- Bioénergétique et Ingénierie des Protéines Laboratory, UMR 7281, Aix-Marseille Université and Centre National de la Recherche Scientifique, Marseille, France
Proteins are highly variable biological systems, not only in their structures but also in their dynamics. The most extreme example of dynamics is encountered within the family of Intrinsically Disordered Proteins (IDPs), which are proteins lacking a well-defined 3D structure under physiological conditions. Among the biophysical techniques well-suited to study such highly flexible proteins, Site-Directed Spin Labeling combined with EPR spectroscopy (SDSL-EPR) is one of the most powerful, being able to reveal, at the residue level, structural transitions such as folding events. SDSL-EPR is based on selective grafting of a paramagnetic label on the protein under study and is limited neither by the size nor by the complexity of the system. The objective of this mini-review is to describe the basic strategy of SDSL-EPR and to illustrate how it can be successfully applied to characterize the structural behavior of IDPs. Recent developments aimed at enlarging the panoply of SDSL-EPR approaches are presented in particular newly synthesized spin labels that allow the limitations of the classical ones to be overcome. The potentialities of these new spin labels will be demonstrated on different examples of IDPs.
Introduction
Characterizing dynamics of macromolecules is a complex task requiring the use of appropriate techniques. In parallel to the development of methods leading to structure resolution of proteins, there is also an increasing need to develop biophysical techniques able to describe structural flexibility, as this dynamical aspect is closely related to protein function (Dyson and Wright, 2002; Salmon et al., 2010). The most highly dynamic biological systems are the so-called Intrinsically Disordered Proteins (IDPs) or Regions (IDRs), which lack a well-defined 3D structure under physiological conditions while being associated to key functions such as regulation, molecular assembly, signaling… (for recent reviews see Uversky and Dunker, 2010; Babu et al., 2011; Chouard, 2011; Habchi et al., 2014). Among the various techniques able to give access to dynamic properties of biomolecules is Site-Directed Spin Labeling combined with Electron Paramagnetic Resonance (SDSL-EPR), a technique that was pioneered about 20 years ago by (Hubbell et al., 1996). SDSL-EPR is very sensitive for probing flexible regions of proteins and revealing dynamic changes or interaction sites in protein-protein interactions (Belle et al., 2007; Lorenzi et al., 2012). It can also be used to determine accessibility profiles, a powerful approach to determine the topology of membrane proteins (Gross et al., 1999; Kaplan et al., 2000). More recently, the development of pulse EPR, in particular Double Electron Electron Resonance (DEER) techniques allowed the measurement of distances between spin labeled sites in the range of 1.8–6.0 nm (Pannier et al., 2000), thus covering a wide range of interest for the study of large conformational transitions and biomolecule associations. Excellent reviews describing all of these approaches, as well as applications of SDSL-EPR on proteins, have been recently published (Klare and Steinhoff, 2009; Bordignon, 2011; Mchaourab et al., 2011; Drescher, 2012; Hubbell et al., 2013). The present contribution will focus on dynamic analyses of extremely flexible biological systems and recent synthesis of new spin labels designed to enlarge the potentialities of the technique.
General Principles and Development of New Spin Labels
SDSL-EPR relies on selective grafting of a paramagnetic species, usually a nitroxide derivative, at a desired position of a protein and subsequent analysis by EPR spectroscopy. Nitroxides are stable radicals in which the unpaired electron is delocalized on the N-O group, leading to a 3-line spectrum arising from the hyperfine interaction between the electron spin and the nuclear spin of the 14N atom. Nitroxide spin labels are classically functionalized to react specifically with cysteine residues. The technique thus requires the construction of cysteine mutants in order to target specific sites. The most frequently used nitroxide spin label is the MTSL (1-oxyl-2,2,5,5-tetramethylpyrroline-3-methyl) methanethiosulfonate leading to the formation of a disulphide bridge between the side-chain of the cysteine and the label (Figure 1A, side-chain R1). Its relatively small size minimizes the potential perturbation of the biological system. Other commercial spin labels are available, such as the maleimide-functionalized ones having the advantage of forming a thio-ether bond with the protein side-chain preventing the label release in the presence of reducing agents. One example of such spin label is the 3-maleimido-2,2,5,5-tetramethyl-pyrrolidinyloxy referred to as Proxyl or P depicted in Figure 1A (spin label N2). These labels are, however, more sterically demanding than MTSL, and they can react with amines at high pH (Hideg et al., 2005). As for all labeling techniques, control experiments are essential for comparing wild-type protein and labeled mutants to check the non-invasiveness of the label with respect to protein structural and functional properties. By investigating the relationship between the mobility of the MTSL nitroxide side-chain and the structural elements of the T4 lysozyme taken as a model protein, Mchaourab and co-workers established the basis for the interpretation of EPR spectral shapes of spin labels (Mchaourab et al., 1996). The power of the technique relies on the sensitivity of the EPR spectral shapes to the mobility of the label in the nanosecond time window described by the rotational correlation time τc (Figure 1B), a parameter that can be obtained by spectral simulation. Indeed the magnetic hyperfine interaction between the electron spin and the 14N nucleus is highly anisotropic. This anisotropy is fully averaged when the radical is highly mobile and the spectrum displays three narrow lines. When the mobility decreases, the averaging becomes partial and the lines broaden progressively until reaching a limit of a fully anisotropic spectrum corresponding to an immobilized spin label. A spectral modification thus represents a change in the environment of the label affecting its mobility and thus indicates a structural transition (Figure 1C).
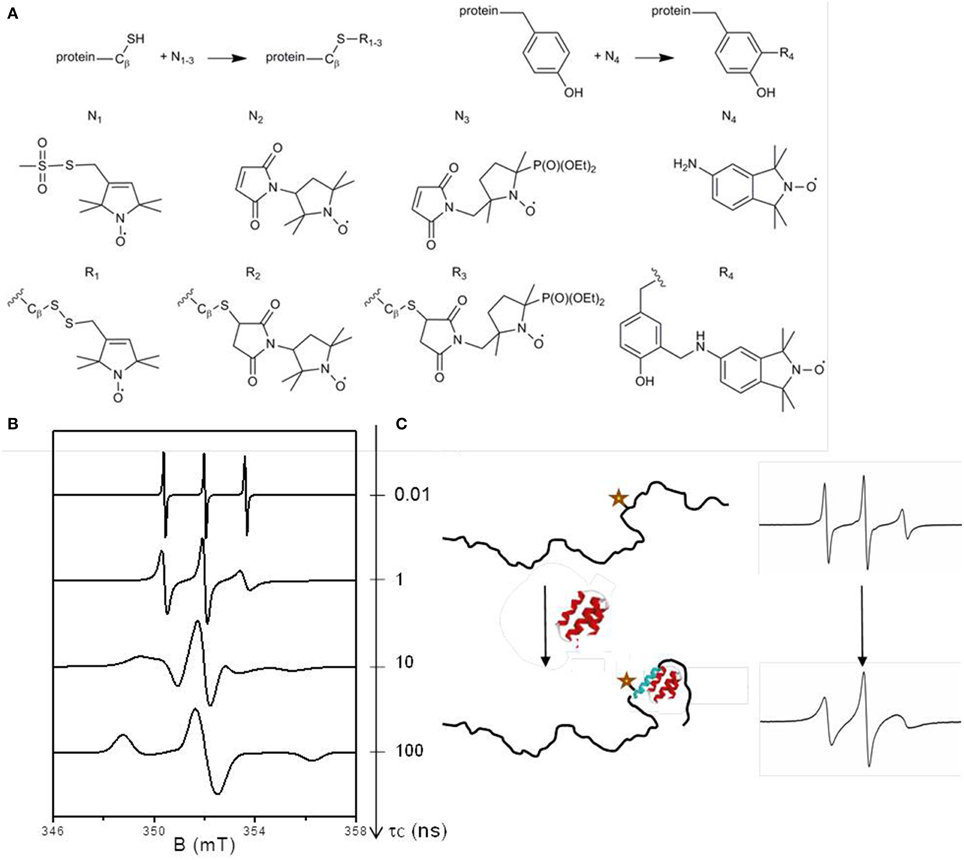
Figure 1. (A) Labeling reaction scheme for cysteine and tyrosine residues. N1−3 are spin labels for grafting on cysteine residues: N1 is the methanethiosulfonate spin label (MTSL), N2 the maleimido proxyl (P) and N3 the phosphorylated maleimido proxyl (PP). N4 is the an isoindoline-based nitroxide (Nox) dedicated to tyrosine labeling. R1−4 are the corresponding spin label side-chains. (B) EPR spectral shape modifications as a function of the mobility of the spin label described by its rotational correlation time τc. The spectra were simulated using the EasySpin software (Stoll and Schweiger, 2006) for different values of τc: 0.01, 1, 10, and 100 ns. (C) Illustration of an EPR spectral shape broadening in the case of a disorder-to-order transition due to an induced folding mechanism. The star represents the spin label.
The technique has still some limitations, in particular due to the chemical nature of the spin labels, which our recent works aim to overcome. One limitation comes from the poor diversity of EPR spectral shapes of nitroxide labels. The similarity of the 3-line spectral shapes precludes the simultaneous investigation of two different regions of a protein or two interacting proteins, a situation that can be encountered in allosteric mechanism in which ligand binding at one site influences binding at another site through a propagated structural change within the protein. To overcome this limitation, we designed and synthesized a new spin label based on a maleimido-functionalized β-phosphorylated nitroxide: the {2-(diethoxyphosphoryl)-5-[(2,5-dioxo-2,5-dihydro-1H-pyrrol-1-yl)methyl]-2,5 dimethylpyrrolidin-1-yl}oxidanyl, referred to as Phosphorylated Proxyl or PP (Figure 1A, N3). Thanks to the supplementary high magnetic coupling between the electron spin and the nuclear spin of 31P, this label gives a well-resolved 6-line spectrum composed of a doublet of triplets (Le Breton et al., 2014). Another limitation of conventional SDSL concerns the fact that only cysteines are specifically targeted by commercial nitroxides spin labels which is unsuitable when cysteines play roles either in the function and/or in structural elements (active sites, disulfide bridges) of the protein under study. We designed and synthesized various nitroxides to react with the phenol group of the tyrosine via a three-components Mannich type reaction (Lorenzi et al., 2011; Mileo et al., 2013a). The best results were obtained with an isoindoline-based nitroxide: 5-amino-1,1,3,3-tetramethyl-isoindolin-2-yloxyl, referred to as Nox (Figure 1A, N4), which has proved to be a good reporter of structural modifications (Mileo et al., 2013a). The successful applications of these two new nitroxides to report on structural transitions on IDPs will be presented in the next section.
Applications
Several IDPs have already been investigated by SDSL-EPR. This approach has been successfully used to reveal various structural states and higher-order organizations of flexible proteins involved in neurodegenerative diseases such as α-synuclein (Chen et al., 2007), amyloid-β peptide (Torok et al., 2002) and tau (Siddiqua and Margittai, 2010). Another example of IDP studied by SDSL-EPR is the small acid protein IA3 that acts as an inhibitor of the yeast proteinase A (Casey et al., 2014). In the following, results with two highly flexible proteins are reviewed to illustrate the potential of SDSL-EPR in the field of IDPs.
Cartography of Induced Folding
Most IDPs undergo an induced folding in presence of a partner protein i.e., a disorder-to-order transition that can be limited to a particular region. An illustrative example concerns the cartography of induced folding of nucleoproteins (N) from three viruses belonging to the Paramyxoviridae family, namely Measles (MeV), Nipah (NiV) and Hendra (HeV) viruses. Multiple copies of N are structurally organized to encapsidate the viral genome. The particular case of MeV nucleoprotein has been the most extensively studied by complementary biophysical approaches (Habchi and Longhi, 2012). MeV N consists of two regions: a N-terminal globular one (aa 1–400) and a C-terminal domain NTAIL (aa 401–525) that is fully disordered. This disordered part is essential for transcription and replication of the virus via interaction with the phosphoprotein of the viral polymerase complex. Our study was focused on the interaction between MeV NTAIL and the C-terminal part (X Domain) of the phosphoprotein PXD (aa 459–507 of P), which is constituted of 3 α-helices (Longhi et al., 2003). In order to precisely localize the regional folding that MeV NTAIL undergoes in the presence of PXD, we targeted 14 sites for spin-labeling (with MTSL) within NTAIL, 12 of which being concentrated in the C-terminal region (aa 488–525) that was known to be specifically involved in the interaction with MeV PXD (Longhi et al., 2003) (Figure 2A, upper panel). For all labeled variants of NTAIL, room temperature EPR spectra were recorded in the absence and presence of PXD (Belle et al., 2008). An important decrease of the mobility of the spin labels was observed in the 488–502 region that we have been able to attribute to the formation of an α-helix using complementary circular dichroism analyses. At one particular position (aa 491) a broad shape component was detected, indicating a very restricted environment of the spin label (Figure 2A, left panel). This observation allowed us to confirm the structural model of a chimera construct between MeV PXD and a small NTAIL region (aa 486–504) in which the amino acid side-chain 491 points toward PXD whereas the other ones are solvent-exposed (Kingston et al., 2004). Interestingly, the mobility of the induced folding region was found to be slightly but significantly restrained even in the absence of the partner protein, a behavior that could indicate the existence of a pre-structuration of this region. This observation has been further confirmed combining SDSL-EPR data and modeling of local rotation conformational space (Kavalenka et al., 2010) and also by NMR studies and modeling of this region as a dynamic equilibrium between a completely unfolded state and different partially helical conformations (Jensen et al., 2011). Concerning the C-terminal end of NTAIL, EPR spectral shapes of the labels grafted in the 505–525 region showed a moderate decrease of mobility that has been attributed to a gain of rigidity arising from α-helical folding of the neighboring 488–502 region (Belle et al., 2008). This observation was consistent with further analyses where the 505–525 region was found to conserve a significant degree of freedom even in the bound form (Kavalenka et al., 2010). Using the same strategy based on multiple individual labeling sites, we also mapped the induced folding of NTAIL in association with PXD for HeV and NiV viruses (Martinho et al., 2013) and validated previously proposed structural models obtained by homology modeling (Habchi et al., 2011).
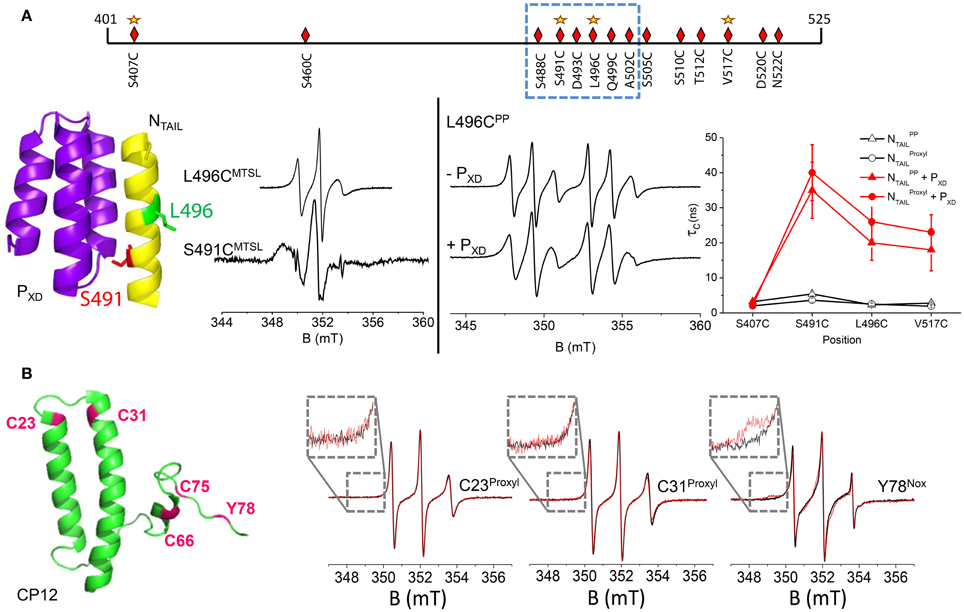
Figure 2. (A) upper panel: schematic representation of positions targeted for cysteine substitution and spin labeling of NTAIL (aa 401–525) with MSTL spin label (diamonds) and PP spin label (stars). The dotted frame indicates the region that undergoes an induced folding in the presence of the partner protein PXD. Left panel: Illustration of two EPR spectral shapes obtained in two positions of the MTSL within the induced folding region of NTAIL: positions 491 and 496. These two positions are highlighted in the crystal structure of the chimera construct between PXD and the NTAIL region encompassing residues 486–504 (pdb code 1T60). Right panel: EPR spectra of the phosphorylated proxyl grafted at position 496 in the absence and in the presence of PXD. Variation of the rotational correlation time τc of Proxyl (circles) and Phosphorylated Proxyl PP (triangles) spin labeled NTAIL variants without (black open symbols) and with (red filled symbols) saturating amounts of PXD as a function of spin label position. τc values have been obtained by simulating the EPR spectra using the program ROKY (Rockenbauer and Korecz, 1996). (B) Left panel: 3D structural model of C. reinhardtii CP12 (pdb 2DDN) in which the positions of the four cysteines and the unique tyrosine are highlighted in pink. Right panel: Superimposition of amplitude-normalized EPR spectra of CP12 C23Proxyl, C31Proxyl, and Y78Nox in absence (black) and presence of GAPDH in equimolar ratio (red). In inset: a zoom on the low-field region.
Thanks to the well-characterized MeV NTAIL-PXD interaction by conventional spin labels, this biological system was used as a model to characterize the new label PP, having a 31P atom in the vicinity of the nitroxide leading to a 6-line spectrum (Figure 1A, N3) and to probe its ability to report structural transitions. Four grafting sites on NTAIL were judiciously chosen (within and outside the induced folding region) (Figure 2A, upper panel). For comparative purposes, proxyl P (Figure 1A, side-chain R2) was grafted at the same positions. The ability of PP to report on structural transitions was evaluated by analyzing the spectral shape modifications induced either by a secondary structure stabilizer (trifluoroethanol) or by the presence of the partner protein PXD (Figure 2A, right panel). All the spectra were simulated to extract the dynamic parameter τc that represents the mobility of the spin labels. The modification of this parameter according to the position of the spin label and the condition (free or bound to PXD) was very similar for both the classical proxyl and the new phosphorylated spin labels (Figure 2B, right panel). Taken together the results demonstrated that PP is able to monitor from subtle to larger structural transitions, as efficiently as the classical spin label. Molecular dynamics (MD) calculations were performed to gain further insights into the binding process between the labeled NTAIL and PXD. MD calculations revealed that the new phosphorylated label does not perturb the interaction between the two partner proteins and reinforced the conclusion on its ability to probe different local environments in a protein (Le Breton et al., 2014). Thanks to its new EPR spectral signature, the combination of PP with classical spin labels opens the way to study two protein sites simultaneously.
Revealing Fuzziness in a Supramolecular Complex
In this section we will show how SDSL-EPR provides a unique tool to reveal regions of an IDP remaining highly flexible after complex formation, information that often escapes to detection by other biostructural techniques. This study concerns the structural flexibility of a small chloroplastic protein called CP12 (80 aa) and its association with the glyceraldehyde-3-phosphate dehydrogenase (GAPDH) from the green alga Chlamydomonas reinhardtii. This association is a key step in the formation of a ternary supramolecular complex involved in the regulation of the Calvin cycle in many photosynthetic organisms (for details see the review Tieulin-Pardo et al in the present journal). In its oxidized state the green alga CP12 contains four cysteine residues engaged in two disulfide bridges (C23–C31 and C66–C75) and presents some α-helical structural elements modeled from each side of the N-terminal disulfide bridge, whereas the C-terminal part appears mainly disordered (Figure 2B) (Gardebien et al., 2006). In contrast, in its reduced state the algal CP12 is fully disordered (Graciet et al., 2003). In a first study, we used MTSL (Figure 1A, N1), which led to the labeling of the only C-terminal cysteine residues leaving the N-terminal unmodified as revealed by mass spectrometry analyses. Surprisingly, the partner protein GAPDH induced the cleavage of the disulfide bridge between the cysteine and the label, resulting in the full release of the label. This result showed the existence of a transitory interaction between both proteins and we proposed a mechanism based on a thiol-disulfide exchange reaction involving cysteines C21 and C291 of the C. reinhardtii GAPDH (Erales et al., 2009). Even if this observation led us to propose a new role for the algal GAPDH, it however doesn't allow us to study the GAPDH-CP12 complex. As the central region of CP12 has been proposed to be involved in the interaction with GAPDH, we used two cysteine-to-serine mutants (C23S and C31S) that were known to be fully disordered, due to the loss of the N-terminal disulfide bridge, but still able to interact with GAPDH (Lebreton et al., 2006). In order to individually target the two N-terminal cysteine residues, proxyl P was chosen as spin label (Figure 1A, N2) to prevent label release induced by GAPDH. EPR analyses revealed that this part of the protein remains fully disordered after association with GAPDH as no spectral modification was detected (Figure 2B) (Mileo et al., 2013b). The association between the two partner proteins was checked by probing the accessibility of the labels using a reducing agent. Indeed, in the absence of GAPDH, spin labels grafted on CP12 are reduced by an excess of DTT and become EPR-silent with a characteristic time of about 25 min. On the contrary, if GAPDH is present in the sample, the same amount of DTT has almost no effect as the EPR signal remains stable for at least 2 h confirming that the association really takes place and that CP12 remains highly dynamic in its bound form (Mileo et al., 2013b).
The C-terminal region has been demonstrated to be mainly responsible for the redox regulation of GAPDH (Lebreton et al., 2006). To gain further insights into the dynamics of this region of CP12 while keeping the 2 disulfide bridges intact, tyrosine 78 was chosen as an alternative labeling site. The location of tyrosine 78, highly conserved in CP12s from different organisms, is particularly interesting as it is close to residues that play a crucial role in the activity modulation of GAPDH either in the binary complex (Erales et al., 2011) or in the ternary complex with PRK (Avilan et al., 2012). The new isoindoline-based nitroxide Nox (Figure 1A, N4) was used to selectively target this unique tyrosine. The presence of GAPDH led to a slight modification of the EPR spectral shape (Figure 2B) indicating that the interaction of the C-tail with the partner protein is not tight. This result showed however that this region is close to the interaction site without being directly involved in it (Mileo et al., 2013b). All together the analyses of the three labeling sites were in good agreement with the partial view of the CP12-GAPDH complexes from other organisms given by recent crystallographic and NMR studies where only the 20 last amino acids of CP12 were detected (Matsumura et al., 2011; Fermani et al., 2012). Finally, the use of different spin labels is this peculiar biological system allowed us to conclude that GAPDH-CP12 from C. reinhardtii is a new example of a fuzzy complex, a concept introduced by Tompa and Fuxreiter (2008), in which the IDP keeps most of its disorder and dynamics upon complex formation. This fuzziness could be one of the keys to facilitate the formation of a ternary complex (CP12, GAPDH and phosphoribulokinase, PRK) as well as functional actions of the machinery which necessitate dynamic assembly and disassembly processes controlled by dark/light transitions.
Conclusion
The examples described above, along with numerous other studies, illustrate the power of SDSL-EPR to access information on protein dynamics. Characterized by a remarkable conformational flexibility, IDPs form a unique protein category that is particularly suited for SDSL-EPR applications. SDSL-EPR is a rapidly growing field, in particular with recent developments focused on the detection of labeled protein in intact cells, an area that promises to be very interesting for IDPs applications. We can anticipate that these recent developments will create further need for the design of new labels with greater stability toward bio-reduction, an environment that is encountered inside the cell.
Conflict of Interest Statement
The authors declare that the research was conducted in the absence of any commercial or financial relationships that could be construed as a potential conflict of interest.
Acknowledgments
This work was supported by the Agence Nationale de la Recherche (ANR SPINFOLD n°09-BLAN-0100), the Centre National de la Recherche Scientifique (CNRS) and Aix-Marseille Université. The authors are grateful to the EPR facilities available at the national TGE RPE facilities (IR 3443). We thank our collaborators and their teams: R. Lebrun (Proteomic center of IMM, Marseille, France), S. Longhi (AFMB, CNRS-AMU UMR 7257, Marseille, France), J. Golebiowsky (ICN, CNRS Université de Nice UMR 7272, Nice, France), B. Gontero (BIP, CNRS-AMU UMR 7281, Marseille, France), S.R.A Marque (ICR, CNRS-AMU UMR 7273, Marseille, France) and A. Rockenbauer (Research Centre of Natural Sciences, Budapest, Hungary). NL is grateful to Aix-Marseille Université and PACA Region for her PhD fellowship.
References
Avilan, L., Puppo, C., Erales, J., Woudstra, M., Lebrun, R., and Gontero, B. (2012). CP12 residues involved in the formation and regulation of the glyceraldehyde-3-phosphate dehydrogenase-CP12-phosphoribulokinase complex in Chlamydomonas reinhardtii. Mol. Biosys. 8, 2994–3002. doi: 10.1039/c2mb25244a
Babu, M. M., Van Der Lee, R., De Groot, N. S., and Gsponer, J. (2011). Intrinsically disordered proteins: regulation and disease. Curr. Opin. Struc. Biol. 21, 432–440. doi: 10.1016/j.sbi.2011.03.011
Belle, V., Fournel, A., Woudstra, M., Ranaldi, S., Prieri, F., Thomé, V., et al. (2007). Probing the opening of the pancreatic lipase lid using site-directed spin labeling and EPR spectroscopy. Biochemistry 46, 2205–2214. doi: 10.1021/bi0616089
Belle, V., Rouger, S., Costanzo, S., Liquière, E., Strancar, J., Guigliarelli, B., et al. (2008). Mapping alpha-helical induced folding within the intrinsically disordered C-terminal domain of the measles virus nucleoprotein by site-directed spin-labeling EPR spectroscopy. Proteins 73, 973–988. doi: 10.1002/prot.22125
Bordignon, E. (2011). Site-directed spin labeling of membrane proteins. Top. Curr. Chem. 321, 121–157. doi: 10.1007/128_2011_243
Casey, T. M., Liu, Z. L., Esquiaqui, J. M., Pirman, N. L., Milshteyn, E., and Fanucci, G. E. (2014). Continuous wave W- and D-Band EPR spectroscopy offer “sweet-spots” for characterizing conformational changes and dynamics in intrinsically disordered proteins. Biochem. Bioph. Res. Com. 450, 723–728. doi: 10.1016/j.bbrc.2014.06.045
Chen, M., Margittai, M., Chen, J., and Langen, R. (2007). Investigation of alpha-synuclein fibril structure by site-directed spin labeling. J. Biol. Chem. 282, 24970–24979. doi: 10.1074/jbc.M700368200
Drescher, M. (2012). EPR in protein science intrinsically disordered proteins. EPR Spectrosc. Appl. Chem. Biol. 321, 91–119. doi: 10.1007/128_2011_235
Dyson, H. J., and Wright, P. E. (2002). Coupling of folding and binding for unstructured proteins. Curr. Opin. Struct. Biol. 12, 54–60. doi: 10.1016/S0959-440X(02)00289-0
Erales, J., Lorenzi, M., Lebrun, R., Fournel, A., Etienne, E., Courcelle, C., et al. (2009). A new function of GAPDH from Chlamydomonas reinhardtii: a thiol/disulfide exchange reaction with CP12. Biochemistry 48, 6034–6040. doi: 10.1021/bi900569h
Erales, J., Mekhalfi, M., Woudstra, M., and Gontero, B. (2011). Molecular mechanism of NADPH-Glyceraldehyde-3-phosphate dehydrogenase regulation through the C-Terminus of CP12 in Chlamydomonas reinhardtii. Biochemistry 50, 2881–2888. doi: 10.1021/bi1020259
Fermani, S., Trivelli, X., Sparla, F., Thumiger, A., Calvaresi, M., Marri, L., et al. (2012). Conformational selection and folding-upon-binding of intrinsically disordered protein CP12 regulate photosynthetic enzymes assembly. J. Biol. Chem. 287, 21372–21383. doi: 10.1074/jbc.M112.350355
Gardebien, F., Thangudu, R. R., Gontero, B., and Offmann, B. (2006). Construction of a 3D model of CP12, a protein linker. J. Mol. Graph. Model 25, 186–195. doi: 10.1016/j.jmgm.2005.12.003
Graciet, E., Gans, P., Wedel, N., Lebreton, S., Camadro, J. M., and Gontero, B. (2003). The small protein CP12: a protein linker for supramolecular complex assembly. Biochemistry 42, 8163–8170. doi: 10.1021/bi034474x
Gross, A., Columbus, L., Hideg, K., Altenbach, C., and Hubbell, W. L. (1999). Structure of the KcsA potassium channel from Streptomyces lividans: a site-directed spin labeling study of the second transmembrane segment. Biochemistry 38, 10324–10335. doi: 10.1021/bi990856k
Habchi, J., Blangy, S., Mamelli, L., Jensen, M. R., Blackledge, M., Darbon, H., et al. (2011). Characterization of the interactions between the nucleoprotein and the phosphoprotein of henipavirus. J. Biol. Chem. 286, 13583–13602. doi: 10.1074/jbc.M111.219857
Habchi, J., and Longhi, S. (2012). Structural disorder within paramyxovirus nucleoproteins and phosphoproteins. Mol. Biosys. 8, 69–81. doi: 10.1039/C1MB05204G
Habchi, J., Tompa, P., Longhi, S., and Uversky, V. N. (2014). Introducing protein intrinsic disorder. Chem. Rev. 114, 6561–6588. doi: 10.1021/cr400514h
Hideg, K., Kalai, T., and Sar, C. P. (2005). Recent results in chemistry and biology of nitroxides. J. Heterocyclic. Chem. 42, 437–450. doi: 10.1002/jhet.5570420311
Hubbell, W. L., Lopez, C. J., Altenbach, C., and Yang, Z. Y. (2013). Technological advances in site-directed spin labeling of proteins. Curr. Opin. Struc. Biol. 23, 725–733. doi: 10.1016/j.sbi.2013.06.008
Hubbell, W. L., Mchaourab, H. S., Altenbach, C., and Lietzow, M. A. (1996). Watching proteins move using site-directed spin labeling. Structure 4, 779–783. doi: 10.1016/S0969-2126(96)00085-8
Jensen, M. R., Communie, G., Ribeiro, E. A., Martinez, N., Desfosses, A., Salmon, L., et al. (2011). Intrinsic disorder in measles virus nucleocapsids. Proc. Natl. Acad. Sci. U.S.A. 108, 9839–9844. doi: 10.1073/pnas.1103270108
Kaplan, R. S., Mayor, J. A., Kotaria, R., Walters, D. E., and Mchaourab, H. S. (2000). The yeast mitochondrial citrate transport protein: determination of secondary structure and solvent accessibility of transmembrane domain IV using site-directed spin labeling. Biochemistry 39, 9157–9163. doi: 10.1021/bi000433e
Kavalenka, A., Urbancic, I., Belle, V., Rouger, S., Costanzo, S., Kure, S., et al. (2010). Conformational analysis of the partially disordered measles virus NTAIL-XD complex by SDSL EPR spectroscopy. Biophys. J. 98, 1055–1064. doi: 10.1016/j.bpj.2009.11.036
Kingston, R. L., Hamel, D. J., Gay, L. S., Dahlquist, F. W., and Matthews, B. W. (2004). Structural basis for the attachment of a paramyxoviral polymerase to its template. Proc. Natl. Acad. Sci. U.S.A. 101, 8301–8306. doi: 10.1073/pnas.0402690101
Klare, J. P., and Steinhoff, H. J. (2009). Spin labeling EPR. Photosynth. Res. 102, 377–390. doi: 10.1007/s11120-009-9490-7
Le Breton, N., Martinho, M., Kabytaev, K., Topin, J., Mileo, E., Blocquel, D., et al. (2014). Diversification of EPR signatures in site directed spin labeling using a beta-phosphorylated nitroxide. Phys. Chem. Chem. Phys. 16, 4202–4209. doi: 10.1039/c3cp54816c
Lebreton, S., Andreescu, S., Graciet, E., and Gontero, B. (2006). Mapping of the interaction site of CP12 with glyceraldehyde-3-phosphate dehydrogenase from Chlamydomonas reinhardtii. Functional consequences for glyceraldehyde-3-phosphate dehydrogenase. FEBS J. 273, 3358–3369. doi: 10.1111/j.1742-4658.2006.05342.x
Longhi, S., Receveur-Brechot, V., Karlin, D., Johansson, K., Darbon, H., Bhella, D., et al. (2003). The C-terminal domain of the measles virus nucleoprotein is intrinsically disordered and folds upon binding to the C-terminal moiety of the phosphoprotein. J. Biol. Chem. 278, 18638–18648. doi: 10.1074/jbc.M300518200
Lorenzi, M., Puppo, C., Lebrun, R., Lignon, S., Roubaud, V., Martinho, M., et al. (2011). Tyrosine-targeted spin labeling and EPR spectroscopy: an alternative strategy for studying structural transitions in proteins. Angew Chem. Int. Edit. 50, 9108–9111. doi: 10.1002/anie.201102539
Lorenzi, M., Sylvi, L., Gerbaud, G., Mileo, E., Halgand, F., Walburger, A., et al. (2012). Conformational selection underlies recognition of a molybdoenzyme by its dedicated chaperone. PLoS ONE 7:e49523. doi: 10.1371/journal.pone.0049523
Martinho, M., Habchi, J., El Habre, Z., Nesme, L., Guigliarelli, B., Belle, V., et al. (2013). Assessing induced folding within the intrinsically disordered C-terminal domain of the Henipavirus nucleoproteins by site directed spin labeling EPR spectroscopy. J. Biomol. Struc. Dyn. 31, 453–471. doi: 10.1080/07391102.2012.706068
Matsumura, H., Kai, A., Maeda, T., Tamoi, M., Satoh, A., Tamura, H., et al. (2011). Structure basis for the regulation of Glyceraldehyde-3-Phosphate dehydrogenase activity via the intrinsically disordered protein CP12. Structure 19, 1846–1854. doi: 10.1016/j.str.2011.08.016
Mchaourab, H. S., Lietzow, M. A., Hideg, K., and Hubbell, W. L. (1996). Motion of spin-labeled side chains in T4 lysozyme. Correlation with protein structure and dynamics. Biochemistry 35, 7692–7704. doi: 10.1021/bi960482k
Mchaourab, H. S., Steed, P. R., and Kazmier, K. (2011). Toward the fourth dimension of membrane protein structure: insight into dynamics from spin-labeling EPR spectroscopy. Structure 19, 1549–1561. doi: 10.1016/j.str.2011.10.009
Mileo, E., Etienne, E., Martinho, M., Lebrun, R., Roubaud, V., Tordo, P., et al. (2013a). Enlarging the panoply of site-directed spin labeling electron paramagnetic resonance (SDSL-EPR): sensitive and selective spin-labeling of tyrosine using an isoindoline-based nitroxide. Bioconj. Chem. 24, 1110–1117. doi: 10.1021/bc4000542
Mileo, E., Lorenzi, M., Erales, J., Lignon, S., Puppo, C., Le Breton, N., et al. (2013b). Dynamics of the intrinsically disordered protein CP12 in its association with GAPDH in the green alga Chlamydomonas reinhardtii: a fuzzy complex. Mol. Biosys. 9, 2869–2876. doi: 10.1039/c3mb70190e
Pannier, M., Veit, S., Godt, A., Jeschke, G., and Spiess, H. W. (2000). Dead-time free measurement of dipole-dipole interactions between electron spins. J. Magn. Reson. 142, 331–340. doi: 10.1006/jmre.1999.1944
Rockenbauer, A., and Korecz, L. (1996). Automatic computer simulations of ESR spectra. Appl. Magn. Reson. 10, 29–43. doi: 10.1007/BF03163097
Salmon, L., Nodet, G., Ozenne, V., Yin, G., Jensen, M. R., Zweckstetter, M., et al. (2010). NMR characterization of long-range order in intrinsically disordered proteins. Proc. Natl. Acad. Sci. U.S.A. 132, 8407–8418. doi: 10.1021/ja101645g
Siddiqua, A., and Margittai, M. (2010). Three- and four-repeat tau coassemble into heterogeneous filaments: an implication for Alzheimer disease. J. Biol. Chem. 285, 37920–37926. doi: 10.1074/jbc.M110.185728
Stoll, S., and Schweiger, A. (2006). EasySpin, a comprehensive software package for spectral simulation and analysis in EPR. J. Magn. Reson. 178, 42–55. doi: 10.1016/j.jmr.2005.08.013
Tompa, P., and Fuxreiter, M. (2008). Fuzzy complexes: polymorphism and structural disorder in protein-protein interactions. Trends Biochem. Sci. 33, 2–8. doi: 10.1016/j.tibs.2007.10.003
Torok, M., Milton, S., Kayed, R., Wu, P., Mcintire, T., Glabe, C. G., et al. (2002). Structural and dynamic features of Alzheimer's A beta peptide in amyloid fibrils studied by site-directed spin labeling. J. Biol. Chem. 277, 40810–40815. doi: 10.1074/jbc.M205659200
Keywords: intrinsically disordered proteins, structural transitions, induced folding, fuzzy complex, nitroxide spin labels, site-directed spin labeling EPR spectroscopy
Citation: Le Breton N, Martinho M, Mileo E, Etienne E, Gerbaud G, Guigliarelli B and Belle V (2015) Exploring intrinsically disordered proteins using site-directed spin labeling electron paramagnetic resonance spectroscopy. Front. Mol. Biosci. 2:21. doi: 10.3389/fmolb.2015.00021
Received: 26 March 2015; Accepted: 03 May 2015;
Published: 19 May 2015.
Edited by:
Peter Tompa, Flanders Institute of Biotechnology (VIB), BelgiumReviewed by:
Patrick Senet, Université de Bourgogne, FranceSteffen P. Graether, University of Guelph, Canada
Copyright © 2015 Le Breton, Martinho, Mileo, Etienne, Gerbaud, Guigliarelli and Belle. This is an open-access article distributed under the terms of the Creative Commons Attribution License (CC BY). The use, distribution or reproduction in other forums is permitted, provided the original author(s) or licensor are credited and that the original publication in this journal is cited, in accordance with accepted academic practice. No use, distribution or reproduction is permitted which does not comply with these terms.
*Correspondence: Valérie Belle, Bioénergétique et Ingénierie des Protéines Laboratory UMR 7281, Aix-Marseille Université and Centre National de la Recherche Scientifique, 31 chemin J. Aiguier, 13402 Marseille, France,YmVsbGVAaW1tLmNucnMuZnI=