (Figure 2). Higher concentrations of SP22A and SP25A were required to give the effects as compared to SRE. SRE stimulated K+ efflux at concentrations starting from 1 μM; while 10 and 100 μM of SP22A and SP25A, respectively, were required to achieve similar degrees of K+ efflux. Cellular Ca2+ influx was observed within 15 min after 5 μM SRE addition (data not shown). But, 40 and 120 μM of SP22A and SP25A, respectively, were required for detection of Ca2+ influx. At these concentrations, Ca2+ influx was not evident until about 20 and 30 min after addition of SP22A and SP25A, respectively.
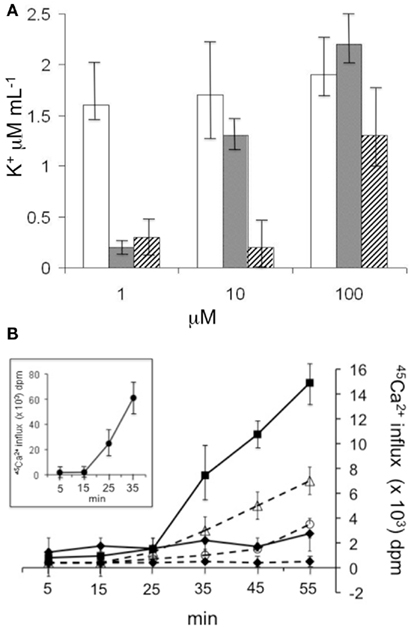
Figure 2. Influence of syringopeptins on ion fluxes of yeast cells. (A) Effects of SRE (blank bars), SP22A (gray bars), and SP25A (striped bars) on K+ efflux by yeast S. cerevisiae strain KZ1-1C cells growing in YPD medium. The cells were exposed to designated concentrations of SRE, SP22A, or SP25A and extracellular K+ concentrations measured. Control efflux (no treatment) was similar to efflux with SP22A treatment at 1 μM. Error bars show relative SEs from three separate experiments. (B) Effects on 45Ca2+ uptake by S. cerevisiae strain KZ1-1C cell suspensions. SRE, SP22A, and SP25A were added 5 min following addition of 45CaCl2. SP22A (solid lines) concentrations were 10 μM (♦) and 40 μM (■); SP25A (dashed lines) concentrations were 10 μM (♦), 40 μM (○), and 120 μM (∆). Total CaCl2 concentration was 50 μM. SRE concentration was 5 μM (inset). Error bars indicate SDs of average values from three separate experiments.
Channel Formation in Planar Lipid Bilayers
SRE forms well-characterized voltage-dependent ion-conducting channels in lipid bilayers (Feigin et al., 1996; Kaulin et al., 1998; Malev et al., 2002). To examine whether the differences in the relative fungicidal capabilities of SRE, SP22A, and SP25A may lie in their different channel-forming properties, the conductances of planar lipid bilayers doped with the three compounds were compared (Figure 3). In all three cases, application of potentials negative from the side of lipodepsipeptide addition induced increases in macroscopic conductance. After a 5-min equilibration, the conductance reached its stationary value, which within the deviations expected for stochastically operating discrete pores, was stable for at least 10 min (Figure 3A). Application of positive voltages produced membrane conductance decreases (not shown), indicating closing of the pores. SRE produced smaller steady-state conductances at concentrations that were significantly higher (~150-fold) than those produced by the syringopeptins. SP22A induced slightly higher conductances than SP25A.
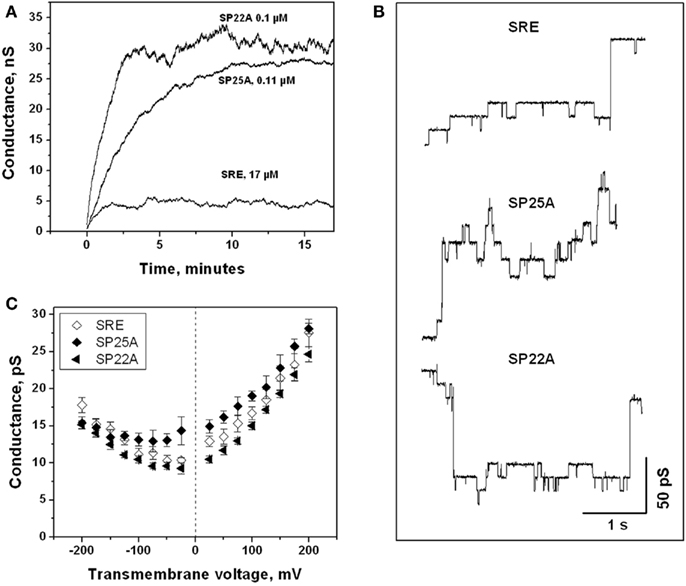
Figure 3. (A) Time courses of the macroscopic ion conductance of bilayers doped with SRE, SP22A, and SP25A, recorded at the applied voltage of −100 mV. Ten individual observations were made for each compound and concentration with one representative trace shown here. (B) Records of conductance fluctuations at −150 mV transmembrane voltage of SRE, SP22A, and SP25A-modified bilayers. (C) Conductance–voltage curves for SRE, SP22A, and SP25A small channels.
Single-channel recordings with all three lipodepsipeptides demonstrated a similar pattern (Figure 3B). In all cases, two types of single-channel conductance fluctuations were observed, small and large, differing in the levels of conductance five to sixfold as was described earlier for SRE (Malev et al., 2002; Kaulin et al., 2005). The dwell times of the large channels were longer than those of the small ones. The values of the single-channel conductance for the small channels over the range of ±200 mV were close for all three lipodepsipeptides (Figure 3C). Thus, the properties of the single transmembrane channels, formed by SRE, SP22A, and SP25A were essentially the same.
Discussion
SRE, SP22A, and SP25A caused cellular K+ and Ca2+ fluxes at relative concentrations that paralleled their relative growth inhibitory activities against yeasts. The concentrations of these compounds needed to elicit the ion fluxes were higher than the corresponding MIC values suggesting that additional events are contributing to growth inhibition. It was also observed that SP22A and SP25A induced voltage-dependent conductance in planar lipid bilayers and that their yeast inhibitory capabilities were influenced by sphingolipid and sterol biosynthesis – phenomena characteristic of SRE effects (Feigin et al., 1996; Grilley et al., 1998; Hama et al., 2000; Stock et al., 2000). Therefore, based on what is known of SRE’s mechanism of action (Malev et al., 2002; Takemoto et al., 2003; Kaulin et al., 2005), it is suggested that the syringopeptins form stable membrane pores comprised of and modulated by host membrane lipids and particularly sphingolipids and sterols.
The membrane channel properties of SP22A and SP25A were examined with planar lipid bilayers to explore the reasons for their lower yeast growth inhibitory activities as compared to SRE. Both syringopeptins turned out to be ~150 times more effective than SRE in inducing macroscopic conductance in DOPC bilayers. At the same time, the properties of the single channels formed by all three lipodepsipeptides were very similar (Figure 3) pointing to their similar structural organization (Ostroumova et al., 2007). It is suggested that the macroscopic conductance differences stem from the structural differences of SRE vs. the syringopeptins. SRE lacks a hydrophobic peptide domain, whereas SP22A and SP25A have hydrophobic peptide domains of 14 and 17 amino acids, respectively (Ballio et al., 1991). As a consequence, the syringopeptins are expected to interact more favorably with lipids than SRE. However, due to SP22A and SP25A increased hydrophobicities, their interaction with the yeast cell wall (Chaffin et al., 1998; Klis et al., 2006) might also be significantly increased thus boosting its filtering function. This additional barrier on their route to the plasma membrane may account for the discrepancy between their activities in planar lipid bilayers vs. growing cultures.
It is not known precisely how the syringopeptins and SRE interact with membrane lipids to form channels (Malev et al., 2002). However, the electrostatic and hydrophobic interactions between SP22A and membrane lipids are known to increase lipid ordering as a prelude to stable pore formation (Szabo et al., 2004). In addition, unlike SRE, the SP22A pores are not thermally inactivated suggesting a higher degree of affinity between SP22A and membrane lipids as compared to SRE (Szabo et al., 2004). It is therefore reasonable to assume that the lipid compositions of the yeast plasma membranes will influence stabilization of syringopeptin channel clusters differently from SRE channel clusters. This may constitute an additional component of the mechanistic basis for the different degrees of fungicidal activities of these lipodepsipeptides.
The present results emphasize the importance of the size and character of the hydrophobic moieties of these cyclic lipodepsipeptides in target cell specificity. SRE is mainly antifungal and the syringopeptins are antibacterial and phytotoxic as well (Iacobellis et al., 1992; Lavermicocca et al., 1997; Grgurina et al., 2005). It appears that the higher degree of hydrophobicity of the syringopeptins imparts a broader range of target cell specificity for plant-associated pseudomonads that produce these compounds. These bacteria are mainly plant epiphytes but also opportunistic plant pathogens, and their cyclic lipodepsipeptide profiles would influence the complex balance between pathogenicity and saprophytic existence.
In summary, the present studies show that like SRE the syringopeptins inhibit the growth of fungal yeasts and with similar physiological responses. Although they are less potent fungicides than SRE, the syringopeptins form lipid-modulated single channels in planar lipid bilayer membranes that are similar to those of SRE.
Conflict of Interest Statement
The authors declare that the research was conducted in the absence of any commercial or financial relationships that could be construed as a potential conflict of interest.
Acknowledgments
This research was supported by the National Science Foundation (9003393), the Utah Agricultural Experiment Station (UTA 569; approved as journal paper no. 7972), the Intramural Research Program of the NIH, Eunice Kennedy Shriver National Institute of Child Health and Human Development, and an N. R. Gandhi Graduate Research Fellowship to Mekki F. Bensaci. We thank Dr. Joanne E. Hughes (Utah State University) for conducting 45Ca2+ flux measurements.
References
Adetuyi, F. C., Isogai, A., Di Giorgio, D., Ballio, A., and Takemoto, J. Y. (1995). Saprophytic Pseudomonas syringae strain M1 of wheat produces cyclic lipodepsipeptides. FEMS Microbiol. Lett. 131, 63–67.
Agner, G., Kaulin, Y. A., Schagina, L. V., Takemoto, J. Y., and Blasko, K. (2000). Effect of temperature on the formation and inactivation of syringomycin E pores in human red blood cells and bimolecular lipid membranes. Biochim. Biophys. Acta 1466, 79–86.
Ballio, A., Barra, D., Bossa, F., Collina, A., Grgurina, I., Marino, G., Moneti, G., Paci, M., Pucci, P., Segre, A., and Simmaco, M. (1991). Syringopeptins, new phytotoxic lipodepsipeptides of Pseudomonas syringae pv. syringae. FEBS Lett. 291, 109–112.
Bensaci, M. F., and Takemoto, J. Y. (2007). Syringopeptin SP25A-mediated killing of gram-positive bacteria and the role of teichoic acid d-alanylation. FEMS Microbiol. Lett. 268, 106–111.
Bidwai, A. P., Zhang, L., Bachmann, R. C., and Takemoto, J. Y. (1987). Mechanism of action of Pseudomonas syringae phytotoxin, syringomycin: stimulation of red beet plasma membrane ATPase activity. Plant Physiol. 83, 39–43.
Blasko, K., Schagina, L. V., Agner, G., Kaulin, Y. A., and Takemoto, J. Y. (1998). Membrane sterol composition modulates the pore forming activity of syringomycin E in human red blood cells. Biochim. Biophys. Acta 1373, 163–169.
Carpaneto, A., Dalla Serra, M., Menestrina, G., Fogliano, V., and Gambale, F. (2002). The phytotoxic lipodepsipeptide syringopeptin 25A from Pseudomonas syringae pv. syringae forms ion channels in sugar beet vacuoles. J. Membr. Biol. 188, 237–248.
Chaffin, W. L., López-Ribot, J. L., Casanova, M., Gozalbo, D., and Martínez, J. P. (1998). Cell wall and secreted proteins of Candida albicans: identification, function, and expression. Microbiol. Mol. Biol. Rev. 62, 130–180.
Cliften, P., Wang, Y., Mochizuki, D., Miyakawa, T., Wangspa, R., Hughes, J., and Takemoto, J. Y. (1996). SYR2, a gene necessary for syringomycin growth inhibition of Saccharomyces cerevisiae. Microbiology 142, 477–484.
Dalla Serra, M., Bernhart, I., Nordera, P., Di Giorgio, D., Ballio, A., and Menestrina, G. (1999). Conductive properties and gating of channels formed by syringopeptin 25A, a bioactive lipodepsipeptide from Pseudomonas syringae pv. syringae, in planar lipid membranes. Mol. Plant Microbe. Interact. 12, 401–409.
Di Giorgio, D., Camoni, L., and Ballio, A. (1994). Toxins of Pseudomonas syringae pv. syringae affect H+-transport across the plasma membrane of maize. Physiol. Plant. 91, 741–746.
Di Giorgio, D., Camoni, L., Mott, K. A., Takemoto, J. Y., and Ballio, A. (1996a). Syringopeptins, Pseudomonas syringae pv. syringae phytotoxins, resemble syringomycin in closing stomata. Plant Pathol. 45, 564–571.
Di Giorgio, D., Lavermicocca, P., Marchiafava, C., Camoni, L., Surico, G., and Ballio, A. (1996b). Effect of syringomycin-E and syringopeptins on isolated plant mitochondria. Physiol. Mol. Plant Pathol. 48, 325–334.
Feigin, A. M., Takemoto, J. Y., Wangspa, R., Teeter, J. H., and Brand, J. G. (1996). Properties of voltage-gated ion channels formed by syringomycin E in planar lipid bilayers. J. Membr. Biol. 149, 41–47.
Grgurina, I., Bensaci, M., Pocsfalvi, G., Mannina, L., Cruciani, O., Fiore, A., Fogliano, V., Sorensen, K. N., and Takemoto, J. Y. (2005). Novel cyclic lipodepsipeptide from Pseudomonas syringae pv. lachrymans strain 508 and syringopeptin antimicrobial activities. Antimicrob. Agents Chemother. (Bethesda) 49, 5037–5045.
Grilley, M., Stock, S. D., Dickson, R. C., Lester, R. L., and Takemoto, J. Y. (1998). Syringomycin action gene SYR2 is essential for sphingolipid 4-hydroxylation in Saccharomyces cerevisiae. J. Biol. Chem. 273, 11062–11068.
Hama, H., Young, D. A., Radding, J. A., Ma, D., Tang, J., Stock, S. D., and Takemoto, J. Y. (2000). Requirement of sphingolipid alpha-hydroxylation for fungicidal action of syringomycin E. FEBS Lett. 478, 26–28.
Hutchison, M. L., and Gross, D. C. (1997). Lipopeptide phytotoxins produced by Pseudomonas syringae pv. syringae: comparison of the biosurfactant and ion channel-forming activities of syringopeptin and syringomycin. Mol. Plant Microbe Interact. 10, 347–354.
Iacobellis, N. S., Lavermicocca, P., Grgurina, I., Simmaco, M., and Ballio, A. (1992). Phytotoxic properties of Pseudomonas syringae pv. syringae toxins. Physiol. Mol. Plant Pathol. 40, 107–116.
Kaulin, Y. A., Schagina, L. V., Bezrukov, S. M., Malev, V. V., Feigin, A. M., Takemoto, J. Y., Teeter, J. H., and Brand, J. G. (1998). Cluster organization of ion channels formed by the antibiotic syringomycin E in bilayer lipid membranes. Biophys. J. 74, 2918–2925.
Kaulin, Y. A., Takemoto, J. Y., Schagina, L. V., Ostroumova, O. S., Wangspa, R., Teeter, J. H., and Brand, J. G. (2005). Sphingolipids influence the sensitivity of lipid bilayers to fungicide, syringomycin E. J. Bioenerg. Biomembr. 37, 339–348.
Klis, F. M., Boorsma, A., and De Groot, P. W. (2006). Cell wall construction in Saccharomyces cerevisiae. Yeast 23, 185–202.
Lavermicocca, P., Iacobellis, N., Simmaco, M., and Graniti, A. (1997). Biological properties and spectrum of activity of Pseudomonas syringae pv. syringae toxins. Physiol. Mol. Plant Pathol. 50, 129–140.
Malev, V. V., Schagina, L. V., Gurnev, P. A., Takemoto, J. Y., Nestorovich, E. M., and Bezrukov, S. M. (2002). Syringomycin E channel: a lipidic pore stabilized by lipopeptide? Biophys. J. 82, 1985–1994.
Montal, M., and Mueller, P. (1972). Formation of bimolecular membranes from lipid monolayers and a study of their electrical properties. Proc. Nat. Acad. Sci. U.S.A. 69, 3561–3566.
NCCLS. (2002). Reference Method for Broth Dilution Antifungal Susceptibility Testing of Yeast: Approved Standard, 2nd Edn. Wayne PA: National Committee for Clinical Laboratory Standards.
Ostroumova, O. S., Gurnev, P. A., Schagina, L. V., and Bezrukov, S. M. (2007). Asymmetry of syringomycin E channel studied by polymer partitioning. FEBS Lett. 581, 804–808.
Stock, S. D., Hama, H., Radding, J. A., Young, D. A., and Takemoto, J. Y. (2000). Syringomycin E inhibition of Saccharomyces cerevisiae: requirement for biosynthesis of sphingolipids with very-long-chain fatty acids and mannose- and phosphoinositol-containing head groups. Antimicrob. Agents Chemother. (Bethesda) 44, 1174–1180.
Szabo, Z., Budai, M., Blasko, K., and Grof, P. (2004). Molecular dynamics of the cyclic lipodepsipeptides’ action on model membranes: effects of syringopeptin22A, syringomycin E, and syringotoxin studied by EPR technique. Biochim. Biophys. Acta 1660, 118–130.
Taguchi, N., Takano, Y., Julmanop, C., Wang, Y., Stock, S., Takemoto, J., and Miyakawa, T. (1994). Identification and analysis of the Saccharomyces cerevisiae SYR1 gene reveals that ergosterol is involved in the action of syringomycin. Microbiology 140 (Pt 2), 353–359.
Takemoto, J. Y., Brand, J. G., Kaulin, Y. A., Malev, V. V., Schagina, L. V., and Blasko, K. (2003). “The syringomycins: lipodepsipeptide pore formers from plant bacterium. Pseudomonas syringae,” in Pore Forming Peptides and Protein Toxins, eds G. Menestrina, M. Dalla Serra, and P. Lazarovici (London: Taylor and Francis), 260–271.
Takemoto, J. Y., Zhang, L., Taguchi, N., Tachikawa, T., and Miyakawa, T. (1991). Mechanism of action of the phytotoxin, syringomycin: a resistant mutant of Saccharomyces cerevisiae reveals an involvement of Ca2+ transport. J. Gen. Microbiol. 137, 653–659.
Vassilev, V., Lavermicocca, P., Di Giorgio, D., and Iacobellis, N. S. (1996). Production of syringomycins and syringopeptins by Pseudomonas syringae pv. atrofaciens. Plant Pathol. 45, 316–322.
Keywords: syringopeptin, fungicide, syringomycin, yeast, Pseudomonas syringae, ion channel
Citation: Bensaci MF, Gurnev PA, Bezrukov SM and Takemoto JY (2011) Fungicidal activities and mechanisms of action of Pseudomonas syringae pv. syringae lipodepsipeptide syringopeptins 22A and 25A. Front. Microbio. 2:216. doi: 10.3389/fmicb.2011.00216
Received: 18 August 2011;
Accepted: 11 October 2011;
Published online: 25 October 2011.
Edited by:
Ana Traven, Monash University, AustraliaReviewed by:
Anand K. Ramasubramanian, University of Texas at San Antonio, USADennis Gross, Texas A&M University, USA
Copyright: © 2011 Bensaci, Gurnev, Bezrukov and Takemoto. This is an open-access article subject to a non-exclusive license between the authors and Frontiers Media SA, which permits use, distribution and reproduction in other forums, provided the original authors and source are credited and other Frontiers conditions are complied with.
*Correspondence: Jon Y. Takemoto, Department of Biology, Utah State University, 5305 Old Main Hill, Logan, UT 84322-5305, USA. e-mail: jon@biology.usu.edu
†Current address: Mekki F. Bensaci, Division of Geographic Medicine and Infectious Diseases, Tufts Medical Center, Boston, MA, USA.