- 1Unit of Environmental Sciences and Management, North-West University, Potchefstroom, South Africa
- 2Microbiology and Environmental Biotechnology Research Group, Agricultural Research Council-Natural Resources and Engineering, Pretoria, South Africa
- 3Department of Environmental Sciences, University of South Africa–Florida Campus, Johannesburg, South Africa
- 4Department of Physics, University of South Africa–Florida Campus, Johannesburg, South Africa
Globally, food security has become a critical concern due to the rise in human population and the current climate change crisis. Usage of conventional agrochemicals to maximize crop yields has resulted in the degradation of fertile soil, environmental pollution as well as human and agroecosystem health risks. Nanotechnology in agriculture is a fast-emerging and new area of research explored to improve crop productivity and nutrient-use efficiency using nano-sized agrochemicals at lower doses than conventional agrochemicals. Nanoparticles in agriculture are applied as nanofertilizers and/or nanopesticides. Positive results have been observed in terms of plant growth when using nano-based agricultural amendments. However, their continuous application may have adverse effects on plant-associated rhizospheric and endospheric microorganisms which often play a crucial role in plant growth, nutrient uptake, and disease prevention. While research shows that the application of nanoparticles has the potential to improve plant growth and yield, their effect on the diversity and function of plant-associated microorganisms remains under-explored. This review provides an overview of plant-associated microorganisms and their functions. Additionally, it highlights the response of plant-associated microorganisms to nanoparticle application and provides insight into areas of research required to promote sustainable and precision agricultural practices that incorporate nanofertilizers and nanopesticides.
1 Introduction
Food security is currently a global concern due to the exponential growth of human population coupled with the ongoing climate crisis. The conventional approach for improvement of crop productivity to sustain the growing population is by applying bulk chemical fertilizers (congruent with terms synthetic, inorganic, and mineral fertilizers) and pesticides. However, there is evidence that only a fraction of the chemical fertilizers and pesticides applied contribute to aiding crop production (Raliya et al., 2018; Tudi et al., 2021). Unfortunately, their residues, to a great extent, pollute the environment and groundwater via leaching. These pollutants cause soil degradation that could be in form of acidification and eutrophication. Such processes are usually hazardous to aquatic and agroecosystems (Tudi et al., 2021).
In spite of the potential yield benefits associated with bulk chemical fertilization, it is capable of changing the chemical properties of the soil and does not improve the richness, diversity, or abundance of soil microbial communities, which are generally indicators of fertile soil (Dincǎ et al., 2022). The viability and metabolic activity of bacterial and fungal species in the soil were reported to be impacted by excessive concentrations of chemical fertilizers (Dincǎ et al., 2022). On the other hand, pesticides may hinder vital cellular processes of non-target microorganisms and other soil biota which inadvertently results in reduced chemical and biological soil fertility (Vischetti et al., 2020; Tudi et al., 2021).
Recently, the use of nanotechnology in agriculture has been explored as an alternative to the conventional use of bulk chemical fertilizers and pesticides (Kalwani et al., 2022). Nanoparticles can potentially provide various benefits over conventional agricultural practices such as large surface area to volume ratios, mass transfer abilities as well as slow, controlled and targeted delivery of lower nutrient or pesticide concentrations to enhance crop productivity, if used appropriately (Hussain et al., 2023). Although the application of nanoparticles has been revolutionary for crop productivity, the response of plant-associated microorganisms to nano-based amendments remains unclear. Similarly to bulk chemical fertilizers or pesticides, nano-based agricultural amendments may have an impact on plant-associated microorganisms. Exposure of plant-associated microorganisms to nanoparticles can either be beneficial or harmful depending on various factors. Hence, further investigation on the interaction and response of plant-associated microorganisms to nanoparticles is warranted to ensure sustainable precision agricultural practices.
Microorganisms are extremely important for overall soil and plant health (Goswami et al., 2016). Diverse genera of microorganisms have been identified in soils and crops, and they play an important role in the regulation of agroecosystem productivity, soil physicochemical characteristics, and plant health (Dincǎ et al., 2022). A vast majority of plant-associated microorganisms are found in the soil, near plant roots in the region called the rhizosphere, where they serve essential ecological functions such as promoting plant growth (Bello-Akinosho et al., 2021). Although plants associated microorganisms can exert both negative and postive impacts of on the host plants, the focus of this review is majorly on the impact on beneficial plant associated microrogamisms. Plant beneficial bacteria residing in the rhizosphere are termed plant growth-promoting rhizobacteria (PGPR) while microorganisms that colonize the endosphere (interior of the plant) are termed endophytes. Both endophytes and PGPR could be claissfied as Plant growth-promoting microorganisms (PGPMs) if they are able to improve plant growth directly and indirectly. The PGPMs perform crucial metabolic functions which include the decomposition of organic matter, nitrogen fixation, nutrient solubilization, phytohormone production, as well as metabolite synthesis that aids in plant growth and disease prevention (Kalwani et al., 2022).
The diversity and abundance of PGPR in the rhizosphere have been studied since the beginning of the twentieth century (Fagorzi and Mengoni, 2022). However, before the discovery of fungal endophytes in the middle of the twentieth century, the endosphere was long believed to be sterile. Endosphere microbiology, initially dominated by fungal studies, has evolved over the years. Recent development has led to the expansion of the scope with more focus on bacteria. All plant species surveyed to date harbour different microbial communities. Examples of plant microbes that could be beneficial for such relationships are Bacillus and Pseudomonas that have been identified as predominant and diverse genera of PGPR. They play an important role as biocontrol agents through the protection of plants against phytopathogens (Santoyo et al., 2012).
Previous research in plant microbe interactions has laid the foundation by describing certain factors, sometimes referred to as filters, that influence the association. Most notable of these filters are the host plant chemistry, environmental conditions as well as microbe-microbe interactions (Saunders et al., 2010). However, what is not clear is the potential influence of other external factors that are introduced through anthropogenic means linked to technological advancement.
One system in which plant-microbe relationship could be impacted is the use of nanomaterials in agro-ecosystem. Applications of nanomaterials are becoming increasingly popular, especially with their usage as nanofertilizers and nanopesticides for precision and sustainable agriculture. Unfortunately, there is no existing guideline that reflects their potential impacts on plant associated microbes. Hence, this review aims to provide insights about existing literatures on this topic and reflects on potential implication for sustainable agricultural practices.
2 Plant-associated microorganisms in agriculture
The survival of the plant is greatly dependent on its plant-associated microorganisms (Backer et al., 2018). Similarly, the diversity abundance, and actvities of plant-associated microorganisms are affected by the variety of compounds actively released from living plants (exudates). Amino acids, carbohydrates, enzymes, organic acids, hormones, metabolites, and vitamins are examples of plant exudates (Li et al., 2019). This is the community assembly rule driven by the host plant chemistry (Comita et al., 2014). In this instance, plant exert considerable control over the constituents and abundance of plant-associated microorganisms through variations in composition, time of release, and concentration of exudates (Backer et al., 2018; Adeleke et al., 2019). By providing exudates that encourage the growth of specific microorganisms, the plant can shape the plant-associated microbial community (Dlamini et al., 2022). In turn, PGPMs provide plants with various benefits, which include nutrient acquisition, defence against plant pathogens, induction of systemic resistance, and plant growth promotion. Furthermore, PGPMs can protect host plants against abiotic stresses such as salinity, floods, droughts, heavy metal contamination, organic pollutants, and extreme temperatures (Lopes et al., 2021). Currently, much research has been directed to the isolation and characterization of plant-associated microorganisms using omics and sequencing approaches to determine their potential use in agriculture (Trivedi et al., 2021). A summary of the findings of such studies involving PGPR was reported by Saeed et al. (2021). The types of agricultural products consisting of PGPMs with various plant growth-promoting functions are summarized in Table 1. Applications of PGPMs as environmentally-friendly alternatives to agrochemicals involve inoculation of agricultural soils, roots, or seeds. However, the success of microbial inoculation in terms of establishing the PGPMs with persistence for beneficial association with host plants is affected by inoculation methods, environmental conditions, and requirements of host plants and should therefore undergo sufficient research trials prior to specific environmental application (Lopes et al., 2021). Furthermore, climate change and the introduction of emerging alternatives to agrochemicals may influence the productivity of plant holobionts (the plant microbiome and its tissues) (Lopes et al., 2021; Hussain et al., 2023). Hence, it is necessary to investigate the long-term effects of emerging agrochemical alternatives, such as nanoparticles, on plant holobionts. Moreover, future research should investigate the combined use of nanoparticle alternatives and PGPMs that can alleviate abiotic stresses for sustainable crop productivity.
2.1 Rhizospheric plant-associated microorganisms
Microorganisms thrive in the rhizosphere by utilizing root exudates as carbon and nutrient sources for growth and metabolic functions (Dlamini et al., 2022). The width of the rhizosphere ranges from 2 to 80 mm from the root surface, depending on the type of plant (Figure 1). The area of the rhizosphere may expand due to increased exudation, which may be stimulated by increased microbial activity. For instance, mycorrhizal fungi allow plant roots to reach a greater volume of soil through their hyphae while forming a mutualistic symbiotic relationship with the root by obtaining nutrients from and for host plants beyond the rhizosphere (Lanfranco et al., 2017). The plant-associated microorganisms present in and around the rhizosphere function as symbionts, pathogens, as well as food sources for other microorganisms (Munir et al., 2022). The most common genera of PGPR utilized for increasing crop productivity include Azospirillum, Bacillus, Burkholderia, Enterobacter, Flavobacterium, Pseudomonas, Rhizobium, Frankia, Clostridium, Klebsiella, Serratia and Streptomyces (Lopes et al., 2021). In addition to rhizobacteria, fungal groups that play a key role in agricultural productivity include Aspergillus, Fusarium, Penicillium, Piriformospora, Phoma and Trichoderma (Hossain et al., 2017). Of all the interactions between plants and microorganisms in the rhizosphere, biological nitrogen fixation is by far the most researched (Chen et al., 2019; Li et al., 2019; Soumare et al., 2020; Aasfar et al., 2021; Klimasmith and Kent, 2022; Zhang et al., 2023). Nitrogen-fixing bacteria like Rhizobia make unavailable N2 accessible to plants. Non-symbiotic or free-living nitrogen-fixing rhizobacteria include Azotobacter, Azospirillum, Bacillus, and Klebsiella. Additionally, Rhizobium, Bradyrhizobium, Mesorhizobium, and Sinorhizobium collaborate with plants in a symbiotic relationship to exchange nitrogen (N) for growth-promoting nutrients and protection (Munir et al., 2022). Besides nitrogen fixation, PGPMs produce organic chelating compounds that help boost the availability of nutrients like phosphorus (P), manganese (Mn), iron (Fe), zinc (Zn), and copper (Cu) to plants and produce secondary metabolites and phytohormones for biocontrol, plant stimulation and health promotion from the rhizosphere (Emmanuel and Babalola, 2020).
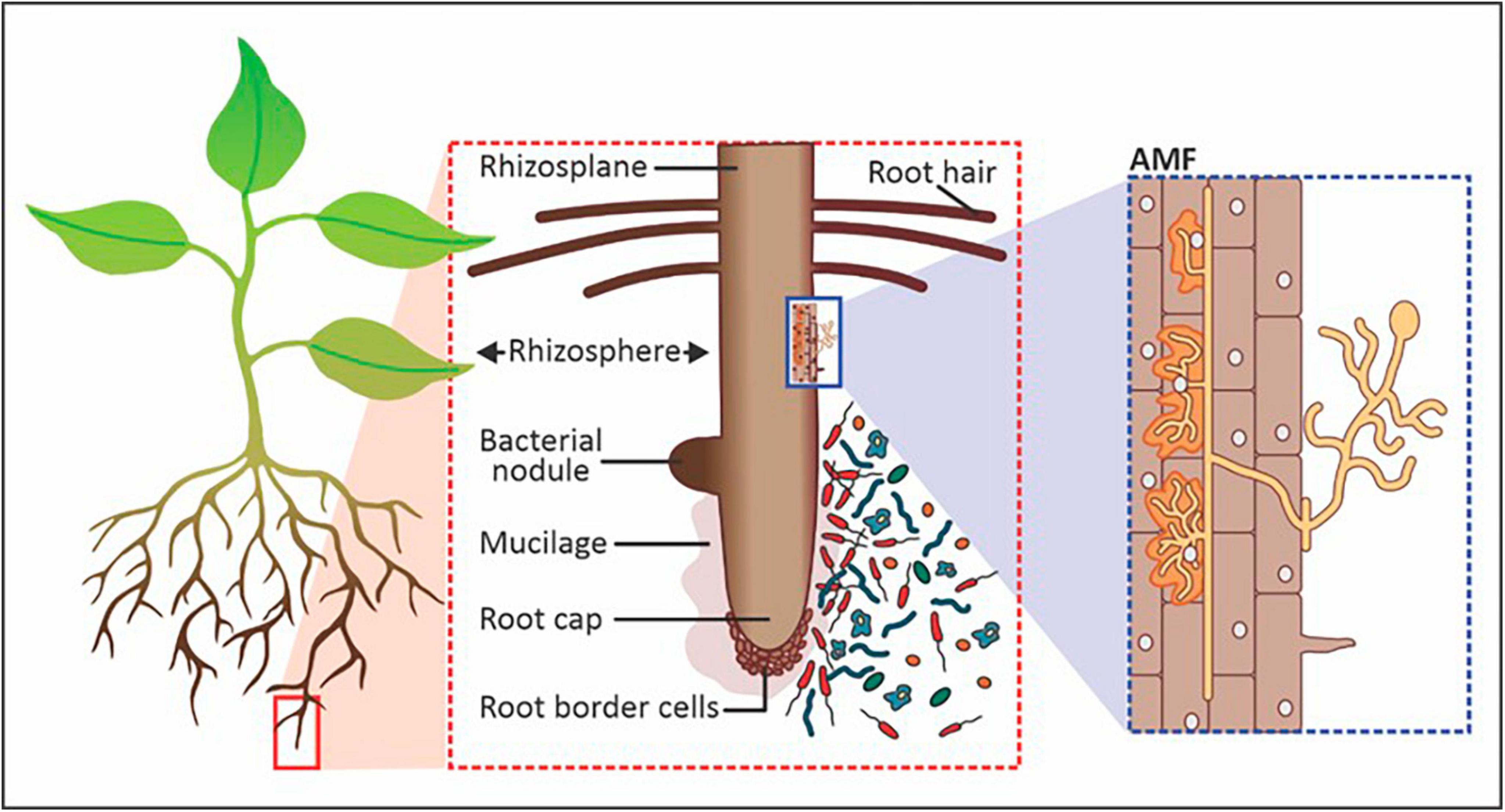
Figure 1. Components of the rhizosphere that includes symbiotic and saprophytic bacteria and arbuscular mycorrhizal fungi (AMF). Image adapted and modified from Philippot et al. (2013).
2.2 Endospheric plant-associated microorganisms
Plant-associated microorganisms that thrive within the roots, stems, and leaves of plants are termed endophytes. The location of endophytes within the plant compared to epiphytes (microorganisms occurring on the plant exterior) is shown in Figure 2. Endophytic diversity and population are highly variable between plant species and depend on components such as host developmental stage, species, and environmental conditions as well as their lifestyle classification (Surjit and Rupa, 2014). Systemic endophytes have long-term mutualistic associations with plants because they interact and evolve with host plants over many generations thus forming part of the core plant endobiome. In contrast, non-systemic endophytes have short-term associations with plants and their abundance, diversity and association can shift from mutualistic to parasitic depending on the plant development stage as well as biotic and abiotic factors (Orozco-Mosqueda and Santoyo, 2021).
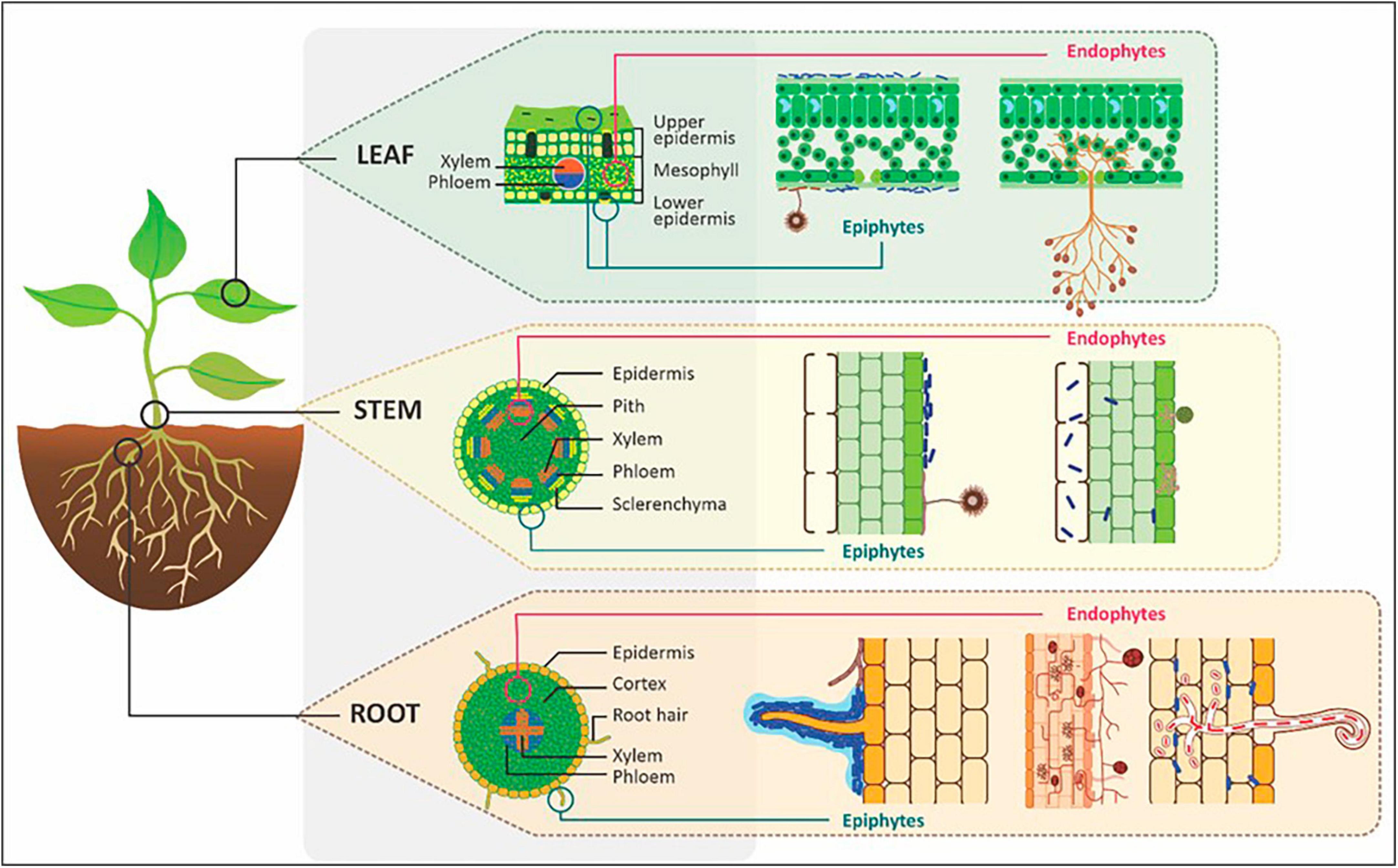
Figure 2. The location of epiphytes and endophytes within plant leaves, stems and roots. Image adapted and modified from Walker et al. (2020).
Often, endophytes are bacterial (actinomycetes or mycoplasma) or fungal (yeasts or filamentous) microorganisms. Endophytic fungi colonize the seed, during germination or through penetration of the plant tissues when recognized as a host (Poveda et al., 2021). Examples of endophytic fungi include Aspergillus, Bipolaris, Chaetomium, Cladosporium, Diaporthe, Fusarium, Alternaria, Mucor, Nigrospora, Paecilomyces, Penicillium, Piriformospora, Porostereum, Phoma, Trichoderma, Ulocladium, and Yarrowia (Orozco-Mosqueda and Santoyo, 2021). Most bacterial endophytes enter plants as rhizobacteria where they perform various plant growth-promoting functions (Pimentel et al., 2011; Nair and Padmavathy, 2014). Gram-positive and gram-negative bacteria, including Achromobacter, Acinetobacter, Agrobacterium, Bacillus, Brevibacterium, Microbacterium, Pseudomonas, Xanthomonas, were identified from the endosphere (Sun et al., 2013). Various plant growth-promoting bacterial endophytes have been isolated from plants as shown in Table 2. Root nodules, as depicted in Figure 1, are often found on the roots of leguminous plants and consist of endophytes termed nodule-associated bacteria. Tapia-García et al. (2020) isolated 257 nodule-associated bacteria from Phaseolus vulgaris plants with the most common plant growth-promoting attributes being indole acetic acid and siderophore production. Pseudomonas, Rhizobium, Cupriavidus, and Paraburkholderia were the most abundant bacterial genera isolated from the nodules of the leguminous plants. In another study, endophytes were shown to have a symbiotic relationship with other plant-associated microorganisms. The study concluded that the production of 1-aminocyclopropane-1-carboxylate deaminase by free-living bacteria (such as Pseudomonas fluorescens) played a key role in rhizobial nodulation processes by regulating impeding ethylene levels (Nascimento et al., 2019). Endophytes can be beneficial to plants by directly promoting plant growth and development through the metabolism of insoluble nutrients and the production of phytohormones, enzymes, and metabolites. On the other hand, endophytes can indirectly promote plant growth and health by stimulating the capacity of plants to withstand various stresses and their resistance to insects and other pests (Li et al., 2022).
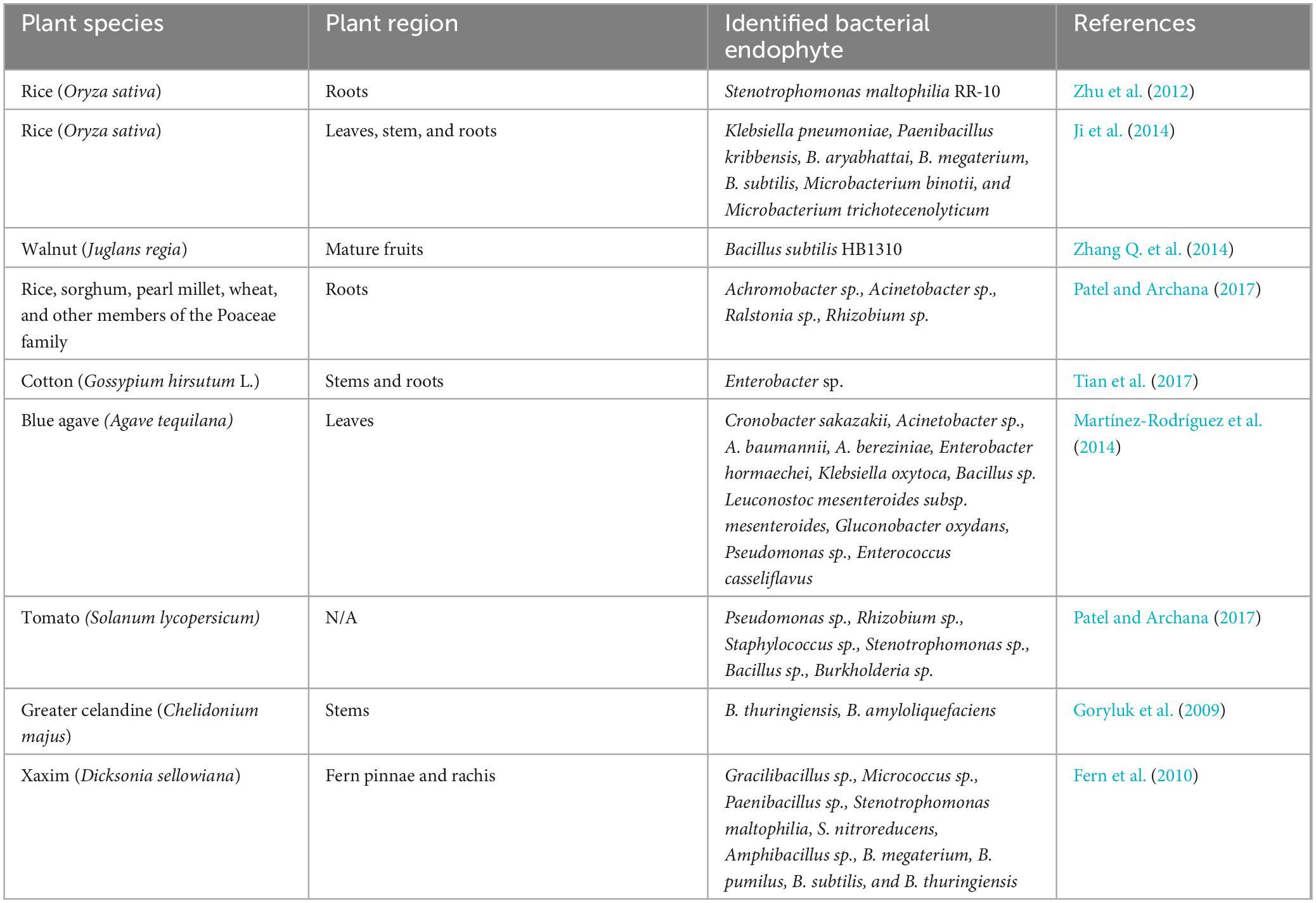
Table 2. Identified plant growth-promoting bacterial endophytes isolated from various plant species.
Overall, plant-associated microorganisms play critical roles in agroecosystems by regulating soil fertility, nutrient availability, water sequestration, and plant disease prevention, among other roles (Adeleke et al., 2019). Although the importance of plant-microorganism interactions in agroecosystems has been established, the response of plant-associated microorganisms to emerging nanoparticle applications in agriculture remains unclear.
3 Application of nanoparticles in agriculture
Nanotechnology has been described as the understanding and control of matter in the range of 1 to 100 nm (Rajput et al., 2018). Particle dimensions within this range are considered nanoparticles (NPs) (Taghavi et al., 2013). Nanoparticles are distinguished based on their core material (organic or inorganic). Inorganic NPs are further divided into metal (Al, Bi, Co, Cu, Au, Fe, In, Mo, Ni, Si, Ag, Sn, Ti, W, Zn), metal oxide (Al2O3, CeO2, CuO, Cu2O, In2O3, La2O3, MgO, NiO, SiO2, TiO2, SnO2, ZnO, ZrO2), of which Ag, ZnO, TiO2, FeO, and CuO are often utilized and their harmful effects on the activity, diversity, and abundance of flora and fauna are closely observed (Rajput et al., 2018).
Nanoparticles can be used in agriculture as fertilizers or pesticides and are generally regarded as nanofertilizers and nanopesticides, respectively. The use of nanoparticles as nanofertilizers in agriculture has the potential to improve the efficiency of nutrient consumption (Toksha et al., 2021; Ndaba et al., 2022; Rabalao et al., 2022). Additionally, the use of nanoparticles in the form of nanopesticides may protect crops from fungal and bacterial infections (Yadav S. A. et al., 2022). However, the impact of continued use of nanoparticles on plant-associated microorganisms remains unclear. Studies on the effects of nanoparticles on soil and plant microbiomes remain rare, even though microbial communities are important and sensitive determinants of the environmental hazards of nanoparticles (Brookes, 1995; Holden et al., 2014).
Despite the potential benefits of applying nanotechnology to agriculture, some researchers have cautioned and expressed concern about the consequences of nanoparticle applications in agriculture (Khan et al., 2022). Table 3 provides a summary of the benefits and drawbacks of using nanoparticles in agriculture. Nanoparticles are introduced into the agroecosystem via the application of nano-based agriculture amendments as well as the direct release of waste from industries and households (Weir et al., 2012; Sánchez-Quiles and Tovar-Sánchez, 2014). The impact of direct exposure of plants to nanoparticles should not be ignored as they may pose both negative and/or positive effects on soil health as well as crop growth and quality. The factors that influence the effects of nanoparticles include the type and size of the nanoparticle, plant species, nanoparticle concentration, and length of time that the soil/crop was exposed to the nanoparticles (Duan and Li, 2013). In a study done by An et al. (2008) silver nanoparticles boosted ascorbate and chlorophyll in the leaves of asparagus (Asparagus officinalis L.). These findings provide examples of the beneficial effects of nanoparticles. In a different study, silica nanoparticles applied to maize seedlings increased seed germination, root and shoot length, photosynthesis, and dry weight (Suriyaprabha et al., 2012).
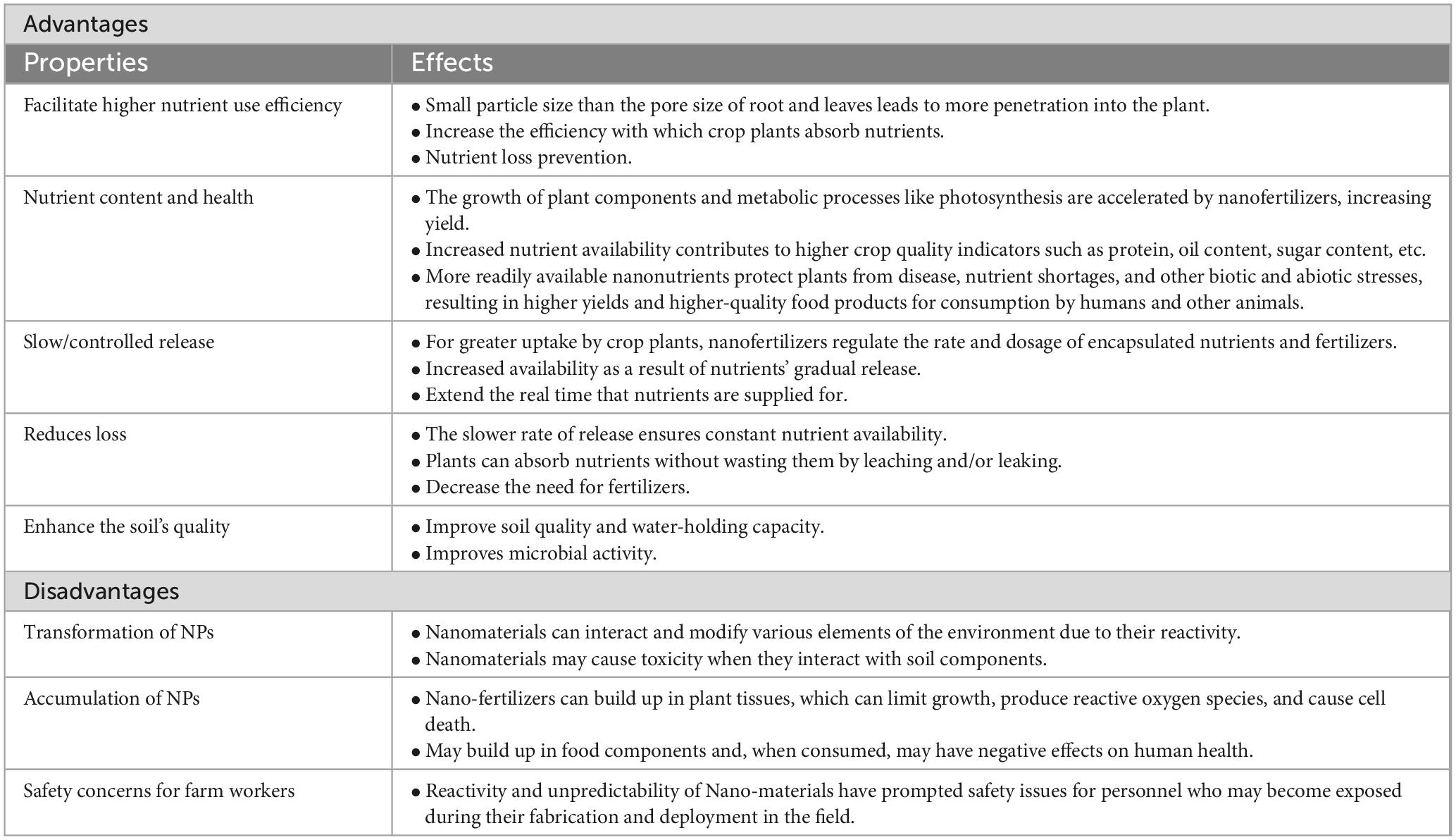
Table 3. Advantages and disadvantages of nanoparticle application in agriculture (Sindhu et al., 2020).
On the other hand, some reports on metal nanoparticles (MNPs) suggest negative impacts on the growth and physiology of internationally significant crops like maize (Zea may L.), wheat (Triticum aestivum), rice (Oryza sativa L.) and soybean (Dimkpa et al., 2012; Nair and Chung, 2014; Thuesombat et al., 2014). The toxic effects of nanoparticle application on crops are both physical and physiological, and examples include a reduction in fruit yield, plant growth, and biomass. Nanoparticles may also cause indirect toxicity to plants by damaging plant roots, enhancing uptake of contaminants by plants, and by altering plant-associated microbial communities (Anjum et al., 2013; Ge et al., 2014). The mechanisms by which nanoparticles interact and impact plant associated microorganisms, following their application, is discussed in the following sections.
3.1 Interaction of nanoparticles with plant-associated microorganisms
The potential use of nanoparticles as nanofertilizers and nanopesticides for precision and sustainable agriculture is still in its infancy and is currently under rigorous investigation (Zulfiqar et al., 2019; Hazarika et al., 2022; Zain et al., 2024). The application of nanofertilizers and nanopesticides may impact various plant growth characteristics (such as seed germination, root and shoot growth, chlorophyll content, photosynthesis, flowering, fruit formation, as well as crop yield), depending on the plant’s genetic makeup, soil and plant microbiology, soil nutrients (macronutrients and micronutrients), soil pH, moisture, and other environmental factors (Juo and Franzluebbers, 2003; Bratovcic et al., 2021; Okey-Onyesolu et al., 2021). Nanoparticles introduced in soil and plants could directly or indirectly affect the type of microorganisms present and alter their functions (Mosquera et al., 2018; Kibbey and Strevett, 2019).
The effects of nanoparticles on plant-associated microbial communities are highly dependent on the plant type, nanoparticle type (physical characteristics and chemical composition), soil properties (i.e., clay and organic matter content), as well as soil physicochemical characteristics (texture, organic matter content, pH, etc.) (Kumar et al., 2018; Kibbey and Strevett, 2019; Peng et al., 2020). In the rhizosphere, plants release a variety of exudates that promote microbial growth. Meanwhile, microorganisms work in concert with plant roots to support plant growth by facilitating a variety of nutrient cycles (Zhang N. et al., 2014). The presence of nanoparticles in soil dramatically affects the microbial communities in the rhizosphere, plant exudates, and extracellular materials produced by the microorganisms (Gao et al., 2018). Additionally, nanoparticles can enter the plant directly through root and stomata pores on leaf surfaces, with diameters ranging from a few tens of nanometers to a few hundred (Carpita et al., 1979; Eichert and Goldbach, 2008; Eichert et al., 2008). Subsequently, nanoparticles are transported by plasmodesmata from cell to cell within the plant, where they affect various physiological functions as well as plant endophytes (Zambryski, 2004).
Nanoparticle-microbe interactions within the plant and soil play a significant role in disease management and subsequent plant improvement. However, this is influenced by either negative or positive nanoparticle effects as antimicrobial agents or microbial growth promoters, respectively. The mechanism in which nanoparticles hinder the development of various microorganisms involves the release of metal ions that interact with cellular components through various pathways. These pathways include generation of reactive oxygen species (ROS), formation of pores in the cell membrane, damage to cell walls, DNA damage, and cell cycle arrest. Ultimately, all these lead to the inhibition of cell growth and in some cases, phytopathogen inhibition (Singh et al., 2019).
While many studies may have focused on nanoparticle mechanisms as antimicrobials, it has also been shown that nanoparticles can play a positive role on microbial metabolism and functions. The beneficial nanoparticle-microbe interactions include nanoparticles’ high bioavailability due to increased specific surface areas. This helps in nutrient uptake by the microbes as nanoparticles provide microorganisms with essential nutrients that stimulate growth and metabolic activity. Nanoparticles can also act as nano-tools for electron transfer, chemotaxis, and storage units (Mansor and Xu, 2020).
Learning about the mechanisms in which microorganisms interact with nanoparticles might help in the development of nanomaterials that are safe for the environment. This can include development of green synthesis approach for nanoparticle production. Overall, the use of nanoparticles as agricultural amendments requires further investigation as it may directly or indirectly affect plant growth by influencing plant-associated microorganisms.
3.1.1 Impact of nanofertilizer on plant-associated microorganisms
Nanoparticles, when used in the form of nanofertilizer, have been proven to enhance crop growth and quality (Merghany et al., 2019; Babu et al., 2022). Unlike bulk chemical fertilizers, which are required in high doses, nanofertilizers can be applied in relatively smaller quantities. Applying a lower dosage of nanofertilizer can minimize the potential for nutrient loss through leaching and volatilization, and thereby improves nutrient use efficiency (Raliya et al., 2018).
Three factors – intrinsic, extrinsic, and mode of administration – affect the efficiency of nanofertilizers. Nano-formulation techniques, particle size, and surface coating are examples of intrinsic variables. While extrinsic factors include soil texture, depth, pH, temperature, organic matter, and microbial activity (Zulfiqar et al., 2019). Moreover, the mechanism of delivery through plant roots or leaves (foliar) has a considerable impact on the uptake, behavior, and bioavailability of nanofertilizers (Mahil and Kumar, 2019). Due to their interaction with organic materials in the soil, nanofertilizers may change the soil surface chemistry, which could have an impact on plants and microorganisms. On the other hand, microorganisms and their actions can potentially alter how nanoparticles behave (Frenk et al., 2013; Zulfiqar et al., 2019; Toksha et al., 2021).
In a study conducted by Kaur et al. (2022), the effect of titanium dioxide (TiO2) NPs on the soil rhizosphere of mung bean crop was evaluated. The TiO2 NPs were shown to stimulate growth of soil microflora (N-fixers and ammonia oxidizers) as well as increase enzymatic activity for dehydrogenase, phosphatase, protease, urease, and catalase at low concentrations (1.0, 2.5, 5.0, and 10.0 mg/L) compared to the higher concentration (20 mg/L). In addition, the nitrate-N content increased with days after treatment and TiO2 NP concentration. An experiment conducted by Helal et al. (2023) demonstrated that the tomato plant (Lycopersicon Esculentum L.) treated with a controlled-release nano-urea (CRU) fertilizer showed better plant growth, yield, and fruit quality compared to the conventional fertilizers. In another study, an increase in nutritional value of spinach (Spinacia oleracea) after treatment with ZnO NPs (500 and 1,000 ppm) was indicated by higher values of protein and dietary fiber, as well as overall leaf quality (width, length, color, and surface area) (Revanappa and Pramod, 2015). Table 4 highlights some of the impacts of different nanofertilizers on microbial processes and related microorganisms in plants.
Despite the many benefits of nanofertilizers, the antibacterial potential of nanoparticles in general has also received substantial attention (Nath et al., 2008; Ramírez Aguirre et al., 2020; Thakral et al., 2021). The applied nanofertilizers may inadvertently have negative impacts on the beneficial microbial populations in the soil and on plants. The concentration and identity of nanoparticles, soil type, pH, and biological factors including root exudates and microbial diversity all have a significant impact on how nanoparticles affect the soil. A study conducted by Xu et al. (2015) investigating the effect of CuO NPs on soil microbes in flooded paddy soil reported CuO NPs (500 and 1,000 mg/kg) to have a negative impact on the soil microbes as was indicated by a significant decrease in microbial biomass and decrease in enzyme activity for urease, phosphatases, and dehydrogenase. The application of silver nanoparticles (Ag NPs) at 100 mg/kg significantly increased the soil pH and altered bacterial groups associated with carbon, nitrogen, and phosphorus cycling both in the absence or presence of cucumber (Cucumis sativus) plants (Zhang et al., 2020). Metal oxide nanoparticles, namely ZnO and CeO2, were observed to inhibit enzymatic activity and reduced the numbers of K-solubilizing and P-solubilizing bacteria as well as soil Azotobacter (Zhang et al., 2018; Kaur et al., 2022). In another study, the activity of soil dehydrogenase was demonstrated to be adversely affected by high quantities of nanoparticles (García-Gómez et al., 2018). Dehydrogenase activity directly correlates with soil microbial biomass, and plays a significant role in the oxidation of organic materials. Therefore, the microbial biomass was impacted by the dose of nanoparticles applied (García-Gómez et al., 2018). Another study by Shah et al. (2014) examining the response of the soil microbial community to nanoparticle application showed that silver nanomaterial caused changes in the microbial community structure, however, zinc oxide and zero-valent copper oxide did not significantly alter the structure of the microbial community.
3.1.2 Impact of nanopesticides on plant-associated microorganisms
Plant diseases and insect pests are effectively managed in agriculture by the application of pesticides. However, the high concentrations of chemical components applied per hectare has given rise to several issues, including environmental deterioration, pest resistance, bioaccumulation, and health risks (Yadav J. et al., 2022). Due to microbial activity, air drift, soil leaching, degradation processes including photolysis and hydrolysis, amongst other factors, more than 90% of the pesticides that are applied are lost. It is only a small amount of the remaining 10% that eventually reaches the target site (Yadav J. et al., 2022). This necessitates repeated application which eventually results in high costs and environmental pollution. Moreover, certain pesticides have been shown to have adverse effects on human health such as cancer, birth defects, reproductive defect, neurological and developmental impairment, immunotoxicity, and disruption of the endocrine system, when ingested through the consumption of pesticide-contaminated food (Toksha et al., 2021).
Although some environment-specific nanopesticides are on the market (Smith et al., 2008), nano-formulations with effective delivery mechanisms which result in application of modest amounts of nanopesticides are required. Nanopesticides provide innovative strategies for delivering the active ingredient of pesticides to the target site (Ahmed et al., 2023). Slow-releasing qualities, enhanced stability, permeability, solubility, and specificity are all features of nano-encapsulated pesticide formulations (Narayanan et al., 2017). They are specifically created to make the active ingredient (AI) more soluble and release it at the target site in a controlled manner. Due to this, only a small amount of the AI needs to be applied for it to be effective for an extended period of time (Oliveira et al., 2019).
Nanopesticides are classified into two types. Type 1 nanopesticides are metal-based, whereas Type 2 materials contain AIs that are enclosed by nanocarriers, such as polymers, clays, and zein nanoparticles. The most prevalent analytes for Type 1 nanopesticides are Ag-, Ti-, and Cu-based nanomaterials (NMs). These nanopesticides can suppress a variety of plant pathogens, including fungal (such as Candida and Fusarium), as well as bacterial (such as Escherichia coli and Staphylococcus) (Wang et al., 2022). If properly applied, nanopesticides could increase crop output, food safety, and nutritional value. For several plants treated with Type 1 nanopesticides (such as Ag-, Ti-, Cu-, and Zn-based NMs), improvements in the concentration of sugar, fatty acids, chlorophyll, carotenes, and important elements (such as P, K, Ca, Mg, S, Fe, Si, Mn, and Zn) have been documented (Gomez et al., 2021; Ma et al., 2021; Rawat et al., 2021; Shang et al., 2021; Yadav J. et al., 2022). Suppression of pathogenic activity is one of the factors contributing to these enhancements.
The abundance, structure, and network functioning of the plant-associated microbiome, which includes archaea, bacteria, and fungi, can be changed by adding metal-based nanopesticides to soil and plant. This in turn may change the bioavailability and recycling of macronutrients (such as C, N, P, and S). More importantly, in order to fully utilize nanopesticides, it is necessary to comprehend how they interact with nutrients, soil, plant-associated microbiota, and other factors. Nanopesticides have obvious pesticidal activity and as such can exhibit toxicity toward non-target organisms. Studies show that, in comparison to their non-nanoscale equivalents, nanopesticides are 43.1% less toxic (Wang et al., 2020). This is primarily due to their AI delivery system, which is target-specific, and thereby minimizes the exposure to non-target organisms.
Cu(OH)2 nanopesticides applied to target soil agroecosystems for 365 days, had only minor negative effects on non-target wetland systems and the bacterial and fungal communities that live there (Carley et al., 2020). However, a few studies have shown negative impacts related to nanopesticide exposure. Zhai et al. (2020) showed that long-term exposure to high concentrations of atrazine-containing nanopesticides (NPATZs) dramatically reduced the metabolic capability of bacterial communities in the rhizosphere and changed the makeup of those communities in comparison to conventional ATZ. An investigation into the long-term (117 days) effects of Ag nanopesticides (100 mg/kg) on the microbiome of the maize rhizosphere revealed negative effects on microbial diversity, the nitrogen cycle, and crop output (Sillen et al., 2020). Low concentrations (0.5, 1.0, and 2.0 mg/g) of zinc oxide (ZnO) applied directly to soil enhanced the relative abundance of the essential bacterial group Bacillus in comparison to the control, but the higher concentrations had harmful effects on the bacterial population (You et al., 2018). A study by Zhao et al. (2017), discovered that exposure of spinach to Cu(OH)2 nanopesticide resulted in a significant reduction in antioxidant or defence-associated metabolites such as ascorbic acid, α-tocopherol, threonic acid, β sitosterol, 4-hydroxybutyric acid, ferulic acid, and total phenolics (Peixoto et al., 2021). Another study showed that captan@ZnO35-45 nm and captan@SiO2 20–30nm nanofungicides influenced soil microorganisms by altering numerous microbial characteristics (Sułowicz et al., 2023).
Overall, literature suggests that nanopesticides may be more effective, resilient, and sustainable than their traditional analogues, with fewer negative environmental effects. However, future research is required to comprehend the effects of realistic nanopesticide doses on the rhizosphere microbiota, crop yield, and agroecosystem health in field settings.
4 Conclusion and future prospects
Based on the literature investigated in this review, the use of nanoparticles as nanofertilizers or nanopesticides were shown to have both beneficial and negative effects on plant-associated microbial populations as well as crop and soil properties. The implications of exposing agricultural environments to nanoparticles can therefore be beneficial or detrimental with respect to the health of agroecosystems and as a result of downstream consumption of crops. Moreover, the environmental risk assessment of nanoparticles is in its infancy. Thus, further research studies investigating the impact of different types and doses of nanoparticles, applied under varying environmental conditions, on microbial communities and function, especially long-term, are necessary. Despite the rise in the manufacturing of nanoparticles for agricultural applications, the majority of risk assessment testing is conducted in-vitro using cells rather than animals as test subjects. Therefore, more research on soil and human health implications is necessary due to the ambiguities surrounding the negative consequences of nanoparticle applications. In the context of impact of nanofertilizers and nanopesticides, plant-associated microorganisms indicative of healthy/unhealthy crops and soils should be employed as sensitive biomarkers to assess the environmental risk of these nanomaterials. Moreover, prospective studies should investigate the impact of nano-based agricultural amendments under different conditions such as crop type, soil properties and microbial community dynamics for the compilation of a database that can provide a case-by-case basis for precision agricultural practices incorporating the utilization of nanoparticles.
Author contributions
KM: Conceptualization, Writing—original draft, Writing—review and editing. BN: Conceptualization, Funding acquisition, Project administration, Supervision, Writing—review and editing. AR: Funding acquisition, Supervision, Writing—review and editing. HR: Writing—review and editing. RA: conceptualization, Funding acquisition, Supervision, Writing—review and editing.
Funding
The author(s) declare financial support was received for the research, authorship, and/or publication of this article. This work was supported by the National Research Foundation of South Africa (NRF; Grant numbers 121924 and 128307).
Acknowledgments
Opinions expressed and conclusions reached are those of the authors and not necessarily endorsed by the NRF.
Conflict of interest
The authors declare that the research was conducted in the absence of any commercial or financial relationships that could be construed as a potential conflict of interest.
The author(s) declared that they were an editorial board member of Frontiers, at the time of submission. This had no impact on the peer review process and the final decision.
Publisher’s note
All claims expressed in this article are solely those of the authors and do not necessarily represent those of their affiliated organizations, or those of the publisher, the editors and the reviewers. Any product that may be evaluated in this article, or claim that may be made by its manufacturer, is not guaranteed or endorsed by the publisher.
References
Aasfar, A., Bargaz, A., Yaakoubi, K., Hilali, A., Bennis, I., Zeroual, Y., et al. (2021). Nitrogen fixing azotobacter species as potential soil biological enhancers for crop nutrition and yield stability. Front. Microbiol. 12:628379. doi: 10.3389/fmicb.2021.628379
Adeleke, R. A., Raimi, A. R., Roopnarain, A., and Mokubedi, S. M. (2019). Status and Prospects of Bacterial Inoculants for Sustainable Management of Agroecosystems BT - Biofertilizers for Sustainable Agriculture and Environment, eds B. Giri, R. Prasad, Q.-S. Wu, and A. Varma (Cham: Springer). doi: 10.1007/978-3-030-18933-4_7
Ahmed, A., He, P., He, P., Wu, Y., He, Y., and Munir, S. (2023). Environmental effect of agriculture-related manufactured nano-objects on soil microbial communities. Environ. Int. 173:107819. doi: 10.1016/j.envint.2023.107819
Ajilogba, C., Babalola, O. O., Adebola, P., and Adeleke, R. (2022). Bambara groundnut rhizobacteria antimicrobial and biofertilization potential. Front. Plant Sci. 13:854937. doi: 10.3389/fpls.2022.854937
An, J., Zhang, M., Wang, S., and Tang, J. (2008). Physical, chemical and microbiological changes in stored green asparagus spears as affected by coating of silver nanoparticles-PVP. Lwt 41, 1100–1107. doi: 10.1016/j.lwt.2007.06.019
Anjum, N. A., Gill, S. S., Duarte, A. C., Pereira, E., and Ahmad, I. (2013). Silver nanoparticles in soil-plant systems. J. Nanoparticle Res. 15:1896. doi: 10.1007/s11051-013-1896-7
Babu, S., Singh, R., Yadav, D., Rathore, S. S., Raj, R., Avasthe, R., et al. (2022). Nanofertilizers for agricultural and environmental sustainability. Chemosphere 292:133451. doi: 10.1016/j.chemosphere.2021.133451
Backer, R., Rokem, J. S., Ilangumaran, G., Lamont, J., Praslickova, D., Ricci, E., et al. (2018). Plant growth-promoting rhizobacteria: context, mechanisms of action, and roadmap to commercialization of biostimulants for sustainable agriculture. Front. Plant Sci. 871:1473. doi: 10.3389/fpls.2018.01473
Bano, A., Waqar, A., Khan, A., and Tariq, H. (2022). Phytostimulants in sustainable agriculture. Front. Sustain. Food Syst. 6:801788. doi: 10.3389/fsufs.2022.801788
Bello-Akinosho, M., Ndaba, B., Roopnarain, A., Bamuza-Pemu, E., Nkuna, R., Rama, H., et al. (2021). “Agro-ecosystemBioremediation mediated by plant-microbe associations: a novel tool for bioremediation,” in Rhizomicrobiome Dynamics in Bioremediation, ed. K. Vivek (Boca Rotan, FL: CRC Press), 210–230. doi: 10.1201/9780367821593-11
Bratovcic, A., Hikal, W. M., Said-Al Ahl, H. A. H., Tkachenko, K. G., Baeshen, R. S., Sabra, A. S., et al. (2021). Nanopesticides and nanofertilizers and agricultural development: scopes, advances and applications. Open J. Ecol. 11, 301–316. doi: 10.4236/oje.2021.114022
Brookes, P. C. (1995). The use of microbial parameters in monitoring soil pollution by heavy metals. Biol. Fertil. Soils 19, 269–279. doi: 10.1007/BF00336094
Carley, L. N., Panchagavi, R., Song, X., Davenport, S., Bergemann, C. M., McCumber, A. W., et al. (2020). Long-term effects of copper nanopesticides on soil and sediment community diversity in two outdoor mesocosm experiments. Environ. Sci. Technol. 54, 8878–8889. doi: 10.1021/acs.est.0c00510
Carpita, N., Sabularse, D., Montezinos, D., and Delmer, D. P. (1979). Determination of the pore size of cell walls of living plant cells. Science 205, 1144–1147. doi: 10.1126/science.205.4411.1144
Chen, C., Guo, L. L., Chen, Y., Qin, P., and Wei, G. (2023). Pristine and sulfidized zinc oxide nanoparticles alter bacterial communities and metabolite profiles in soybean rhizocompartments. Sci. Total Environ. 855:158697. doi: 10.1016/j.scitotenv.2022.158697
Chen, J., Shen, W., Xu, H., Li, Y., and Luo, T. (2019). The composition of nitrogen-fixing microorganisms correlates with soil nitrogen content during reforestation: a comparison between legume and non-legume plantations. Front. Microbiol. 10:508. doi: 10.3389/fmicb.2019.00508
Comita, L. S., Queenborough, S. A., Murphy, S. J., Eck, J. L., Xu, K., Krishnadas, M., et al. (2014). Testing predictions of the Janzen-Connell hypothesis: A meta-analysis of experimental evidence for distance- and density-dependent seed and seedling survival. J. Ecol. 102, 845–856. doi: 10.1111/1365-2745.12232
Dimkpa, C. O., McLean, J. E., Latta, D. E., Manangón, E., Britt, D. W., Johnson, W. P., et al. (2012). CuO and ZnO nanoparticles: phytotoxicity, metal speciation, and induction of oxidative stress in sand-grown wheat. J. Nanoparticle Res. 14, 1125. doi: 10.1007/s11051-012-1125-9
Dincǎ, L. C., Grenni, P., Onet, C., and Onet, A. (2022). Fertilization and soil microbial community: A review. Appl. Sci. 12:1198. doi: 10.3390/app12031198
Dlamini, S. P., Akanmu, A. O., and Babalola, O. O. (2022). Rhizospheric microorganisms: the gateway to a sustainable plant health. Front. Sustain. Food Syst. 6:925802. doi: 10.3389/fsufs.2022.925802
Duan, X., and Li, Y. (2013). Physicochemical characteristics of nanoparticles affect circulation, biodistribution, cellular internalization, and trafficking. Small 9, 1521–1532. doi: 10.1002/smll.201201390
Eichert, T., and Goldbach, H. E. (2008). Equivalent pore radii of hydrophilic foliar uptake routes in stomatous and astomatous leaf surfaces - further evidence for a stomatal pathway. Physiol. Plant. 132, 491–502. doi: 10.1111/j.1399-3054.2007.01023.x
Eichert, T., Kurtz, A., Steiner, U., and Goldbach, H. E. (2008). Size exclusion limits and lateral heterogeneity of the stomatal foliar uptake pathway for aqueous solutes and water-suspended nanoparticles. Physiol. Plant. 134, 151–160. doi: 10.1111/j.1399-3054.2008.01135.x
Emmanuel, O. C., and Babalola, O. O. (2020). Productivity and quality of horticultural crops through co-inoculation of arbuscular mycorrhizal fungi and plant growth promoting bacteria. Microbiol. Res. 239:126569. doi: 10.1016/j.micres.2020.126569
Fagorzi, C., and Mengoni, A. (2022). Endophytes: improving plant performance. Microorganisms 10, 19–21. doi: 10.3390/microorganisms10091777
Fern, T. H. E., Dicksoniaceae, H., Barros, I. D. A., Araújo, W. L., Azevedo, J. L., Paulo, U. D. S., et al. (2010). The effect of different growth regimes on the endophytic bacterial communities of the fern. Brazilian J. Microbiol. 41, 956–965. doi: 10.1590/S1517-83822010000400014
Frenk, S., Ben-Moshe, T., Dror, I., Berkowitz, B., and Minz, D. (2013). Effect of metal oxide nanoparticles on microbial community structure and function in two different soil types. PLoS One 8:e0084441. doi: 10.1371/journal.pone.0084441
Gao, X., Avellan, A., Laughton, S., Vaidya, R., Rodrigues, S. M., Casman, E. A., et al. (2018). CuO nanoparticle dissolution and toxicity to wheat (Triticum aestivum) in Rhizosphere soil. Environ. Sci. Technol. 52, 2888–2897. doi: 10.1021/acs.est.7b05816
García-Gómez, C., Fernández, D., García, S., Obrador, A. F., Letón, M., and Babín, M. (2018). Soil pH effects on the toxicity of zinc oxide nanoparticles to soil microbial community. Environ. Sci. Pollut. Res. 25, 28140–28152. doi: 10.1007/s11356-018-2833-1
Ge, Y., Priester, J. H., Van De Werfhorst, L. C., Walker, S. L., Nisbet, R. M., An, Y. J., et al. (2014). Soybean plants modify metal oxide nanoparticle effects on soil bacterial communities. Environ. Sci. Technol. 48, 13489–13496. doi: 10.1021/es5031646
Gomez, A., Narayan, M., Zhao, L., Jia, X., Bernal, R. A., Lopez-Moreno, M. L., et al. (2021). Effects of nano-enabled agricultural strategies on food quality: current knowledge and future research needs. J. Hazard. Mater. 401:123385. doi: 10.1016/j.jhazmat.2020.123385
Goryluk, A., Rekosz-Burlaga, H., and Błaszczyk, M. (2009). Isolation and characterization of bacterial endophytes of Chelidonium majus L. Polish J. Microbiol. 58, 355–361. doi: 10.3389/fmicb.2021.628379
Goswami, D., Thakker, J. N., and Dhandhukia, P. C. (2016). Portraying mechanics of plant growth promoting rhizobacteria (PGPR): a review. Cogent Food Agric. 2:1127500. doi: 10.1080/23311932.2015.1127500
Hazarika, A., Yadav, M., Yadav, D. K., and Yadav, H. S. (2022). An overview of the role of nanoparticles in sustainable agriculture. Biocatal. Agric. Biotechnol. 43:102399. doi: 10.1016/j.bcab.2022.102399
He, S., Feng, Y., Ni, J., Sun, Y., Xue, L., Feng, Y., et al. (2016). Different responses of soil microbial metabolic activity to silver and iron oxide nanoparticles. Chemosphere 147, 195–202. doi: 10.1016/j.chemosphere.2015.12.055
Helal, M. I. D., El-Mogy, M. M., Khater, H. A., Fathy, M. A., Ibrahim, F. E., Li, Y. C., et al. (2023). A controlled-release nanofertilizer improves tomato growth and minimizes nitrogen consumption. Plants 12:1978. doi: 10.3390/plants12101978
Holden, P. A., Schimel, J. P., and Godwin, H. A. (2014). Five reasons to use bacteria when assessing manufactured nanomaterial environmental hazards and fates. Curr. Opin. Biotechnol. 27, 73–78. doi: 10.1016/j.copbio.2013.11.008
Hossain, M. M., Sultana, F., and Islam, S. (2017). Plant Growth-Promoting Fungi (PGPF): Phytostimulation and Induced Systemic Resistance. Berlin: Springer. doi: 10.1007/978-981-10-6593-4_6
Hussain, M., Zahra, N., Lang, T., Zain, M., Raza, M., Shakoor, N., et al. (2023). Integrating nanotechnology with plant microbiome for next-generation crop health. Plant Physiol. Biochem. 196, 703–711. doi: 10.1016/j.plaphy.2023.02.022
Ji, S. H., Gururani, M. A., and Chun, S. C. (2014). Isolation and characterization of plant growth promoting endophytic diazotrophic bacteria from Korean rice cultivars. Microbiol. Res. 169, 83–98. doi: 10.1016/j.micres.2013.06.003
Juo, A. S. R., and Franzluebbers, K. (2003). Soil biology and microbiology. Trop. Soils 6, 71–87. doi: 10.1093/oso/9780195115987.003.0008
Kalwani, M., Chakdar, H., Srivastava, A., Pabbi, S., and Shukla, P. (2022). Effects of nanofertilizers on soil and plant-associated microbial communities: emerging trends and perspectives. Chemosphere 287:132107. doi: 10.1016/j.chemosphere.2021.132107
Kaur, H., Kalia, A., Sandhu, J. S., Dheri, G. S., Kaur, G., and Pathania, S. (2022). Interaction of TiO2 nanoparticles with soil: effect on microbiological and chemical traits. Chemosphere 301:134629. doi: 10.1016/j.chemosphere.2022.134629
Kaur, P., and Purewal, S. S. (2019). “Biofertilizers and their role in sustainable agriculture,” in Biofertilizers for Sustainable Agriculture Environment, eds B. Giri, R. Prasad, Q. Wu, and A. Varma (Berlin: Springer). doi: 10.1007/978-3-030-18933-4_12
Khan, S. T., Adil, S. F., Shaik, M. R., Alkhathlan, H. Z., Khan, M., and Khan, M. (2022). Engineered nanomaterials in soil: their impact on soil microbiome and plant health. Plants 11:109. doi: 10.3390/plants11010109
Kibbey, T. C. G., and Strevett, K. A. (2019). The effect of nanoparticles on soil and rhizosphere bacteria and plant growth in lettuce seedlings. Chemosphere 221, 703–707. doi: 10.1016/j.chemosphere.2019.01.091
Klimasmith, I. M., and Kent, A. D. (2022). Micromanaging the nitrogen cycle in agroecosystems. Trends Microbiol. 30, 1045–1055. doi: 10.1016/j.tim.2022.04.006
Kolesnikov, S. I., Timoshenko, A. N., Kazeev, K. S., Akimenko, Y. V., and Myasnikova, M. A. (2019). Ecotoxicity of copper, nickel, and zinc nanoparticles assessment on the basis of biological indicators of chernozems. Eurasian Soil Sci. 52, 982–987. doi: 10.1134/S106422931908009X
Kumar, P., Burman, U., and Kaul, R. K. (2018). Ecological Risks of Nanoparticles: Effect on Soil Microorganisms. Amsterdam: Elsevier. doi: 10.1016/B978-0-12-811487-2.00019-0
Lanfranco, L., Bonfante, P., and Genre, A. (2017). “The mutualistic interaction between plants and arbuscular mycorrhizal fungi,” in The Fungal Kingdom, eds J. Heitman, B. J. Howlett, P. W. Crous, E. H. Stukenbrock, T. Y. James, and N. A. R. Gow (New York, NY: Wiley), 727–747. doi: 10.1128/9781555819583.ch35
Li, Y., Pan, F., and Yao, H. (2019). Response of symbiotic and asymbiotic nitrogen-fixing microorganisms to nitrogen fertilizer application. J. Soils Sediments 19, 1948–1958. doi: 10.1007/s11368-018-2192-z
Li, Z., Wen, W., Qin, M., He, Y., Xu, D., and Li, L. (2022). Biosynthetic mechanisms of secondary metabolites promoted by the interaction between endophytes and plant hosts. Front. Microbiol. 13:928967. doi: 10.3389/fmicb.2022.928967
Lopes, M. J. S., Dias-Filho, M. B., and Gurgel, E. S. C. (2021). Successful plant growth-promoting microbes: inoculation methods and abiotic factors. Front. Sustain. Food Syst. 5:606454. doi: 10.3389/fsufs.2021.606454
Ma, C., Li, Q., Jia, W., Shang, H., Zhao, J., Hao, Y., et al. (2021). Role of nanoscale hydroxyapatite in disease suppression of fusarium-infected tomato. Environ. Sci. Technol. 55, 13465–13476. doi: 10.1021/acs.est.1c00901
Mahil, E. I. T., and Kumar, B. N. A. (2019). Foliar application of nanofertilizers in agricultural crops -a review Foliar application of nanofertilizers in agricultural crops – a review. J. Farm Sci. 32, 239–249.
Mansor, M., and Xu, J. (2020). Benefits at the nanoscale: a review of nanoparticle-enabled processes favouring microbial growth and functionality. Environ. Microbiol. 22, 3633–3649. doi: 10.1111/1462-2920.15174
Martínez-Rodríguez, J. D. C., De La Mora-Amutio, M., Plascencia-Correa, L. A., Audelo-Regalado, E., Guardado, F. R., Hernández-Sánchez, E., et al. (2014). Cultivable endophytic bacteria from leaf bases of Agave tequilana and their role as plant growth promoters. Brazilian J. Microbiol. 45, 1333–1339. doi: 10.1590/S1517-83822014000400025
Merghany, M. M., Shahein, M. M., Sliem, M. A., Abdelgawad, K. F., and Radwan, A. F. (2019). Effect of nano-fertilizers on cucumber plant growth, fruit yield and it’ s quality. Plant Arch. 19, 165–172.
Moll, J., Klingenfuss, F., Widmer, F., Gogos, A., Bucheli, T. D., Hartmann, M., et al. (2017). Effects of titanium dioxide nanoparticles on soil microbial communities and wheat biomass. Soil Biol. Biochem. 111, 85–93. doi: 10.1016/j.soilbio.2017.03.019
Mosquera, J., García, I., and Liz-Marzán, L. M. (2018). Cellular uptake of nanoparticles versus small molecules: a matter of size. Acc. Chem. Res. 51, 2305–2313. doi: 10.1021/acs.accounts.8b00292
Munir, N., Hanif, M., Abideen, Z., Sohail, M., El-Keblawy, A., Radicetti, E., et al. (2022). Mechanisms and strategies of plant microbiome interactions to mitigate abiotic stresses. Agronomy 12, 1–33. doi: 10.3390/agronomy12092069
Nair, D. N., and Padmavathy, S. (2014). Impact of endophytic microorganisms on plants, environment and humans. Sci. World J. 2014:250693. doi: 10.1155/2014/250693
Nair, P. M. G., and Chung, I. M. (2014). A mechanistic study on the toxic effect of copper oxide nanoparticles in soybean (Glycine max L.) root development and lignification of root cells. Biol. Trace Elem. Res. 162, 342–352. doi: 10.1007/s12011-014-0106-5
Narayanan, N., Gupta, S., Gajbhiye, V. T., and Manjaiah, K. M. (2017). Optimization of isotherm models for pesticide sorption on biopolymer-nanoclay composite by error analysis. Chemosphere 173, 502–511. doi: 10.1016/j.chemosphere.2017.01.084
Nascimento, F. X., Tavares, M. J., Franck, J., Ali, S., Glick, B. R., and Rossi, M. J. (2019). ACC deaminase plays a major role in Pseudomonas fluorescens YsS6 ability to promote the nodulation of Alpha- and Betaproteobacteria rhizobial strains. Arch. Microbiol. 201, 817–822. doi: 10.1007/s00203-019-01649-5
Naseer, I., Ahmad, M., Nadeem, S. M., Ahmad, I., and Zahir, Z. A. (2019). “Rhizobial inoculants for sustainable agriculture: prospects and applications,” in Biofertilizers for Sustainable Agriculture Environment, eds B. Giri, R. Prasad, Q.-S. Wu, and A. Varma (Berlin: Springer). doi: 10.1007/978-3-030-18933-4_11
Nath, S., Kaittanis, C., Tinkham, A., and Perez, J. M. (2008). Dextran-coated gold nanoparticles for the assessment of antimicrobial susceptibility. Anal. Chem. 80, 1033–1038. doi: 10.1021/ac701969u
Nawaz, S., and Bano, A. (2019). Effects of PGPR (Pseudomonas sp.) and Ag-nanoparticles on enzymatic activity and physiology of cucumber. Recent Pat. Food Nutr. Agric. 11, 124–136. doi: 10.2174/2212798410666190716162340
Ndaba, B., Roopnarain, A., Rama, H., and Maaza, M. (2022). Biosynthesized metallic nanoparticles as fertilizers: an emerging precision agriculture strategy. J. Integr. Agric. 21, 1225–1242. doi: 10.1016/S2095-3119(21)63751-6
Obieze, C. C., Chikere, C. B., Adeleke, R., Selvarajan, R., Ntushelo, K., and Akaranta, O. (2022). Field-scale biostimulation shifts microbial community composition and improves soil pollution recovery at an artisanal crude oil refining site. Int. J. Environ. Stud. 1–20. doi: 10.1080/00207233.2021.2017198
Okey-Onyesolu, C. F., Hassanisaadi, M., Bilal, M., Barani, M., Rahdar, A., Iqbal, J., et al. (2021). Nanomaterials as nanofertilizers and nanopesticides: an overview. ChemistrySelect 6, 8645–8663. doi: 10.1002/slct.202102379
Oliveira, C. R., Domingues, C. E. C., de Melo, N. F. S., Roat, T. C., Malaspina, O., Jones-Costa, M., et al. (2019). Nanopesticide based on botanical insecticide pyrethrum and its potential effects on honeybees. Chemosphere 236:124282. doi: 10.1016/j.chemosphere.2019.07.013
Orozco-Mosqueda, M. C., and Santoyo, G. (2021). Plant-microbial endophytes interactions: scrutinizing their beneficial mechanisms from genomic explorations. Curr. Plant Biol. 25:100189. doi: 10.1016/j.cpb.2020.100189
Patel, J. K., and Archana, G. (2017). Diverse culturable diazotrophic endophytic bacteria from Poaceae plants show cross-colonization and plant growth promotion in wheat. Plant Soil 417, 99–116. doi: 10.1007/s11104-017-3244-7
Peixoto, S., Henriques, I., and Loureiro, S. (2021). Long-term effects of Cu(OH)2 nanopesticide exposure on soil microbial communities. Environ. Pollut. 269:116113. doi: 10.1016/j.envpol.2020.116113
Peng, C., Tong, H., Shen, C., Sun, L., Yuan, P., He, M., et al. (2020). Bioavailability and translocation of metal oxide nanoparticles in the soil-rice plant system. Sci. Total Environ. 713:136662. doi: 10.1016/j.scitotenv.2020.136662
Philippot, L., Raaijmakers, J. M., Lemanceau, P., and Van Der Putten, W. H. (2013). Going back to the roots: the microbial ecology of the rhizosphere. Nat. Rev. Microbiol. 11, 789–799. doi: 10.1038/nrmicro3109
Pimentel, M. R., Molina, G., Dionísio, A. P., Maróstica Junior, M. R., and Pastore, G. M. (2011). The use of endophytes to obtain bioactive compounds and their application in biotransformation process. Biotechnol. Res. Int. 2011:576286. doi: 10.4061/2011/576286
Poveda, J., Eugui, D., Abril-Urías, P., and Velasco, P. (2021). Endophytic fungi as direct plant growth promoters for sustainable agricultural production. Symbiosis 85, 1–19. doi: 10.1007/s13199-021-00789-x
Rabalao, T. M., Ndaba, B., Roopnarain, A., and Vatsha, B. (2022). Towards a circular economy: the influence of extraction methods on phytosynthesis of metallic nanoparticles and their impact on crop growth and protection. JSFA Rep. 2, 208–221. doi: 10.1002/jsf2.44
Raimi, A., and Adeleke, R. (2023). 16S rRNA gene-based identification and plant growth-promoting potential of cultivable endophytic bacteria. Agron. J. 115, 1447–1462. doi: 10.1002/agj2.21241
Raimi, A., Adeleke, R., and Roopnarain, A. (2017). Soil fertility challenges and biofertiliser as a viable alternative for increasing smallholder farmer crop productivity in sub-Saharan Africa. Cogent Food Agric. 3:1400933. doi: 10.1080/23311932.2017.1400933
Rajput, V. D., Minkina, T., Sushkova, S., Tsitsuashvili, V., Mandzhieva, S., Gorovtsov, A., et al. (2018). Effect of nanoparticles on crops and soil microbial communities. J. Soils Sediments 18, 2179–2187. doi: 10.1007/s11368-017-1793-2
Raliya, R., Saharan, V., Dimkpa, C., and Biswas, P. (2018). Nanofertilizer for precision and sustainable agriculture: current state and future perspectives. J. Agric. Food Chem. 66, 6487–6503. doi: 10.1021/acs.jafc.7b02178
Ramírez Aguirre, D. P., Flores Loyola, E., De la Fuente Salcido, N. M., Rodríguez Sifuentes, L., Ramírez Moreno, A., and Marszalek, J. E. (2020). Comparative antibacterial potential of silver nanoparticles prepared via chemical and biological synthesis. Arab. J. Chem. 13, 8662–8670. doi: 10.1016/j.arabjc.2020.09.057
Rangaraj, S., Gopalu, K., Rathinam, Y., Periasamy, P., Venkatachalam, R., and Narayanasamy, K. (2014). Effect of silica nanoparticles on microbial biomass and silica availability in maize rhizosphere. Biotechnol. Appl. Biochem. 61, 668–675. doi: 10.1002/bab.1191
Rashid, M. I., Shahzad, T., Shahid, M., Ismail, I. M. I., Shah, G. M., and Almeelbi, T. (2017). Zinc oxide nanoparticles affect carbon and nitrogen mineralization of Phoenix dactylifera leaf litter in a sandy soil. J. Hazard. Mater. 324, 298–305. doi: 10.1016/j.jhazmat.2016.10.063
Rawat, S., Cota-Ruiz, K., Dou, H., Pullagurala, V. L. R., Zuverza-Mena, N., White, J. C., et al. (2021). Soil-weathered cuo nanoparticles compromise foliar health and pigment production in spinach (spinacia oleracea). Environ. Sci. Technol. 55, 13504–13512. doi: 10.1021/acs.est.0c06548
Revanappa, S., and Pramod, N. (2015). Effect of nano-zinc oxide on the leaf physical and nutritional quality of spinach. Agrotechnology 5:135. doi: 10.4172/2168-9881.1000135
Saeed, Q., Xiukang, W., Haider, F. U., Kučerik, J., Mumtaz, M. Z., Holatko, J., et al. (2021). Rhizosphere bacteria in plant growth promotion, biocontrol, and bioremediation of contaminated sites: a comprehensive review of effects and mechanisms. Int. J. Mol. Sci. 22:10529. doi: 10.3390/ijms221910529
Sánchez-Quiles, D., and Tovar-Sánchez, A. (2014). Sunscreens as a source of hydrogen peroxide production in coastal waters. Environ. Sci. Technol. 48, 9037–9042. doi: 10.1021/es5020696
Santoyo, G., del Orozco-Mosqueda, M. C., and Govindappa, M. (2012). Mechanisms of biocontrol and plant growth-promoting activity in soil bacterial species of Bacillus and Pseudomonas: a review. Biocontrol Sci. Technol. 22, 855–872. doi: 10.1080/09583157.2012.694413
Saunders, M., Glenn, A. E., and Kohn, L. M. (2010). Exploring the evolutionary ecology of fungal endophytes in agricultural systems: using functional traits to reveal mechanisms in community processes. Evol. Appl. 3, 525–537. doi: 10.1111/j.1752-4571.2010.00141.x
Shah, V., Collins, D., Walker, V. K., and Shah, S. (2014). The impact of engineered cobalt, iron, nickel and silver nanoparticles on soil bacterial diversity under field conditions. Environ. Res. Lett. 9:24001. doi: 10.1088/1748-9326/9/2/024001
Shang, H., Ma, C., Li, C., Zhao, J., Elmer, W., White, J. C., et al. (2021). Copper oxide nanoparticle-embedded hydrogels enhance nutrient supply and growth of lettuce (Lactuca sativa) infected with Fusarium oxysporum f. sp. lactucae. Environ. Sci. Technol. 55, 13432–13442. doi: 10.1021/acs.est.1c00777
Sillen, W. M. A., Thijs, S., Abbamondi, G. R., De La Torre Roche, R., and Weyens, N. (2020). Nanoparticle treatment of maize analyzed through the metatranscriptome: compromised nitrogen cycling, possible phytopathogen selection, and plant hormesis. Microbiome 8:127. doi: 10.1186/s40168-020-00904-y
Sindhu, R. K., Chitkara, M., Sandhu, I. S., and Malik, P. (2020). “Nanofertilizers: applications and future prospects,” in Nanotechnology, eds R K. Sindhu, M Chitkara, and I S Sandhu. New York, NY: Jenny Stanford Publishing doi: 10.1201/9781003120261
Singh, J., Vishwakarma, K., Ramawat, N., Rai, P., Singh, V. K., Mishra, R. K., et al. (2019). Nanomaterials and microbes’ interactions: a contemporary overview. 3 Biotech 9:68. doi: 10.1007/s13205-019-1576-0
Smith, K., Evans, D. A., and El-Hiti, G. A. (2008). Role of modern chemistry in sustainable arable crop protection. Philos. Trans. R. Soc. B Biol. Sci. 363, 623–637. doi: 10.1098/rstb.2007.2174
Soumare, A., Diedhiou, A. G., Thuita, M., and Hafidi, M. (2020). Exploiting biological nitrogen fixation: a route. Plants 9:1011. doi: 10.3390/plants9081011
Sułowicz, S., Markowicz, A., Dulski, M., Nowak, A., Środek, D., and Borymski, S. (2023). Assessment of the ecotoxicological impact of captan@ZnO35–45nm and captan@SiO2 20–30nm nanopesticide on non-target soil microorganisms – a 100-day case study. Appl. Soil Ecol. 184:104789. doi: 10.1016/j.apsoil.2022.104789
Sun, H., He, Y., Xiao, Q., Ye, R., and Tian, Y. (2013). Isolation, characterization, and antimicrobial activity of endophytic bacteria from Polygonum cuspidatum. African J. Microbiol. Res. 7, 1496–1504. doi: 10.5897/AJMR12.899
Sun, H., Peng, Q., Guo, J., Zhang, H., Bai, J., and Mao, H. (2022). Effects of short-term soil exposure of different doses of ZnO nanoparticles on the soil environment and the growth and nitrogen fixation of alfalfa. Environ. Pollut. 309:119817. doi: 10.1016/j.envpol.2022.119817
Suriyaprabha, R., Karunakaran, G., Yuvakkumar, R., Prabu, P., Rajendran, V., and Kannan, N. (2012). Growth and physiological responses of maize (Zea mays L.) to porous silica nanoparticles in soil. J. Nanoparticle Res. 14:1294. doi: 10.1007/s11051-012-1294-6
Surjit, S. D., and Rupa, G. (2014). Beneficial properties, colonization, establishment and molecular diversity of endophytic bacteria in legumes and non legumes. African J. Microbiol. Res. 8, 1562–1572. doi: 10.5897/AJMR2013.6541
Taghavi, S. M., Momenpour, M., Azarian, M., Ahmadian, M., Souri, F., Taghavi, S. A., et al. (2013). Effects of nanoparticles on the environment and outdoor workplaces. Electron. Physician 5, 706–712.
Tapia-García, E. Y., Hernández-Trejo, V., Guevara-Luna, J., Rojas-Rojas, F. U., Arroyo-Herrera, I., Meza-Radilla, G., et al. (2020). Plant growth-promoting bacteria isolated from wild legume nodules and nodules of Phaseolus vulgaris L. trap plants in central and southern Mexico. Microbiol. Res. 239:126522. doi: 10.1016/j.micres.2020.126522
Thakral, F., Bhatia, G. K., Tuli, H. S., Sharma, A. K., and Sood, S. (2021). Zinc oxide nanoparticles: from biosynthesis, characterization, and optimization to synergistic antibacterial potential. Curr. Pharmacol. Reports 7, 15–25. doi: 10.1007/s40495-021-00248-7
Thuesombat, P., Hannongbua, S., Akasit, S., and Chadchawan, S. (2014). Effect of silver nanoparticles on rice (Oryza sativa L. cv. KDML 105) seed germination and seedling growth. Ecotoxicol. Environ. Saf. 104, 302–309. doi: 10.1016/j.ecoenv.2014.03.022
Tian, B., Zhang, C., Ye, Y., Wen, J., Wu, Y., Wang, H., et al. (2017). Beneficial traits of bacterial endophytes belonging to the core communities of the tomato root microbiome. Agric. Ecosyst. Environ. 247, 149–156. doi: 10.1016/j.agee.2017.06.041
Toksha, B., Sonawale, V. A. M., Vanarase, A., Bornare, D., Tonde, S., Hazra, C., et al. (2021). Nanofertilizers: a review on synthesis and impact of their use on crop yield and environment. Environ. Technol. Innov. 24:101986. doi: 10.1016/j.eti.2021.101986
Trivedi, P., Mattupalli, C., Eversole, K., and Leach, J. E. (2021). Enabling sustainable agriculture through understanding and enhancement of microbiomes. New Phytol. 230, 2129–2147. doi: 10.1111/nph.17319
Tudi, M., Ruan, H. D., Wang, L., Lyu, J., Sadler, R., Connell, D., et al. (2021). Agriculture development, pesticide application and its impact on the environment. Int. J. Environ. Res. Public Health 18, 1–24. doi: 10.3390/ijerph18031112
Vischetti, C., Casucci, C., De Bernardi, A., Monaci, E., Tiano, L., Marcheggiani, F., et al. (2020). Sub-lethal effects of pesticides on the DNA of soil organisms as early ecotoxicological biomarkers. Front. Microbiol. 11:1892. doi: 10.3389/fmicb.2020.01892
Walker, R., Otto-Pille, C., Gupta, S., Schillaci, M., and Roessner, U. (2020). Current perspectives and applications in plant probiotics. Microbiol. Aust. 41, 95–99. doi: 10.1071/MA20024
Wang, D., Saleh, N. B., Byro, A., Zepp, R., Sahle-Demessie, E., Luxton, T. P., et al. (2022). Nano-enabled pesticides for sustainable agriculture and global food security. Nat. Nanotechnol. 17, 347–360. doi: 10.1038/s41565-022-01082-8
Wang, Z., Yue, L., Dhankher, O. P., and Xing, B. (2020). Nano-enabled improvements of growth and nutritional quality in food plants driven by rhizosphere processes. Environ. Int. 142:105831. doi: 10.1016/j.envint.2020.105831
Weir, A., Westerhoff, P., Fabricius, L., Hristovski, K., and Von Goetz, N. (2012). Titanium dioxide nanoparticles in food and personal care products. Environ. Sci. Technol. 46, 2242–2250. doi: 10.1021/es204168d
Xu, C., Peng, C., Sun, L., Zhang, S., Huang, H., Chen, Y., et al. (2015). Distinctive effects of TiO2 and CuO nanoparticles on soil microbes and their community structures in flooded paddy soil. Soil Biol. Biochem. 86, 24–33. doi: 10.1016/j.soilbio.2015.03.011
Xu, J., Luo, X., Wang, Y., and Feng, Y. (2018). Evaluation of zinc oxide nanoparticles on lettuce (Lactuca sativa L.) growth and soil bacterial community. Environ. Sci. Pollut. Res. 25, 6026–6035. doi: 10.1007/s11356-017-0953-7
Yadav, J., Jasrotia, P., Kashyap, P. L., Bhardwaj, A. K., Kumar, S., Singh, M., et al. (2022). Nanopesticides: current status and scope for their application in agriculture. Plant Prot. Sci. 58, 1–17. doi: 10.17221/102/2020-PPS
Yadav, S. A., Suvathika, G., Alghuthaymi, M. A., and Abd-Elsalam, K. A. (2022). Fungal-Derived Nanoparticles for the Control of Plant Pathogens and Pests. Amsterdam: Elsevier. doi: 10.1016/B978-0-323-99922-9.00009-X
You, T., Liu, D., Chen, J., Yang, Z., Dou, R., Gao, X., et al. (2018). Effects of metal oxide nanoparticles on soil enzyme activities and bacterial communities in two different soil types. J. Soils Sediments 18, 211–221. doi: 10.1007/s11368-017-1716-2
Zain, M., Ma, H., Ur Rahman, S., Nuruzzaman, M., Chaudhary, S., Azeem, I., et al. (2024). Nanotechnology in precision agriculture: advancing towards sustainable crop production. Plant Physiol. Biochem. 206:108244. doi: 10.1016/j.plaphy.2023.108244
Zambryski, P. (2004). Cell-to-cell transport of proteins and fluorescent tracers via plasmodesmata during plant development. J. Cell Biol. 164, 165–168. doi: 10.1083/jcb.200310048
Zhai, Y., Abdolahpur Monikh, F., Wu, J., Grillo, R., Arenas-Lago, D., Darbha, G. K., et al. (2020). Interaction between a nano-formulation of atrazine and rhizosphere bacterial communities: atrazine degradation and bacterial community alterations. Environ. Sci. Nano 7, 3372–3384. doi: 10.1039/D0EN00638F
Zhang, H., Huang, M., Zhang, W., Gardea-Torresdey, J. L., White, J. C., Ji, R., et al. (2020). Silver nanoparticles alter soil microbial community compositions and metabolite profiles in unplanted and cucumber-planted soils. Environ. Sci. Technol. 54, 3334–3342. doi: 10.1021/acs.est.9b07562
Zhang, N., Wang, D., Liu, Y., Li, S., Shen, Q., and Zhang, R. (2014). Effects of different plant root exudates and their organic acid components on chemotaxis, biofilm formation and colonization by beneficial rhizosphere-associated bacterial strains. Plant Soil 374, 689–700. doi: 10.1007/s11104-013-1915-6
Zhang, Q., Li, Y., and Xia, L. (2014). An oleaginous endophyte Bacillus subtilis HB1310 isolated from thin-shelled walnut and its utilization of cotton stalk hydrolysate for lipid production. Biotechnol. Biofuels 7:152. doi: 10.1186/s13068-014-0152-4
Zhang, W., Chen, Y., Huang, K., Wang, F., and Mei, Z. (2023). Molecular mechanism and agricultural application of the NifA–NifL system for nitrogen fixation. Int. J. Mol. Sci. 24:907. doi: 10.3390/ijms24020907
Zhang, X., Zhou, Y., Xu, T., Zheng, K., Zhang, R., Peng, Z., et al. (2018). Toxic effects of CuO, ZnO and TiO2 nanoparticles in environmental concentration on the nitrogen removal, microbial activity and community of Anammox process. Chem. Eng. J. 332, 42–48. doi: 10.1016/j.cej.2017.09.072
Zhao, L., Huang, Y., Adeleye, A. S., and Keller, A. A. (2017). Metabolomics reveals Cu(OH)2 nanopesticide-activated anti-oxidative pathways and decreased beneficial antioxidants in spinach leaves. Environ. Sci. Technol. 51, 10184–10194. doi: 10.1021/acs.est.7b02163
Zhu, B., Tian, W. X., Fan, X. Y., Li, B., Zhou, X. P., Jin, G. L., et al. (2012). Genome sequence of Stenotrophomonas maltophilia RR-10, isolated as an endophyte from rice root. J. Bacteriol. 194, 1280–1281. doi: 10.1128/JB.06702-11
Keywords: agriculture, rhizosphere, endosphere, nanoparticles, microorganisms
Citation: Mgadi K, Ndaba B, Roopnarain A, Rama H and Adeleke R (2024) Nanoparticle applications in agriculture: overview and response of plant-associated microorganisms. Front. Microbiol. 15:1354440. doi: 10.3389/fmicb.2024.1354440
Received: 12 December 2023; Accepted: 30 January 2024;
Published: 06 March 2024.
Edited by:
Ji-Hoon Lee, Jeonbuk National University, Republic of KoreaReviewed by:
Solabomi Olaitan Ogunyemi, Zhejiang University, ChinaHemraj Chhipa, Agriculture University, Kota, India
Copyright © 2024 Mgadi, Ndaba, Roopnarain, Rama and Adeleke. This is an open-access article distributed under the terms of the Creative Commons Attribution License (CC BY). The use, distribution or reproduction in other forums is permitted, provided the original author(s) and the copyright owner(s) are credited and that the original publication in this journal is cited, in accordance with accepted academic practice. No use, distribution or reproduction is permitted which does not comply with these terms.
*Correspondence: Busiswa Ndaba, ndabab@arc.agric.za; Rasheed Adeleke, rasheed.adeleke@nwu.ac.za