- 1Red de Manejo Biorracional de Plagas y Vectores, Instituto de Ecología, AC–INECOL, Clúster Científico y Tecnológico BioMimic®, Xalapa, Mexico
- 2Fundación para el Fomento de la Investigación Sanitaria y Biomédica de la Comunitat Valenciana (FISABIO), Valencia, Spain
- 3Red de Biodiversidad y Sistemática, Instituto de Ecología, AC–INECOL, Clúster Científico y Tecnológico BioMimic®, Xalapa, Mexico
- 4Red de Estudios Moleculares Avanzados, Instituto de Ecología, AC–INECOL, Clúster Científico y Tecnológico BioMimic®, Xalapa, Mexico
- 5Instituto de Biología Integrativa de Sistemas (I2Sysbio), Universidad de Valencia-CSIC, Valencia, Spain
We studied the microbiota of a highly polyphagous insect, Anastrepha ludens (Diptera: Tephritidae), developing in six of its hosts, including two ancestral (Casimiroa edulis and C. greggii), three exotic (Mangifera indica cv. Ataulfo, Prunus persica cv. Criollo, and Citrus x aurantium) and one occasional host (Capsicum pubescens cv. Manzano), that is only used when extreme drought conditions limit fruiting by the common hosts. One of the exotic hosts (“criollo” peach) is rife with polyphenols and the occasional host with capsaicinoids exerting high fitness costs on the larvae. We pursued the following questions: (1) How is the microbial composition of the larval food related to the composition of the larval and adult microbiota, and what does this tell us about transience and stability of this species’ gut microbiota? (2) How does metamorphosis affect the adult microbiota? We surveyed the microbiota of the pulp of each host fruit, as well as the gut microbiota of larvae and adult flies and found that the gut of A. ludens larvae lacks a stable microbiota, since it was invariably associated with the composition of the pulp microbiota of the host plant species studied and was also different from the microbiota of adult flies indicating that metamorphosis filters out much of the microbiota present in larvae. The microbiota of adult males and females was similar between them, independent of host plant and was dominated by bacteria within the Enterobacteriaceae. We found that in the case of the “toxic” occasional host C. pubescens the microbiota is enriched in potentially deleterious genera that were much less abundant in the other hosts. In contrast, the pulp of the ancestral host C. edulis is enriched in several bacterial groups that can be beneficial for larval development. We also report for the first time the presence of bacteria within the Arcobacteraceae family in the gut microbiota of A. ludens stemming from C. edulis. Based on our findings, we conclude that changes in the food-associated microbiota dictate major changes in the larval microbiota, suggesting that most larval gut microbiota is originated from the food.
Introduction
The critical role the microbiome plays in supporting or outright regulating key metabolic pathways in organisms including humans or in dealing with emerging environmental challenges is being unraveled at an unprecedented speed (Gevers et al., 2012; Gilbert et al., 2014; Bauer et al., 2020; Ayyasamy et al., 2021; Schmidt and Engel, 2021). Although the role that microorganisms play in the life cycle of many organisms became already clear over 100 years ago (Petri, 1909) the interdependency and sophistication of the interactions, as well as their evolution and impact on overall host health and fitness, is being comprehensively investigated by the scientific community worldwide (Sommer and Bäckhed, 2013; Rowland et al., 2018; Jing et al., 2020; Ke et al., 2020). In the case of insects, it has been demonstrated that the gut microbiota plays multiple roles in nutrient uptake (e.g., the synthesis and absorption of nutrients), detoxification processes (Kikuchi, 2009; Ben-Yosef et al., 2015; Ceja-Navarro et al., 2015; Cheng et al., 2017), sexual fitness, health maintenance or longevity, and overall host physiology (Brummel et al., 2004; Dillon and Dillon, 2004; Engel and Moran, 2013; Pernice et al., 2014; Damodaram et al., 2016; Sudakaran et al., 2017; Jose et al., 2019; Raza et al., 2020). As insects are the most diverse group of animals on Earth, with over one million species (Grimaldi and Engel, 2005), they are considered as an ideal group to study the different degrees of association between the gut microbiota and their hosts (Hammer et al., 2019; Muñoz-Benavent et al., 2020), even if the complexity of the insect gut microbiota is far less complex than that of mammals (Sender et al., 2016).
As is the case in many other animals, the gut microbiota of insects can be classified as resident or transient. Some of these microorganisms were discovered during the beginning of the last century (Glasgow, 1914; Stammer, 1929) and may switch from an intra to an extracellular existence during host development (Estes et al., 2009). The resident microbiota is the microbial community that establishes long-lasting interactions with the host (Derrien and van Hylckama Vlieg, 2015; Hammer et al., 2017, 2019; Ma and Leulier, 2018) and can be of two types: (1) Obligatory endosymbionts embedded inside cells, that have taken over specific metabolic functions and are heritable and transmitted vertically (Akman et al., 2002; Baumann et al., 2002; Baumann, 2005) or that have become unwelcome parasites, such as is sometimes the case with Wolbachia, that can harm the host by, for example, controlling sex ratios (Werren et al., 2008; Fukui et al., 2015; Jiggins, 2016). (2) A large and diverse suite of microorganisms, particularly bacteria, yeasts, and viruses, that are found in the gut of both adults and larvae, and that are transmitted vertically or horizontally [i.e., are taken up from the environment and thus greatly vary in species composition according to environment, diet, age, physiological state, and health status (Engel and Moran, 2013; Johnston and Rolff, 2015; Pérez-Cobas et al., 2015; Muñoz-Benavent et al., 2020)]. In contrast to resident microbiota, the transient microbiota is exclusively acquired from the environment and usually passes through the gut and is expelled via the feces. It is not capable of establishing itself permanently, since its rate of loss exceeds its rate of permanence (Erkosar and Leulier, 2014; Derrien and van Hylckama Vlieg, 2015; Ma and Leulier, 2018; Hammer et al., 2019).
In general, those species with a stable gut microbiota closely rely on the functions provided by it. In contrast, the alteration of the gut microbiota has little influence on survival and development in insects with an unstable gut microbiota (Hammer et al., 2019). A direct relationship has been suggested between the metabolic self-sufficiency of the host and the absence of a stable gut microbiota (Hammer and Bowers, 2015; Hammer et al., 2017), supporting the idea that a stable gut microbiota is not equally important for all insect species (Hammer et al., 2019; Phalnikar et al., 2019). For example, the lack of a stable microbiota in certain caterpillars is related to the fact that caterpillars possess host-encoded mechanisms for degrading or tolerating plant allelochemicals (Després et al., 2007). In addition, it has been proposed that the lack of a stable gut microbiota could constitute an adaptive strategy, through which certain species can avoid establishing permanent interactions with microorganisms (Hammer et al., 2019).
Alternatively, other studies support the critical role gut microbiota plays on the physiology, adaptation, or ecological interactions of insects (Janson et al., 2008; Kageyama et al., 2012; Killiny et al., 2017; Gupta and Nair, 2020; Lemoine et al., 2020). For example, there is evidence that during plant-insect interactions, the gut microbiota can play a key role degrading plant structural compounds and supplementing missing nutrients, but also detoxifying deleterious compounds of chemically defended plants that they attack, by providing counter-defenses to plant toxins (Dowd, 1989; Douglas, 2009; Hansen and Moran, 2014; Hammer and Bowers, 2015). Based on this, Hammer and Bowers outlined the gut microbial facilitation hypothesis, which proposes that “variation among herbivores in their ability to consume chemically defended plants can be due, in part, to variation in their associated microbial communities” (Hammer and Bowers, 2015). One direct consequence of this hypothesis is that the gut microbiota may contribute to rapidly expanding the range of plants that insects can consume and assimilate.
Excluding termites, most recent work on insect microbiota has concentrated on cockroaches (Ayayee et al., 2018; Kakumanu et al., 2018; Guzman and Vilcinskas, 2020), beetles (Tsuchida et al., 2010; Zepeda-Paulo et al., 2010; Guyomar et al., 2018; McLean et al., 2019), and Drosophila (Drosophilidae) (Wong et al., 2013; Douglas, 2018; Ma and Leulier, 2018; Pais et al., 2018; Selkrig et al., 2018; Ludington and Ja, 2020). In the case of true fruit flies (Tephritidae), there are some classical studies on the interaction of them with microorganisms, but most of this research was focused on a few species within the genera Anastrepha, Bactrocera, Ceratitis, and Rhagoletis. For example, Stammer (1929) compared 37 species within the Tephritidae (formerly Trypetidae) in 24 genera, finding in almost all cases close associations between the flies and bacteria. Allen et al. (1934) described consistent associations between Phytomonas (Pseudomonas) melophthora and the apple maggot, Rhagoletis pomonella (Walsh), discussing the possibility that the female was contaminating the fruit with the bacteria while ovipositing, a broad topic retaken by Mazzini and Vita (1981), Stoffolano and Yin (1987), Raghu et al. (2002) and Behar et al. (2008). By far the best studied genus is Bactrocera in which, bacteria (Fitt and O’Brien, 1985; Capuzzo et al., 2005; Estes et al., 2009, 2014; Ben-Yosef et al., 2015; Yong et al., 2017; Heys et al., 2018; Liu et al., 2018; Akami et al., 2019), yeasts (Deutscher et al., 2017; Piper et al., 2017), fungi (Malacrinò et al., 2017; Yao et al., 2019), and viruses (Zhang et al., 2020), have been studied.
The case of B. oleae and its symbiotic interaction with bacteria represents an instructive example on the importance of the gut microbiota in allowing the host use of chemically defended plants (i.e., Olea europaea L.). In this fruit fly (formerly Dacus oleae), Petri (1904, 1909) was the first to describe a guild of larval gut bacteria, highlighting Bacterium (Pseudomonas) savastanoi and Ascobacterium luteum. He emphasized the relevance of B. savastanoi in possibly providing critical nutrients to the fly, also hinting at the bacterial breakdown of deleterious substances for the larvae, as well as dwelling on its importance in the immune system, by keeping A. luteum at bay and defending against infections produced by fungi and bacteria. Petri (1909) observed that if B. savastanoi was not present in the tract of B. oleae feeding in fruit of O. europaea, development was halted and individuals died, concluding that there was an obligatory symbiosis between the bacteria and the host, and that the bacteria were vertically transmitted from the female to the larvae via the “contaminated” eggs. Then, Hagen (1966) performed a series of experiments with streptomycin to demonstrate that B. oleae larvae need the bacteria to detoxify deleterious chemicals contained in unripe olives. He inferred that B. savastanoi “may be involved in the synthesis of methionine and threonine,” a topic also studied by Miyazaki et al. (1968) working with Pseudomonas melophthora and R. pomonella. More recently, Yuval et al. highlighted the central role the fruit plays with respect to fruit fly-bacteria interactions in which the latter aid in nitrogen fixation (Behar et al., 2008) and unraveled the specific role of Candidatus Erwinia dacicola [formerly referred to as B. savastanoi (Capuzzo et al., 2005)] in dealing with the toxic allelochemical oleuropein (a phenolic glycoside) present in unripe olives on which larvae feed (Ben-Yosef et al., 2015; Sinno et al., 2020), confirming the visionary suggestions previously made by Petri (1910) and Hagen (1966).
Based on all the above, but pointedly also motivated by the outstanding questions posited by Frago et al. (2012), Hansen and Moran (2014), Hammer and Bowers (2015), Hammer et al. (2017), Sudakaran et al. (2017), and Majumder et al. (2019), here we aim at providing a more in-depth ecological context to insect gut microbiota studies by comparing the microbiota in the gut of larvae reared in six hosts in nature of the highly pestiferous and polyphagous tephritid fly, Anastrepha ludens (Loew), also known as the Mexican Fruit Fly. We selected the two purportedly ancestral native hosts of A. ludens [Casimiroa greggii (S. Watson) F. Chiang and Casimiroa edulis La Llave (both Rutaceae)], an additional native one [Capsicum pubescens cv. Manzano (Solanaceae)], as well as three exotic ones [Citrus x aurantium (Rutaceae), Mangifera indica cv. Ataulfo (Anacardiaceae), and Prunus persica cv. Criollo (Rosaceae)], ranging from commonly used fruit (e.g., C. x aurantium) to rarely used hosts (e.g., C. pubescens) so as to render our comparison more robust and ecologically meaningful (Aluja and Mangan, 2008). Birke and Aluja (2018) working with A. ludens and almost the same hosts we studied here, report that pupal weights (mg) of larvae developing in these fruits were: C. edulis 23.02 ± 0.19, M. indica 21.25 ± 0.16, C. aurantium 18.23 ± 0.15, C. pubescens 16.12 ± 0.35, and P. persica 11.55 ± 0.25 mg, respectively. That is, there is a significant fitness cost when A. ludens infests C. pubescens cv. Manzano and P. persica cv. Criollo, suggesting the presence of potentially toxic capsaicinoids (Manzano pepper) and polyphenols (Criollo peach). Given that we were principally interested in determining bacterial diversity associated with host plants and the possible role of the microbiota in host exploitation, we concentrated our effort in determining bacteria in fruit pulp (tissue in which larvae had naturally developed), gut of larvae and freshly emerged adults stemming from the latter larvae. We pursued the following questions: (1) How is the microbial composition of the larval and adult microbiota related to the composition of food microbiota? and (2) what does this tell us about transience and stability of this species’ gut microbiota from larvae to adult metamorphosis? Based on the extreme polyphagy of A. ludens, we hypothesized that independent of host, we would find a core microbiota aiding the fly in the digestion of multiple substrates encountered by the larvae in the pulp of the many hosts into which a female lays eggs over the various fruiting seasons along the year.
Materials and Methods
Hosts and Collection Sites
The six hosts selected are representative of the wide array of fruit exploited by A. ludens over its geographic range [S Texas to Costa Rica (Norrbom et al., 2000)]. We collected naturally infested fruit mainly in the State of Veracruz, Mexico but also in the States of San Luis Potosí, Nuevo León and Morelos (Figure 1 and Supplementary Table 1).
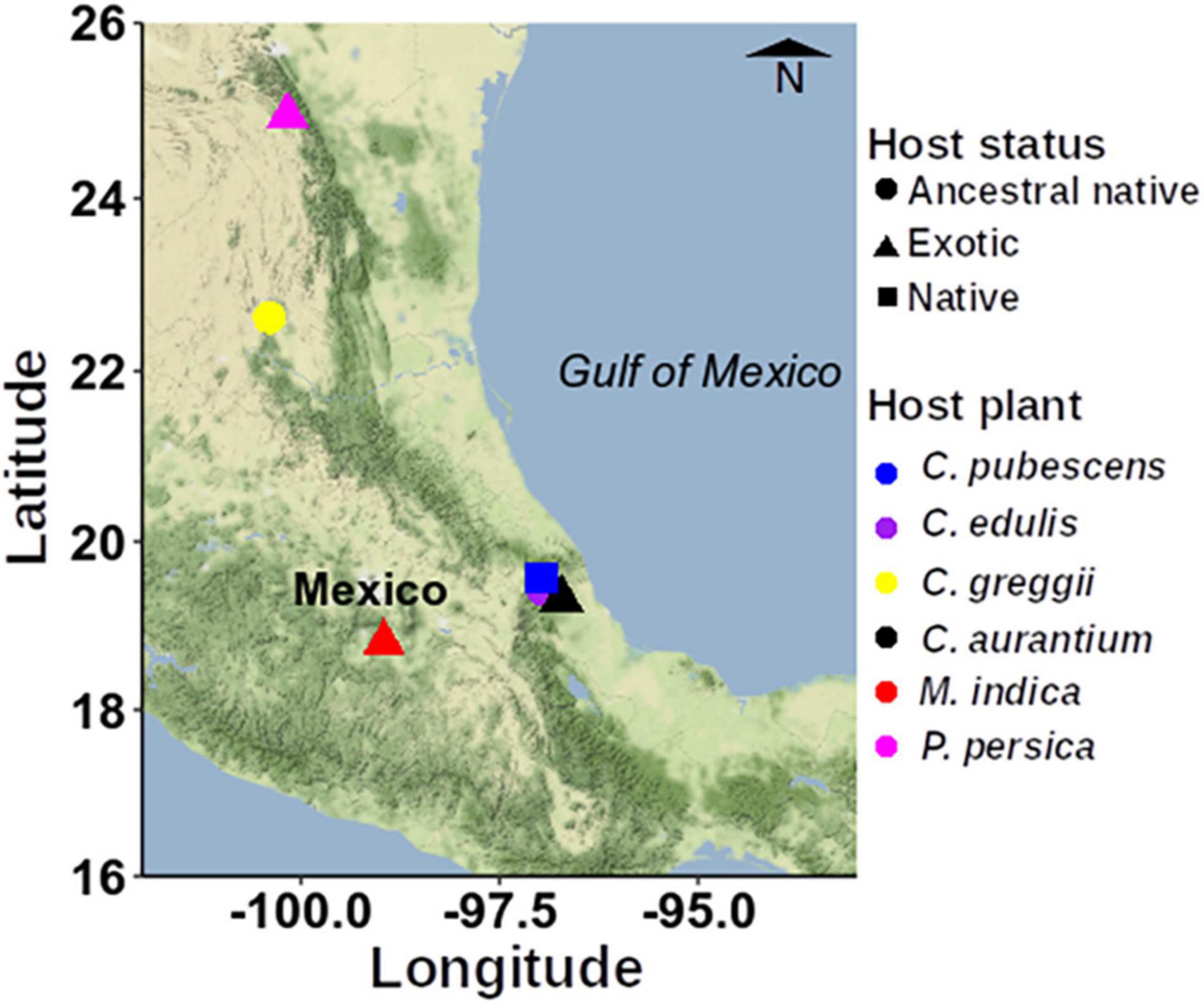
Figure 1. Map showing the sampling sites within Mexico used to collect the infested fruits. To construct this figure, we used the ggmap spatial visualization package (Kahle and Wickham, 2013).
Sample Processing
In the field, individual overripe fruit of the six Anastrepha hosts studied (details in Figure 1), with signs of infestation by fruit fly larvae (e.g., breathing/exit orifices) were collected while still attached to branches and immediately dissected on site to obtain pulp and larva samples. We cut open the fruit with a sharp, sterile knife, and in the vicinity where third instar larvae were detected, a sample of ca. 0.5 g of pulp was obtained with a sterile spatula. A caveat related to this sampling approach, is that we cannot rule out the possibility that the pulp in the vicinity of where larvae were feeding was not contaminated by bacteria present in the eggs or the feces of larvae and recognize that ideally, we should have compared this pulp from infested fruit with pulp from uninfested fruit stemming from the same tree to conclusively determine if indeed the presence of larvae contaminated the undamaged pulp. But as females are highly selective when choosing fruit to lay their eggs, we thought that sampling pulp from uninfested fruit would have biased our results as there was the possibility that undamaged fruit surrounding the infested fruit were somehow unsuitable based on the decision made by the female (Birke and Aluja, 2018).
Samples were immediately transferred to a 2 mL vial and frozen in liquid nitrogen. In addition, we fetched five larvae crawling in the pulp with the help of sterile tweezers. As was the case with pulp samples, larvae were individually placed in 2 mL vials, and immediately frozen in liquid nitrogen. Before DNA extraction, larvae were individually surface sterilized using a sterile washing solution (SDS 1%, Tris 10 mM, and NaCl 10 mM) for 1 min, 1% commercial bleach for one min, 70% ethanol for one min and finally, two 1-min washes with sterile distilled water.
Additional fruit in the same condition and collected directly from the tree were transported back to the laboratory (some field sites where over 700 km away) and handled in 10 L plastic containers into which a 1-cm thick layer of sterile vermiculite was placed in the bottom part of the container. This allowed any larvae inside fruit to exit it and crawl into the vermiculite to pupate. Once back in the laboratory, vermiculite or any pupae that were already formed were sprayed with sterile distilled water until they emerged as adults. Newly emerged adults were dissected under sterile conditions to isolate the complete digestive system, from the cardia to the anus. Ten adult gut samples from specimens emerged from each of the six fruits studied were stored in RNA later and preserved at −80°C until their analysis.
DNA Extraction, 16S Gene Amplification and Sequencing
Each pulp DNA extract represented a combined pool of five 20 mg pulp samples, each larval DNA extract stemmed from a single larva, and each adult DNA extract represented a combined pool of two adult guts. We used five replicates per sample type (pulp, larva, or gut). Total DNA was extracted using QIAamp DNA Mini Kit (Qiagen©, Hilden, Germany). Amplicons of the variable V3 region of the 16S gene were amplified from 100 ng of total DNA samples using the primers described by Klindworth et al. (2013). PCRs (50 μL) containing Qiagen© buffer 1X, dNTPs 0.2 mM, 16s F&R 0.2 μM, Taq polymerase 2.5 U were performed using the following amplification program: 94°C/2 min of initial denaturation followed by 25 cycles of 94°C/15 s denaturation, 55°C/30 s annealing, and 72°C/1 min amplification, and a final amplification at 72°C/5 min. Illumina libraries were constructed by adding Nextera XT adapters (Illumina Inc.©). Initial amplification and adapter-ligation PCRs were performed using Kapa polymerase® (Kappa Biosystems©) and purified immediately after finishing each PCR using 0.8X Agencourt Ampure XP cleaning beads® (Beckman Coulter©). Library concentration was quantified using a DNA HS kit (Invitrogen©) in a Qubit 2.0® fluorometer (Invitrogen©). Library size was evaluated using a Bioanalyzer DNA HS® chip (Agilent©). Libraries were diluted and pooled to an equimolar concentration to be denatured and loaded into a MiSeq® sequencer using a MiSeq 600-cycle Reagent kit V3® (Illumina Inc.©). Sequencing procedures were performed at INECOL’s sequencing unit in the BioMimic® Scientific and Technological Cluster.
Bioinformatic Analyses
Raw sequences were processed using the DADA2 package to resolve the amplicon sequence variants (ASVs) (Callahan et al., 2016). We included the following filter criteria: (i) cut of the sequences at 280 and 230 bp, of the sense and antisense reads, respectively; (ii) an error threshold of one biased assigned base in sense reads and two in antisense reads, respectively; and (iii) deletion of sequences with ambiguous bases. From the filtered sequences, error modeling was performed (Callahan et al., 2016). The paired sequences were merged and filtered to remove chimeric sequences using the “removeBimeraDenovo” algorithm with the “consensus” method (Callahan et al., 2016). Sequences were used to construct merged sequences. Then, the taxonomic assignment was performed with DECIPHER 2.14.0 (Wright, 2016) using the SILVA database version 138 (Quast et al., 2013). Sequences that were unidentified, those with a relative abundance of less than 1%, and those identified as “Mitochondria” and “Chloroplast” were removed at the phylum level (Callahan et al., 2016). The feature table was standardized to the number of sequences of the smallest library. The statistical analyses were performed with the phyloseq (McMurdie and Holmes, 2013), ggplot2 (Ginestet, 2011), ggalt1, and vegan (Oksanen et al., 2008) packages.
To test whether the microbiota differed between adult males and females, we assessed the Beta diversity of the bacterial communities between males and females using a PERMANOVA analysis (pairwise test with 1,000 permutations), based on Bray-Curtis similarities. The Shannon diversity indices were calculated, and statistical significance was estimated using a paired t-test with an α = 0.05. Networks were calculated running the make_network function of the phyloseq package initially using the object phyloseq. Results were graphed with the function plot_network. For the make_network function we used the following parameters: max.dist = 0.9, dist.fun = “jaccard.” The parameter max.dist defined the maximum ecological distance allowed between two samples to still be able to consider them connected by an “edge” in the graphic model. For the plot_netword function, we specified the following parameters: color = “origin,” shape = “host,” line_weight = 0.2, label = NULL.
Non-metric multidimensional scaling (NMDS) with the unweighted unifrac distance was applied to the data to assess grouping by host plant among the microbiota of the pulp, larvae, and adults. In each case, we drew grouping lines, which were obtained with the geom_encircle function of the ggalt package (see footnote text 1). To visualize the top-most representative genera, we plotted those genera that represent more than 10% of the relative abundance per host plant. The core microbiota, or the set of amplicon sequence variants detected in 50–100% (prevalence) of the samples with a relative abundance threshold value above 0.01%, was identified using the core function in the microbiome R package version 1.5.28 (Leo and Sudarshan, 2017). A linear discriminant analysis (LDA) effect size (LEfSe) method was applied to identify taxonomic biomarkers, which were performed with the microbial2 package. All analyses were performed in the R environment (“R Team3”), version 3.6.3.
All raw sequence data were deposited in the NCBI Short Read Archive under the Bio project PRJNA715941.
Results
The number of raw sequences per sample, after filtering, merging, and removing chimeras is shown in Supplementary Table 2. Across all the samples, ASVs identified were distributed among 13 bacterial phyla, 96 families, and 190 genera. The rarefaction curves constructed to estimate the extent of the analysis showed that the curves of all libraries reached the plateau phase or almost did so, supporting the depth of the sequencing effort (Supplementary Figure 1).
Figure 2 shows the relative abundances at the family level (details in Supplementary Table 3). In this figure, only taxa with >1% of relative abundance are shown. It is evident that the relative composition profile of the larvae was more like the one observed in the pulp but dissimilar to the adult (male or female) stage and that the structure was simpler in adults than in larvae and pulp (Figures 2, 3). Moreover, males and females exhibited very similar composition profiles with the Enterobacteriaceae being clearly dominant, with exception of adults emerged from P. persica, in which the Rhizobiaceae family was also dominant. In pulp and larvae, Acetobacteraceae and Enterobacteriaceae were dominant, except in C. edulis, where no clear dominance was observed.
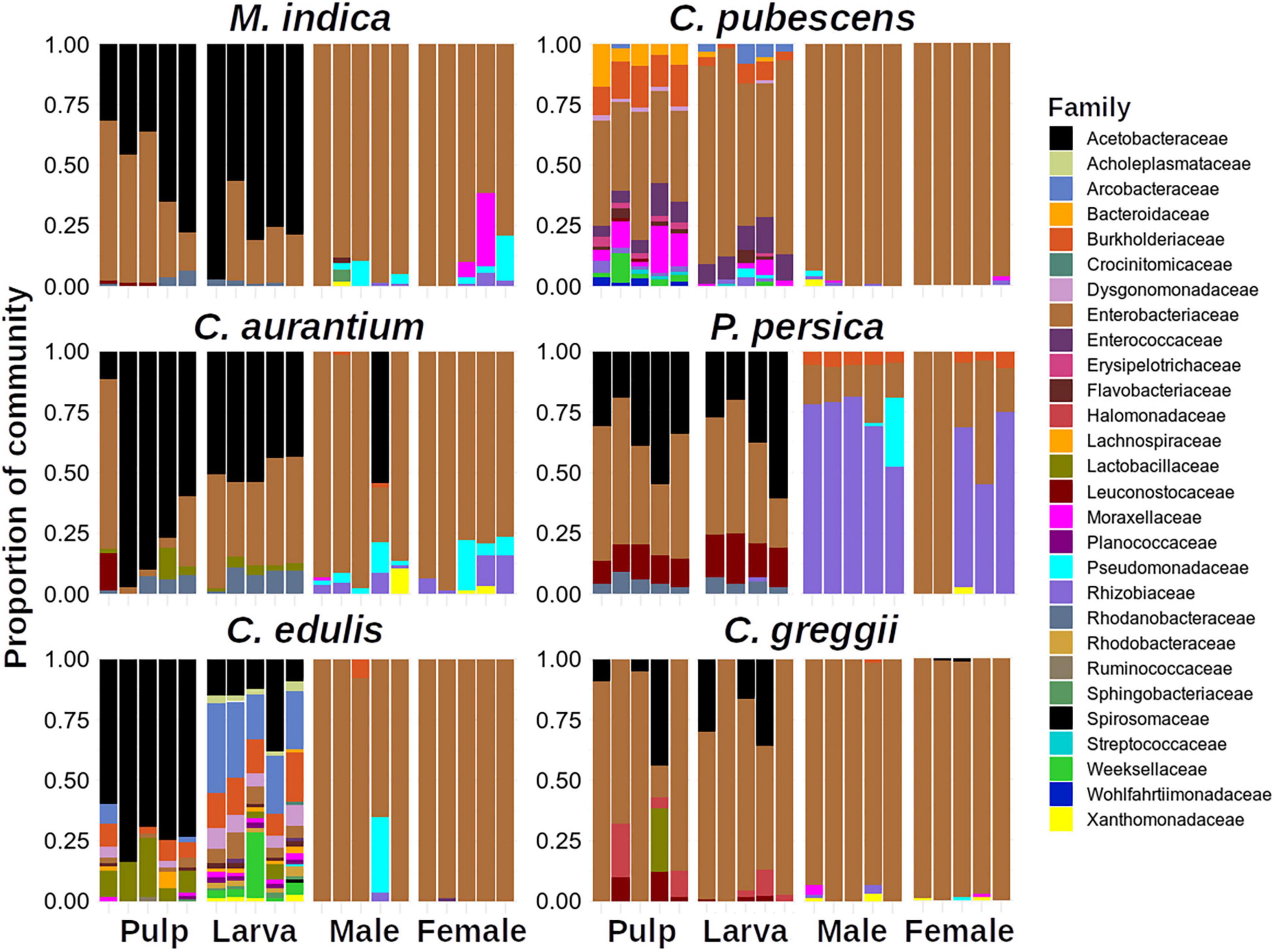
Figure 2. Relative abundances of Amplicon Sequence Variants (ASVs) among host plants at family level. Note that little differences are observed between males and females.
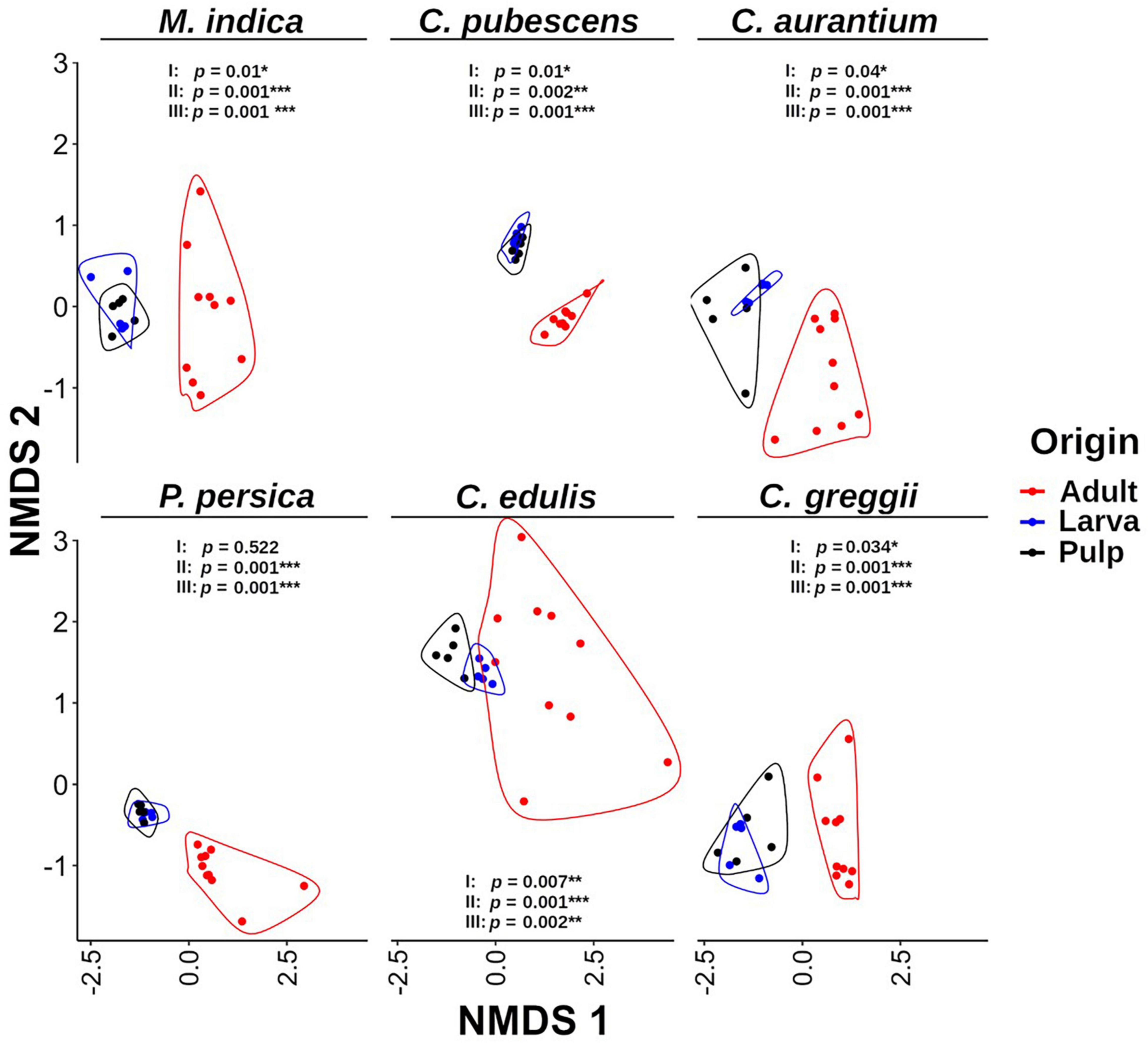
Figure 3. Non-metric multidimensional scaling (NMDS) analysis of the microbiota of pulp, larvae, and adults within host plant. It is evident that the gut microbiota of larvae is grouped with the pulp microbiota and both are separated from the microbiota of adult samples. The p-value corresponds to the results of the PERMANOVA test. I: larva vs. pulp; II: larva vs. adult; III: pulp vs. adult. Grouping lines were performed with the geom_encircle function of the ggalt package.
As the relative abundance of taxa at the family level between males and females was quite similar, we decided to perform a statistical comparison between the microbiota of both sexes. In the comparison of male versus female gut microbiota, the PERMANOVA analysis detected no statistically significant differences between males and females [df = 1, F = 0.70247, R2 = 0.01197, Pr(>F) = 0.893; Table 1]. The data from females and males were therefore grouped under the category of “adults” for the comparisons of the microbiota of the pulp, larvae, and adults by host plant.
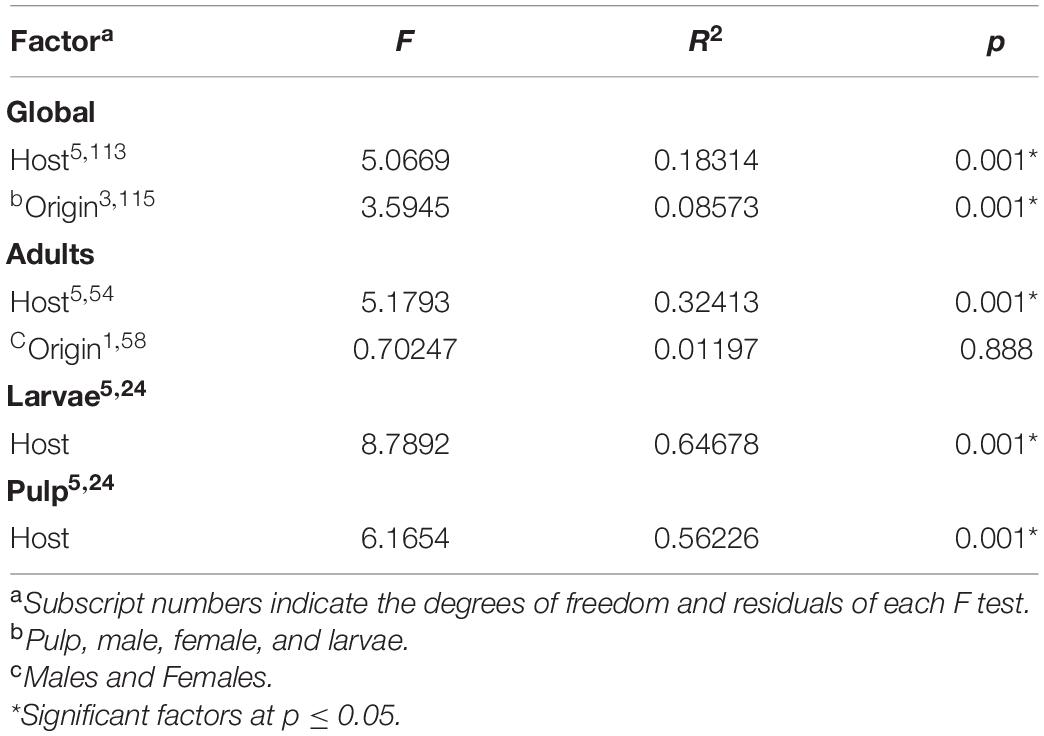
Table 1. PERMANOVA analyses of the bacterial communities associated with Anastrepha ludens considering all factors (i.e., host species, larvae, and fruit pulp).
In general, within each host plant, adults, pulp, and larvae exhibited similar alpha diversity with C. pubescens being a notable exception (Figure 4). The pulp of this fruit had a more diverse microbiota than the larvae and adults. Interestingly, the gut microbiota of the larvae that developed in C. edulis had a more diverse microbiota than the pulp or adults. The comparisons of alpha diversity flushed out large differences in pulp, larval, and adult microbiota among host plant species (Supplementary Figure 2). Notably, the pulp microbiota of C. pubescens was the most diverse while the pulp of C. edulis was the least diverse. The microbiota of larvae that developed in C. pubescens was also the most diverse, while the microbiota of larvae that developed in C. greggii was the least diverse. In general, differences in adult microbiota among host species were less pronounced than in pulp and larvae.
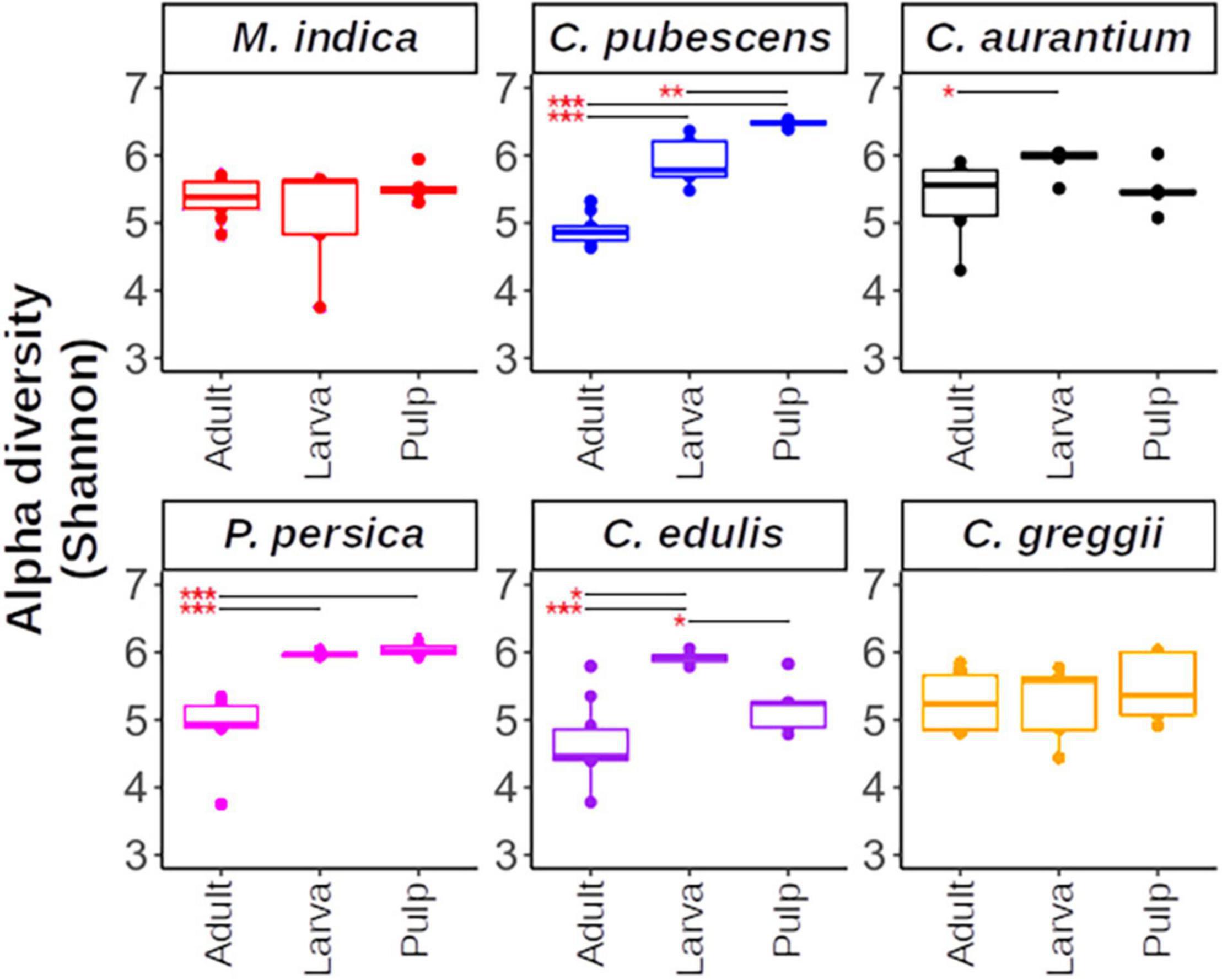
Figure 4. Alpha diversity within host plants estimated using the Shannon index. Upper and lower whiskers, 3rd, and 1st quartiles (top and bottom of the box), and median (horizontal line within the box) are displayed. Asterisks indicate significant differences between the pairs connected by the horizontal line. For paired t-test, *, **, *** represent p < 0.05, 0.01, and 0.001, respectively.
Based on the NMDS analysis of the Bray-Curtis distances of the microbiota of the pulp, larvae, and adults within each host plant species, the microbiota of the pulp and the larva were grouped together in each of the hosts and were separated from the adult microbiota (Figure 3). Except for P. persica, the PERMANOVA test indicated that the microbiota of the fruit pulp was different from the microbiota of the larvae in all host plants. However, it is worth mentioning that these differences were statistically less pronounced when compared to the differences detected among the microbiota of the pulp or the larva and the microbiota of the adult (Figure 3). When we compare the NMDS of larvae and pulp considering all host species, it also becomes clear that the microbiota of the larvae was related to the microbiota associated with the pulp the larvae fed on in all cases (Figure 5A). Importantly, in almost all cases, the microbiota of the pulp, larva and adults differed among host plants (Figure 5B).
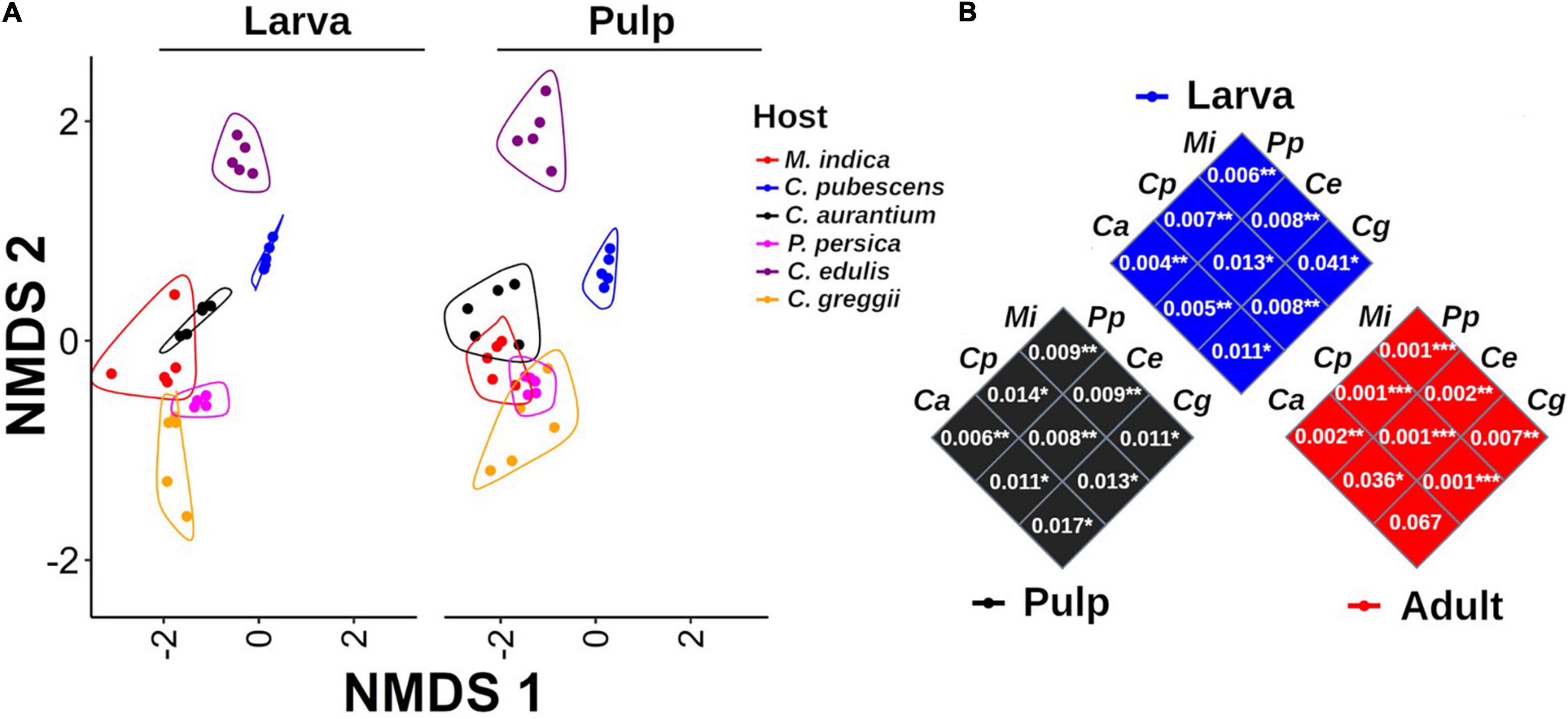
Figure 5. Non-metric multidimensional scaling analysis of the microbiota of pulp and larvae including all host species. (A) The microbiota of pulp and larvae exhibit a highly similar pattern among all host species. Grouping lines were drawn with the geom_encircle function of the ggalt package. (B) PERMANOVA comparisons among samples per origin (pulp, larva, and adult). P-values of the paired comparison resulting after running the PERMANOVA test are shown inside each square. Mangifera indica (Mi), C. x aurantium (Ca), Casimiroa edulis (Ce), C. greggii (Cg), Capsicum pubescens (Cp), and Prunus persica (Pp).
In the network analyses we observed that samples clustered according to the origin of the microbiota (adult, larvae, and pulp) and its host (Figure 6). The pulp microbiota clearly separated into three groups: (i) C. edulis, (ii) the hosts in which the fly develops well (the other native and all exotic hosts) and (iii) C. pubescens. The microbiota of the larvae was well grouped with the microbiota of the pulp in all cases and the microbiota of the adults was different from the microbiota of the pulp and larvae.
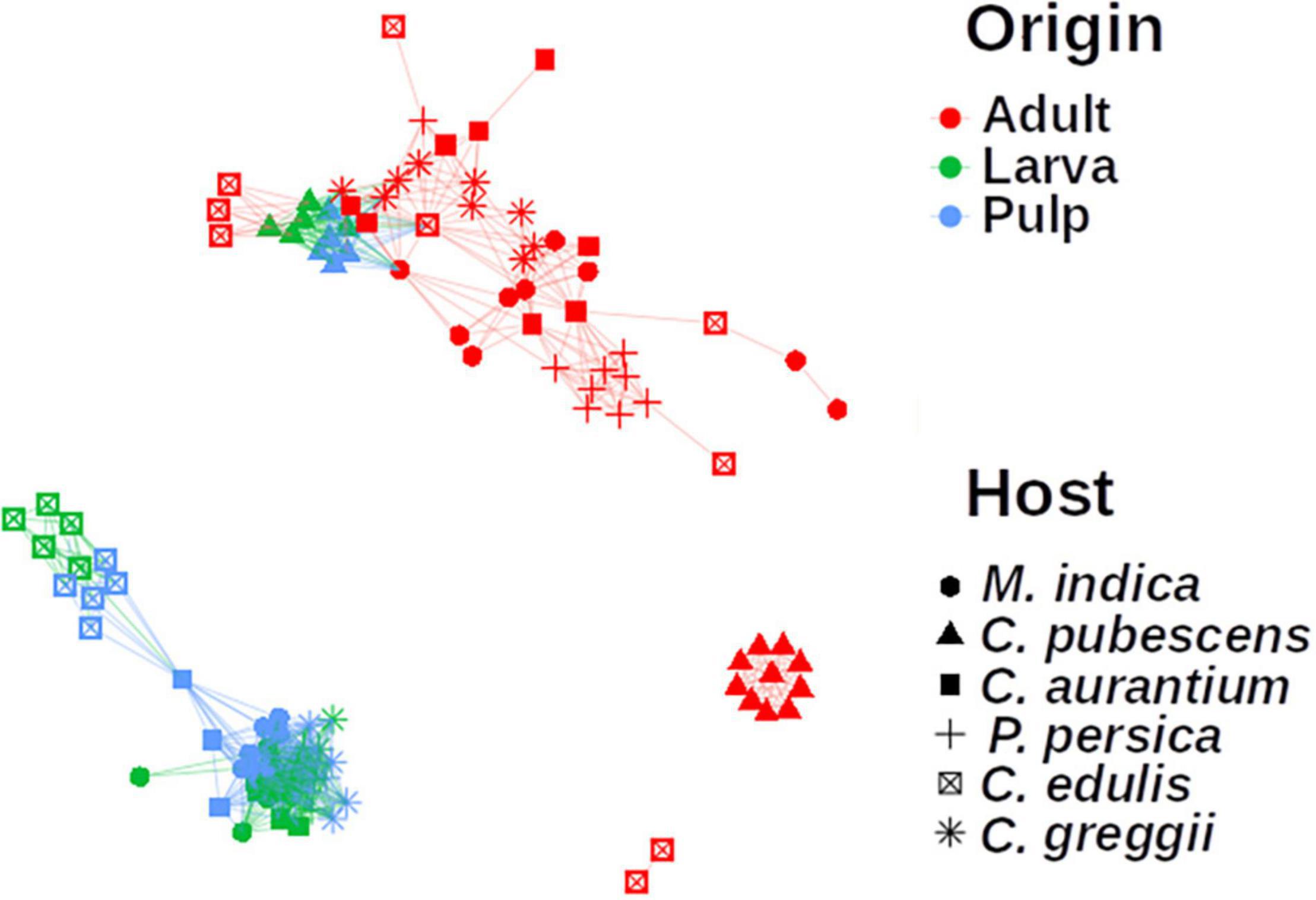
Figure 6. Network analysis of the microbiota of pulp, larvae, and adults, including all plant host species. The microbiota of pulp and larvae clustered together for all samples. Nodes represent the microbiota of each sample. Links between the nodes indicate significant correlations. The colors represent the origin the sample came from and the shape represents which host the microbiota is related to. The microbiota of the pulp and larvae of C. edulis and C. pubescens are grouped separately.
Since the microbiota of larvae developing in C. edulis and C. pubescens were clear outliers with respect to the microbiota of the larvae of the other host species analyzed, we compared the microbiota of larvae that developed in these two fruits with that of the other host plants to explore their differential taxa (Supplementary Figure 3). Based on this analysis, the family Acetobacteraceae was underrepresented in C. pubescens compared to the microbiota of the rest of the host species, while the genera Providencia, Morganella, and Vagoccocus were over-represented. In C. edulis, the Weeksellaceae, Acholeplasmataceae, Arcobacteraceae (newly reclassified group with interesting characteristics), Burkholderiaceae families, and the Empedobacter, Lampropedia, and Dysgonomonas genera were over-represented compared to the remaining host plants.
Finally, we found that the microbiota of the larva was strongly influenced by the host it developed in, which becomes evident when we observe the topmost abundant taxa among the larvae microbiota from the different host plants (Figure 7). The size and composition of the core microbiota of the larva varies according to the host species (Figure 8). Therefore, we integrated the data of all hosts into this analysis and found that the estimated core microbiota of the larvae was more diverse than the core microbiota of newly emerged adults, and it is equivalent to that of the pulp. Most of the bacteria identified as part of the adult core microbiota belonged to the Enterobacteriaceae, and to a lesser extent to the Rhizobiaceae, Pseudomonadaceae, and Burkholderiaceae families and the Stenotrophomonas genera. Notably, except for the genera within the Enterobacteriaceae, none of the other genera that constitute the core microbiota of adults are shared with the core microbiota of the pulp or the larvae. Given that the adults studied emerged from puparia in a sterilized cage, these bacteria, likely of environmental origin (pulp of fruit the larvae developed in), must have been transferred from the larvae to the adult via the pupae surviving metamorphosis.
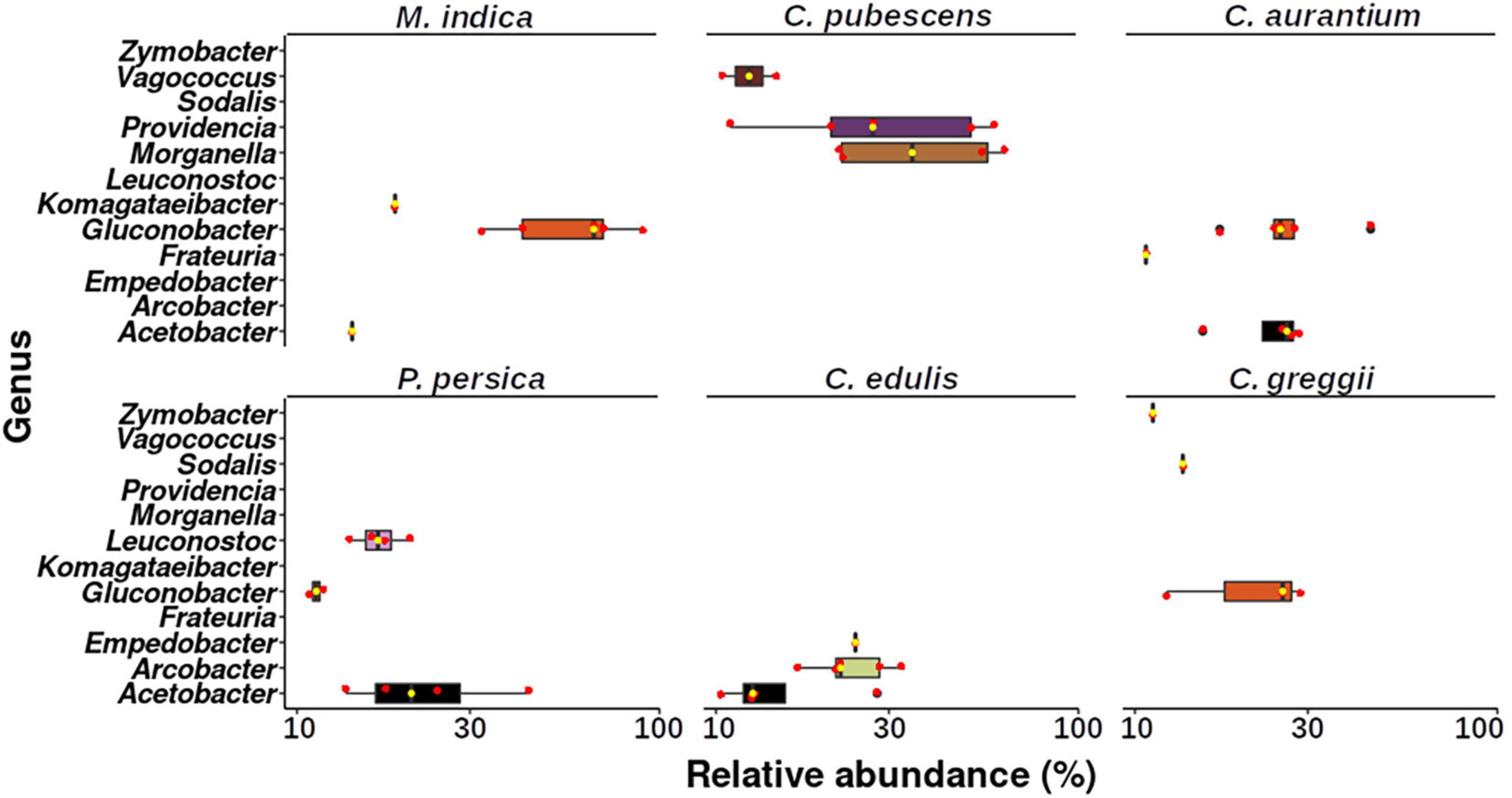
Figure 7. Boxplot showing the genera that represent more than 10% of the relative abundance in each larva sample per host plant. Upper and lower whiskers, 3rd and 1st quartiles (top and bottom of the box), and median (horizontal line within the box) are displayed. Median is also indicated as a yellow dot. Red dots represent the individual values of each sample.
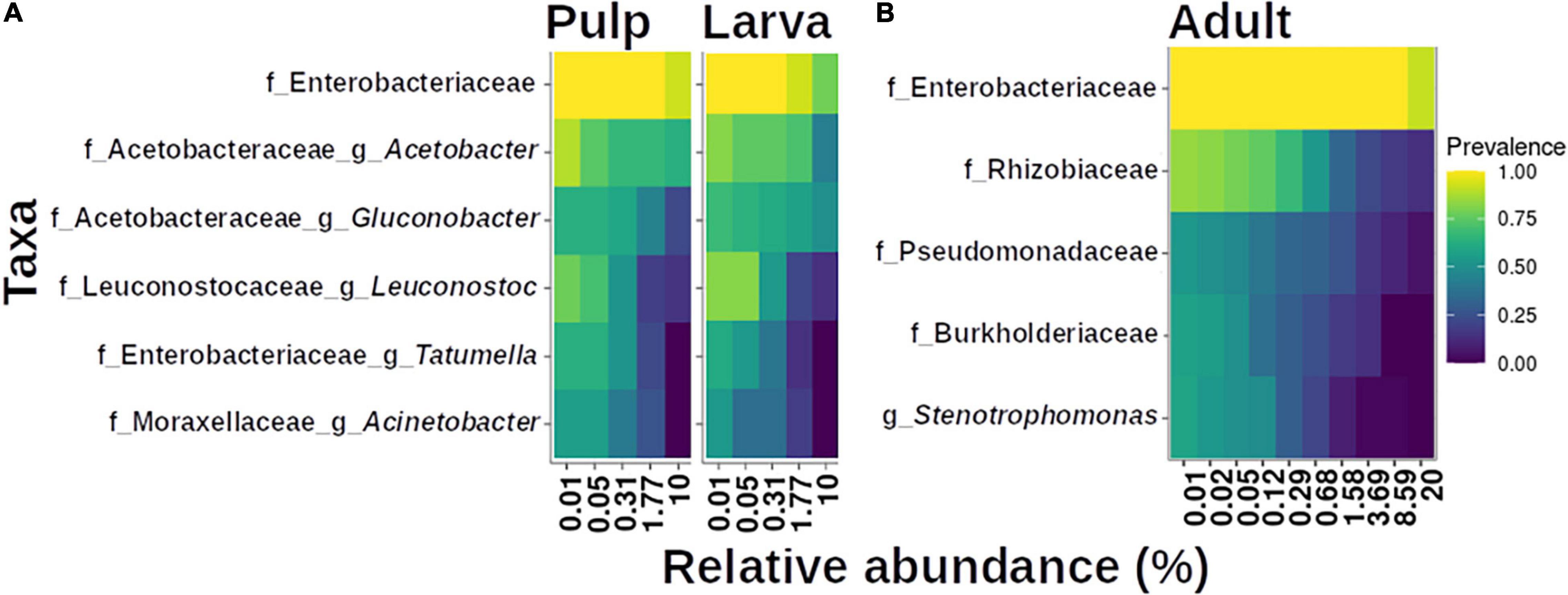
Figure 8. Core microbiota analysis based on relative abundance and sample prevalence of bacterial genera in pulp, larvae (A), and adult (B) gut microbiota. For this analysis, the Amplicon Sequence Variants (ASVs) were agglomerated at the genus level. Only genera that were present in at least 50% of the samples within each category are displayed.
Discussion
In this study, we pursued the questions of how the microbiota of the larval food is related to the composition of the larval and adult microbiota, and what does this tell us about transience and stability of this species’ gut microbiota and how does metamorphosis affect the adult microbiota by analyzing the microbiota of A. ludens developing in six of its hosts. This included two ancestral hosts (C. edulis and C. greggii), three exotic hosts (M. indica, P. persica, and C. x aurantium) and one occasional host (C. pubescens) that is only used when environmental conditions (such as extreme drought) limit fruiting by the fly’s typical hosts (Thomas, 2004). We deliberately included four highly amendable hosts (C. edulis, C. greggii, M. indica, and C. x aurantium) where the larvae of A. ludens develop with little fitness costs, and two hosts in which larvae suffer various fitness costs (C. pubescens and P. persica; Birke and Aluja, 2018). So, by including these host species, we worked with a gradient spanning all the way from the two ancestral hosts (C. edulis and C. greggii), several exotic but highly suitable hosts, to two natural but sub-optimal hosts (C. pubescens and P. persica). Notably, the pulp microbiota of the marginal host C. pubescens was the most diverse while the pulp of one of the purported ancestral hosts, C. edulis, was the least diverse. Furthermore, the microbiota of larvae that developed in C. pubescens is also the most diverse, while the microbiota of larvae that developed in the other purported ancestral host, C. greggii, was the least diverse.
As can be ascertained in Figure 1, some sampling sites are quite distant from each other. We recognize that ideally, we should have sampled all fruit in a relatively small geographic area to avoid a possible confounding effect between host plant species and geographic site. But this was impossible given biological/ecological considerations. For example, in the case of the two purported ancestral hosts within the Rutaceae, C. edulis, and C. greggii, they very rarely co-occur, and when this is marginally the case, it only happens in highly isolated areas that are not only difficult to reach, but also dangerous because of local drug trafficking. But in the case of C. greggii and C. pubescens/Mangifera indica, natural co-occurrence never happens. Based on Liu et al. (2018) and Nikolouli et al. (2020), we are addressing this issue in an additional massive study with A. ludens larvae developing in C. x aurantium a host that has a very wide distribution, which was sampled along a latitudinal and an altitudinal gradient in the state of Veracruz, that is the “longest” state in Mexico, with 803 km separating the northernmost and southernmost borders. In the case of the altitudinal gradient, we sampled all the way from 0 to 100 m above sea level, to 1,800–2,000 m. According to Nobles and Jackson (2020) we could expect an influence of collection site (geography) in larvae but not adults, or vice versa. In contrast, Majumder et al. (2019), working with the tephritid fly B. tryoni, concluded that “geographic location may play a quite limited role in structuring of larval microbiomes.”
Based on our data, we found that the composition of the gut microbiota of A. ludens larvae is plastic rather than stable, depending largely on the microbiota associated with the pulp on which they fed. The proportion of microorganisms in the gut that belong to the resident versus the transient microbiota can vary enormously from one host species to another. For example, some insect species possess a gut microbiota mainly constituted by stable, resident microbiota, as occurs with the American cockroach or B. tryoni (Tinker and Ottesen, 2016; Majumder et al., 2019). Other species have a much less stable gut microbiota mainly conformed by transient microbiota, as has been documented in larval or adult butterflies (Hammer et al., 2017; Phalnikar et al., 2019; Ravenscraft et al., 2019a,b), the giant neotropical bullet ant, Paraponera clavata or other rainforest ants (Sanders et al., 2017), Drosophila melanogaster or other species within Drosophila (Wong et al., 2013; Hammer et al., 2017, 2019; Moreau and Rubin, 2017; Ma and Leulier, 2018; Ross et al., 2018; Phalnikar et al., 2019), the cabbage stem flea beetle Psylliodes chrysocephala (Shukla and Beran, 2020) or other beetles (Kelley and Dobler, 2011), hard ticks (Guizzo et al., 2020), dragonflies (Deb et al., 2019), and mosquitoes (Coon et al., 2016), but this phenomenon had not been documented previously in the highly polyphagous and pestiferous A. ludens or any other Anastrepha species. In relation to other fruit flies, a study conducted in Australia with Bactrocera tryoni (Majumder et al., 2019), indicates that the larval gut microbiota is weakly related to the host fruit microbiota, and rather more strongly associated with vertical transfer of bacteria by females during egg laying. These contrasting results are important, since they suggest that the stability of the gut associated microbiota is not a conserved trait among different fruit fly species. Thus, the degree of plasticity of the gut microbiota among fruit fly species is a topic that deserves further attention. Interestingly, in the case of B. tryoni the existence of a high similarity between mycobiome in larvae and their respective host fruit source was documented (Majumder et al., 2020a), suggesting that these fungal communities are closely interconnected, which is in direct contrast with what occurs with bacteria.
The notable differences among the pulp-associated microbiota of the six different Anastrepha hosts discovered here, suggest that plants have developed associations with microorganisms along their evolution. So far, few studies have addressed the specificity of fruit microbiota (Dees et al., 2015; Wassermann et al., 2017; Kõiv et al., 2019), but the implication of this specificity could also have important repercussions for plant-insect interactions. In general, there seems to be a consensus indicating that the transient microbiota plays no significant role on the insect’s physiology (Hammer et al., 2017), though notable exceptions exist (Kikuchi et al., 2007; Coon et al., 2016; Heys et al., 2018; Ross et al., 2018). Sometimes the acquisition of microbes from the environment can provide symbionts with functions that are uniquely relevant, including the ability to digest the food which the microbes originally came from Ravenscraft et al. (2019a) and Shukla and Beran (2020).
Here, we provide consistent evidence documenting that pulp-associated microbiota shapes the gut microbiota of A. ludens larvae which seems to be mainly transient. But despite this, there are bacterial genera that can have positive or negative effects on larval development, as occurs with mosquitoes (Coon et al., 2016). Given that these transient microorganisms are continuously entering the gut of larvae through their voracious feeding, their positive/negative effects should not be underestimated. Based on our results, perhaps the larvae of A. ludens do not develop well in C. pubescens and P. persica not only because of the intrinsic biochemical composition of their pulp (containing deleterious compounds for the larvae), but also because of the associated microbiota found in the pulp of these fruit. We highlight the fact that the pulp-associated microbiota of C. pubescens is clearly different from the microbiota of the other host species (Figures 2, 3, 6). For example, when the pulp microbiota of this fruit was compared with the microbiota of the other five host species, we found that C. pubescens is enriched with Providencia and Morganella, two bacterial genera widely recognized as pathogenic for insects. Providencia has been reported as pathogenic for D. melanogaster and C. capitata (Galac and Lazzaro, 2011; Msaad-Guerfali et al., 2018), although this genus is often found in the gut of A. ludens adult flies (Kuzina et al., 2001; Nikolouli et al., 2020). On the other hand, Morganella is a recognized pathogen for the larvae of this fruit fly species (Salas et al., 2017). So, the role of both genera in the larval development of A. ludens in C. pubescens must be addressed in the future. In the same comparisons, the microbiota of C. pubescens shows under-representation of symbiotic bacteria with positive effects for the development of insects, as is the case of Acetobacter. Acetobacter is a generalist bacterial genus which usually thrives in carbohydrate-rich environments. Not surprisingly, it is often found in insects developing/feeding on a carbohydrate-rich diet (Crotti et al., 2010). Species within this genus are frequently symbionts of species within Bactrocera, such as B. oleae (Kounatidis et al., 2009) or B. tryoni (Woruba et al., 2019).
A caveat related to the above is that we did not study the bacteria present in the pulp of uninfested fruit, with no eggs or larvae in them (absolute control). We therefore recognize that our results here need to be confirmed by additional studies contemplating the following two sources of bacteria in the microbiota of A. ludens guts: (1) microbes naturally present in unaltered fruit; (2) microbes inoculated into the pulp by the mother, the egg surface, or possibly the egg interior. Furthermore, we recognize that the growing larvae could have altered the composition of resident microbes in the pulp via chemical changes, such as introduction of fecal material containing nitrogenous waste. Likely, all three forces may be at play, and this needs to be further investigated.
Since Acetobacteraceae is an over-represented family in the A. ludens gut microbiota when it feeds in the pulp of natural hosts where it develops well, this taxon may promote metabolic homeostasis of A. ludens by acting as probiotics. Similarly, the families and genera that are over-represented in C. edulis with respect to the other hosts also deserve attention, since they could provide new clues about how transient bacteria benefit their hosts. Although the families Weeksellaceae, Acholeplasmataceae, Burkholderiaceae, and the genera Empedobacter, Lampropedia and Dysgonomonas have been previously reported as part of the gut microbiota of A. ludens (Salas et al., 2017; Ventura et al., 2018) and other fruit fly species (Martinson et al., 2017; Hadapad et al., 2019; Robert et al., 2019), the description of their association with this ancestral host species is new. In addition, we found that the genus Leuconostoc was more abundant in the larvae reared in P. persica than in C. edulis or C. pubescens. Interestingly, in B. tryoni, Leuconostoc was found to induce a delay in larval development (Shuttleworth et al., 2019) and it could contribute to explain the fitness cost observed in the A. ludens larvae reared in this fruit (Birke and Aluja, 2018). Besides, to our knowledge, the family Arcobacteraceae has not been previously described as part of the gut microbiota of A. ludens. The few studies on this microorganism have focused on its potential as emergent pathogens in other animal models (Shah et al., 2011; Waite et al., 2017; Chieffi et al., 2020). Since bacteria of the Arcobacteraceae family were present in the C. edulis pulp in much higher proportion than in A. ludens larvae stemming from this fruit, identifying the species of Arcobacteraceae and their functions could be important for the development of new probiotic formulas for mass rearing of this species. Furthermore, all this could not only imply that A. ludens is able to tolerate a wide range of microorganisms in its gut, but also suggests that A. ludens larvae are self-sufficient digestors since its functionality does not depend on the co-occurrence of a constant, specific microbiota, as has been previously proposed for insects without a stable microbiota (Hammer et al., 2019). In addition, it is likely that pulp-associated microbiota also promotes or limits the polyphagy of A. ludens by adding positive or negative impacts on larval development/fitness. If this is true, a similar dynamic may occur in other plant-insect interactions.
We found that the gut microbiota composition of adult flies (males and females) is remarkably different from that of larvae. This is an important finding, because although the larvae feed on different plants and thus interact with a very heterogeneous microbiota associated to the pulp of the particular host plant they are developing in, adult flies are much less dependent on the plant for their metabolic maintenance. We found a dominance of bacteria within the Enterobacteriaceae in the gut of adults. The dominance of members of this family in the gut microbiota of adults of other species of fruit flies has been widely reported, as is the case of Bactrocera, C. capitata, A. obliqua, or Zeugodacus tau (Andongma et al., 2015; Yong et al., 2017; Gallo-Franco and Toro-Perea, 2020; Naaz et al., 2020; Nikolouli et al., 2020, Noman et al., 2021). In contrast, the lack of differences between males and females should come as no surprise, as similar results have been reported for other fruit flies as is the case with B. tryoni (Woruba et al., 2019; Majumder et al., 2020b), Zeugodacus cucurbitae (Hadapad et al., 2019), or C. capitata (Nikolouli et al., 2020). According to our data, the relationship between the richness of the gut microbiota of larvae and adults is not constant, because it depends on the particular fruit or substrate where the larvae developed.
Based on our results, metamorphosis plays a key role in shaping the gut microbiota of newly emerged adults. Our findings coincide with other studies that demonstrated sharp differences in the microbiota of immatures and adults of other insects such as butterflies, Drosophila melanogaster, or other true fruit flies (i.e., Tephritidae), all documenting a decrease in the diversity of the microbiota in pupae and adults when compared to larvae (Kingsley, 1972; Wong et al., 2011; Hammer et al., 2014; Yong et al., 2017; Malacrinò et al., 2018; Andongma et al., 2019; Majumder et al., 2020b; Nobles and Jackson, 2020). Like us, these authors also found that the microbiota of larvae (in holometabolous insects) or nymphs (in hemimetabolous insects) is influenced by the microbes present in their feeding substrate or surrounding environment and attributed the loss of diversity in pupae and adults to metamorphosis. Kingsley (1972), working with the monarch butterfly (Danaus plexippus), was one of the first in suggesting that the loss of diversity in pupae and adults could be due to the major anatomical reconstruction of the alimentary canal during the metamorphosis process and that the larvae could promote the elimination of their bacterial complement to facilitate the introduction and establishment of new bacteria more suitable/amendable for the adults (and their diet). In holometabolous insects that exhibit a complete metamorphosis, the larval gut epithelium is completely replaced during pupation, and during this process, the insect must control the gut microbiota to avoid septicemia and at the same time avoid the loss of beneficial mutualists (Johnston and Rolff, 2015). In lepidopterans it has been reported that within the newly formed gut epithelium immune effectors or lysozyme are synthetized before delamination of the larval epithelium, which are discharged into the lumen when the epithelium is complete as a prophylactic response to protect the insect from microbial infections (Russell and Dunn, 1991, 1996; Johnston et al., 2019). Recently, Huang et al. (2019) studying the microbial communities in different developmental stages of B. dorsalis found that microorganisms in larvae and adults are primarily Gram-negative and the major components in pupae are Gram-positive bacteria. They suggested that maintenance of the microbiota in the different developmental stages is associated with expression of the genes encoding peptidoglycan recognition proteins (PGRP-LB and PGRP-SB1). In the case of A. ludens, all the latter still needs to be studied. However, based on detailed recent studies on the gut of this insect (Guillén et al., 2019), we suggest that flies could recover important bacteria from other structures linked to the gut that harbor microbes such as the dorsal esophageal bulb and crop (Lloyd et al., 1986; Stoffolano, 2019) that despite suffering structural changes “survive” metamorphosis (Chapman, 1998). We note, that A. ludens adults have a very different diet when compared to larvae and that when they emerge from the puparia after metamorphosis, they need to quickly find nutrients for survival. Perhaps the larval microbiota that persists in the adult gut could help newly emerged flies extract the first critical nutrients from the various feeding substrates commonly used [honeydew, bird feces, rotting pulp, or liquids oozing from decomposing fruit (Aluja et al., 2000)], while they acquire the “adult microbiota” (Drew et al., 1983; Drew and Yuval, 2000; Morimoto et al., 2019). For adults, protein is necessary to reach sexual maturity and develop healthy ovaries in females and testes in males (Aluja et al., 2001). All this needs to be studied in the future.
In a previous study, it was reported that the gut microbiota of A. ludens adults is more diverse than the microbiota of gut larvae (Ventura et al., 2018) which contrasts with our findings here. This inconsistency could be due to the method used to sample adults. In our case, we used newly emerged flies because we wanted to know if flies emerged with a core microbiota. In the case of Ventura et al. (2018), they used flies captured in Multilure® traps baited with CeraTrap®, a liquid protein-based attractant where flies were trapped. Fly aspects that could influence microbiota diversity as the age or the life history (e.g., types of food consumed) were unknown; besides, since flies were immersed in the liquid bait, they could have acquired an unnatural load of microorganisms from it. In fact, this was the reason why we left out adults collected in the field in the plants we sampled as they are highly mobile and therefore could have picked up bacteria from feeding [rotten fruit (likely containing yeasts, fungi, and bacteria), bird feces, honey dew, protein baits used in traps] and mating/resting sites (leaves) that had little to do with metabolizing the pulp ingested by larvae inside fruit or aiding in the process. In future studies such as the one by Ventura et al. (2018), more attention must be paid to possible sources of off-target microorganisms to avoid being misled.
Finally, one of our goals here was to determine if there was a “core microbiota” in the larvae of A. ludens infesting various hosts. In this sense, the comparison between the two ancestral hosts, C. edulis and C. greggii (both Rutaceae) was highly pertinent. The composition of the microbiota of larvae developing in these two hosts was remarkably different, with little overlap, except for bacteria within the Acetobacteraceae and Enterobacteriaceae. As noted, before, with few exceptions, the geographical distribution of these two ancestral hosts does not overlap. So, as we found that the gut of larvae principally harbors bacteria accrued from the pulp they fed on, possibly over evolutionary time, these two host plants developed significantly different associations with bacteria, likely related to fitness advantages to the plant, not the fruit fly larvae developing in them. But broadly speaking, and as clearly depicted in Figure 8, we were unable to find an important core microbiota in neither larvae nor adults stemming from six different hosts encountered in nature. In fact, the estimated core microbiota of larvae is equivalent to that of the microbiota associated to the pulp. However, these results do not imply that the bacterial groups found as constituents of the core microbiota are not important. In fact, it is likely that these taxa represent preponderant players for the larval development of A. ludens. It is noteworthy that most of the very small core microbiota estimated for larval gut samples did not share elements with the estimated core microbiota of adult flies, with exception of the bacteria that belong to the Enterobacteriaceae. However, even in this case, the ubiquity of the members of Enterobacteriaceae could explain these results. Additional studies are needed to confirm if the identified bacterial species in adults stem from previous development stages (i.e., larvae or pupae) or are derived from the environment via feeding activities by newly emerged adults.
Conclusion
We found that the gut microbiota of A. ludens is unstable and that its composition and richness depend on the microbiota associated with the particular plant the larvae of this highly polyphagous species fed on. The pulp of each host plant harbors a specific microbiota, which may affect the compatibility of A. ludens with a particular fruit, as is the case of the occasional host C. pubescens, in which we found a significant presence of Providencia and Morganella, two genera of bacteria that are known to be pathogenic for insects. In this sense, we failed in our prediction that A. ludens larvae would harbor a core microbiota providing flies with a broad community of bacteria useful in metabolizing undigestible or toxic chemicals in the fruit pulp. However, the apparent lack of a stable microbiota in the larval stage in A. ludens paves the way to researching which biochemical mechanisms and metabolic pathways A. ludens larvae use to degrade toxic chemicals in the pulp or supplement deficient larval diets in the field. Finally, and more broadly (i.e., beyond tephritid flies), our findings add new perspectives on the role that metamorphosis plays in shaping the gut microbiota present in insect adults.
Data Availability Statement
The datasets presented in this study can be found in online repositories. The names of the repository/repositories and accession number(s) can be found in the article/Supplementary Material.
Author Contributions
MA, LG, and AM: experimental design. AA-M: sample collection. AA-M and AA-S: experimental procedures. JAZ-B and MA: writing. JAZ-B, VP-B, DD, and MV-R-L: data analysis. JAZ-B: formal analysis. JAZ-B, MA, LG, AA-M, AM, VP-B, DD, MV-R-L, and EI-L: editing. MA and AM: supervising and founding resources. All authors contributed to the article and approved the submitted version.
Funding
This study was principally financed with resources from the Mexican Ministry of Agriculture (SADER), Dirección del Programa Nacional de Moscas de la Fruta (DGSV – SENASICA) via the National Consultative Phytosanitary Council (CONACOFI) through projects 41011–2017, 41012–2018, 41013–2019, 80124–2020, and 80147–2021 awarded to MA. Additional funds were provided by the Asociación de Productores y Empacadores Exportadores de Aguacate de México (APEAM), Project 41010 to MA, the Universidad de Valencia via a Distinguished Professor Fellowship to MA (UV-INV-EPC17-548793), the Consejo Nacional de Ciencia y Tecnología (CONACyT)–Gobierno del Estado de Veracruz FOMIX grant (Project VER-2017-01-292397) to MA, and by the Instituto de Ecología, AC–INECOL. This project was also financed by Generalitat Valenciana (Valencia, Spain), Prometeo/2018/A/133 grant to AM.
Conflict of Interest
The authors declare that the research was conducted in the absence of any commercial or financial relationships that could be construed as a potential conflict of interest.
Publisher’s Note
All claims expressed in this article are solely those of the authors and do not necessarily represent those of their affiliated organizations, or those of the publisher, the editors and the reviewers. Any product that may be evaluated in this article, or claim that may be made by its manufacturer, is not guaranteed or endorsed by the publisher.
Acknowledgments
We acknowledge the seminal contributions to this manuscript by the editor and the two referees who critically evaluated an earlier version. In particular, reviewer AR, made many useful suggestions for improvement, suggested a different overall conceptual framing, and shared valuable insights that helped us produce a better-quality manuscript. Some of her suggestions were incorporated ad verbatim and we therefore gratefully acknowledge her generosity and professionalism. We also gratefully acknowledge the technical support of Emilio Acosta (field collections), Erick Enciso and Olinda Velázquez (fruit fly dissections and larval/adult gut recoveries), Juan Carlos Conde (DNA extraction and amplicon processing), and Emmanuel Villafán (managing computer center). We also thank Manuel Ochoa Sánchez and Essicka A. García Saldaña for helping with literature searches.
Supplementary Material
The Supplementary Material for this article can be found online at: https://www.frontiersin.org/articles/10.3389/fmicb.2021.685937/full#supplementary-material
Supplementary Figure 1 | Rarefaction curves for each host fruit considering microbiota in fruit pulp, and larval and adult (female and male) guts.
Supplementary Figure 2 | Alpha diversity in the microbiota identified in each host fruit (considering the fruit pulp, and Anastrepha ludens larvae and adults) estimated with the Shannon index. Upper and lower whiskers, 3rd, and 1st quartiles (top and bottom of the box), and median (horizontal line within the box) are displayed. Asterisks indicate significant differences between the pairs connected by the horizontal line. For paired t-test, *, **, *** represent p < 0.05, 0.01, and 0.001, respectively.
Supplementary Figure 3 | Linear discriminant analysis (LDA) effect size (LEfSe) results showing the significant results (horizontal bars) and the differential taxa for larvae gut microbiota among host plants. Each bar represents the enriched bacterial taxa in the larvae microbiota from the corresponding host plant when it is compared with other host plants. F, family; g, genera. Only taxa with at least family annotation were plotted.
Supplementary Table 1 | Details on A. ludens’s hosts used in study as well as on collection sites in different states of Mexico.
Supplementary Table 2 | Number of raw sequences per sample, after filtering, merging, and removing chimeras.
Supplementary Table 3 | Abundance of ASVs and their annotation detected across the libraries. We only report taxa with >1% relative abundance across the dataset.
Footnotes
- ^ https://github.com/hrbrmstr/ggalt
- ^ https://CRAN.R-project.org/package=microbial
- ^ http://www.R-project.org/
References
Akami, M., Andongma, A. A., Zhengzhong, C., Nan, J., Khaeso, K., Jurkevitch, E., et al. (2019). Intestinal bacteria modulate the foraging behavior of the oriental fruit fly Bactrocera dorsalis (Diptera: Tephritidae). PloS One 14, e0210109. doi: 10.1371/journal.pone.0210109
Akman, L., Yamashita, A., Watanabe, H., Oshima, K., Shiba, T., Hattori, M., et al. (2002). Genome sequence of the endocellular obligate symbiont of tsetse flies, Wigglesworthia glossinidia. Nat. Genet. 32, 402–407. doi: 10.1038/ng986
Allen, T. C., Pinckard, J. A., and Riker, A. J. (1934). Frequent association of Phytomonus melophthora with various stages in the life cycle of the apple maggot, Rhagoletis pomonella. Phytopathology 24, 226–237.
Aluja, M., Díaz-Fleischer, F., Papaj, D. R., Lagunes, G., and Sivinski, J. (2001). Effects of age, diet, female density and the host resource on egg load in Anastrepha ludens and Anastrepha obliqua (Diptera: Tephritidae). J. Insect Physiol. 47, 975–988. doi: 10.1016/s0022-1910(01)00072-5
Aluja, M., and Mangan, R. L. (2008). Fruit fly (Diptera: Tephritidae) host status determination: critical conceptual, methodological, and regulatory considerations. Annu. Rev. Entomol. 53, 473–502. doi: 10.1146/annurev.ento.53.103106.093350
Aluja, M., Piñero, J., Jácome, I., Díaz-Fleischer, F., and Sivinski, J. (2000). “Behavior of flies in the genus Anastrepha (Trypetinae: Toxotrypanini),” in Fruit Flies (Tephritidae): Phylogeny and Evolution of Behavior, eds M. Aluja and A. Norrbom (Boca Raton, FL: CRC Press), 944.
Andongma, A. A., Wan, L., Dong, Y. C., Li, P., Desneux, N., White, J. A., et al. (2015). Pyrosequencing reveals a shift in symbiotic bacteria populations across life stages of Bactrocera dorsalis. Sci. Rep. 5:9470. doi: 10.1038/srep09470
Andongma, A. A., Wan, L., Dong, Y. C., Wang, Y. L., He, J., and Niu, C. Y. (2019). Assessment of the bacteria community structure across life stages of the chinese citrus fly, Bactrocera minax (Diptera: Tephritidae). BMC Microbiol. 19(Suppl. 1):285. doi: 10.1186/s12866-019-1646-9
Ayayee, P. A., Ondrejech, A., Keeney, G., and Munõz-García, A. (2018). The role of gut microbiota in the regulation of standard metabolic rate in female Periplaneta americana. Peer J. 6:e4717. doi: 10.7717/peerj.4717
Ayyasamy, A., Kempraj, V., and Pagadala Damodaram, K. J. (2021). Endosymbiotic bacteria aid to overcome temperature induced stress in the oriental fruit fly, Bactrocera dorsalis. Microb. Ecol. doi: 10.1007/s00248-021-01682-2 [Epub ahead of print].
Bauer, E., Kaltenpoth, M., and Salem, H. (2020). Minimal fermentative metabolism fuels extracellular symbiont in a leaf beetle. ISME J. 14, 866–870. doi: 10.1038/s41396-019-0562-1
Baumann, L., Thao, M. L. L., Hess, J. M., Johnson, M. W., and Baumann, P. (2002). The genetic properties of the primary endosymbionts of mealybugs differ from those of other endosymbionts of plant sap-sucking insects. Appl. Environ. Microbiol. 68, 3198–3205. doi: 10.1128/AEM.68.7.3198-3205.2002
Baumann, P. (2005). Biology of bacteriocyte-associated endosymbionts of plant sap-sucking insects. Annu. Rev. Microbiol. 59, 155–189. doi: 10.1146/annurev.micro.59.030804.121041
Behar, A., Jurkevitch, E., and Yuval, B. (2008). Bringing back the fruit into fruit fly-bacteria interactions. Mol. Ecol. 17, 1375–1386. doi: 10.1111/j.1365-294X.2008.03674.x
Ben-Yosef, M., Pasternak, Z., Jurkevitch, E., and Yuval, B. (2015). Symbiotic bacteria enable olive fly larvae to overcome host defences. R. Soc. Open Sci. 2:150170. doi: 10.1098/rsos.150170
Birke, A., and Aluja, M. (2018). Do mothers really know best? Complexities in testing the preference-performance hypothesis in polyphagous frugivorous fruit flies. Bull. Entomol. Res. 108, 674–684. doi: 10.1017/S0007485317001213
Brummel, T., Ching, A., Seroude, L., Simon, A. F., and Benzer, S. (2004). Drosophila lifespan enhancement by exogenous bacteria. Proc. Natl. Acad. Sci. U.S.A. 101, 12974–12979. doi: 10.1073/pnas.0405207101
Callahan, B. J., McMurdie, P. J., Rosen, M. J., Han, A. W., Johnson, A. J. A., and Holmes, S. P. (2016). DADA2: high-resolution sample inference from Illumina amplicon data. Nat. Methods 13, 581–583. doi: 10.1038/nmeth.3869
Capuzzo, C., Firrao, G., Mazzon, L., Squartini, A., and Girolami, V. (2005). “Candidatus Erwinia dacicola”, a coevolved symbiotic bacterium of the olive fly Bactrocera oleae (Gmelin). Int. J. Syst. Evol. Microbiol. 55, 1641–1647. doi: 10.1099/ijs.0.63653-0
Ceja-Navarro, J. A., Vega, F. E., Karaoz, U., Hao, Z., Jenkins, S., Lim, H. C., et al. (2015). Gut microbiota mediate caffeine detoxification in the primary insect pest of coffee. Nat. Commun. 6:7618. doi: 10.1038/ncomms8618
Cheng, D., Guo, Z., Riegler, M., Xi, Z., Liang, G., and Xu, Y. (2017). Gut symbiont enhances insecticide resistance in a significant pest, the oriental fruit fly Bactrocera dorsalis (Hendel). Microbiome 5:13. doi: 10.1186/s40168-017-0236-z
Chieffi, D., Fanelli, F., and Fusco, V. (2020). Arcobacter butzleri: up-to-date taxonomy, ecology, and pathogenicity of an emerging pathogen. Compr. Rev. Food Sci. Food Saf. 19, 2071–2109. doi: 10.1111/1541-4337.12577
Coon, K. L., Brown, M. R., and Strand, M. R. (2016). Mosquitoes host communities of bacteria that are essential for development but vary greatly between local habitats. Mol. Ecol. 25, 5806–5826. doi: 10.1111/mec.13877
Crotti, E., Rizzi, A., Chouaia, B., Ricci, I., Favia, G., Alma, A., et al. (2010). Acetic acid bacteria, newly emerging symbionts of insects. Appl. Environ. Microbiol. 76, 6963–6970. doi: 10.1128/AEM.01336-10
Damodaram, K. J., Ayyasamy, A., and Kempraj, V. (2016). Commensal bacteria aid mate-selection in the fruit fly, Bactrocera dorsalis. Microb. Ecol. 72, 725–729. doi: 10.1007/s00248-016-0819-4
Deb, R., Nair, A., and Agashe, D. (2019). Host dietary specialization and neutral assembly shape gut bacterial communities of wild dragonflies. Peer J. 7:e8058. doi: 10.7717/peerj.8058
Dees, M. W., Lysøe, E., Nordskog, B., and Brurberg, M. B. (2015). Bacterial communities associated with surfaces of leafy greens: shift in composition and decrease in richness over time. Appl. Environ. Microbiol. 81, 1530–1539. doi: 10.1128/AEM.03470-14
Derrien, M., and van Hylckama Vlieg, J. E. T. (2015). Fate, activity, and impact of ingested bacteria within the human gut microbiota. Trends Microbiol. 23, 354–366. doi: 10.1016/j.tim.2015.03.002
Després, L., David, J. P., and Gallet, C. (2007). The evolutionary ecology of insect resistance to plant chemicals. Trends Ecol. Evol. 22, 298–307. doi: 10.1016/j.tree.2007.02.010
Deutscher, A. T., Reynolds, O. L., and Chapman, T. A. (2017). Yeast: an overlooked component of Bactrocera tryoni (Diptera: Tephritidae) larval gut microbiota. J. Econ. Entomol. 110, 298–300. doi: 10.1093/jee/tow262
Dillon, R. J., and Dillon, V. M. (2004). The gut bacteria of insects: nonpathogenic interactions. Annu. Rev. Entomol. 49, 71–92. doi: 10.1146/annurev.ento.49.061802.123416
Douglas, A. E. (2009). The microbial dimension in insect nutritional ecology. Funct. Ecol. 12:e0170332. doi: 10.1111/j.1365-2435.2008.01442.x
Douglas, A. E. (2018). Drosophila and its gut microbes: a model for drug-microbiome interactions. Drug Discov. Today Dis. Model. 28, 43–49. doi: 10.1016/j.ddmod.2019.08.004
Dowd, P. F. (1989). In Situ production of hydrolytic detoxifying enzymes by symbiotic yeasts in the cigarette beetle (Coleoptera: Anobiidae). J. Econ. Entomol. 28, 43–49. doi: 10.1093/jee/82.2.396
Drew, R. A. I., Courtice, A. C., and Teakle, D. S. (1983). Bacteria as a natural source of food for adult fruit flies (Diptera: Tephritidae). Oecologia 60, 279–284. doi: 10.1007/bf00376839
Drew, R. A. I., and Yuval, B. (2000). “The evolution of fruit fly feeding behavior,” in Fruit Flies (Tephritidae): Phylogeny and Evolution of Behavior, eds M. Aluja and A. Norrbom (Boca Raton, FL: CRC Press), 944.
Engel, P., and Moran, N. A. (2013). The gut microbiota of insects - diversity in structure and function. FEMS Microbiol. Rev. 37, 699–735. doi: 10.1111/1574-6976.12025
Erkosar, B., and Leulier, F. (2014). Transient adult microbiota, gut homeostasis and longevity: novel insights from the Drosophila model. FEBS Lett. 588, 4250–4257. doi: 10.1016/j.febslet.2014.06.041
Estes, A. M., Hearn, D. J., Bronstein, J. L., and Pierson, E. A. (2009). The olive fly endosymbiont, Candidatus Erwinia dacicola, switches from an intracellular existence to an extracellular existence during host insect development. Appl. Environ. Microbiol. 75, 7097–7106. doi: 10.1128/AEM.00778-09
Estes, A. M., Segura, D. F., Jessup, A., Wornoayporn, V., and Pierson, E. A. (2014). Effect of the symbiont Candidatus Erwinia dacicola on mating success of the olive fly Bactrocera oleae (Diptera: Tephritidae). Int. J. Trop. Insect Sci. 34, S123–S131. doi: 10.1017/S1742758414000174
Fitt, G. P., and O’Brien, R. W. (1985). Bacteria associated with four species of Dacus (Diptera: Tephritidae) and their role in the nutrition of the larvae. Oecologia 67, 447–454. doi: 10.1007/BF00384954
Frago, E., Dicke, M., and Godfray, H. C. J. (2012). Insect symbionts as hidden players in insect-plant interactions. Trends Ecol. Evol. 27, 705–711. doi: 10.1016/j.tree.2012.08.013
Fukui, T., Kawamoto, M., Shoji, K., Kiuchi, T., Sugano, S., Shimada, T., et al. (2015). The endosymbiotic bacterium Wolbachia selectively kills male hosts by targeting the masculinizing gene. PLoS Pathog. 11:e1005048. doi: 10.1371/journal.ppat.1005048
Galac, M. R., and Lazzaro, B. P. (2011). Comparative pathology of bacteria in the genus Providencia to a natural host, Drosophila melanogaster. Microbes Infect. 13, 673–683. doi: 10.1016/j.micinf.2011.02.005
Gallo-Franco, J. J., and Toro-Perea, N. (2020). Variations in the bacterial communities in Anastrepha obliqua (Diptera: Tephritidae) according to the insect life stage and host plant. Curr. Microbiol. 77, 1283–1291. doi: 10.1007/s00284-020-01939-y
Gevers, D., Knight, R., Petrosino, J. F., Huang, K., McGuire, A. L., Birren, B. W., et al. (2012). The Human Microbiome project: a community resource for the healthy human microbiome. PLoS Biol. 10:e1001377. doi: 10.1371/journal.pbio.1001377
Gilbert, J. A., Jansson, J. K., and Knight, R. (2014). The Earth microbiome project: successes and aspirations. BMC Biol. 12:69. doi: 10.1186/s12915-014-0069-1
Ginestet, C. (2011). ggplot2: elegant graphics for data analysis. J. R. Stat. Soc. Ser. A 174, 245–246. doi: 10.1111/j.1467-985x.2010.00676_9.x
Glasgow, H. (1914). The gastric caeca and the caecal bacteria of Heteroptera. Biol. Bull. 26, 101–172. doi: 10.5962/bhl.title.70904
Grimaldi, D., and Engel, M. S. (2005). Evolution of Insects. Cambridge: Cambridge Univ. Press, 1–16.
Guillén, L., Pascacio-Villafán, C., Stoffolano, J. G. Jr., López-Sánchez, L., Velázquez, O., Rosas-Saito, G., et al. (2019). Structural differences in the digestive tract between females and males could modulate regurgitation behavior in Anastrepha ludens (Diptera: Tephritidae). J. Insect Behav. 19:7. doi: 10.1093/jisesa/iez070
Guizzo, M. G., Neupane, S., Kucera, M., Perner, J., Frantová, H., da Silva Vaz, I. J., et al. (2020). Poor unstable midgut microbiome of hard ticks contrasts with abundant and stable monospecific microbiome in ovaries. Front. Cell. Infect. Microbiol 10:211. doi: 10.3389/fcimb.2020.00211
Gupta, A., and Nair, S. (2020). Dynamics of insect–microbiome interaction influence host and microbial symbiont. Front. Microbiol. 11:1357. doi: 10.3389/fmicb.2020.01357
Guyomar, C., Legeai, F., Jousselin, E., Mougel, C., Lemaitre, C., and Simon, J. C. (2018). Multi-scale characterization of symbiont diversity in the pea aphid complex through metagenomic approaches. Microbiome 66:181. doi: 10.1186/s40168-018-0562-9
Guzman, J., and Vilcinskas, A. (2020). Bacteria associated with cockroaches: health risk or biotechnological opportunity? Appl. Microbiol. Biotechnol. 104, 10369–10387. doi: 10.1007/s00253-020-10973-6
Hadapad, A. B., Shettigar, S. K. G., and Hire, R. S. (2019). Bacterial communities in the gut of wild and mass-reared Zeugodacus cucurbitae and Bactrocera dorsalis revealed by metagenomic sequencing. BMC Microbiol. 19:282. doi: 10.1186/s12866-019-1647-8
Hagen, K. S. (1966). Dependence of the olive fly, Dacus oleae, larvae on symbiosis with Pseudomonas savastanoi for the utilization of olive. Nature 209, 423–424. doi: 10.1038/209423a0
Hammer, T. J., and Bowers, M. D. (2015). Gut microbes may facilitate insect herbivory of chemically defended plants. Oecologia 179, 1–14. doi: 10.1007/s00442-015-3327-1
Hammer, T. J., Janzen, D. H., Hallwachs, W., Jaffe, S. P., and Fierer, N. (2017). Caterpillars lack a resident gut microbiome. Proc. Natl. Acad. Sci. U.S.A. 114, 9641–9646. doi: 10.1073/pnas.1707186114
Hammer, T. J., McMillan, W. O., and Fierer, N. (2014). Metamorphosis of a butterfly-associated bacterial community. PLoS One 9:e86995. doi: 10.1371/journal.pone.0086995
Hammer, T. J., Sanders, J. G., and Fierer, N. (2019). Not all animals need a microbiome. FEMS Microbiol. Lett. 366:fnz117. doi: 10.1093/femsle/fnz117
Hansen, A. K., and Moran, N. A. (2014). The impact of microbial symbionts on host plant utilization by herbivorous insects. Mol. Ecol. 23, 1473–1496. doi: 10.1111/mec.12421
Heys, C., Lizé, A., Blow, F., White, L., Darby, A., and Lewis, Z. J. (2018). The effect of gut microbiota elimination in Drosophila melanogaster: a how-to guide for host–microbiota studies. Ecol. Evol. 8, 4150–4161. doi: 10.1002/ece3.3991
Huang, H., Li, H., Ren, L., and Cheng, D. (2019). Microbial communities in different developmental stages of the oriental fruit fly, Bactrocera dorsalis, are associated with differentially expressed peptidoglycan recognition protein-encoding genes. Appl. Environ. Microbiol. 85:e00803-19. doi: 10.1128/AEM.00803-19
Janson, E. M., Stireman, J. O., Singer, M. S., and Abbot, P. (2008). Phytophagous insect-microbe mutualisms and adaptive evolutionary diversification. Evolution 62, 997–1012. doi: 10.1111/j.1558-5646.2008.00348.x
Jiggins, F. M. (2016). Open questions: How does Wolbachia do what it does? BMC Biol. 14:92. doi: 10.1186/s12915-016-0312-z
Jing, T. Z., Qi, F. H., and Wang, Z. Y. (2020). Most dominant roles of insect gut bacteria: Digestion, detoxification, or essential nutrient provision? Microbiome 8:38. doi: 10.1186/s40168-020-00823-y
Johnston, P. R., Paris, V., and Rolff, J. (2019). Immune gene regulation in the gut during metamorphosis in a holo- versus a hemimetabolous insect. Philos. Trans. R. Soc. B 374:20190073. doi: 10.1098/rstb.2019.0073
Johnston, P. R., and Rolff, J. (2015). Host and symbiont jointly control gut microbiota during complete metamorphosis. PLoS Pathog. 11:e1005246. doi: 10.1371/journal.ppat.1005246
Jose, P. A., Ben-Yosef, M., Jurkevitch, E., and Yuval, B. (2019). Symbiotic bacteria affect oviposition behavior in the olive fruit fly Bactrocera oleae. J. Insect Physiol. 117:103917. doi: 10.1016/j.jinsphys.2019.103917
Kageyama, D., Narita, S., and Watanabe, M. (2012). Insect sex determination manipulated by their endosymbionts: incidences, mechanisms and implications. Insects 3, 161–199. doi: 10.3390/insects3010161
Kahle, D., and Wickham, H. (2013). ggmap: spatial visualization with ggplot2. R J. 5, 144–161. doi: 10.32614/rj-2013-014
Kakumanu, M. L., Maritz, J. M., Carlton, J. M., and Schal, C. (2018). Overlapping community compositions of gut and fecal microbiomes in lab-reared and field-collected German cockroaches. Appl. Environ. Microbiol. 84:e01037-18. doi: 10.1128/AEM.01037-18
Ke, J., An, Y., Cao, B., Lang, J., Wu, N., and Zhao, D. (2020). Orlistat-induced gut microbiota modification in obese mice. Evid. Based Complement. Altern. Med. 2020:9818349. doi: 10.1155/2020/9818349
Kelley, S. T., and Dobler, S. (2011). Comparative analysis of microbial diversity in Longitarsus flea beetles (Coleoptera: Chrysomelidae). Genetica 139, 541–550. doi: 10.1007/s10709-010-9498-0
Kikuchi, Y. (2009). Endosymbiotic bacteria in insects: their diversity and culturability. Microbes Environ. 24, 195–204. doi: 10.1264/jsme2.ME09140S
Kikuchi, Y., Hosokawa, T., and Fukatsu, T. (2007). Insect-microbe mutualism without vertical transmission: a stinkbug acquires a beneficial gut symbiont from the environment every generation. Appl. Environ. Microbiol. 73, 4308–4316. doi: 10.1128/AEM.00067-07
Killiny, N., Hijaz, F., Ebert, T. A., and Rogers, M. E. (2017). A plant bacterial pathogen manipulates its insect vector’s energy metabolism. Appl. Environ. Microbiol. 83:e03005-16. doi: 10.1128/AEM.03005-16
Kingsley, V. V. (1972). Persistence of intestinal bacteria in the developmental stages of the monarch butterfly (Danaus plexippus). J. Invertebr. Pathol. 20, 51–58. doi: 10.1016/0022-2011(72)90081-x
Klindworth, A., Pruesse, E., Schweer, T., Peplies, J., Quast, C., Horn, M., et al. (2013). Evaluation of general 16S ribosomal RNA gene PCR primers for classical and next-generation sequencing-based diversity studies. Nucleic. Acids Res. 41:e1. doi: 10.1093/nar/gks808
Kõiv, V., Arbo, K., Maiväli, Ü., Kisand, V., Roosaare, M., Remm, M., et al. (2019). Endophytic bacterial communities in peels and pulp of five root vegetables. PLoS One 14:e0210542. doi: 10.1371/journal.pone.0210542
Kounatidis, I., Crotti, E., Sapountzis, P., Sacchi, L., Rizzi, A., Chouaia, B., et al. (2009). Acetobacter tropicalis is a major symbiont of the olive fruit fly (Bactrocera oleae). Appl. Environ. Microbiol. 75, 3281–3288. doi: 10.1128/AEM.02933-08
Kuzina, L. V., Peloquin, J. J., Vacek, D. C., and Miller, T. A. (2001). Isolation and identification of bacteria associated with adult laboratory Mexican fruit flies, Anastrepha ludens (Diptera: Tephritidae). Curr. Microbiol. 42, 290–294. doi: 10.1007/s002840110219
Lemoine, M. M., Engl, T., and Kaltenpoth, M. (2020). Microbial symbionts expanding or constraining abiotic niche space in insects. Curr. Opin. Insect Sci. 39, 14–20. doi: 10.1016/j.cois.2020.01.003
Leo, L., and Sudarshan, S. (2017). Tools for Microbiome Analysis in R, Version 1.5.28. Available online at: https://microsud.github.io/microbiomeutilities/ (accessed December, 2020).
Liu, S. H., Chen, Y., Li, W., Tang, G. H., Yang, Y., Jiang, H. B., et al. (2018). Diversity of bacterial communities in the intestinal tracts of two geographically distant populations of Bactrocera dorsalis (Diptera: Tephritidae). J. Econ. Entomol. 111, 2861–2868. doi: 10.1093/jee/toy231
Lloyd, A. C., Drew, R. A. I., Teakle, D. S., and Hayward, A. C. (1986). Bacteria associated with some Dacus species (Diptera: Tephritidae) and their host fruit in Queensland. Aust. J. BioI. Sci. 39, 361–368. doi: 10.1071/bi9860361
Ludington, W. B., and Ja, W. W. (2020). Drosophila as a model for the gut microbiome. PLoS Pathog. 16:e1008398. doi: 10.1371/journal.ppat.1008398
Ma, D., and Leulier, F. (2018). The importance of being persistent: the first true resident gut symbiont in Drosophila. PLoS Biol. 16:e2006945. doi: 10.1371/journal.pbio.2006945
Majumder, P., Sutcliffe, B., Taylor, P. W., and Chapman, T. A. (2019). Next-Generation Sequencing reveals relationship between the larval microbiome and food substrate in the polyphagous Queensland fruit fly. Sci. Rep. 9:14292. doi: 10.1038/s41598-019-50602-5
Majumder, R., Sutcliffe, B., Taylor, P. W., and Chapman, T. A. (2020a). Fruit host-dependent fungal communities in the microbiome of wild Queensland fruit fly larvae. Sci. Rep. 10:16550. doi: 10.1038/s41598-020-73649-1
Majumder, R., Sutcliffe, B., Taylor, P. W., and Chapman, T. A. (2020b). Microbiome of the Queensland fruit fly through metamorphosis. Microorganisms 8:795. doi: 10.3390/microorganisms8060795
Malacrinò, A., Campolo, O., Medina, R. F., and Palmeri, V. (2018). Instar- and host-associated differentiation of bacterial communities in the Mediterranean fruit fly Ceratitis capitata. PLoS One 13:e0194131. doi: 10.1371/journal.pone.0194131
Malacrinò, A., Schena, L., Campolo, O., Laudani, F., Mosca, S., Giunti, G., et al. (2017). A metabarcoding survey on the fungal microbiota associated to the olive fruit fly. Microb. Ecol. 73, 677–684. doi: 10.1007/s00248-016-0864-z
Martinson, V. G., Douglas, A. E., and Jaenike, J. (2017). Community structure of the gut microbiota in sympatric species of wild Drosophila. Ecol. Lett. 20, 629–639. doi: 10.1111/ele.12761
Mazzini, M., and Vita, G. (1981). Submicroscopic identification of the transmission mechanism of the symbiotic bacteria in Dacus oleae (Gmelin) (Diptera, Trypetidae). Redia G. Zool. 64, 277–301.
McLean, A. H. C., Godfray, H. C. J., Ellers, J., and Henry, L. M. (2019). Host relatedness influences the composition of aphid microbiomes. Environ. Microbiol. Rep. 11, 808–816. doi: 10.1111/1758-2229.12795
McMurdie, P. J., and Holmes, S. (2013). Phyloseq: an R package for reproducible interactive analysis and graphics of microbiome census data. PLoS One 8:e61217. doi: 10.1371/journal.pone.0061217
Miyazaki, S., Mallory Boush, G., and Baerwald, R. J. (1968). Amino acid synthesis by Pseudomonas melophthora, bacterial symbiote of Rhagoletis pomonella (Diptera). J. Insect Physiol. 14, 513–518. doi: 10.1016/0022-1910(68)90066-8
Moreau, C. S., and Rubin, B. E. R. (2017). Diversity and persistence of the gut microbiome of the giant neotropical bullet ant. Integr. Comp. Biol. 57, 682–689. doi: 10.1093/icb/icx037
Morimoto, J., Nguyen, B., Tabrizi, S. T., Lundbäck, I., Taylor, P. W., Ponton, F., et al. (2019). Commensal microbiota modulates larval foraging behaviour, development rate and pupal production in Bactrocera tryoni. BMC Microbiol. 19:286. doi: 10.1186/s12866-019-1648-7
Msaad-Guerfali, M., Djobbi, W., Charaabi, K., Hamden, H., Fadhl, S., Marzouki, W., et al. (2018). Evaluation of Providencia rettgeri pathogenicity against laboratory Mediterranean fruit fly strain (Ceratitis capitata). PLoS One 13:e0196343. doi: 10.1371/journal.pone.0196343
Muñoz-Benavent, M., Pérez-Cobas, A. E., García-Ferris, C., Moya, A., and Latorre, A. (2020). Insects’ potential: understanding the functional role of their gut microbiome. J. Pharm. Biomed. Anal. 194:113787. doi: 10.1016/j.jpba.2020.113787
Naaz, N., Choudhary, J. S., Choudhary, A., Dutta, A., and Das, B. (2020). Developmental stage-associated microbiota profile of the peach fruit fly, Bactrocera zonata (Diptera: Tephritidae) and their functional prediction using 16S rRNA gene metabarcoding sequencing. 3 Biotech 10:390. doi: 10.1007/s13205-020-02381-4
Nikolouli, K., Augustinos, A. A., Stathopoulou, P., Asimakis, E., Mintzas, A., Bourtzis, K., et al. (2020). Genetic structure and symbiotic profile of worldwide natural populations of the Mediterranean fruit fly, Ceratitis capitata. BMC Genet. 21(Suppl. 2):128. doi: 10.1186/s12863-020-00946-z
Nobles, S., and Jackson, C. R. (2020). Effects of life stage, site, and species on the dragonfly gut microbiome. Microorganisms 8:183. doi: 10.3390/microorganisms8020183
Noman, M. S., Shi, G., Liu, L. J., and Li, Z. H. (2021). Diversity of bacteria in different life stages and their impact on the development and reproduction of Zeugodacus tau (Diptera: Tephritidae). Insect Sci. 28, 363–376. doi: 10.1111/1744-7917.12768
Norrbom, A. L., Zucchi, R. A., and Hernández-Ortiz, V. (2000). “Phylogeny of the genera Anastrepha and Toxotrypana (trypetinae: Toxotrypanini) based on morphology,” in Fruit Flies (Tephritidae): Phylogeny and Evolution of Behavior, eds M. Aluja and A. Norrbom (Boca Raton, FL: CRC Press), 944.
Oksanen, J., Kindt, R., Legendre, P., O’Hara, B., Simpson, G. L., Solymos, P. M., et al. (2008). The vegan Package. Available online at: https://cran.r-project.org/web/packages/vegan/index.html (accessed December, 2020).
Pais, I. S., Valente, R. S., Sporniak, M., and Teixeira, L. (2018). Drosophila melanogaster establishes a species-specific mutualistic interaction with stable gut-colonizing bacteria. PLoS Biol. 16:e2005710. doi: 10.1371/journal.pbio.2005710
Pérez-Cobas, A. E., Maiques, E., Angelova, A., Carrasco, P., Moya, A., and Latorre, A. (2015). Diet shapes the gut microbiota of the omnivorous cockroach Blattella germanica. FEMS Microbiol. Ecol. 91:fiv022. doi: 10.1093/femsec/fiv022
Pernice, M., Simpson, S. J., and Ponton, F. (2014). Towards an integrated understanding of gut microbiota using insects as model systems. J. Insect Physiol. 69, 12–18. doi: 10.1016/j.jinsphys.2014.05.016
Petri, L. (1904). Sopra la particolare localizzazione di una colonia batterica nel tubo digerente della larva della mosca olearia. Atti della R. Accad. dei Lincei Rendiconti V. ser. Classe sci. fis. mat. nat. 13:560.
Petri, L. (1909). Ricerche Sopra i Batteri Intestinali della Mosca Olearia. (Roma: Memorie della Regia Stazione di Patologia Vegetale di Roma), 1–130.
Petri, L. (1910). Untersuchungen über die Darmbakterien der Olivenfliege. Zentralbl. Bacteriol. Parasit. Infect. Hyg. 26, 357–367.
Phalnikar, K., Kunte, K., and Agashe, D. (2019). Disrupting butterfly caterpillar microbiomes does not impact their survival and development. Proc. R. Soc. B Biol. Sci. 286:20192438. doi: 10.1098/rspb.2019.2438
Piper, A. M., Farnier, K., Linder, T., Speight, R., and Cunningham, J. P. (2017). Two gut-associated yeasts in a Tephritid fruit fly have contrasting effects on adult attraction and larval survival. J. Chem. Ecol. 43, 891–901. doi: 10.1007/s10886-017-0877-1
Quast, C., Pruesse, E., Yilmaz, P., Gerken, J., Schweer, T., Yarza, P., et al. (2013). The SILVA ribosomal RNA gene database project: improved data processing and web-based tools. Nucleic Acids Res. 41, D590–D596. doi: 10.1093/nar/gks1219
Raghu, S., Clarke, A. R., and Bradley, J. (2002). Microbial mediation of fruit fly-host plant interactions: Is the host plant the “centre of activity”? Oikos 97, 319–328. doi: 10.1034/j.1600-0706.2002.970302.x
Ravenscraft, A., Berry, M., Hammer, T., Peay, K., and Boggs, C. (2019a). Structure and function of the bacterial and fungal gut microbiota of Neotropical butterflies. Ecol. Monogr. 89:e01346. doi: 10.1002/ecm.1346
Ravenscraft, A., Kish, N., Peay, K., and Boggs, C. (2019b). No evidence that gut microbiota impose a net cost on their butterfly host. Mol. Ecol. 28, 2100–2117. doi: 10.1111/mec.15057
Raza, M. F., Wang, Y., Cai, Z., Bai, S., Yao, Z., Awan, U. A., et al. (2020). Gut microbiota promotes host resistance to low-temperature stress by stimulating its arginine and proline metabolism pathway in adult Bactrocera dorsalis. PLoS Pathog. 16:e1008441. doi: 10.1371/journal.ppat.1008441
Robert, A., Qadri, M., Blair, J., and Wong, A. C. N. (2019). The Microbiome of fruit flies as novel targets for pest management. ACS Symp. Ser. 1334, 1–37. doi: 10.1021/bk-2019-1334.ch001
Ross, B. D., Hayes, B., Radey, M. C., Lee, X., Josek, T., Bjork, J., et al. (2018). Ixodes scapularis does not harbor a stable midgut microbiome. ISME J. 12, 2596–2607. doi: 10.1038/s41396-018-0161-6
Rowland, I., Gibson, G., Heinken, A., Scott, K., Swann, J., Thiele, I., et al. (2018). Gut microbiota functions: metabolism of nutrients and other food components. Eur. J. Nutr. 57, 1–24. doi: 10.1007/s00394-017-1445-8
Russell, V., and Dunn, P. E. (1996). Antibacterial proteins in the midgut of Manduca sexta during metamorphosis. J. Insect. Physiol. 42, 65–71. doi: 10.1016/0022-1910(95)00083-6
Russell, V. W., and Dunn, P. E. (1991). Lysozyme in the midgut of Manduca sexta during metamorphosis. Arch. Insect. Biochem. Physiol. 17, 67–80. doi: 10.1002/arch.940170202
Salas, B., Conway, H. E., Schuenzel, E. L., Hopperstad, K., Vitek, C., and Vacek, D. C. (2017). Morganella morganii (Enterobacteriales: Enterobacteriaceae) is a lethal pathogen of Mexican Fruit Fly (Diptera: Tephritidae) larvae. Fla. Entomol. 100, 743–751. doi: 10.1653/024.100.0422
Sanders, J. G., Lukasik, P., Frederickson, M. E., Russell, J. A., Koga, R., Knight, R., et al. (2017). Dramatic differences in gut bacterial densities correlate with diet and habitat in rainforest ants. Integr. Comp. Biol. 57, 705–722. doi: 10.1093/icb/icx088
Schmidt, K., and Engel, P. (2021). Mechanisms underlying gut microbiota–host interactions in insects. J. Exp. Biol. 224:jeb207696. doi: 10.1242/jeb.207696
Selkrig, J., Mohammad, F., Ng, S. H., Chua, J. Y., Tumkaya, T., Ho, J., et al. (2018). The Drosophila microbiome has a limited influence on sleep, activity, and courtship behaviors. Sci. Rep. 8:10646. doi: 10.1038/s41598-018-28764-5
Sender, R., Fuchs, S., and Milo, R. (2016). Revised estimates for the number of human and bacteria cells in the body. PLoS Biol. 14:e1002533. doi: 10.1371/journal.pbio.1002533
Shah, A. H., Saleha, A. A., Zunita, Z., and Murugaiyah, M. (2011). Arcobacter - An emerging threat to animals and animal origin food products? Trends Food Sci. Technol. 22, 225–236. doi: 10.1016/j.tifs.2011.01.010
Shukla, P. S., and Beran, F. (2020). Gut microbiota degrades toxic isothiocyanates in a flea beetle pest. Mol. Ecol. 29, 4692–4705. doi: 10.1111/mec.15657
Shuttleworth, L. A., Khan, M. A. M., Osborne, T., Collins, D., Srivastava, M., and Reynolds, O. L. (2019). A walk on the wild side: gut bacteria fed to mass-reared larvae of Queensland fruit fly [Bactrocera tryoni (Froggatt)] influence development. BMC Biotechnol. 19:95. doi: 10.1186/s12896-019-0579-6
Sinno, M., Bézier, A., Vinale, F., Giron, D., Laudonia, S., Garonna, A. P., et al. (2020). Symbiosis disruption in the olive fruit fly, Bactrocera oleae (Rossi), as a potential tool for sustainable control. Pest Manag. Sci. 76, 3199–3207. doi: 10.1002/ps.5875
Sommer, F., and Bäckhed, F. (2013). The gut microbiota-masters of host development and physiology. Nat. Rev. Microbiol. 11, 227–238. doi: 10.1038/nrmicro2974
Stammer, H. J. (1929). Die bakteriensymbiose der trypetiden (Diptera). Z. Morphol. Öekol. Tiere 15, 481–523. doi: 10.1007/BF00410561
Stoffolano, J. G. Jr. (2019). Fly foregut and transmission of microbes. Adv. Insect Physiol. 57, 27–95. doi: 10.1016/bs.aiip.2019.10.001
Stoffolano, J. G., and Yin, L. R. S. (1987). Structure and function of the ovipositor and associated sensilla of the apple maggot, Rhagoletis pomonella (Walsh) (Diptera: Tephritidae). Int. J. Insect Morphol. Embryol. 16, 41–69. doi: 10.1016/0020-7322(87)90055-9
Sudakaran, S., Kost, C., and Kaltenpoth, M. (2017). Symbiont acquisition and replacement as a source of ecological innovation. Trends Microbiol. 25, 375–390. doi: 10.1016/j.tim.2017.02.014
Thomas, D. B. (2004). Hot peppers as a host for the Mexican fruit fly Anastrepha ludens (Diptera: Tephritidae). Fla. Entomol. 87, 603–608. doi: 10.1653/0015-4040(2004)087[0603:hpaahf]2.0.co;2
Tinker, K. A., and Ottesen, E. A. (2016). The core gut microbiome of the American cockroach, Periplaneta americana, is stable and resilient to dietary shifts. Appl. Environ. Microbiol. 82, 6603–6610. doi: 10.1128/AEM.01837-16
Tsuchida, T., Koga, R., Horikawa, M., Tsunoda, T., Maoka, T., Matsumoto, S., et al. (2010). Symbiotic bacterium modifies aphid body color. Science 330, 1102–1104. doi: 10.1126/science.1195463
Ventura, C., Briones-Roblero, C. I., Hernández, E., Rivera-Orduña, F. N., and Zúñiga, G. (2018). Comparative analysis of the gut bacterial community of four Anastrepha fruit flies (Diptera: Tephritidae) based on pyrosequencing. Curr. Microbiol. 75, 966–976. doi: 10.1007/s00284-018-1473-5
Waite, D. W., Vanwonterghem, I., Rinke, C., Parks, D. H., Zhang, Y., Takai, K., et al. (2017). Comparative genomic analysis of the class Epsilonproteobacteria and proposed reclassification to epsilon bacteraeota (phyl. nov.). Front. Microbiol. 8:682. doi: 10.3389/fmicb.2017.00682
Wassermann, B., Rybakova, D., Müller, C., and Berg, G. (2017). Harnessing the microbiomes of Brassica vegetables for health issues. Sci. Rep. 7:17649. doi: 10.1038/s41598-017-17949-z
Werren, J. H., Baldo, L., and Clark, M. E. (2008). Wolbachia: master manipulators of invertebrate biology. Nat. Rev. Microbiol. 6, 741–751. doi: 10.1038/nrmicro1969
Wong, A. C. N., Chaston, J. M., and Douglas, A. E. (2013). The inconstant gut microbiota of Drosophila species revealed by 16S rRNA gene analysis. ISME J. 7, 1922–1932. doi: 10.1038/ismej.2013.86
Wong, A. C. N., Ng, P., and Douglas, A. E. (2011). Low-diversity bacterial community in the gut of the fruit fly Drosophila melanogaster: bacterial community in Drosophila melanogaster. Environ. Microbiol. 13, 1889–1900. doi: 10.1111/j.1462-2920.2011.02511.x
Woruba, D. N., Morrow, J. L., Reynolds, O. L., Chapman, T. A., Collins, D. P., and Riegler, M. (2019). Diet and irradiation effects on the bacterial community composition and structure in the gut of domesticated teneral and mature Queensland fruit fly, Bactrocera tryoni (Diptera: Tephritidae). BMC Microbiol. 19:281. doi: 10.1186/s12866-019-1649-6
Wright, E. S. (2016). Using DECIPHER v2.0 to analyze big biological sequence data in R. R J. 8, 1–8. doi: 10.32614/rj-2016-025
Yao, Z., Ma, Q., Cai, Z., Raza, M. F., Bai, S., Wang, Y., et al. (2019). Similar Shift patterns in gut bacterial and fungal communities across the life stages of Bactrocera minax larvae from two field populations. Front. Microbiol. 10:2262. doi: 10.3389/fmicb.2019.02262
Yong, H. S., Song, S. L., Chua, K. O., and Lim, P. E. (2017). High diversity of bacterial communities in developmental stages of Bactrocera carambolae (Insecta: Tephritidae) revealed by illumina MiSeq sequencing of 16S rRNA gene. Curr. Microbiol. 74, 1076–1082. doi: 10.1007/s00284-017-1287-x
Zepeda-Paulo, F. A., Simon, J. C., Ramírez, C. C., Fuentes-Contreras, E., Margaritopoulos, J. T., Wilson, A. C. C., et al. (2010). The invasion route for an insect pest species: the tobacco aphid in the New World. Mol. Ecol. 19, 4738–4752. doi: 10.1111/j.1365-294X.2010.04857.x
Keywords: microbiota, plant-insect interactions, Anastrepha ludens, Tephritidae, gut
Citation: Aluja M, Zamora-Briseño JA, Pérez-Brocal V, Altúzar-Molina A, Guillén L, Desgarennes D, Vázquez-Rosas-Landa M, Ibarra-Laclette E, Alonso-Sánchez AG and Moya A (2021) Metagenomic Survey of the Highly Polyphagous Anastrepha ludens Developing in Ancestral and Exotic Hosts Reveals the Lack of a Stable Microbiota in Larvae and the Strong Influence of Metamorphosis on Adult Gut Microbiota. Front. Microbiol. 12:685937. doi: 10.3389/fmicb.2021.685937
Received: 26 March 2021; Accepted: 21 June 2021;
Published: 02 August 2021.
Edited by:
Christoph Vorburger, Swiss Federal Institute of Aquatic Science and Technology, SwitzerlandReviewed by:
Alison Ravenscraft, University of Texas at Arlington, United StatesBeatriz Sabater-Munoz, Polytechnic University of Valencia, Spain
Copyright © 2021 Aluja, Zamora-Briseño, Pérez-Brocal, Altúzar-Molina, Guillén, Desgarennes, Vázquez-Rosas-Landa, Ibarra-Laclette, Alonso-Sánchez and Moya. This is an open-access article distributed under the terms of the Creative Commons Attribution License (CC BY). The use, distribution or reproduction in other forums is permitted, provided the original author(s) and the copyright owner(s) are credited and that the original publication in this journal is cited, in accordance with accepted academic practice. No use, distribution or reproduction is permitted which does not comply with these terms.
*Correspondence: Martín Aluja, martin.aluja@inecol.mx; Jesús Alejandro Zamora-Briseño, alejandro.zamora@inecol.mx