- Department of Comparative Biomedical Sciences, College of Veterinary Medicine, Mississippi State University, Mississippi, MS, United States
Listeria monocytogenes causes listeriosis, an infectious and potentially fatal disease of animals and humans. A diverse network of transcriptional regulators, including LysR-type catabolite control protein C (CcpC), is critical for the survival of L. monocytogenes and its ability to transition into the host environment. In this study, we explored the physiological and genetic consequences of deleting ccpC and the effects of such deletion on the ability of L. monocytogenes to cause disease. We found that ccpC deletion did not impact hemolytic activity, whereas it resulted in significant reductions in phospholipase activities. Western blotting revealed that the ΔccpC strain produced significantly reduced levels of the cholesterol-dependent cytolysin LLO relative to the wildtype F2365 strain. However, the ΔccpC mutant displayed no significant intracellular growth defect in macrophages. Furthermore, ΔccpC strain exhibited reduction in plaque numbers in fibroblasts compared to F2365, but plaque size was not significantly affected by ccpC deletion. In a murine model system, the ΔccpC strain exhibited a significantly reduced bacterial burden in the liver and spleen compared to the wildtype F2365 strain. Interestingly, the deletion of this gene also enhanced the survival of L. monocytogenes under conditions of H2O2-induced oxidative stress. Transcriptomic analyses performed under H2O2-induced oxidative stress conditions revealed that DNA repair, cellular responses to DNA damage and stress, metalloregulatory proteins, and genes involved in the biosynthesis of peptidoglycan and teichoic acids were significantly induced in the ccpC deletion strain relative to F2365. In contrast, genes encoding internalin, 1-phosphatidylinositol phosphodiesterase, and genes associated with sugar-specific phosphotransferase system components, porphyrin, branched-chain amino acids, and pentose phosphate pathway were significantly downregulated in the ccpC deletion strain relative to F2365. This finding highlights CcpC as a key factor that regulates L. monocytogenes physiology and responses to oxidative stress by controlling the expression of important metabolic pathways.
1 Introduction
Listeria monocytogenes is a foodborne pathogen responsible for listeriosis, which is characterized by high hospitalization and fatality rates (Drevets and Bronze, 2008; Eallonardo et al., 2023). In healthy individuals, listeriosis generally manifests in the form of noninvasive gastroenteritis, whereas immunocompromised individuals can experience severe outcomes including septicemia, abortion, and neurological disorders such as meningoencephalitis (Buchanan et al., 2017). The ability of L. monocytogenes to cause diseases is attributable to a coordinated series of virulence activities mostly regulated by the pleiotropic transcriptional activator, PrfA (Positive Regulatory Factor A) (Lampidis et al., 1994). PrfA serves as a master virulence factor responsible for controlling the transcription of several virulence factors, including phosphatidylinositol-specific phospholipase C (plcA), the cholesterol-dependent cytolysin LLO (hly), the zinc metalloproteinase Mpl (mpl), the actin assembly-inducing protein ActA (actA), phosphatidylcholine phospholipase C (plcB), and internalins A and B (inlA and inlB). These gene clusters are integral for host cell invasion, intracellular growth and replication, and the cell-to-cell spread of L. monocytogenes (Camejo et al., 2011; Kanki et al., 2018; Wiktorczyk-Kapischke et al., 2023).
Listeria monocytogenes is considered a ubiquitous microorganism that can adapt, survive, and even grow in a wide variety of habitats under various environmental stress conditions (Gray et al., 2021; Lakicevic et al., 2022; Osek et al., 2022). A large network of complex transcriptional regulators enables L. monocytogenes to rapidly respond and adapt to diverse settings including extracellular, abiotic, and intracellular environments (Xayarath and Freitag, 2012). Among these networks of regulators, LysR-type transcriptional regulators (LTTRs) have been reported to play roles in regulating gene expression in response to environmental changes and various stressors (Reniere et al., 2015; Biswas et al., 2020). LTTRs control genes involved in cellular metabolism, pathogen virulence, cell wall production, flagellar attachment/modification, pathogen motility, quorum sensing, stress responses, and toxin production and secretion (Cao et al., 2001; Russell et al., 2004; Maddocks and Oyston, 2008; Zhang et al., 2018).
The catabolite control protein C (CcpC) is an uncharacterized LTTR in L. monocytogenes, encoded by the ccpC gene (Mittal et al., 2013). The ccpC gene is flanked upstream by cbpB gene encoding a protein with tandem cystathionine-β-synthase (CBS) domains that binds c-di-AMP and contributes to solute importation (Kim et al., 2002; Huynh and Woodward, 2016). Downstream, ccpC is flanked by the dapD gene, which encodes a protein involved in diaminopimelate and lysine biosynthesis (Kim et al., 2006). In L. monocytogenes, CcpC plays an integral role in controlling genes encoding enzymes involved in the tricarboxylic acid (TCA) cycle including citrate synthase (citZ), aconitase (citB), and isocitrate dehydrogenase (citC) in response to the intracellular concentration of citrate (Kim et al., 2006; Mittal et al., 2013). The promoter regions of the citZ and citB genes are potential binding sites for CcpC, leading to the disruption of citrate synthesis by preventing read-through transcription (Mittal et al., 2013). The presence of citrate inhibits the interaction of CcpC with these promoter regions (Kim et al., 2002; Pechter et al., 2013). While the role that CcpC plays in metabolism and the regulation of the TCA cycle is known, information on the contributions of CcpC to pathogenesis is limited. The goal of this study was thus to investigate the role of CcpC in virulence and intracellular survival of L. monocytogenes, to evaluate the impact of CcpC deletion on biofilm formation and survival of L. monocytogenes under different stress conditions, and to identify associated regulons.
2 Materials and methods
2.1 Bacterial strains and growth conditions
Listeria monocytogenes F2365 4b strains were grown in brain heart infusion (BHI) broth and agar (Difco) at 37°C. Escherichia coli DH5α strain was grown in Luria-Bertani (LB) (Difco Laboratories) broth and agar (Table 1). Macrophage J774A and fibroblast (CRL-2648; ATCC) cell lines were grown in Dulbecco’s modified Eagle’s medium (DMEM) (ATCC, Manassas, VA) supplemented with 10% fetal bovine serum (FBS) (Atlanta Biologicals, Norcross, GA) and 1% glutamine. Cultures were maintained at 37°C with 5% CO2 under humidified conditions. Furthermore, BHI broth supplemented with 1% glucose (Sigma-Aldrich) was used to determine biofilm formation. Antibiotics such as erythromycin (20 μg/mL), ampicillin (50 μg/mL), kanamycin (50 μg/mL), and neomycin (20 μg/mL) were used for mutant and complemented strain construction while gentamicin (20 μg/mL) was used for cell line culturing. Brilliance Listeria agar (BLA) supplemented with lecithin (Oxoid) was used to detect phospholipase activity of bacterial strains.
2.2 Construction of ∆ccpC and complemented strains
The catabolite control protein C (ccpC) gene was targeted for in-frame deletion from L. monocytogenes F2365 using an allelic exchange technique as previously described (Abdelhamed et al., 2015). Four primers (A, B, C, and D) were designed for amplification of upstream (A and B) and downstream (C and D) regions of the ccpC gene using PCR (Table 1). The upstream and downstream amplicons were ligated through overlap extension PCR using A and D primers. This was followed by cloning into pHoss1 plasmid using E. coli DH5α. The resulting plasmid was transformed into F2365 by electroporation for integration by homologous recombination. The ccpC deletion was confirmed by PCR and sequencing. A complementation strain was made by amplifying a DNA fragment containing the entire ccpC gene and its promoter from the F2365 genome and ligating it into pPL2 shuttle integration vector (Lauer et al., 2002). The resulting plasmid was electroporated into ∆ccpC to obtain the complemented strain designated as F2365ΔccpC::pPL2-ccpC (C∆ccpC) (Table 1).
2.3 Hemolytic activity assays
The hemolytic activity of F2365, ΔccpC, and C∆ccpC strains was determined as previously described (Alonzo et al., 2009). Briefly, overnight cultures of bacterial strains were diluted in 1:10 in BHI broth and grown at 37°C for 4 h to an OD600 of approximately 0.7. Bacterial supernatants were obtained by centrifugation, and 500 μL of 1% sheep RBC (in activation buffer) was added and incubated at 37°C for 1 h. After incubation, the supernatant was collected by centrifugation and transferred into 48 well plates. Hemolytic activities were quantified by measuring the absorbance at OD450 nm with SpectraMax M5 ELISA reader (Molecular Devices, Sunnyvale, CA, United States). All experiments were performed three times independently with three replicates in each.
2.4 Phospholipase activity assay
Phospholipase activity of F2365, ΔccpC, and C∆ccpC strains was tested using BLA supplemented with lecithin (Oxoid) as previously described (Blank et al., 2014). Briefly, bacteria were streaked onto the BLA and incubated for 48 h at 37°C, followed by measurement of the zone of opacity surrounding the bacterial growth. All experiments were performed three independent times with three replicates each.
2.5 Detection of Listeriolysin O protein levels in Listeria monocytogenes
The amount of LLO protein present in ΔccpC was compared to F2365 and C∆ccpC strains using Western blot with LLO polyclonal antibodies. Protein from samples was extracted as previously described, with modifications (Alonzo et al., 2009). Bacterial pellets were obtained by centrifugation and lysed with cell lysis buffer (50 mM Tris–HCl pH 8.0, 5% glycerol, 0.5% triton X-100, 2 mM PMSF, and 1.5 mM EDTA) at 4°C for 30 min. Sonication was performed shortly before addition of 20 μL/mL DNase followed by incubation at 4°C for 1 h, and supernatant was obtained by centrifugation. The protein was suspended into 4X Laemmli sample buffer containing β-mercaptoethanol. The samples were heated for 10 min at 100°C, followed by Western blotting. LLO (primary antibody) and HRP-conjugated goat anti-rabbit antibody were used to detect LLO expression in the protein extracts. P-60 antibody (primary antibody) and HRP-conjugated anti-mouse antibody (secondary antibody) was used for P60 expression as the control (Bubert et al., 1997).
2.6 Biofilm formation
Biofilm formation by ΔccpC and C∆ccpC was compared to the wildtype F2365 by static growth after crystal violet staining as described previously (Wakimoto et al., 2004). Briefly, overnight cultures were diluted 100-fold in BHI broth supplemented with 1% glucose (Sigma-Aldrich) and incubated in 48-well plates under static condition for 24, 48 and 72 h, including negative control. At the indicated time points, wells were gently washed with PBS, and adherent cells were stained with 0.1% crystal violet (Sigma-Aldrich) for 10 min at room temperature. Plates were rinsed with PBS, and the residual crystal violet was solubilized with 70% ethanol. Biofilm formation was quantified by measuring absorbance at 538 nm with a SpectraMax M5 ELISA reader (Molecular Devices, Sunnyvale, CA, United States). Biofilm formation was determined three independent times with eight replicates each.
2.7 Intracellular replication
Intracellular replication in macrophages by ΔccpC was compared to F2365 and C∆ccpC using a method previously described (Vargas García et al., 2015). Macrophages were seeded in 48-well tissue culture plates and confluent monolayers were infected with bacterial suspension in phosphate buffered solution (PBS) at multiplicity of infection (MOI) of approximately 1 to 10. After 1 h incubation, the cells were washed with PBS and incubated in DMEM containing a low dose of gentamicin (20 μg/mL) to kill extracellular bacteria. After 4 h incubation, cells were washed with PBS, lysed, and the released bacteria were resuspended before being plated on BHI agar. Bacterial colonies were counted, and CFU/mL calculated (log10). All infections were performed three independent times, and four replicates were performed for each infection.
2.8 Plaque formation
Plaque formation by ΔccpC was compared to F2365 and C∆ccpC using murine L2 fibroblast cells as described previously (Jones and Portnoy, 1994). Fibroblast monolayers were grown in 75 cm2 plastic flasks (Sigma-Aldrich) at 37°C under 5% CO2. Cells were seeded at a concentration of 106 cells/well in a 6-well tissue culture plate to form a confluent monolayer before inoculation with L. monocytogenes strains. After incubation for 1 h, DMEM containing 20 ug/mL gentamicin was added, and plates were incubated at 37°C and 5% CO2 for 4 days. Living cells were visualized by adding an additional overlay consisting of DMEM, 0.5% agarose, and 0.1% neutral red and incubated overnight. Plaque sizes and numbers were determined using a compound microscope with ImageJ, and scores were converted to percentages. This experiment was done three independent times with at least 3 replicates.
2.9 Quantitative real-time PCR analysis of gene transcription
The expression of six virulence genes, namely plcA, plcB, inlA, inlB, actA, and hly, was compared in wildtype F2365 and ΔccpC strains during growth in BHI. The wildtype F2365 and ΔccpC strains were grown in BHI broth overnight at 37°C and bacterial pellets were obtained by centrifugation at 15,000 × g for 10 min at 4°C. Total RNA was extracted using the FastRNA spin kit for microbes and the FastPrep-24 instrument (MP Biomedicals, Santa Ana, CA) by following the manufacturer’s instructions. For each strain, total RNA was isolated from 3 independent biological replicates. Genomic DNA was eliminated from the total RNA by using on-column DNase treatment with an RNase-free DNase set (Qiagen, Hilden, Germany). The quantity and quality of total RNA were analyzed using a NanoDrop ND-1000 spectrophotometer (Thermo Scientific, United States) by measuring the OD260/OD280 ratio. Extracted RNA was transcribed into complementary DNA (cDNA) by reverse transcriptase from total RNA, and the cDNA was then used as the template for the RT-qPCR. Primers were designed with IDT (Integrated DNA Technologies) software. The product of the first-strand cDNA synthesis was diluted 50 times before use. RT-qPCR was performed in a 20- μl reaction volume containing 5 μL of cDNA, 10 μL of SYBR green real-time PCR master mix (Roche Diagnostic GmbH, Mannheim, Germany), 0.6 μL of gene-specific primers (10 μM), and 3.8 μL water. Amplification and detection of specific products were performed with the Mx3000P real-time PCR system (Stratagene) with the following cycle profile: initial denaturation at 95°C for 10 min, 40 cycles of 95°C for 30 s, 55°C for 30 s, and 72°C for 1 min. The expression of each gene was normalized against the expression of the housekeeping gene, 16S rRNA, before comparative analysis. Further, DNA melting curve analysis at the end of each run ensured that the desired amplicon was detected and that no secondary products were amplified. For each gene, triplicate assays were done. Expression levels of the tested genes were quantified by the relative quantitative method (2−^∆∆CT). RT-qPCR was performed to validate 13 differentially expressed genes from RNA-seq data. Primers and gene information are listed in Table 1. RT-qPCR was performed on the same RNAs used for RNA-seq. cDNA synthesis and RT-qPCR were performed as described above.
2.10 Scanning electron microscopy
Scanning electron microscopy (SEM) was performed to observe biofilm formation following overnight growth in BHI at 37°C. The biofilm formation of L. monocytogenes ΔccpC and F2365 was studied with three replicates. Briefly, bacterial culture was gently centrifuged, washed with PBS, and fixed with fixative (2% paraformaldehyde, 2.5% glutaraldehyde, and 2 mM CaCl2 in 0.1 M sodium cacodylate buffer pH 7.4) for 2 h at room temperature. The samples were then washed twice in sodium-cacodylate buffer (0.1 M, pH 7.4) before post-fixation using 1% osmium tetroxide for 1 h Glass cover slides were placed into a sterile polystyrene 6 well plate. Then 1:10 suspension of poly L- Lysine was used to coat the cover slide. After drying and sterilization of the coated glass slide with UV light, aliquots of 2 mL bacterial suspension were inoculated in each well and incubated for 24 h. The non-adherent bacteria were removed by washing with sterile water. Then the adherent bacteria were transferred into a graded mixture of hexamethyldisilazane (HMDS) and ethanol (30, 50, 70, 80, 85, 95 and 100%), followed by a second 100% dehydration for 1 h. This was followed by overnight air-drying, mounting on metal stub using two-sided carbon sticky tape, coating by 45 nm of platinum in EMS Coater operations, and finally examining by SEM.
2.11 In vivo virulence in Swiss Webster mice
Approval was obtained from the Institutional Animal Care and Use Committee (IACUC) for animal procedures (18-508), and experiments were conducted at the College of Veterinary Medicine. Swiss Webster mice were obtained from Charles River laboratories and housed at 5 mice per cage. Virulence of the ΔccpC and C∆ccpC strains were compared to F2365 and negative controls in this study. Overnight culture of bacterial strains (OD600 of approximately 1.00) was diluted to a final concentration of 2 × 104 colony forming units (CFU)/mL and injected intravenously through tail vein (Bou Ghanem et al., 2012). At 72 h post-infection, mice were euthanized, and livers and spleens of infected animals were collected, homogenized with saline, and spread on agar plates for CFU determination. All experiments were performed two independent times.
2.12 Oxidative stress response
The oxidative stress response of ΔccpC and C∆ccpC strains was compared to F2365 using hydrogen peroxide (H2O2) as the oxidative stressor. Briefly, overnight cultures of bacterial strains were diluted 1:10 in BHI and incubated at 37°C with shaking for ~1 h to reach OD600 ~ 0.2–0.3. Pellets were obtained by centrifugation and resuspended in PBS. BHI containing hydrogen peroxide (H2O2) at 6, 8, and 10 mM were inoculated with the corresponding bacterial strain and used for bacterial enumerations and growth curves. Bacterial enumeration was performed by serially diluting 100 μL samples of the incubated BHI at 1-, 3-, 6-, and 24 h time points and spreading on BHI plates for colony count. Growth curves were conducted using a Cytation 5 Cell Imaging Multi-Mode Reader (BioTek) over 48 h. Oxidative stress response tests were conducted in at least 3 independent experiments with 6 replicates in each experiment.
2.13 RNA extraction, library preparation, and transcriptome sequencing
The wildtype F2365 and ΔccpC strains were observed for phenotypic changes under oxidative stress condition and prepared for transcriptomic analysis. Total RNA was extracted from bacterial cultures of F2365 and ΔccpC following exposure to 8 mM H2O2 for 2.5 h. RNA was extracted as described above in section 2.9. A Ribo-Zero magnetic kit for Gram-positive bacteria (Epicentre) was used to remove rRNAs, and then a fragmentation buffer was added to fragment mRNAs. Before library construction, the concentration of RNA was normalized using specific ScriptSeq kits (Epicentre). Library construction and sequencing was performed by Novogen©. Briefly, mRNA fragments were reverse transcribed to single-stranded cDNAs using random hexamers as primers (Promega). The cDNA libraries were subjected to sequencing using the HiSeq platform (Illumina). For each strain, three independent biological replicates were sequenced.
2.14 Sequence mapping, differential expression, and GO and KEGG pathway enrichment analyses
Raw data were filtered to remove reads containing adapters or low-quality reads. The resulting reads were mapped to the genome of L. monocytogenes F2365 using Bowtie2. Transcriptomic analysis was conducted using the Bioconductor Edge R analysis package. Transcripts for each sample were quantified and normalized as the number of reads per kilobase per million reads (RPKM). Three replicate RPKM values for each sample were standardized on the basis of their mean transcript values and used to assess gene expression and fold change differences in expression. To minimize false-positive results, a stringent cutoff false discovery rate (FDR) of 1 was applied when identifying differentially expressed genes of the wildtype compared to the mutant strains. GO enrichment analysis was conducted by GOseq (Young et al., 2010), which is based on Wallenius noncentral hypergeometric distribution. GO covers molecular functions, biological processes, and cellular components. The differentially expressed gene list was mapped to the Kyoto Encyclopedia of Genes and Genomes (KEGG) to identify significantly enriched metabolic pathways or signal transduction pathways.
2.15 Statistical analysis
Dot plots and median values of bacterial concentrations in each mouse tissue were generated using GraphPad Prism 9.0 software. For in vivo experiments, a nonparametric Mann–Whitney test was used to detect statistical significance between F2365, ΔccpC, and C∆ccpC treatment groups in bacterial concentrations in liver and spleen of infected mice. Statistical analysis of the hemolytic activity assay, intracellular replication, plaque scores, phospholipase activity, biofilm formation, and oxidative stress were done to compare ΔccpC and wildtype F2365 using Student t-test using GraphPad Prism 9.0 software. p values of < 0.05 were considered statistically significant in all analyses. ImageJ was used for quantification of bands from Western blot images, and statistical analysis was performed using Student t-test.
3 Results
3.1 CcpC influences the phospholipase activity of Listeria monocytogenes
Expression of phospholipase genes plcA and plcB and the LLO encoded by hly gene are controlled by PrfA following its activation in host cells (Moors et al., 1999; Seveau, 2014). To establish whether CcpC impacts PrfA-regulon function, the hemolytic activity of ΔccpC and F2365 was assessed by monitoring the lysis of sheep erythrocytes by bacterial supernatant (Nilsson and Nilsson, 1984). The ΔccpC strain exhibited a non-significant ~2% reduction in hemolytic activity compared to the wildtype F2365 (Figure 1A).
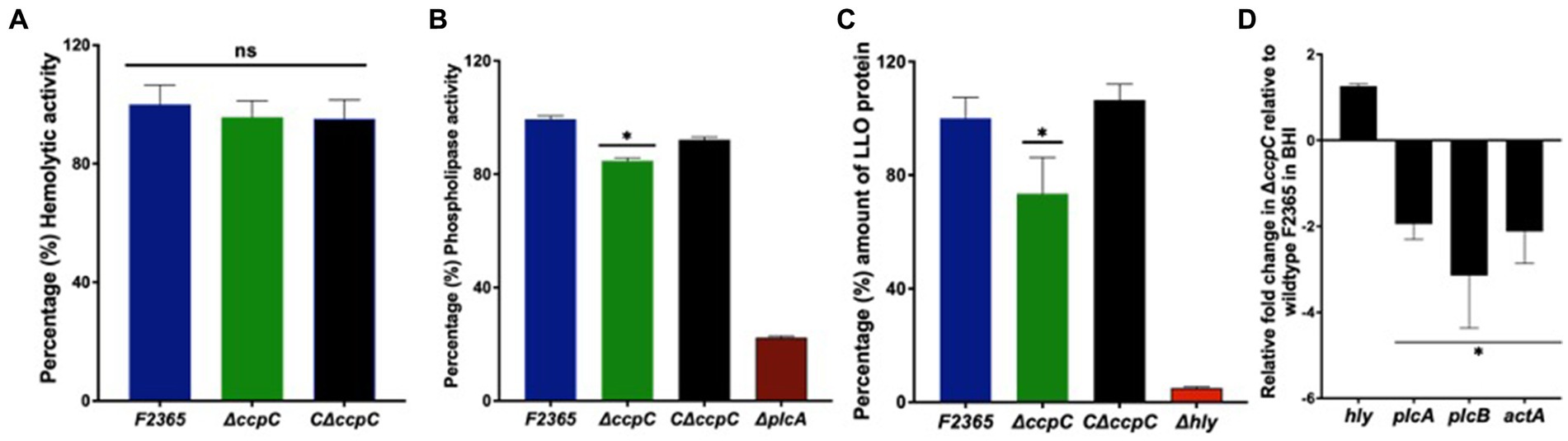
Figure 1. The hemolytic activity, phospholipase activity, and intracellular replication of the ΔccpC and wildtype F2365. (A) Percentages of hemolytic activity in sheep erythrocytes by ΔccpC strain showed non-significant ~2% reduction in hemolytic activity compared to the wildtype F2365 (p > 0.05). (B) The ΔccpC strain exhibited ~20% reduction in phospholipase activity compared to the wildtype F2365 strain when examined on BLA plates containing lecithin (p < 0.05). The C∆ccpC partially restored the phospholipase activity with opacity zone size similar to F2365. (C) The ΔccpC strain on western blot showed a significantly reduced LLO protein level (~25%) compared to the wildtype F2365 (p < 0.05), while the C∆ccpC restored the amount of LLO protein to the wildtype F2365 level. (D) The effect of ccpC deletion on the relative expression of plcA, plcB, actA, and hly during the growth of L. monocytogenes strains in BHI broth using RT-qPCR. The expression of plcA, plcB, and actA genes was significantly downregulated in the ΔccpC strain compared to the wildtype F2365 (p < 0.05), whereas hly gene was insignificantly upregulated (p > 0.05). Bars indicate the standard error of the mean (SEM) of six replicates. The data were compared with Student’s t-tests. Asterisks (*) indicate significant differences, p < 0.05. NS, No significant difference.
To characterize phospholipase activity, the lecithinase activity of the ΔccpC and wildtype strains was examined on BLA plates containing lecithin. After incubation at 37°C for 72 h, the mean opaque zone size surrounding the ∆ccpC colonies was about 20% smaller than that produced by the wildtype strain, indicating reduced secreted phospholipase activity. The C∆ccpC exhibited partially restored phospholipase activity with opacity zone size similar to F2365 (Figure 1B). Surprisingly, western blotting showed that LLO protein levels were significantly reduced by approximately 25% in the ΔccpC strain compared to the wildtype F2365 strain (Figure 1C). The LLO protein levels of the complemented C∆ccpC strain was similar to wildtype levels. Our results thus revealed a significant impact of the ccpC deletion on L. monocytogenes virulence factor expression. Furthermore, we examined the impact of ccpC deletion on the relative expression of plcA, plcB, actA, and hly during the growth of L. monocytogenes strains in BHI broth (Figure 1D). The plcA, plcB, and actA genes were significantly downregulated in the ΔccpC strain compared to the wildtype F2365, while the hly gene was not significantly different in the ΔccpC strain compared to the wildtype F2365.
3.2 CcpC is required for Listeria monocytogenes biofilm formation
The impact of ccpC deletion on biofilm formation was determined under static conditions at 24, 48, and 72 h using crystal violet staining. The ΔccpC strain exhibited significantly (p < 0.05) reduced biofilm formation compared to F2365 (set to 100%) by approximately 30, 20, and 35% at 24, 48, and 72 h (Figure 2A). Furthermore, scanning electron microscopy revealed that F2365 biofilm exhibited more pronounced complex three-dimensional architecture compared to ΔccpC. Wildtype strain biofilm was characterized by dense microcolonies embedded in a well-defined extracellular polymeric substance (EPS) matrix, unlike the ΔccpC strain, which exhibited individual and scattered bacterial cells with minimal extracellular materials (Figure 2B).
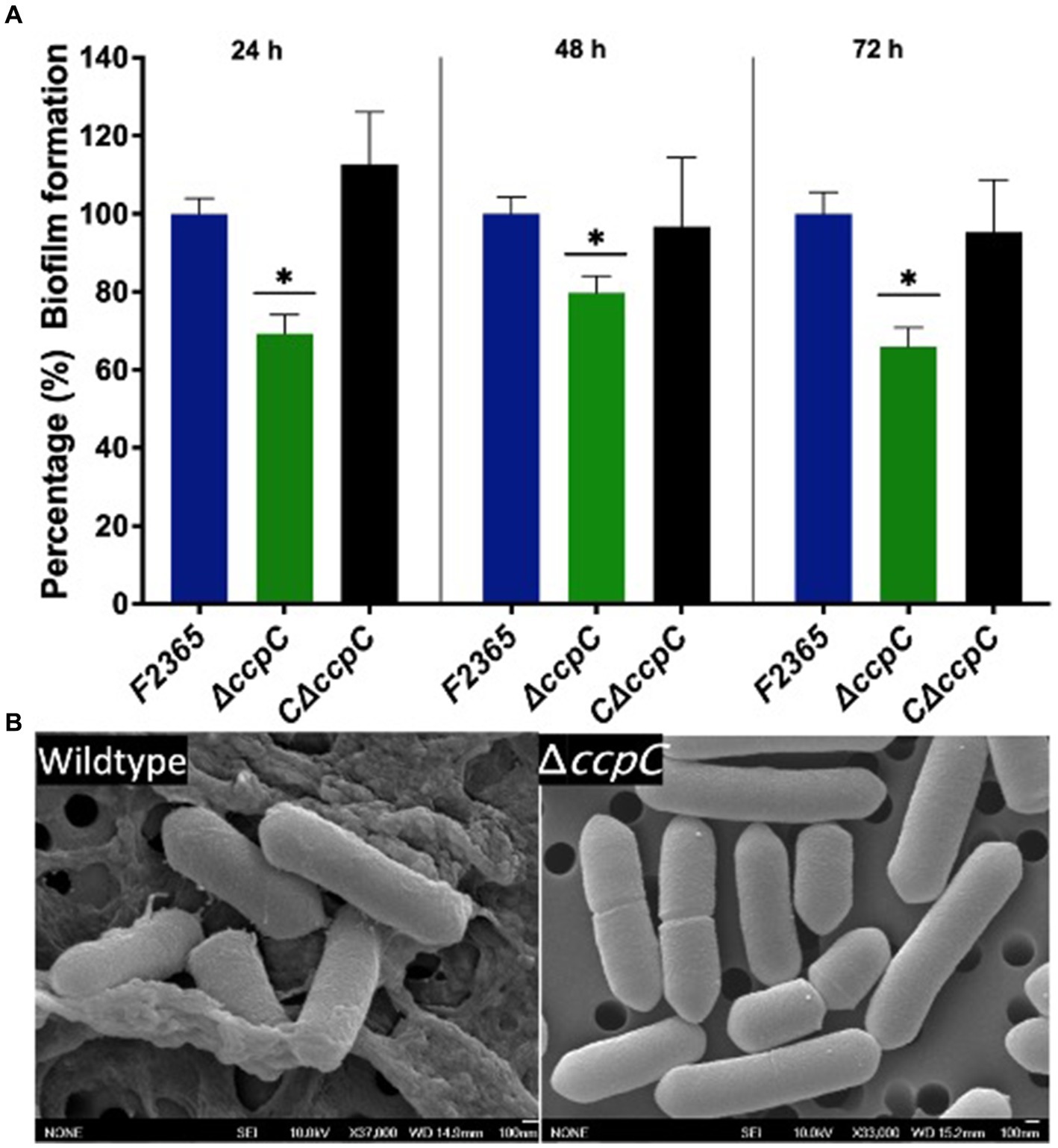
Figure 2. The ΔccpC strain exhibited a reduction in biofilm formation relative to the wildtype F2365 strain. (A) Biofilm formation was quantified by measuring the absorbance at 538 nm to detect biofilm crystal violet staining after 24, 48, and 72 h incubations in polystyrene plates. Data represent mean of six replicates ± standard error (SEM). (B) Scanning electron microscopy results showing the presence of biofilm formation in wildtype and the absence of biofilm formation for the ΔccpC strain. Data of wildtype F2365 and ΔccpC was compared with Student’s t-tests. * Shows significant differences only in the ∆ccpC mutant (p < 0.05).
3.3 CcpC is not required for efficient intracellular survival of Listeria monocytogenes
The ability of ΔccpC to replicate intracellularly in J774 macrophages was reduced by 0.4 log10 compared to F2365 based on CFU recovered from infected macrophages after 5 h, but this difference was not significant (p > 0.05) (Figure 3A).
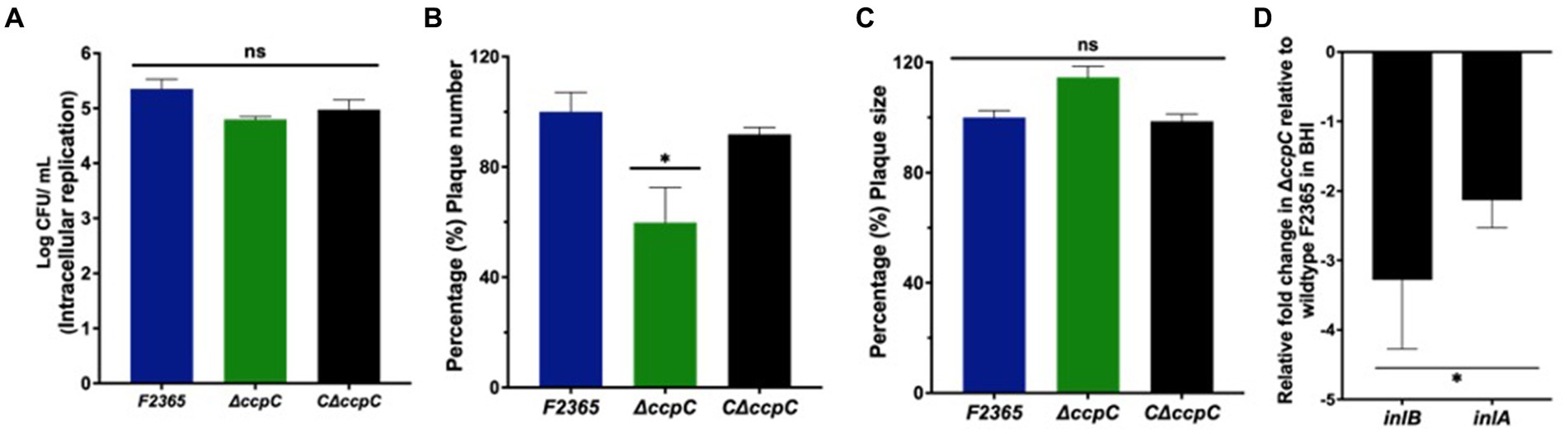
Figure 3. The ΔccpC strain exhibited insignificant intracellular replication in macrophages compared to the wildtype F2365 strain. (A) The intracellular replication of the ΔccpC is reduced insignificantly by 0.4 log10 compared to F2365 based on CFU recovered from infected macrophages after 5 h (p > 0.05). (B) Plaque numbers formed by the ΔccpC strain in L2 fibroblasts is approximately 40% reduced compared to the wildtype F2365 strain (p < 0.05). The C∆ccpC is found to restore the number of plaques to wildtype F2365 level. (C) Plaque sizes formed by the ΔccpC were not significantly different when compared to the wildtype F2365 (p > 0.05). (D) The expression of inlB and inlA genes during the growth of L. monocytogenes strains in BHI broth was significantly downregulated in the ΔccpC strain compared to the wildtype F2365 (p < 0.05). The data represent the mean ± SEM. Data were compared with Student’s t-tests. Asterisks (*) indicate significant differences, p < 0.05. NS, No significant difference.
Cell to cell spreading of ΔccpC in L2 fibroblasts was significantly decreased compared to F2365 based on plaque diameters and numbers. The ΔccpC strain had 40% reduction in plaque numbers compared to F2365 (Figure 3B). However, plaque size was not significantly affected by the ΔccpC mutation, with a ~ 9% increase relative to wildtype and the CΔccpC strains (Figure 3C). In order to provide further insights on the reduced plaque number and decipher the impact of ccpC deletion on bacterial invasion, we examined the expression of inlB and inlA genes in the ΔccpC strain. The inlB and inlA genes were significantly downregulated in the ΔccpC strain compared to the wildtype F2365 during the growth of L. monocytogenes strains in BHI broth (Figure 3D).
3.4 CcpC contributes to Listeria monocytogenes virulence
We characterized the virulence of ΔccpC strain using a murine model. At 72 h post-infection, bacterial concentrations in the spleen and liver of mice infected with ΔccpC were significantly lower (2.5 and 1.2 log10 CFU reductions, respectively) compared to those infected with F2365 (Figure 4). There were no significant differences in the bacterial concentrations in spleen and liver when comparing mice infected with the C∆ccpC and wildtype F2365 strains, indicating that the complemented strain restored the virulence of ΔccpC to wildtype levels.
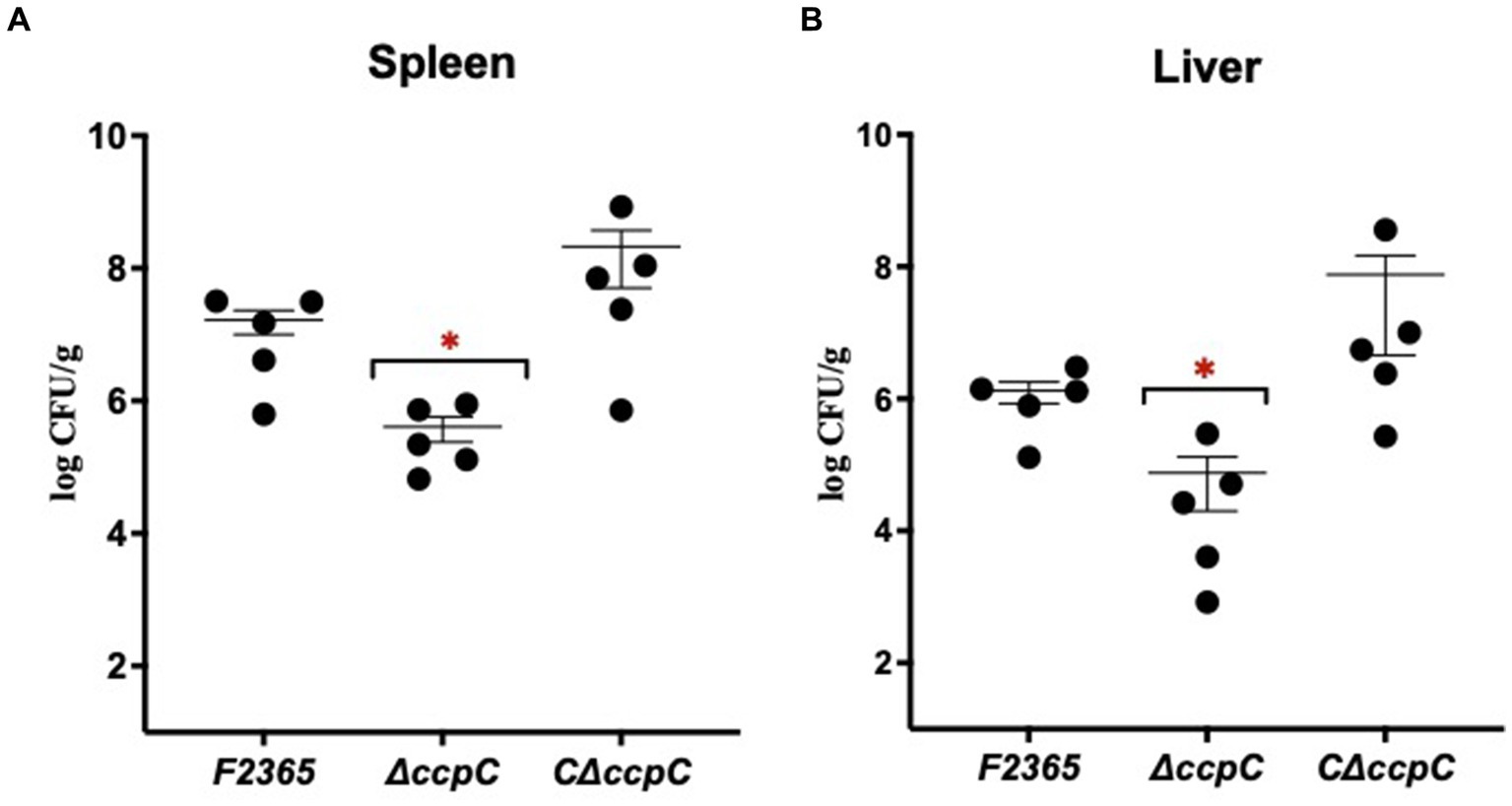
Figure 4. The virulence of ∆ccpC bacteria is attenuated in mice. Mice (n = 5/cage) were injected with 2 × 104 CFU of the indicated strains. Bacterial burdens were determined for livers (A) and spleens (B) at 72 h post-infection. Each point represents one mouse. Statistical analysis was performed using a nonparametric Mann–Whitney test. *p < 0.05.
3.5 Deletion of ccpC confers better growth and survival to Listeria monocytogenes under oxidative stress conditions
When cultured in BHI broth, ΔccpC strain exhibited growth kinetics similar to F2365, implying that ccpC is not essential for growth under nutrient-rich conditions (Figure 5A). We further assessed the growth and survival of ΔccpC strain under oxidative stress in BHI containing H2O2 at concentrations of 6, 8, or 10 mM. Relative to the wildtype strain, the ΔccpC strain exhibited a shorter lag phase (approximately 9 h) compared to F2365 at 6 mM H2O2 (Figure 5B). At 8 mM H2O2, the ΔccpC strain exhibited an approximately 9 h difference in the duration of the lag phase as compared to F2365 (Figure 5C). The ΔccpC strain reached the exponential phase faster than wildtype strain. Under high levels of oxidative stress (10 mM H2O2), the ΔccpC strain showed a longer lag phase duration compared to the lower concentrations of H2O2, but it entered the exponential phase after approximately 20 h whereas the wildtype strain did not get to the exponential phase even after 48 h (Figure 5D).
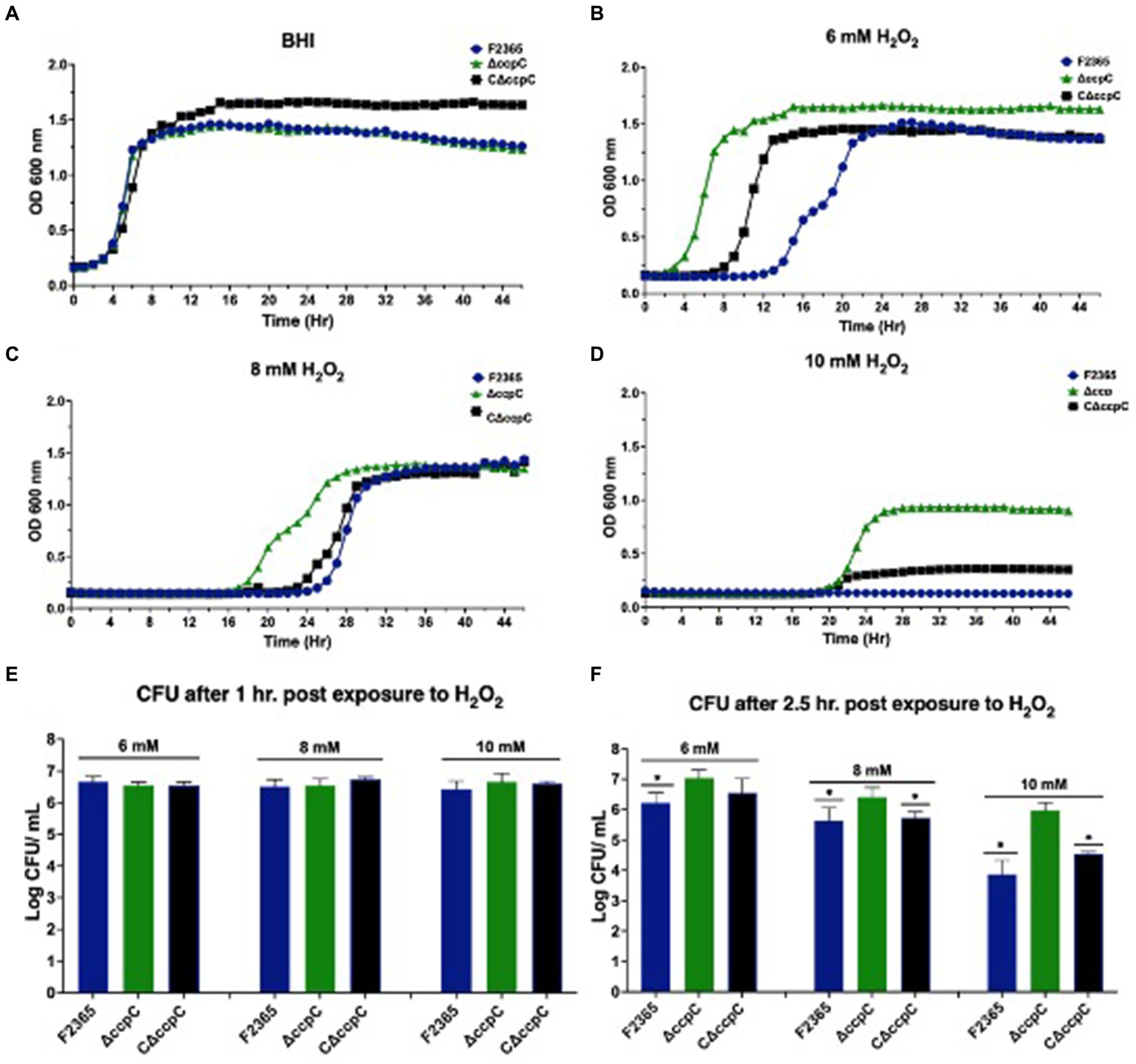
Figure 5. Growth and survival of ΔccpC strain and wildtype F2365 under oxidative stress. (A) The ΔccpC strain exhibited a similar growth curve compared to wildtype F2365 and complement strains when grown in BHI at 37°C without oxidative stress. The ΔccpC strain exhibited a decreased lag phase compared to the wildtype F2365 strain when cultured in BHI broth with 6 mM (B), 8 mM (C), and 10 mM (D) of H2O2. Growth assays were performed in a 48-well plate and were repeated at least six times independently with five replicates. Error bars represent the SEM. The survival of the ΔccpC and wildtype F2365 strains was assessed following exposure to 6, 8, or 10 mM H2O2 for 1 h (E) and 2.5 h (F). L. monocytogenes strains were grown to the mid-log phase, resuspended in BHI, and treated with H2O2, after which CFUs were enumerated by colony counts on BHI agar plates. Statistical analysis was performed using Student t-test.
We also compared the survival of the wildtype, ΔccpC, and complemented strains after exposure to H2O2 for 1 and 2.5 h in BHI. In the presence of 6, 8, and 10 mM H2O2 concentrations, the survival of the ΔccpC strain was higher (0.02, 0.05, and 0.2 log10 CFU differences) than F2365 after 1 h, but the differences were not significant (p > 0.05) (Figure 5E). After 2.5 h, however, the survival of the ΔccpC strain was significantly higher than that of the wildtype strain under these three H2O2 exposure levels (0.8, 0.9, and 2.7 log10 CFU differences) (Figure 5F). The survival and growth of complemented strain after exposure to H2O2 was similar to wildtype F2365 strain.
3.6 Transcriptomic analyses reveal the extensive impact of CcpC on Listeria monocytogenes gene expression
To further investigate the mechanism underlying the reduced susceptibility of the ΔccpC strain to oxidative stress conditions, we examined the regulatory role of CcpC following exposure to H2O2-induced oxidative stress. RNA-seq was performed on total RNA harvested from wildtype and ΔccpC cultures that had been grown to exponential phase and then exposed to 8 mM H2O2 for 2.5 h. Overall, 929 genes were found to be significantly differentially expressed by greater than two-fold (p < 0.05), indicating the broad impact of CcpC on L. monocytogenes physiology during oxidative stress. Of these differentially expressed genes (DEGs), 560 were upregulated and 369 were downregulated in the ΔccpC strain compared to levels in the wildtype strain (Figure 6). The most upregulated (n = 42) and downregulated (n = 79) DEGs are summarized in Tables 2, 3.
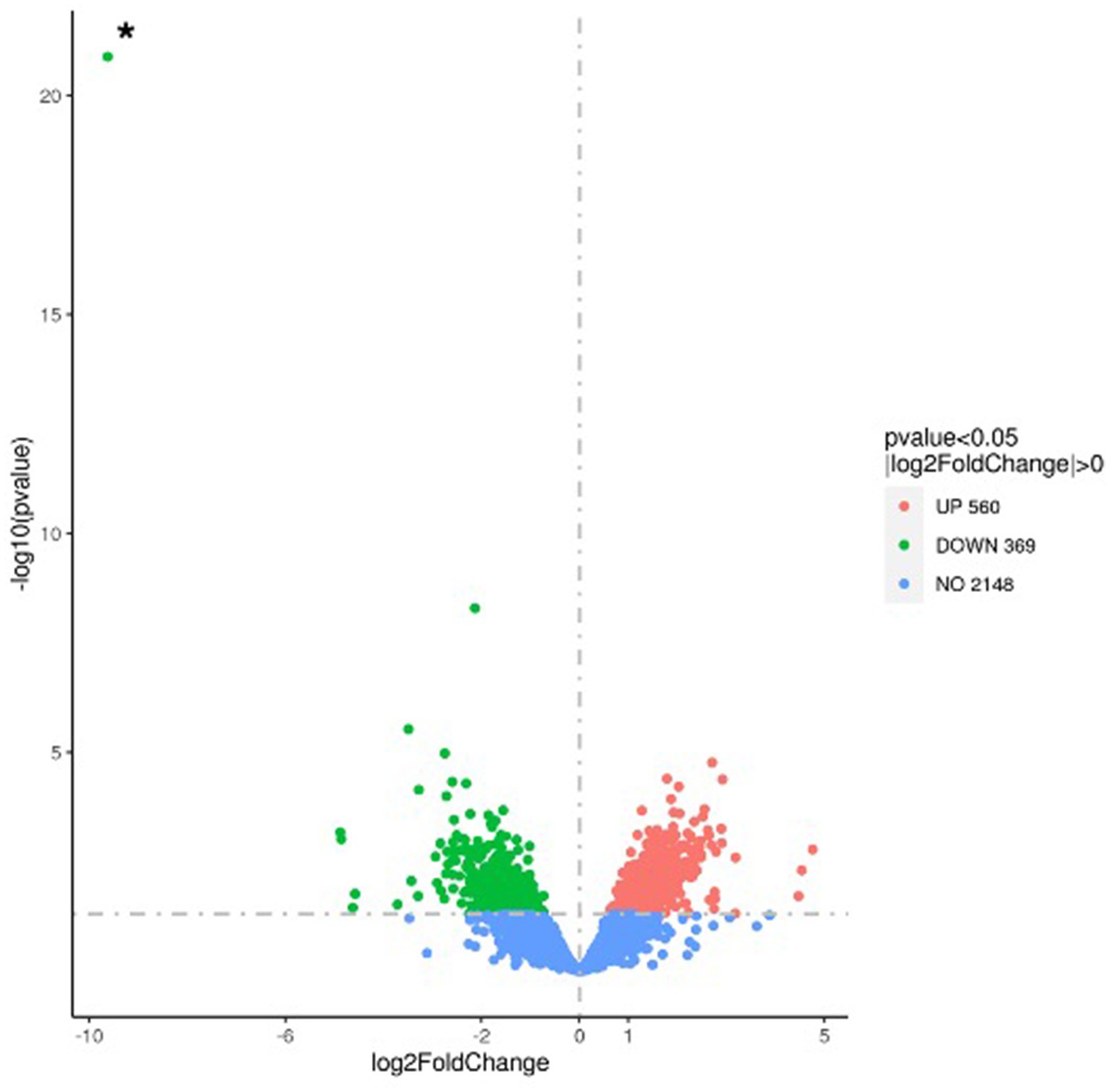
Figure 6. Volcano plot highlighting the differentially expressed genes in the ΔccpC compared to L. monocytogenes wildtype F2365 strain following oxidative stress induced by H2O2. The fold change in expression of each gene is plotted against the significant level (p-value) of the corresponding gene expression change. Upregulated genes are plotted in red, and downregulated genes are in green. The asterisk (*) indicates the ccpC gene, shown here as the most highly downregulated gene in this comparative analysis.
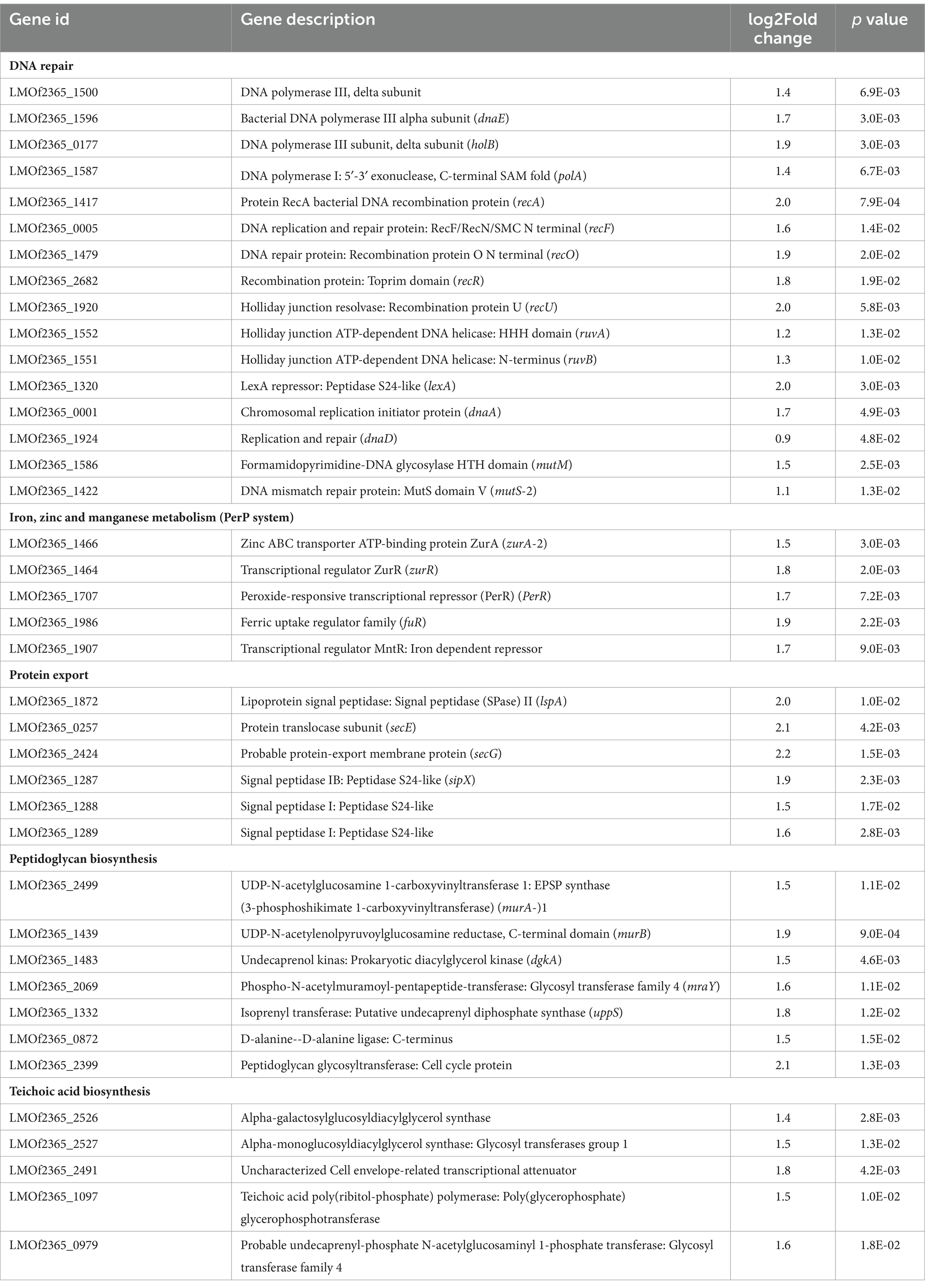
Table 2. The most upregulated genes in the ΔccpC strain compared to the levels in the wildtype F2365 strain.
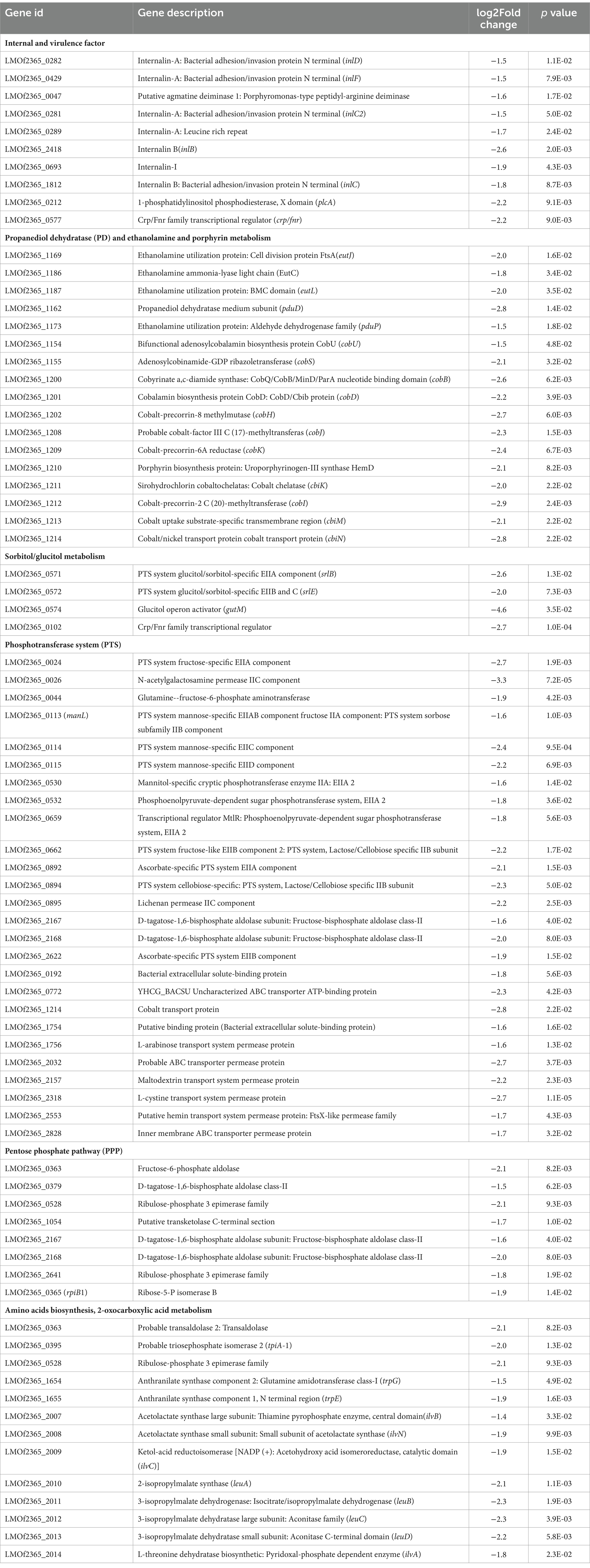
Table 3. The most downregulated genes in the ΔccpC strain compared to the levels in the wildtype F2365 strain.
To further identify the significantly enriched metabolic pathways that differed between the ΔccpC and F2365 strains, DEGs were mapped to reference pathways in the KEGG database. Pathways associated with DEGs upregulated in ΔccpC compared to F2365 included homologous recombination, mismatch repair, DNA replication (23 genes), protein export (8 genes), teichoic acid biosynthesis (9 genes), and peptidoglycan biosynthesis (8 genes) pathways (Table 4). Pathways associated with DEGs downregulated in the ΔccpC strain included BCAA biosynthesis (30 genes), PTS (28 genes), fructose and mannose metabolism (15 genes), porphyrin metabolism, 2-oxocarboxylic acid metabolism (7 genes), PPP (8 genes), and selenocompound metabolism pathways (3 genes) (Table 4).
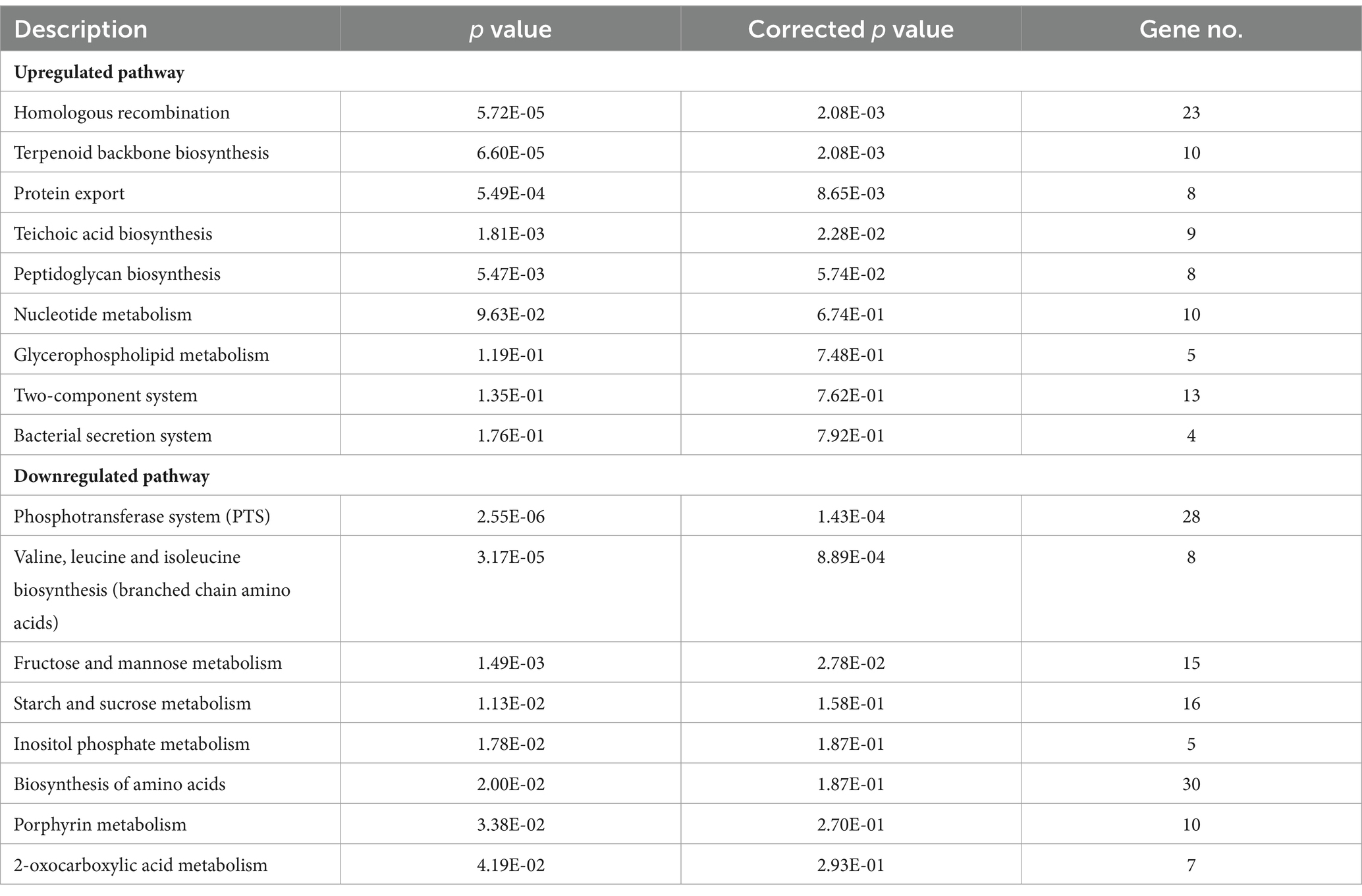
Table 4. KEGG pathway enrichment analysis for upregulated and downregulated genes in ΔccpC strain compared to the levels in the wildtype F2365 strain.
To better understand the impact of CcpC on L. monocytogenes following H2O2-induced oxidative stress, gene ontology (GO) analyses were conducted to functionally categorize differentially expressed genes into three broad categories, including biological processes, cellular components, and molecular functions. The most enriched biological process clusters were composed of genes encoding proteins involved in DNA repair, and responses to stress. With respect to cellular component terms, genes associated with the cell periphery, cytoplasmic metabolism, integral membrane component, ribonucleic complex, and ribosome were the most enriched. In the molecular function category, carbon–carbon lyase activity, kinase activity, and transferase activity terms were the most enriched groups (Table 5).
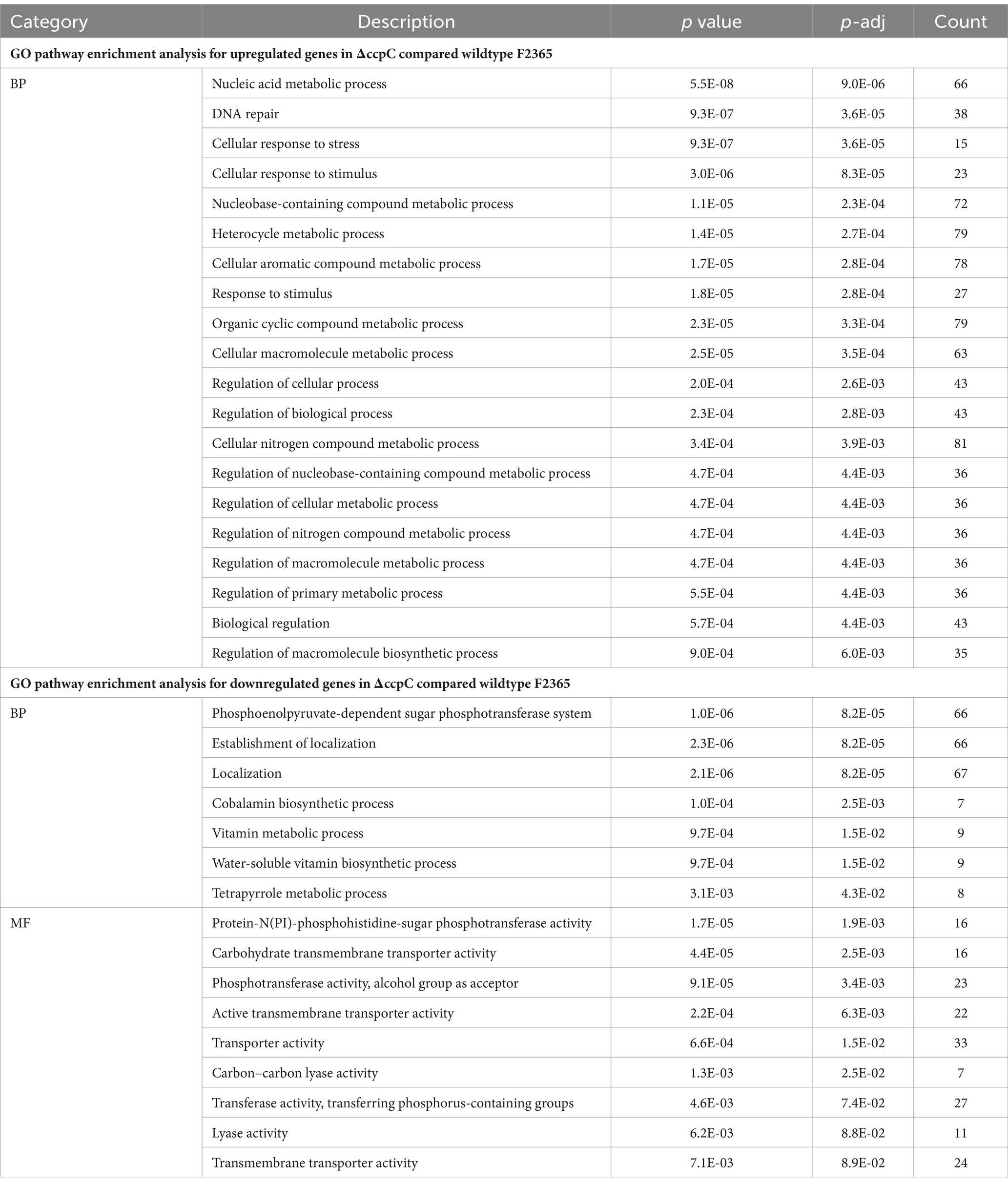
Table 5. Gene ontology (GO) enrichment analysis of the differentially expressed upregulated and downregulated genes in ΔccpC compared to the levels in the wildtype F2365 strain.
GO enrichment analyses revealed that a majority of the genes involved in the cellular response to DNA damage (15 genes), DNA metabolic processes (23 genes), DNA repair (15 genes), cellular responses to stress, and the regulation of nitrogen compound metabolic processes were significantly upregulated in ∆ccpC strain relative to the wildtype. In contrast, the main categories represented among downregulated genes were associated with organic substance transport (34 genes), PTS (27 genes), transport localization (66 genes), establishment of localization (67 genes), carbohydrate transport (66 genes), and cobalamin metabolic process (27 genes) (Table 5).
Five upregulated DEGs (dnaA, ispA, recA, lexA, and fuR) and eight downregulated DEGs (plcA, plcB, inlB, hly, crp/fnr, gutM, and eutL) were selected to validate their expression by RT-qPCR. The 16S rRNA housekeeping gene was used for normalization. The expression profile of the upregulated and downregulated genes was consistent with the transcriptomic sequencing results (Figure 7).
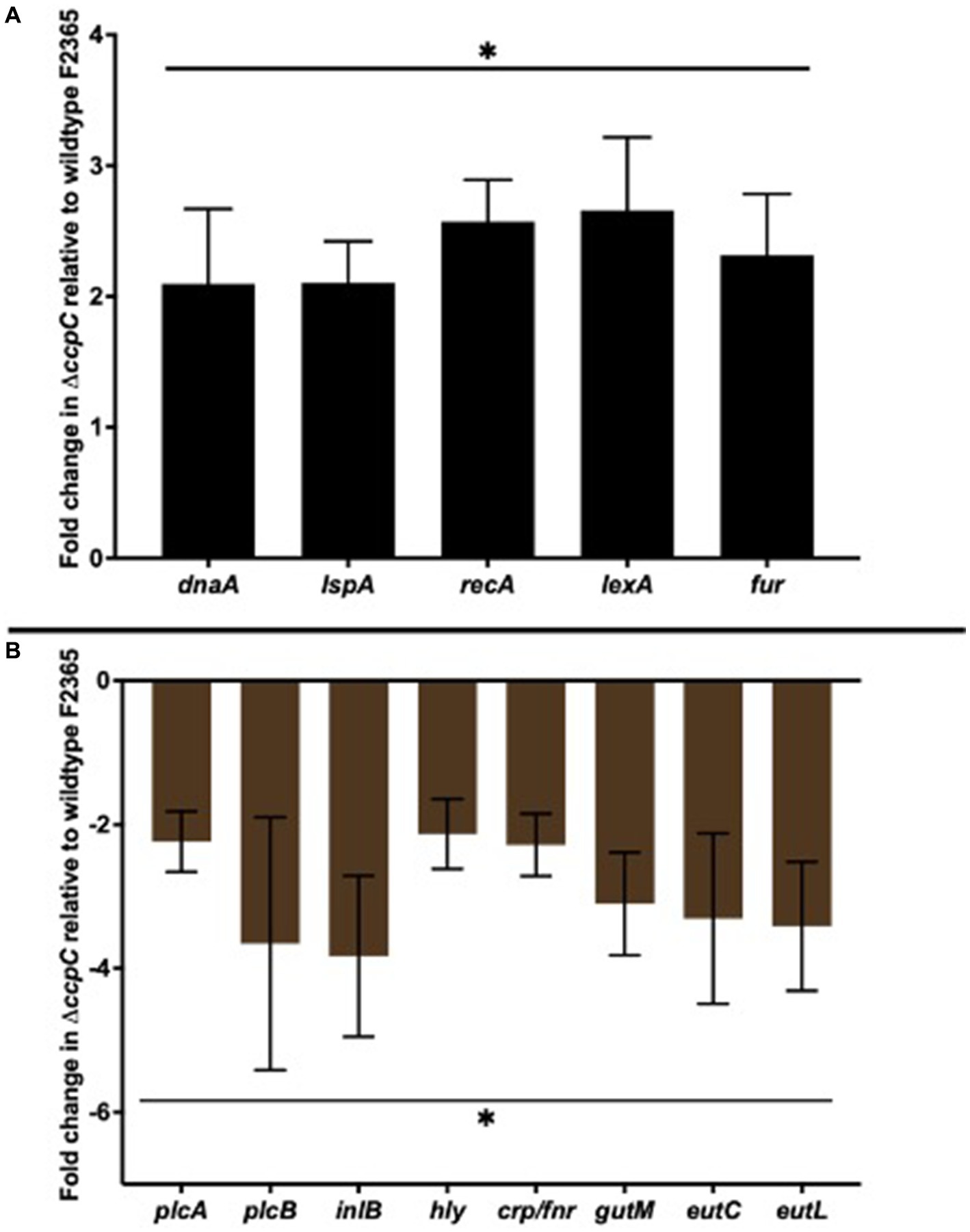
Figure 7. RT-qPCR analyses of select upregulated (A) and downregulated (B) differentially expressed genes. The selected five upregulated DEGs (dnaA, ispA, recA, lexA, and fuR) and eight downregulated DEGs (plcA, plcB, inlB, hly, crp/fnr, gutM, and eutL) showed expression profiles consistent with the transcriptomic sequencing results. Bars indicate the SEM of the mean of six biological replicates. The data were compared with Student’s t-tests. Asterisks (*) indicate significant differences, p < 0.05.
4 Discussion
This study aimed to provide new insight regarding the contribution of LysR-type transcriptional regulator (LTTR) CcpC to the pathogenesis, physiology, and stress responses of L. monocytogenes while also seeking to clarify the relationship between CcpC and other virulence factors. Given that a number of gene products directly regulated by PrfA are known to contribute to L. monocytogenes virulence, we evaluated the LLO-mediated hemolytic activity and phospholipase activities (mediated by PlcA and PlcB) of the ΔccpC strain. L. monocytogenes uses phospholipase and LLO to mediate vacuolar escape into host cell cytoplasm and to achieve cell-to-cell spread (Freitag et al., 2009; Kanki et al., 2018).
Here, we found that the deletion of ccpC slightly reduced hemolysis to ~98% of wildtype levels, while reducing the amount of LLO protein to ~75% of wildtype levels and that of phospholipase activity to ~80%. It is possible that other factors contribute to the reduction of LLO secretion in the ΔccpC strain, as there is no correlation observed between LLO protein levels and hemolytic activity. It is also reasonable to believe that a 25% reduction in LLO level is insufficient to yield a low hemolysis level. The expression level of hly was not significantly changed in the ΔccpC strain compared to wildtype, providing evidence that deletion of ccpC not affect the hemolytic activity of L. monocytogenes toward sheep erythrocytes. Although there have been limited reports linking CcpC to hemolysis and LLO expression in L. monocytogenes. This finding suggests that CcpC may be involved directly or indirectly in the secretion and activity of LLO and phospholipases (Mitchell et al., 2018). However, further insights is needed on the impacts of CcpC on the secretion of these virulence proteins.
We found that deletion of ccpC reduced the ability of L. monocytogenes to form biofilms. Despite the paucity of information regarding the contribution of CcpC to biofilm formation in L. monocytogenes, many LTTRs (PrhO, BvlR, VtlR, LeuO, and BvlR0) contribute to biofilm formation in R. solanacearum, P. aeruginosa, Agrobacterium tumefaciens, E. coli, Salmonella enterica, V. cholerae, and Yersinia enterocolitica (Shimada et al., 2011; McCarthy et al., 2014; Zhang et al., 2018; Budnick et al., 2020; Islam et al., 2021). Biofilms are important for the persistence of L. monocytogenes on many different surfaces (Nowak et al., 2021). Therefore, it is possible that CcpC plays a complex regulatory role in L. monocytogenes, and its functions appear to include several cellular processes.
Interestingly, the ΔccpC strain displayed no significant defects in intracellular growth in macrophages and exhibited normal sized plaques, indicating that the impact of CcpC on the cell to cell spread by L. monocytogenes is limited. This finding suggests that CcpC does not play a major role as a regulator of intracellular replication in tissue culture despite the observed reductions in LLO levels and phospholipase activity. Modest reductions in secreted LLO activity have generally not been linked with significant intracellular growth defects, as bacterial strains that exhibit ~25% LLO activity in comparison to wildtype strains still display normal patterns of vacuole escape and intracellular growth (Xayarath and Freitag, 2012; Phelps et al., 2018). The significant reduction in plaque numbers and expression of inlB and inlA, which could indicate that L. monocytogenes has reduced invasive and adhesion abilities after ccpC deletion.
In this study, the ΔccpC strain exhibited decreased bacterial concentrations in the liver and spleen of Swiss Webster mice. This result strongly supports a role for CcpC in L. monocytogenes pathogenesis. LTTRs have been associated with virulence in other bacterial species, such as MetR, GlyA1, MetJ, and LeuO in E. coli, PrhO in Ralstonia solanacearum, MvfR in Pseudomonas aeruginosa, and ShvR in B. cenocepacia (Bernier et al., 2008; Bogard et al., 2012; Aktories et al., 2016; Zhang et al., 2018). Together, this data suggests that the in vivo virulence defect observed in the ΔccpC strain occurs through a mechanism other than a defect in intracellular replication.
Surprisingly, our results indicated that deletion of ccpC increased the ability of L. monocytogenes to tolerate H2O2, indicating that CcpC regulates oxidative stress response. Oxidative stress-related damage is a potent bactericidal mechanism by which professional phagocytes can limit the systemic spread of L. monocytogenes (Flannagan et al., 2015; Herb and Schramm, 2021). Previous studies have revealed that the deletion of the LTTR oxyR in N. gonorrhoeae resulted in a strain that was highly resistant to H2O2-induced stress. It is noteworthy that ccpC deletion improved stress response under the H2O2-induced stress, however wildtype F2365 may be able to respond to oxidative stress just as well as the ∆ccpC strain under other conditions, including during intracellular growth in macrophages.
To investigate the role of CcpC in L. monocytogenes under conditions of oxidative stress, we identified the genes controlled by CcpC via RNA-seq. Sixteen integral genes involved with DNA repair machinery were upregulated in the ∆ccpC strain compared to F2365 after exposure to H2O2-induced oxidative stress. These genes include DNA recombination proteins (recU, recA, recF, recR, and recO), holliday junction ATP-dependent DNA helicase (ruvA, ruvB), lexA repressor, replication and repair (dnaA, dnaD, and dnaE), DNA polymerase (polA and holB), and DNA mismatch repair proteins (mutM and mutS). These genes are involved in various processing steps of DNA replication and play roles in the restarting of stalled replication forks, homologous recombination, the repair of double-stranded DNA breaks, and the introduction of adaptive point mutations (Koroleva et al., 2007; Henry and Henrikus, 2021; Matsuda et al., 2022). These results indicate that the increased expression of DNA damage repair proteins in the absence of CcpC regulation improves the response of ∆ccpC bacteria to oxidative stress-induced DNA damage.
Previous studies have shown that bacterial responses to DNA damage are mediated by the SOS response pathway, which promotes the repair and survival of DNA-damaged bacteria and the induction of genetic variation in stressed and stationary-phase bacteria (Matsuda et al., 2022). In L. monocytogenes, the SOS response pathway is regulated by LexA and RecA (Henry and Henrikus, 2021), and expression of L. monocytogenes recombination proteins is elevated in response to DNA-damaging agents (Ojha and Patil, 2020). Indeed, studies of stress-related survival have shown that a ΔrecA L. monocytogenes mutant is less resistant to heat, H2O2, and acid exposure relative to wild-type strain. Overall, this finding clearly demonstrates the importance of the ccpC gene for the ability of L. monocytogenes to adapt to oxidative stress by significantly contributing to various forms of SOS response pathway activity such as DNA repair and DNA stability.
In addition to effects on DNA repair proteins, the deletion of ccpC increased the transcription of five metalloregulatory proteins, including ferric uptake regulator family (fur), the peroxide-responsive transcriptional repressor PerR (perR), zinc ABC transporter ATP-binding protein (zurA), a zinc transcriptional regulator (zurR), and the transcriptional regulator MntR. In many Gram-positive bacteria, Fur regulates iron uptake and siderophore biosynthesis, Zur regulates two ABC zinc transporters, and PerR regulates the oxidative stress response (Horsburgh et al., 2001; Zhang et al., 2012; Pi and Helmann, 2017). Deletion of perR causes enhanced resistance to H2O2 in B. subtilis (Zhang et al., 2012), C. acetobutylicum (Hillmann et al., 2008), S. aureus (Horsburgh et al., 2001), S. pyogenes (Brenot et al., 2005), and Streptococcus suis (Zhang et al., 2012). The upregulation of these metalloregulatory genes (perR, fuR, zurR, and zurA) directly supports the observed increased resistance and survival of ∆ccpC strain under conditions of H2O2-induced stress. It is also possible that CcpC plays an important role in metal ion homeostasis in L. monocytogenes by regulating zinc and iron uptake genes. Together, these findings indicate that L. monocytogenes reacts to oxidative stress by downregulating expression of Fur/PerR-regulated genes involved in iron/zinc uptake and utilization through CcpC.
In this study, several genes involved in different routes of protein export were found to be upregulated in ∆ccpC following H2O2-induced oxidative stress, including genes encoding secretomes (secE and secG), ATP-dependent Clp endopeptidase (clp), Type-I signal peptidases (sipX, sipY, and sipZ), and lipoprotein signal peptidase (lspA). These pathways are likely used for the export of certain virulence factors to the bacterial surface in response to changes in the environment. It is likely that signal peptidases and secretory proteins allow L. monocytogenes to deal with oxidative stress following H2O2 exposure by increasing its capacity to export certain proteins, reacting in parallel to prevent further uptake of H2O2.
Genes involved in the biosynthesis of peptidoglycan, teichoic acids, and cell wall proteins were also upregulated in ∆ccpC as compared to F2365 following H2O2-induced oxidative stress. Both peptidoglycan and teichoic acid biosynthesis stem from the precursor molecule UDP-N-acetyl-α-D-glucosamine (UDP-GlcNAc). These findings suggest an increased rate of peptidoglycan and cell envelope turnover following H2O2 exposure. This increased turnover rate may reflect the increased growth rate and survival of the ∆ccpC strain.
Transcriptomic analysis revealed repression of genes encoding internalins and 1-phosphatidylinositol phosphodiesterase in ∆ccpC compared to the wildtype F2365 strain. PrfA regulates internalins (inlA and inlB) and plcA, which are critical for L. monocytogenes pathogenicity. InlA and InlB are important for cell invasion, the induction of bacterial uptake into the nonphagocytic/epithelial cells, and traversal of the intestine–blood barrier. Internalin proteins all share a leucine-rich-repeat domain that allows them to bind to structurally unrelated ligands, thereby implicating them in a wide range of functions (Kobe and Kajava, 2001).
Another important finding of this study was decreased expression of propanediol dehydratase (PD) utilization genes, ethanolamine (EA) pathway genes, and cobalamin biosynthesis genes in the ccpC mutant relative to F2365 under oxidative stress. Enzymes required for the metabolism of PD and EA are dependent on cobalamin derivatives as cofactors. L. monocytogenes uses PD and EA to maintain the bacterial microcompartment (Joseph and Goebel, 2007; Chowdhury et al., 2014; Anast et al., 2020). EA is used by L. monocytogenes as an alternative to nitrogen source (Kutzner et al., 2016; Kaval and Garsin, 2018). The pdu and eut genes are important for L. monocytogenes pathogenicity, and increased expression of these genes has been reported in the gastrointestinal tract and blood of mice (Kaval and Garsin, 2018; Anast et al., 2020). Several transcriptomic studies have shown the upregulation of EA and PD metabolism genes and cobalamin biosynthesis genes under a variety of food and food production environment-related stress conditions (Hingston et al., 2017). This finding indicates that L. monocytogenes employs CcpC to promote the expression of these genes during oxidative stress.
Several genes that play a role in the sorbitol/glucitol transport and metabolism exhibited reduced expression in the ∆ccpC strain as compared to the wildtype F2365 strain, including glucitol operon activator (gutM), glucitol/sorbitol-specific EIIA component (srlB), glucitol/sorbitol-specific EIIB and C (srlE), LMOf265_0573, SAF domain-containing protein, sugar-binding transcriptional regulator, and Crp/Fnr family transcriptional regulator. The operon is involved in the transport and phosphorylation of sorbitol to sorbitol-6-phosphate and the conversion of sorbitol-6-phosphate to fructose-6-phosphate with the associated reduction of NAD+ to NADH (Boyd et al., 2000; Murinda et al., 2004). The downregulation of the genes in the gut operon would translate to a reduced nutrient pool and subsequent energy deprivation owing to ccpC deletion in L. monocytogenes. This result supports a role for CcpC as a positive regulator of the expression of this operon in L. monocytogenes wildtype strain.
In this study, 27 PTS genes were downregulated in ∆ccpC strain as compared to the wildtype F2365 strain. The PTS is an integral system for sugar transportation and phosphorylation through three to four protein domains termed IIA, IIB, IIC, and IID (Jeckelmann and Erni, 2019). PTSs utilize phosphate to facilitate the uptake of simple sugars and thus consume more energy than other membrane kinases with the same sugar specificity (Mengaud et al., 1991). This suggests that ∆ccpC cells may benefit from employing alternative sugar uptake systems and downregulating sugar uptake when exposed to oxidative stress to conserve energy for more critical ROS defense mechanisms.
Intriguingly, some of the genes associated with the PPP were downregulated in the ∆ccpC strain relative to wildtype strain, including genes encoding including fructose-6-phosphate aldolase, D-tagatose-1,6-bisphosphate aldolase, ribulose-phosphate 3-epimerase, transketolase, C-terminal domain, probable transaldolase, and ribose/galactose isomerase. The PPP is composed of two branches, an oxidative and a non-oxidative branch. Glucose flux through the oxidative branch produces NADPH, an essential reducing agent involved in detoxification and the protection of bacteria from ROS (Dons et al., 2014; Cheng et al., 2017; Abdelhamed et al., 2022). The non-oxidative branch generates the five-carbon sugar from glucose. Previous studies have reported that the upregulation of oxidative PPP is particularly important for supplying NADPH during acute oxidative stress. Therefore, our findings may indicate that L. monocytogenes may benefit from upregulation of PPP components during oxidative stress. This finding highlights CcpC as a key factor that regulates L. monocytogenes physiology and responses to diverse stressors by controlling the expression of important metabolic pathways.
In this study, genes encoding enzymes involved in the BCAA biosynthesis operon were downregulated in the ∆ccpC strain as compared to the wildtype F2365 strain. BCAAs are integral for the nutritional requirements of L. monocytogenes (Kang et al., 2019). Previous studies have noted a link between BCAAs and virulence gene expression through CodY, which positively regulates PrfA expression in response to low BCAA levels (Keeney et al., 2009). This finding suggests a direct and/or indirect role for CcpC in modulating amino acid metabolism and the expression of the ilv-leu operon under stressful conditions. We thus speculate that the downregulation of BCAA biosynthesis may be a consequence of the adaptation and increased resistance of ∆ccpC bacteria to oxidative stress.
The ccpC gene is flanked upstream by cbpB gene, which encodes a c-di-AMP binding protein that acts as a homeostatic regulator of cellular concentrations of (p)ppGpp in response to reduced c-di-AMP levels by regulating the enzymatic functions of RelA (Peterson et al., 2020). In the present study, deletion of ccpC had no effect on expression of cbpB under H2O2-induced oxidative stress. However, we could not exclude interactions between ccpC and cbpB under other environmental conditions, like growth in nutrient rich mediums or in in different nitrogen sources. Interestingly, genes encoding enzymes involved in the TCA (citZ, citB, and citC) were not differentially expressed in the ∆ccpC strain relative to the wildtype strain in response to H2O2 treament, suggesting that the TCA metabolites and citric acid play a limited role in the L. monocytogenes response to oxidative stress.
These data, together with previous results, suggest that CcpC is likely to be involved in the fine-tuning of the expression of genes involved in stress responses, metabolic activity, and virulence. Bacterial species possess a diverse range of defense mechanisms for sensing, avoiding, and removing oxidants. We herein demonstrated that the induction of DNA repair machinery, SOS responses, and the PerR system in L. monocytogenes allows better survival under H2O2-induced oxidative stress. This study provides insights into the mechanisms that govern oxidative stress defenses in L. monocytogenes, which may aid in the future development of treatments and preventative strategies for diseases caused by these bacteria. In response to the food processing plant environment, L. monocytogenes appear to have developed a variety of defense mechanisms to protect itself against an oxidative environment. Furthermore, research findings from studies of H2O2 can be directly applicable to bacterial damage and death caused by chemicals or radiation that generate of either free radical species or reactive oxygen species.
Data availability statement
The data presented in this study are deposited in the NCBI GEO repository with accession number GSE267669.
Ethics statement
The animal study was approved by the Institutional Animal Care and Use Committee (IACUC) for animal procedures (18-508), and experiments were conducted at the College of Veterinary Medicine. The study was conducted in accordance with the local legislation and institutional requirements.
Author contributions
SO: Writing – original draft, Software, Methodology, Data curation, Formal analysis. SI: Writing – review & editing, Methodology, Formal analysis. QC: Writing – review & editing, Methodology. OO: Writing – review & editing, Methodology. ML: Validation, Writing – review & editing. HA: Visualization, Supervision, Resources, Investigation, Funding acquisition, Formal analysis, Writing – review & editing.
Funding
The author(s) declare that financial support was received for the research, authorship, and/or publication of this article. This study was supported (HA) by National Institutes of Health, National Institute of Allergy and Infectious Diseases grant no R15AI180880 and Center for Biomedical Research Excellence in Pathogen-Host Interactions, National Institute of General Medical Sciences, National Institutes of Health (P20 GM103646-09).
Acknowledgments
We thank the Laboratory Animal Resources and Care Unit at Mississippi State University for animal and veterinary care.
Conflict of interest
The authors declare that the research was conducted in the absence of any commercial or financial relationships that could be construed as a potential conflict of interest.
Publisher’s note
All claims expressed in this article are solely those of the authors and do not necessarily represent those of their affiliated organizations, or those of the publisher, the editors and the reviewers. Any product that may be evaluated in this article, or claim that may be made by its manufacturer, is not guaranteed or endorsed by the publisher.
References
Abdelhamed, H., Lawrence, M. L., and Karsi, A. (2015). A novel suicide plasmid for efficient gene mutation in Listeria monocytogenes. Plasmid 81, 1–8. doi: 10.1016/J.PLASMID.2015.05.003
Abdelhamed, H., Ramachandran, R., Narayanan, L., Islam, S., Ozan, O., Freitag, N., et al. (2022). Role of FruR transcriptional regulator in virulence of Listeria monocytogenes and identification of its regulon. PLoS One 17:e0274005. doi: 10.1371/JOURNAL.PONE.0274005
Aktories, K., Schwan, C., and Lang, A. E. (2016). ADP-Ribosylation and cross-linking of actin by bacterial protein toxins. Handb. Exp. Pharmacol. 235, 179–206. doi: 10.1007/164_2016_26
Alonzo, F., Port, G. C., Cao, M., and Freitag, N. E. (2009). The Posttranslocation chaperone PrsA2 contributes to multiple facets of Listeria monocytogenes pathogenesis. Infect. Immun. 77, 2612–2623. doi: 10.1128/IAI.00280-09
Anast, J. M., Bobik, T. A., and Schmitz-Esser, S. (2020). The cobalamin-dependent gene cluster of Listeria monocytogenes: implications for virulence, stress response, and food safety. Front. Microbiol. 11:601816. doi: 10.3389/fmicb.2020.601816
Bernier, S. P., Nguyen, D. T., and Sokol, P. A. (2008). A LysR-type transcriptional regulator in Burkholderia cenocepacia influences Colony morphology and virulence. Infect. Immun. 76, 38–47. doi: 10.1128/IAI.00874-07
Biswas, R., Sonenshein, A. L., and Belitsky, B. R. (2020). Role of GlnR in controlling expression of nitrogen metabolism genes in Listeria monocytogenes. J. Bacteriol. 202, 209–229. doi: 10.1128/JB.00209-20
Blank, B. S., Abi Abdallah, D. S., Park, J. J., Nazarova, E. V., Bitar, A. P., Maurer, K. J., et al. (2014). Misregulation of the broad-range phospholipase C activity increases the susceptibility of Listeria monocytogenes to intracellular killing by neutrophils. Microbes Infect. 16, 104–113. doi: 10.1016/J.MICINF.2013.10.014
Bogard, R. W., Davies, B. W., and Mekalanos, J. J. (2012). MetR-regulated vibrio cholerae metabolism is required for virulence. MBio 3:e00236-12. doi: 10.1128/mBio.00236-12
Bou Ghanem, E. N., Jones, G. S., Myers-Morales, T., Patil, P. D., Hidayatullah, A. N., and D’Orazio, S. E. F. (2012). InlA promotes dissemination of Listeria monocytogenes to the mesenteric lymph nodes during food borne infection of mice. PLoS Pathog. 8:e1003015. doi: 10.1371/JOURNAL.PPAT.1003015
Boyd, D. A., Thevenot, T., Gumbmann, M., Honeyman, A. L., and Hamilton, I. R. (2000). Identification of the operon for the sorbitol (glucitol) phosphoenolpyruvate:sugar phosphotransferase system in Streptococcus mutans. Infect. Immun. 68, 925–930. doi: 10.1128/IAI.68.2.925-930.2000
Brenot, A., King, K. Y., and Caparon, M. G. (2005). The PerR regulon in peroxide resistance and virulence of Streptococcus pyogenes. Mol. Microbiol. 55, 221–234. doi: 10.1111/J.1365-2958.2004.04370.X
Bubert, A., Riebe, J., Schnitzler, N., Schönberg, A., Goebel, W., and Schubert, P. (1997). Isolation of catalase-negative Listeria monocytogenes strains from listeriosis patients and their rapid identification by anti-p60 antibodies and/or PCR. J. Clin. Microbiol. 35, 179–183. doi: 10.1128/JCM.35.1.179-183.1997
Buchanan, R. L., Gorris, L. G. M., Hayman, M. M., Jackson, T. C., and Whiting, R. C. (2017). A review of Listeria monocytogenes: an update on outbreaks, virulence, dose-response, ecology, and risk assessments. Food Control 75, 1–13. doi: 10.1016/J.FOODCONT.2016.12.016
Budnick, J. A., Sheehan, L. M., Ginder, M. J., Failor, K. C., Perkowski, J. M., Pinto, J. F., et al. (2020). A central role for the transcriptional regulator VtlR in small RNA-mediated gene regulation in Agrobacterium tumefaciens. Sci. Rep. 10, 1–16. doi: 10.1038/s41598-020-72117-0
Camejo, A., Carvalho, F., Reis, O., Leitão, E., Sousa, S., Cabanes, D., et al. (2011). The arsenal of virulence factors deployed by Listeria monocytogenes to promote its cell infection cycle. Virulence 2, 379–394. doi: 10.4161/viru.2.5.17703
Cao, H., Krishnan, G., Goumnerov, B., Tsongalis, J., Tompkins, R., and Rahme, L. G. (2001). A quorum sensing-associated virulence gene of Pseudomonas aeruginosa encodes a LysR-like transcription regulator with a unique self-regulatory mechanism. Proc. Natl. Acad. Sci. USA 98, 14613–14618. doi: 10.1073/PNAS.251465298
Cheng, C., Dong, Z., Han, X., Wang, H., Jiang, L., Sun, J., et al. (2017). Thioredoxin a is essential for motility and contributes to host infection of listeria monocytogenes via redox interactions. Front. Cell. Infect. Microbiol. 7:287. doi: 10.3389/fcimb.2017.00287
Chowdhury, C., Sinha, S., Chun, S., Yeates, T. O., and Bobik, T. A. (2014). Diverse bacterial microcompartment organelles. Microbiol. Mol. Biol. Rev. 78, 438–468. doi: 10.1128/MMBR.00009-14
Dons, L. E., Mosa, A., Rottenberg, M. E., Rosenkrantz, J. T., Kristensson, K., and Olsen, J. E. (2014). Role of the Listeria monocytogenes 2-Cys peroxiredoxin homologue in protection against oxidative and nitrosative stress and in virulence. Pathog. Dis. 70, 70–74. doi: 10.1111/2049-632X.12081
Drevets, D. A., and Bronze, M. S. (2008). Listeria monocytogenes: epidemiology, human disease, and mechanisms of brain invasion. FEMS Immunol. Med. Microbiol. 53, 151–165. doi: 10.1111/J.1574-695X.2008.00404.X
Eallonardo, S. J., Wang, Y., and Freitag, N. E. (2023). “Listeria Monocytogenes” in Molecular Medical Microbiology. 3rd ed, 1249–1267.
Flannagan, R. S., Heit, B., and Heinrichs, D. E. (2015). Antimicrobial mechanisms of macrophages and the immune evasion strategies of Staphylococcus aureus. Pathogens 4, 826–868. doi: 10.3390/PATHOGENS4040826
Freitag, N. E., Port, G. C., and Miner, M. D. (2009). Listeria monocytogenes — from saprophyte to intracellular pathogen. Nat. Rev. Microbiol. 7, 623–628. doi: 10.1038/NRMICRO2171
Gray, J., Chandry, P. S., Kaur, M., Kocharunchitt, C., Fanning, S., Bowman, J. P., et al. (2021). Colonisation dynamics of Listeria monocytogenes strains isolated from food production environments. Sci. Rep. 11, 1–17. doi: 10.1038/s41598-021-91503-w
Henry, C., and Henrikus, S. S. (2021). Elucidating recombination mediator function using biophysical tools. Biology (Basel) 10:288. doi: 10.3390/BIOLOGY10040288
Herb, M., and Schramm, M. (2021). Functions of ROS in macrophages and antimicrobial immunity. Antioxidants 10:313. doi: 10.3390/ANTIOX10020313
Hillmann, F., Fischer, R. J., Saint-Prix, F., Girbal, L., and Bahl, H. (2008). PerR acts as a switch for oxygen tolerance in the strict anaerobe Clostridium acetobutylicum. Mol. Microbiol. 68, 848–860. doi: 10.1111/J.1365-2958.2008.06192.X
Hingston, P., Chen, J., Allen, K., Hansen, L. T., and Wang, S. (2017). Strand specific RNA-sequencing and membrane lipid profiling reveals growth phase-dependent cold stress response mechanisms in Listeria monocytogenes. PLoS One 12:e0180123. doi: 10.1371/JOURNAL.PONE.0180123
Horsburgh, M. J., Ingham, E., and Foster, S. J. (2001). In Staphylococcus aureus, Fur is an interactive regulator with PerR, contributes to virulence, and is necessary for oxidative stress resistance through positive regulation of catalase and Iron homeostasis. J. Bacteriol. 183, 468–475. doi: 10.1128/JB.183.2.468-475.2001
Huynh, T. A. N., and Woodward, J. J. (2016). Too much of a good thing: regulated depletion of c-di-AMP in the bacterial cytoplasm. Curr. Opin. Microbiol. 30, 22–29. doi: 10.1016/J.MIB.2015.12.007
Islam, M. M., Kim, K., Lee, J. C., and Shin, M. (2021). LeuO, a LysR-type transcriptional regulator, is involved in biofilm formation and virulence of Acinetobacter baumannii. Front. Cell. Infect. Microbiol. 11:738706. doi: 10.3389/fcimb.2021.738706
Jeckelmann, J. M., and Erni, B. (2019). Carbohydrate transport by group translocation: the bacterial phosphoenolpyruvate: sugar phosphotransferase system. Subcell. Biochem. 92, 223–274. doi: 10.1007/978-3-030-18768-2_8
Jones, S., and Portnoy, D. A. (1994). Characterization of Listeria monocytogenes pathogenesis in a strain expressing perfringolysin O in place of listeriolysin O. Infect. Immun. 62, 5608–5613. doi: 10.1128/IAI.62.12.5608-5613.1994
Joseph, B., and Goebel, W. (2007). Life of Listeria monocytogenes in the host cells’ cytosol. Microbes Infect. 9, 1188–1195. doi: 10.1016/J.MICINF.2007.05.006
Kang, J., Burall, L., Mammel, M. K., and Datta, A. R. (2019). Global transcriptomic response of Listeria monocytogenes during growth on cantaloupe slices. Food Microbiol. 77, 192–201. doi: 10.1016/J.FM.2018.09.012
Kanki, M., Naruse, H., and Kawatsu, K. (2018). Comparison of listeriolysin O and phospholipases PlcA and PlcB activities, and initial intracellular growth capability among food and clinical strains of Listeria monocytogenes. J. Appl. Microbiol. 124, 899–909. doi: 10.1111/JAM.13692
Kaval, K. G., and Garsin, D. A. (2018). Ethanolamine utilization in bacteria. MBio 9:e00066-18. doi: 10.1128/mBio.00066-18
Keeney, K., Colosi, L., Weber, W., and O’Riordan, M. (2009). Generation of branched-chain fatty acids through Lipoate-dependent metabolism facilitates intracellular growth of Listeria monocytogenes. J. Bacteriol. 191, 2187–2196. doi: 10.1128/JB.01179-08
Kim, H. J., Mittal, M., and Sonenshein, A. L. (2006). CcpC-dependent regulation of citB and lmo0847 in Listeria monocytogenes. J. Bacteriol. 188, 179–190. doi: 10.1128/JB.188.1.179-190.2006
Kim, H. J., Roux, A., and Sonenshein, A. L. (2002). Direct and indirect roles of CcpA in regulation of Bacillus subtilis Krebs cycle genes. Mol. Microbiol. 45, 179–190. doi: 10.1046/J.1365-2958.2002.03003.X
Kobe, B., and Kajava, A. V. (2001). The leucine-rich repeat as a protein recognition motif. Curr. Opin. Struct. Biol. 11, 725–732. doi: 10.1016/S0959-440X(01)00266-4
Koroleva, O., Makharashvili, N., Courcelle, C. T., Courcelle, J., and Korolev, S. (2007). Structural conservation of RecF and Rad50: implications for DNA recognition and RecF function. EMBO J. 26, 867–877. doi: 10.1038/SJ.EMBOJ.7601537
Kutzner, E., Kern, T., Felsl, A., Eisenreich, W., and Fuchs, T. M. (2016). Isotopologue profiling of the listerial N-metabolism. Mol. Microbiol. 100, 315–327. doi: 10.1111/MMI.13318
Lakicevic, B. Z., Den Besten, H. M. W., and De Biase, D. (2022). Landscape of stress response and virulence genes among Listeria monocytogenes strains. Front. Microbiol. 12:738470. doi: 10.3389/fmicb.2021.738470
Lampidis, R., Gross, R., Sokolovic, Z., Goebel, W., and Kreft, J. (1994). The virulence regulator protein of Listeria ivanovii is highly homologous to PrfA from Listeria monocytogenes and both belong to the Crp-Fnr family of transcription regulators. Mol. Microbiol. 13, 141–151. doi: 10.1111/J.1365-2958.1994.TB00409.X
Lauer, P., Chow, M. Y. N., Loessner, M. J., Portnoy, D. A., and Calendar, R. (2002). Construction, characterization, and use of two Listeria monocytogenes site-specific phage integration vectors. J. Bacteriol. 184, 4177–4186. doi: 10.1128/JB.184.15.4177-4186.2002
Maddocks, S. E., and Oyston, P. C. F. (2008). Structure and function of the LysR-type transcriptional regulator (LTTR) family proteins. Microbiology (N Y) 154, 3609–3623. doi: 10.1099/MIC.0.2008/022772-0
Matsuda, R., Suzuki, S., and Kurosawa, N. (2022). Genetic study of four candidate Holliday junction processing proteins in the thermophilic Crenarchaeon Sulfolobus acidocaldarius. Int. J. Mol. Sci. 23:707. doi: 10.3390/IJMS23020707
McCarthy, R. R., Mooij, M. J., Reen, F. J., Lesouhaitier, O., and O’Gara, F. (2014). A new regulator of pathogenicity (bvlR) is required for full virulence and tight microcolony formation in Pseudomonas aeruginosa. Microbiology (Reading) 160, 1488–1500. doi: 10.1099/MIC.0.075291-0
Mengaud, J., Braun-Breton, C., and Cossart, P. (1991). Identification of phosphatidylinositol-specific phospholipase C activity in Listeria monocytogenes: a novel type of virulence factor? Mol. Microbiol. 5, 367–372. doi: 10.1111/J.1365-2958.1991.TB02118.X
Mitchell, G., Cheng, M. I., Chen, C., Nguyen, B. N., Whiteley, A. T., Kianian, S., et al. (2018). Listeria monocytogenes triggers noncanonical autophagy upon phagocytosis, but avoids subsequent growth-restricting xenophagy. Proc. Natl. Acad. Sci. USA 115, E210–E217. doi: 10.1073/PNAS.1716055115
Mittal, M., Pechter, K. B., Picossi, S., Kim, H. J., Kerstein, K. O., and Sonenshein, A. L. (2013). Dual role of CcpC protein in regulation of aconitase gene expression in Listeria monocytogenes and Bacillus subtilis. Microbiology (Reading) 159, 68–76. doi: 10.1099/MIC.0.063388-0
Moors, M. A., Levitt, B., Youngman, P., and Portnoy, D. A. (1999). Expression of listeriolysin O and ActA by intracellular and extracellular Listeria monocytogenes. Infect Immun. 67, 131–139. doi: 10.1128/IAI.67.1.131-139.1999/ASSET/9725BB05-70FC-4842-A286-0A815598BE8E/ASSETS/GRAPHIC/II0190966007.JPEG
Murinda, S. E., Nguyen, L. T., Nam, H. M., Almeida, R. A., Headrick, S. J., and Oliver, S. P. (2004). Detection of sorbitol-negative and sorbitol-positive Shiga toxin-producing Escherichia coli, Listeria monocytogenes, campylobacter jejuni, and Salmonella spp. in dairy farm environmental samples. Foodborne Pathog. Dis. 1, 97–104. doi: 10.1089/153531404323143611
Nelson, K. E., Fouts, D. E., Mongodin, E. F., Ravel, J., DeBoy, R. T., Kolonay, J. F., et al. (2004). Whole genome comparisons of serotype 4b and 1/2a strains of the food-borne pathogen Listeria monocytogenes reveal new insights into the core genome components of this species. Nucleic Acids Res. 32, 2386–2395. doi: 10.1093/NAR/GKH562
Nilsson, U. R., and Nilsson, B. (1984). Simplified assays of hemolytic activity of the classical and alternative complement pathways. J Immunol Methods. 72, 49–59. doi: 10.1016/0022-1759(84)90432-0
Nowak, J., Visnovsky, S. B., Pitman, A. R., Cruz, C. D., Palmer, J., Fletcher, G. C., et al. (2021). Biofilm formation by Listeria monocytogenes 15G01, a persistent isolate from a seafood-processing plant, is influenced by inactivation of multiple genes belonging to different functional groups. Appl. Environ. Microbiol. 87, 1–19. doi: 10.1128/AEM.02349-20
Ojha, D., and Patil, K. N. (2020). Molecular and functional characterization of the Listeria monocytogenes RecA protein: insights into the homologous recombination process. Int. J. Biochem. Cell Biol. 119:105642. doi: 10.1016/J.BIOCEL.2019.105642
Osek, J., Lachtara, B., and Wieczorek, K. (2022). Listeria monocytogenes – how this pathogen survives in food-production environments? Front. Microbiol. 13:866462. doi: 10.3389/FMICB.2022.866462
Pechter, K. B., Meyer, F. M., Serio, A. W., Stülke, J., and Sonenshein, A. L. (2013). Two roles for aconitase in the regulation of tricarboxylic acid branch gene expression in Bacillus subtilis. J. Bacteriol. 195, 1525–1537. doi: 10.1128/JB.01690-12
Peterson, B. N., Young, M. K. M., Luo, S., Wang, J., Whiteley, A. T., Woodward, J. J., et al. (2020). (P)ppGpp and c-di-AMP homeostasis is controlled by Cbpb in listeria monocytogenes. MBio 11, 1–16. doi: 10.1128/MBIO.01625-20/SUPPL_FILE/MBIO.01625-20-ST003.TIF
Phelps, C. C., Vadia, S., Arnett, E., Tan, Y., Zhang, X., Pathak-Sharma, S., et al. (2018). Relative roles of Listeriolysin O, InlA, and InlB in Listeria monocytogenes uptake by host cells. Infect. Immun. 86:e00555-18. doi: 10.1128/IAI.00555-18
Pi, H., and Helmann, J. D. (2017). Sequential induction of Fur-regulated genes in response to iron limitation in Bacillus subtilis. Proc. Natl. Acad. Sci. USA 114, 12785–12790. doi: 10.1073/pnas.1713008114
Reniere, M. L., Whiteley, A. T., Hamilton, K. L., John, S. M., Lauer, P., Brennan, R. G., et al. (2015). Glutathione activates virulence gene expression of an intracellular pathogen. Nature 517, 170–173. doi: 10.1038/NATURE14029
Russell, D. A., Byrne, G. A., O’Connell, E. P., Boland, C. A., and Meijer, W. G. (2004). The LysR-type transcriptional regulator VirR is required for expression of the virulence gene vapA of Rhodococcus equi ATCC 33701. J. Bacteriol. 186, 5576–5584. doi: 10.1128/JB.186.17.5576-5584.2004
Seveau, S. (2014). Multifaceted activity of listeriolysin O, the cholesterol-dependent cytolysin of Listeria monocytogenes. Subcell Biochem. 80, 161–195. doi: 10.1007/978-94-017-8881-6_9/COVER
Shimada, T., Bridier, A., Briandet, R., and Ishihama, A. (2011). Novel roles of LeuO in transcription regulation of E. coli genome: antagonistic interplay with the universal silencer H-NS. Mol. Microbiol. 82, 378–397. doi: 10.1111/J.1365-2958.2011.07818.X
Vargas García, C. E., Petrova, M., Claes, I. J. J., De Boeck, I., Verhoeven, T. L. A., Dilissen, E., et al. (2015). Piliation of Lactobacillus rhamnosus GG promotes adhesion, phagocytosis, and cytokine modulation in macrophages. Appl. Environ. Microbiol. 81, 2050–2062. doi: 10.1128/AEM.03949-14
Wakimoto, N., Nishi, J., Sheikh, J., Nataro, J. P., Sarantuya, J., Iwashita, M., et al. (2004). Quantitative biofilm assay using a microtiter plate to screen for enteroaggregative Escherichia coli. Am. J. Trop. Med. Hyg. 71, 687–690. doi: 10.4269/AJTMH.2004.71.687
Wiktorczyk-Kapischke, N., Skowron, K., and Wałecka-Zacharska, E. (2023). Genomic and pathogenicity islands of Listeria monocytogenes—overview of selected aspects. Front. Mol. Biosci. 10:1161486. doi: 10.3389/fmolb.2023.1161486
Xayarath, B., and Freitag, N. E. (2012). Optimizing the balance between host and environmental survival skills: lessons learned from Listeria monocytogenes. Future Microbiol. 7, 839–852. doi: 10.2217/FMB.12.57
Young, M. D., Wakefield, M. J., Smyth, G. K., and Oshlack, A. (2010). Gene ontology analysis for RNA-seq: accounting for selection bias. Genome Biol. 11, 1–12. doi: 10.1186/GB-2010-11-2-R14/TABLES/4
Zhang, T., Ding, Y., Li, T., Wan, Y., Li, W., Chen, H., et al. (2012). A Fur-like protein PerR regulates two oxidative stress response related operons dpr and metQIN in Streptococcus suis. BMC Microbiol. 12:85. doi: 10.1186/1471-2180-12-85
Keywords: Listeria, oxidative stress, RNA-seq, virulence factor, biofilm
Citation: Ogunleye SC, Islam S, Monzur Kader Chowdhury QMMK, Ozdemir O, Lawrence ML and Abdelhamed H (2024) Catabolite control protein C contributes to virulence and hydrogen peroxide-induced oxidative stress responses in Listeria monocytogenes. Front. Microbiol. 15:1403694. doi: 10.3389/fmicb.2024.1403694
Edited by:
Hidetada Hirakawa, Gunma University, JapanReviewed by:
Yong Fu, Washington University in St. Louis, United StatesFilipe Carvalho, INRAE Centre Jouy-en-Josas, France
Copyright © 2024 Ogunleye, Islam, Monzur Kader Chowdhury, Ozdemir, Lawrence and Abdelhamed. This is an open-access article distributed under the terms of the Creative Commons Attribution License (CC BY). The use, distribution or reproduction in other forums is permitted, provided the original author(s) and the copyright owner(s) are credited and that the original publication in this journal is cited, in accordance with accepted academic practice. No use, distribution or reproduction is permitted which does not comply with these terms.
*Correspondence: Hossam Abdelhamed, abdelhamed@cvm.msstate.edu