- 1Faculty of Graduate Studies, Chengde Medical University, Chengde, China
- 2Department of Immunology, Basic Medical Institute, Chengde Medical University, Chengde, China
- 3Reproductive Medicine Center, Department of Obstetrics and Gynecology, Peking University International Hospital, Beijing, China
- 4CAS Key Laboratory of Genome Sciences and Information, Beijing Institute of Genomics, Chinese Academy of Sciences, Beijing, China
In the field of assisted reproductive technology, female fertility preservation, particularly ovarian tissue cryopreservation in adolescent cancer patients, has attracted much attention. Melatonin (MLT) is well known for its antioxidative and anti-apoptotic properties; however, whether it can ameliorate the cryoinjury and inhibit the generation of reactive oxygen species (ROS) in cryopreserved ovarian tissues (OTs) has not yet been reported. Here, we demonstrated that MLT could protect follicular integrity; prevent cell apoptosis; decrease ROS, malondialdehyde (MDA), and nitric oxide (NO) levels; and increase activities of glutathione peroxidases (GSH-Px), glutathione (GSH), catalase (CAT), and superoxide dismutase (SOD) in cryopreserved OTs. Furthermore, these effects may be related with the activation of the nuclear factor erythroid 2-related factor 2 (Nrf2) signaling pathway, as evidenced by increased mRNA levels of Nrf2 downstream genes, including heme oxygenase-1 (HO-1), glutathione S-transferase M1 (GSTM1), SOD, and CAT. In summary, MLT can not only directly scavenge ROS but also significantly induce the activation of antioxidative enzymes via the Nrf2 signaling pathway, which is a new mechanism underlying the protection effects of MLT on cryopreserved OTs.
Introduction
Nowadays, female fertility preservation still remains a challenge, particularly in the case of some cancer patients (Grynberg et al., 2018). Especially for prepubertal girls with cancer, whose chemotherapy and radiotherapy cannot be delayed, cryopreservation of OTs seems to be the only fertility preservation treatment modality (Van Den Heuvel-Eibrink et al., 2018; Pang et al., 2020). The development of new cryopreservation technology for tissues brings hope and enlightenment to the field of female fertility preservation. To date, 86 successful births and 9 ongoing pregnancies have been reported in women using frozen-thawed OTs worldwide (Anderson et al., 2017; Donnez and Dolmans, 2017; Jensen et al., 2017).
Cryopreservation of OTs results in physical and chemical stress, which causes morphological changes and damages phospholipid membrane, and mitochondria of follicles (Rajabi et al., 2018). Moreover, cryopreservation induces ROS generation that finally leads to oxidative stress, which affects the recovery efficiency and clinical pregnancy rate (Agarwal et al., 2012; Won et al., 2013; Grynberg et al., 2018; Van Den Heuvel-Eibrink et al., 2018; Pang et al., 2020). The production of ROS by oocytes and somatic cells and the reduced levels of antioxidant enzymes in the follicular microenvironment may induce follicular apoptosis (Gao et al., 2020; Yang et al., 2020). Some antioxidants (such as MLT and resveratrol) have been shown to counteract the negative effects of cryopreservation on the quantity and quality of OTs, probably through the enhancement of free radical scavenging mechanisms in the follicles (Tarín et al., 2002; Rocha et al., 2018; Olcese, 2020). Therefore, antioxidants are required for elimination of ROS (Tamura et al., 2020).
Melatonin (N-acetyl-5-methoxytryptamine), one of the most well-studied molecules in recent years, is mainly synthesized and secreted by pineal organ of animal and human. It acts as an antioxidant in several reproductive organs and can directly scavenge a variety of free radicals (Reiter et al., 2016; Tamura et al., 2020). It also functions to upregulate antioxidant protein expression and to protect against oxidant-mediated damage in many cryopreserved sperm/tissues (human sperm and ovary) (Li et al., 2009; Wang et al., 2012; Gao et al., 2016; Deng et al., 2017; Goodarzi et al., 2017). It is reported that oxidative stress is one of the most frequent side effects that induce follicular morphological changes and cell apoptosis in cryopreserved OTs (Goodarzi et al., 2017). Some studies demonstrated that MLT, as a highly lipophilic molecule, can easily cross the cell membranes and show antioxidant and anti-apoptotic effects (Kleszczyński et al., 2016; Reiter et al., 2016; Zhang et al., 2019; Tamura et al., 2020). Moreover, some recent studies have reported the beneficial effects of MLT on improving the preservation of OTs and auto-transplantation (Abir et al., 2017; Goodarzi et al., 2017; Gül et al., 2018). Nevertheless, the specific mechanisms of MLT underlying its protective role in stress-induced oxidative damage of follicles are less studied.
In this study, the effect and mechanism of MLT on follicles from cryopreserved OTs were investigated. We analyzed (a) follicle integrity, apoptotic rate, and antioxidant enzyme activities and (b) whether MLT can prevent the ROS production by activating the nuclear factor E2-related factor 2 (Nrf2) signaling pathway and its downstream antioxidant enzymes such as HO-1, SOD, CAT, and GSTM1.
Materials and Methods
Reagents
All chemical reagents used in this study were purchased from Sigma-Aldrich (St. Louis, MO, United States) unless otherwise stated.
Animals and Experimental Design
Forty healthy female adolescent Sprague–Dawley (SD) rats (Certificate No. 11401300084289) were used. Ovaries (n = 80) were collected from these rats aseptically. The experimental protocols were approved by the Animal Ethics Committee of the Chengde Medical University (Chengde, China). Immediately after collection, the whole ovaries were washed with 70% alcohol for 10 s, washed twice in PBS, and kept at 4°C (Rocha et al., 2018).
Melatonin powder was dissolved in dimethyl sulfoxide (DMSO) at different concentrations and then further diluted 1:10 with vitrification solution-1 (SV-1) and vitrification solution-2 (SV-2) to yield a final concentration. The ovarian cortex was cut into approximately 3 × 3 × 1-mm fragments, randomly divided to six groups, and vitrified using different MLT concentrations [I: fresh control (n = 5), ovarian fragments immediately fixed; II: 0 mM MLT-untreated group (n = 15); III: 0.001 mM MLT-treated group (n = 15); IV: 0.01 mM MLT-treated group (n = 15); V: 0.1 mM MLT-treated group (n = 15); VI: 1 mM MLT-treated group (n = 15)]. After the freeze-thaw process, the ovarian fragments were subjected to histological analysis and TUNEL (TdT-mediated dUTP nick-end labeling) (n = 25), Western blotting (n = 25), and ROS level (n = 25) analysis. Each experiment was repeated at least three times (Chaves et al., 2008).
Vitrification and Warming Procedures
The compositions and exposure times of all cryopreserved OTs are presented in Table 1. The vitrification and warming protocols were based on the previous study (Rocha et al., 2018). At the first step, the fragments were equilibrated in SV-1 and then transferred to a SV-2. The treatment with MLT used the same solutions (SV-1 and SV-2) at 0.001-mM, 0.01-mM, 0.1-mM, and 1-mM concentrations. Ovarian fragments were immersed in SV-1 for 4 min and then to SV-2 for 1 min. All the procedures were carried out at room temperature. At last, the ovarian fragments were placed in a cryopreservation tube with SV-2, exposed to nitrogen vapor for 30–60 min (−30°C), and then directly stored in liquid nitrogen (−196°C).
After 2 weeks of storage, all samples were thawed as described. The OTs were rapidly immersed in a water bath at 38°C for 1–2 min. The OTs were subjected to removal of SV-2 by using washing solutions (WS-1, WS-2, and WS-3) (Rocha et al., 2018). All OTs were kept in each solution for 5 min and then incubated for 1–12 h in a culture dish containing a base medium supplemented with PBS and 20% FBS (Won et al., 2013).
Histological Analysis
All freeze-thawed OTs were recovered and fixed in 4% paraformaldehyde for 1 h, embedded in a paraffin block, and serially sectioned at a thickness of 4–5 μm. The sections were stained with hematoxylin–eosin, mounted, and observed under light microscopy (Olympus, Tokyo, Japan) at ×400 magnification.
Each preantral follicle type was classified according to the developmental stage (Chaves et al., 2008; Won et al., 2013):
a) Primordial follicle: oocyte surrounded by a single layer of flattened pre-granulosa cells.
b) Primary follicle: single layer of granulosa cells and oocyte surrounded by one layer of cuboidal cells.
c) Secondary follicle: oocyte surrounded by two or more layers of cuboidal granulosa cells.
d) Antral follicle: multiple layers of cuboidal granulosa cells.
The follicles were evaluated with the following morphological criteria (Gandolfi et al., 2006):
a) integrity of each follicle with oocyte and granulosa cells.
b) the presence or absence of pyknotic bodies.
c) cytoplasmic retraction.
d) the organization of granulosa cells.
Based on this evaluation, when the oocytes with non-pyknotic nuclei were surrounded by granulosa cells organized in discrete layers, they were classified as morphologically normal follicles. Degenerated follicles (abnormal) were defined as those with retracted cytoplasm, pyknotic nucleus, or disorganized granular cells detached from the basal membrane (Wang et al., 2001; Won et al., 2013). The diameters of follicles and oocytes were measured and calculated based on the average of two perpendicular axes of each morphological integrity of follicles. The ratio of morphologically intact follicles (%) was expressed as follows: the number of morphological integrity of follicles and oocytes/the total number of follicles and oocytes × 100%.
Analysis of Apoptosis by TUNEL Assays
Cell apoptosis was analyzed by using TUNEL kit (Roche Diagnostics, Mannheim, Germany). Apoptotic follicular and oocytes were indicated by brown-yellow granules in the cytoplasm. The number of apoptotic follicles and oocytes in random fields of view (magnification, ×1000) was calculated. The apoptosis rate was expressed as follows: the number of apoptotic follicles and oocytes/the total number of follicles and oocytes × 100%.
Western Blot Analysis
Frozen ovarian fragments were thawed and mixed with cracking liquid and centrifuged at 12,000 rpm at 4°C. Proteins in the liquid supernatant were quantitated via BCA Protein Assay Kit (Beijing Solarbio Science & Technology Co., Ltd., China). SDS-PAGE (12% protein gels) protein electrophoresis was performed, and then proteins were transferred to a PVDF membrane. After blocking with 5% non-fat milk for 60 min at room temperature, the membrane was incubated overnight at 4°C with 0.2 μg/mL rabbit anti-Bcl-2 antibody (ab59348), rabbit anti-Bax antibody (ab53154) (Abcam), and rabbit anti-GAPDH polyclonal antibody. After washing, the samples were incubated for 120 min with goat anti-rabbit IgG H&L (HRP) (ab205718). Proteins were visualized using Ultra ECL Kit [MultiSciences (LIANKE) BIOTECH, CO., LTD., Hangzhou, China] and exposed with photographic film. The images were analyzed with BandScan 5.0 software. The ratios of the gray values of the target protein to those of GAPDH were calculated as the relative level of target protein.
Total RNA Isolation and First-Strand cDNA Synthesis
Total RNA was extracted from cryopreserved OTs using TRIzol reagent (Invitrogen, Carlsbad, CA, United States) as described previously (Kleszczyński et al., 2016). The yield of total RNA for each sample was quantified with a NanoDrop ND-1000 spectrophotometer (Thermo Fisher Scientific, Wilmington, DE, United States). First-strand cDNA of the total RNA was synthesized by reverse transcription using the SuperScriptTM III First-Strand Synthesis System Kit (Invitrogen, Carlsbad, CA, United States).
Quantitative Real-Time PCR Based on Gene Expression Analysis
Quantitative real-time PCR was performed for the detection of Nrf2, HO-1, SOD, CAT, GSTM1, Bcl-2, and Bax mRNA expression using the LightCycler 480 II System (Roche, Mannheim, Germany) and Maxima SYBR Green qPCR Master Mix (Fermentas GmbH, St. Leon-Rot, Germany). The primers in this study are described in Table 2, and actin was used as the reference. The cDNA (10 ng) was used as a template for each sample. The amplification conditions were set as follows: 95°C for 10 min, followed by 50 cycles of denaturation at 95°C for 15 s, annealing for 30 s at 59°C, and extension for 30 s at 72°C. Relative expression of the genes was calculated with the 2-ddCt method.
Measurement of ROS Generation and Antioxidant Enzyme Activities
Ovarian fragments were prepared as 10% tissue homogenates in normal saline and centrifuged at 3000 rpm at 4°C for 15 min. The supernatant was collected. The levels of ROS, GSH-Px, SOD, GSH, and CAT, as well as MDA, NO, and total antioxidant capacity (T-AOC) in OTs were measured with antioxidant kits (Nanjing Jiancheng Bioengineering Institute). Absorbance at the corresponding wavelength was determined by a microplate reader (Deng et al., 2017; Rocha et al., 2018;Yu et al., 2019).
Cytotoxicity Assessment
The lactate dehydrogenase (LDH) released from cells to the culture medium was measured by Cytotoxicity Detection Kit (LDH) (Nanjing Jiancheng Bioengineering Institute, Nanjing, China) and evaluated with ELISA reader at 492 nm following the manufacturer’s instructions.
Statistical Analysis
All statistical analyses were performed using SPSS version 22.0 (IBM Inc., Armonk, NY, United States). Data were expressed as mean (±standard error of the mean) of three independent experiments and presented with tables and bar graphs. The differences among the groups were analyzed by one-way analysis of variance (ANOVA), and results were considered significant when P < 0.05.
Results
Morphological Analysis of the MLT-Treated and Cryopreserved OTs
The procedure of freeze-thaw OTs will produce a large number of ROS, cause damage to various stages of developmental follicles, and ultimately compromise the outcome of OT transplantation and pregnancy rate. The morphological changes were measured in vitrified OTs. The morphology of follicles and oocytes was irregular and deformed, with granular cells arranged loosely and detached from the stromal membrane (Figure 1). In all of the five groups, the number of follicles at various stages with morphological integrity significantly decreased and the diameters of follicles and oocytes increased compared to fresh OTs (Figures 2A–C). However, the percentages of morphologically intact follicles in MLT-treated groups were markedly increased by ∼40% (0.001 mM, P < 0.05), ∼45% (0.01 mM, P < 0.05), ∼65% (0.1 mM, P < 0.01), and ∼66% (1 mM, P < 0.01), respectively, compared with those in the non-MLT-treated group (Figure 2A). These results indicate that 0.1 mM and 1 mM MLT can effectively protect the follicular integrity.
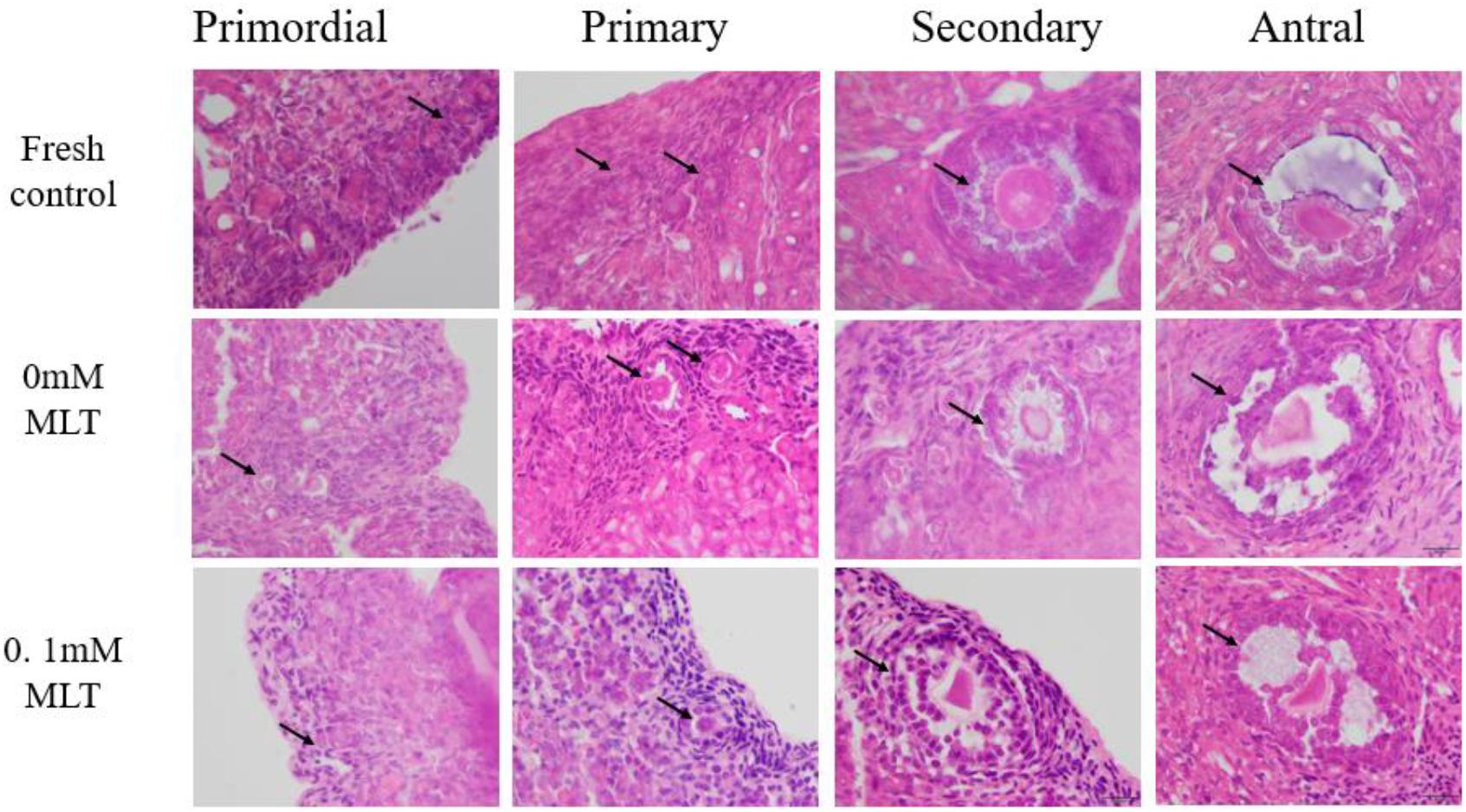
Figure 1. MLT protected the morphological integrity of follicles at various developmental stages in cryopreserved OTs. The horizontal panel represents the developmental stage of follicles. The vertical panel represents three groups (fresh, 0 mM, and 0.1 mM), respectively.
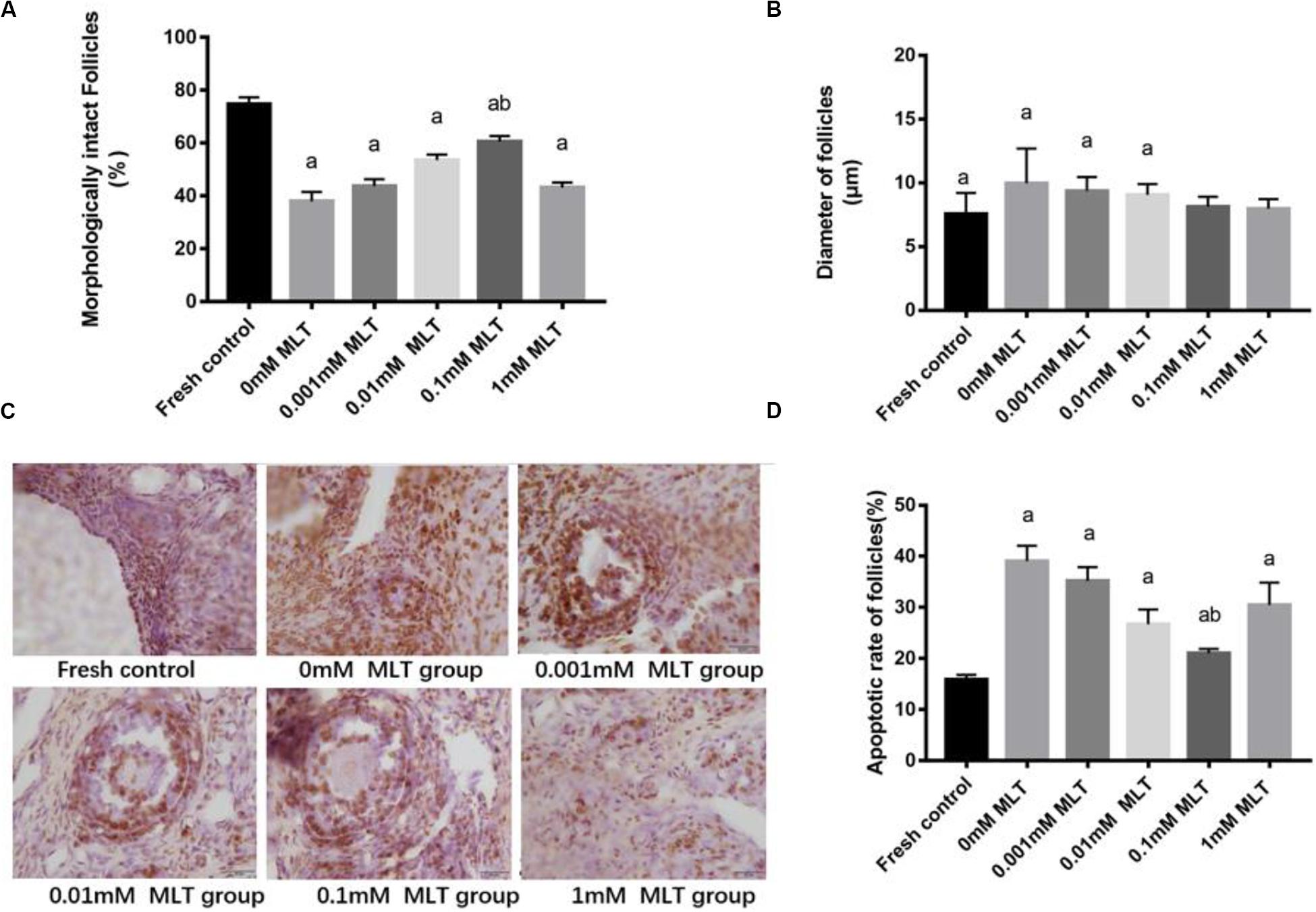
Figure 2. MLT protected the morphological integrity, alleviated the diameter change of follicles, and reduced the apoptotic rate in cryopreserved OTs. (A) Percentage of morphologically intact follicles in fresh control and each MLT-(un)treated group. (B) Diameter of follicles (μm) in fresh control and each MLT-(un)treated group. (C) Morphological integrity of follicles in fresh control and each MLT-(un)treated group. (D) Percentage of apoptotic rate in each MLT-(un)treated group. Data are shown as the mean ± SEM. a P < 0.05 compared with the 0-mM MLT-untreated group; b P < 0.05 compared with the MLT-treated group.
In addition to protecting follicles at various stages, MLT decreased the apoptotic rates during TUNEL assays in Figure 2D. Significant decreases in apoptotic rates (P < 0.005) were shown in MLT-treated groups, among which 0.1 mM MLT had the optimal effect (P < 0.001), which was consistent with the morphological analysis results in Figures 1, 2A–C.
Expression Levels of Bax and Bcl-2 in Cryopreserved OTs
Bax and Bcl-2 expression levels were further detected with RT-PCR and Western blot. The mRNA and protein expressions of Bax in the 0.1-mM MLT group significantly declined compared to those in the 0-mM MLT group (P < 0.01) and the other groups (P < 0.05, in Figures 3A,C,E). Figures 3B,D,E showed a statistically significant increase in Bcl-2 mRNA and protein expressions in the 0.1-mM MLT group (P < 0.05). These results suggest that 0.1 mM MLT has an optimal anti-apoptosis effect when MLT is used as cryoprotectant.
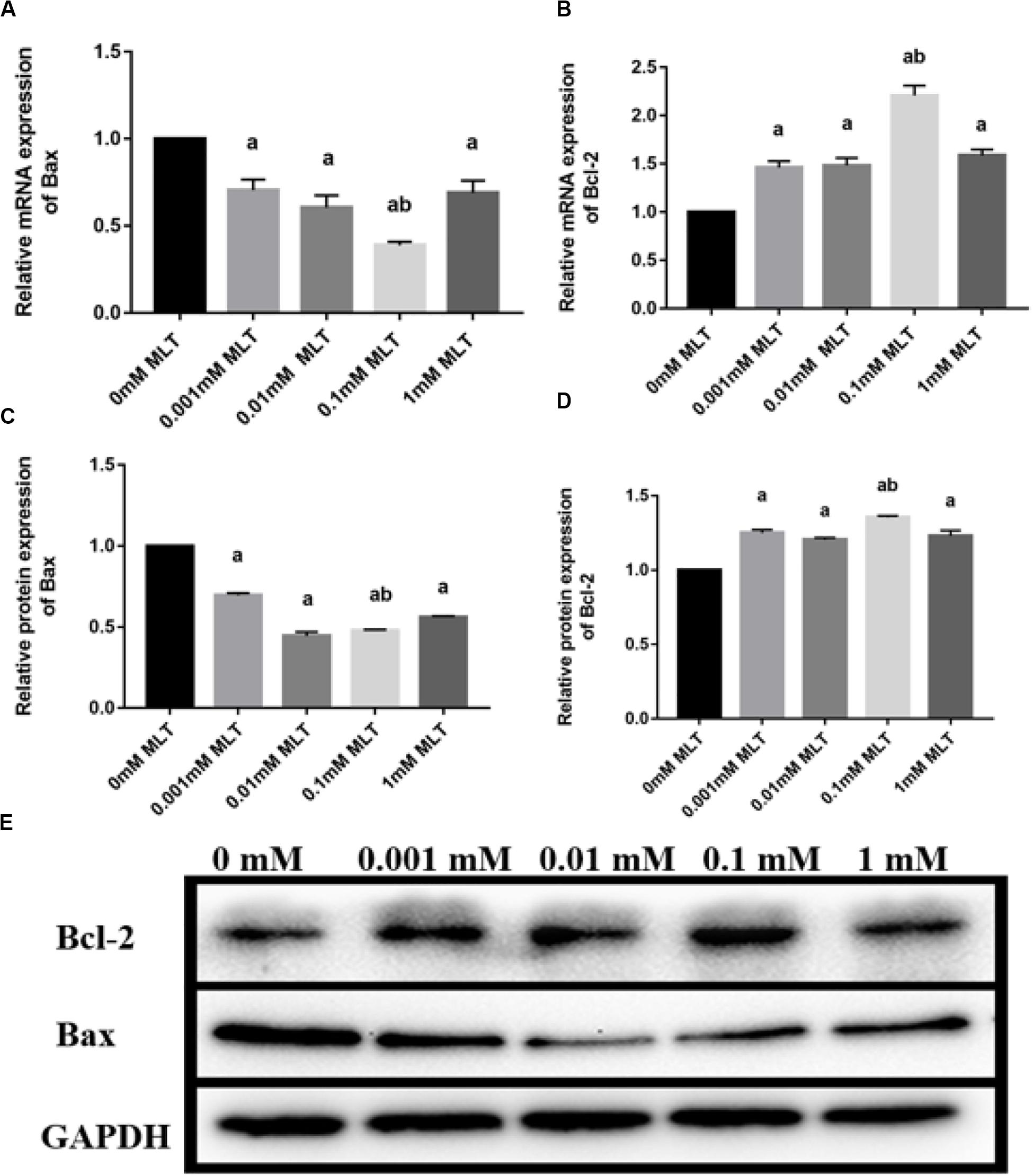
Figure 3. Effect of MLT on mRNA and protein expressions of Bax and Bcl-2 in cryopreserved OTs. (A) Relative mRNA expressions of Bax in each MLT-(un)treated group. (B) Relative mRNA expressions of Bcl-2 in each MLT-(un)treated group. (C) Protein expressions of Bax in each MLT-(un)treated group. (D) Protein expressions of Bcl-2 in each MLT-(un)treated group. (E) Representative Western blot image of Bax and Bcl-2 in each MLT-(un)treated group. Data are shown as the mean ± SEM. a P < 0.05 compared with the 0-mM MLT-untreated group; b P < 0.05 compared with the MLT-treated group.
Effect of MLT on Oxidative Stress in Cryopreserved OTs
In order to detect the oxidative damage status of cryopreserved OTs, the levels of ROS, MDA, and NO were analyzed. ROS generation was decreased after pretreatments with MLT by ∼35% (0.001 mM MLT, P < 0.01), ∼28% (0.01 mM MLT, P < 0.01), ∼18% (0.01 mM MLT, P < 0.001), and ∼25% (1 mM MLT, P < 0.01), respectively (Figure 4A). As shown in Figures 4B,C, similar results were found for the levels of MDA and NO compared with the 0-mM MLT group (P < 0.01). Meanwhile, consistent with the findings mentioned above, the highest antioxidant activity was observed in the 0.1-mM MLT group.
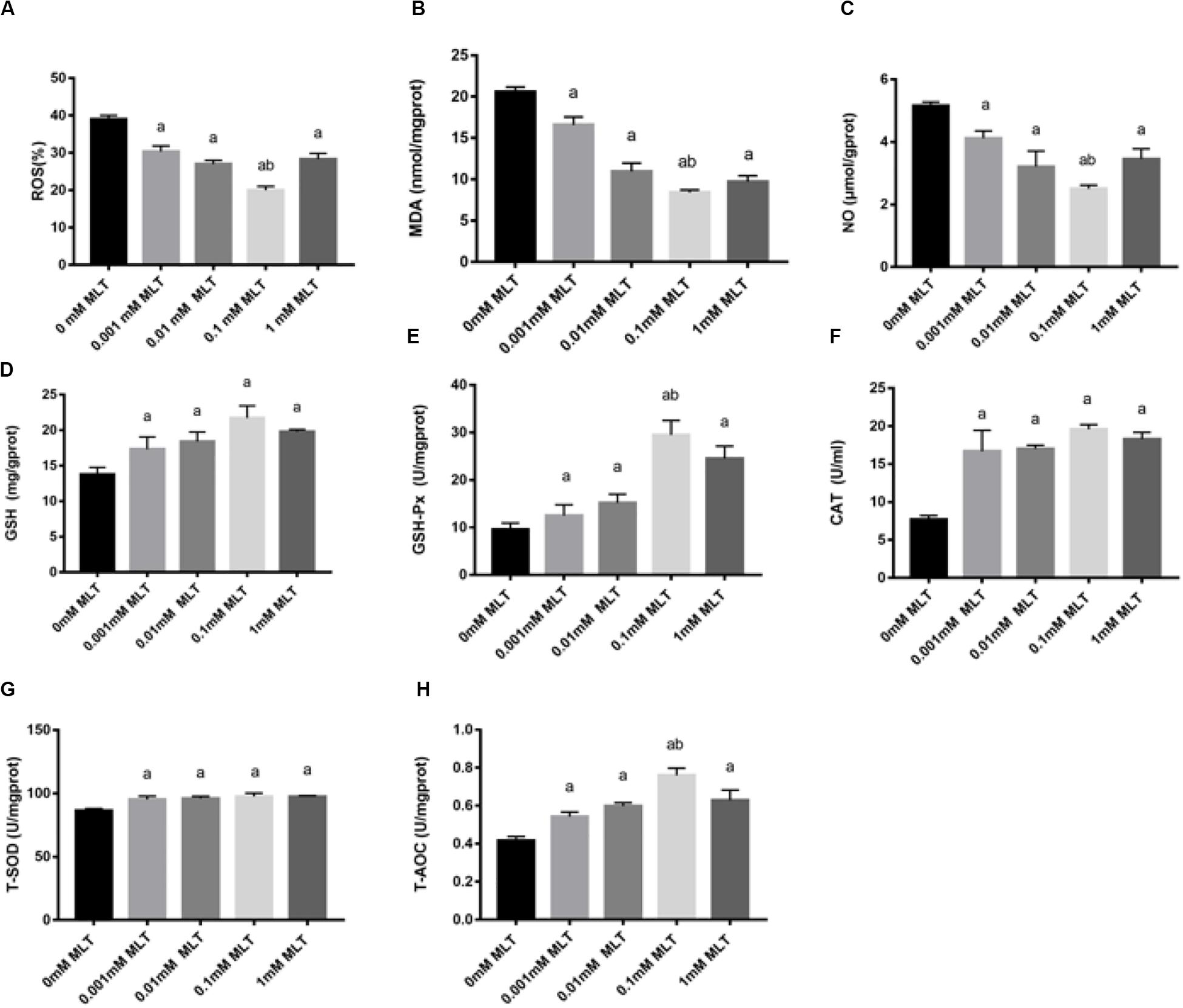
Figure 4. MLT alleviated oxidative stress in cryopreserved OTs. ROS (A), MDA (B), NO (C), GSH (D), GSH-Px (E), CAT (F), T-SOD (G), and T-AOC (H). Data are shown as the mean ± SEM. a P < 0.05 compared with the 0-mM MLT-untreated group; b P < 0.05 compared with the MLT-treated group.
In addition, activities of GSH, GSH-Px, CAT, SOD, and T-AOC were also measured in order to detect the antioxidant capacity of MLT in cryopreserved OTs. As shown in Figures 4D–H, the activities of GSH, GSH-Px, CAT, T-SOD, and T-AOC significantly decreased in the groups treated with various concentrations of MLT (especially at the 0.1 mM), compared with those in the 0-mM MLT group (P < 0.01).
MLT Promotes Nrf2 and Its Downstream Related Gene Expression in Cryopreserved OTs
Melatonin has been well investigated as a strong antioxidant, which is involved in many activities of protection via the Nrf2 signaling pathway. To determine the effect of MLT on key genes in the Nrf2 signaling pathway, RT-PCR was performed. As shown in Figure 5A, the level of Nrf2 mRNA in the 0.1-mM MLT group was significantly higher than those in the 0-mM MLT group (P < 0.01) or other groups with different concentrations of MLT (P < 0.001).
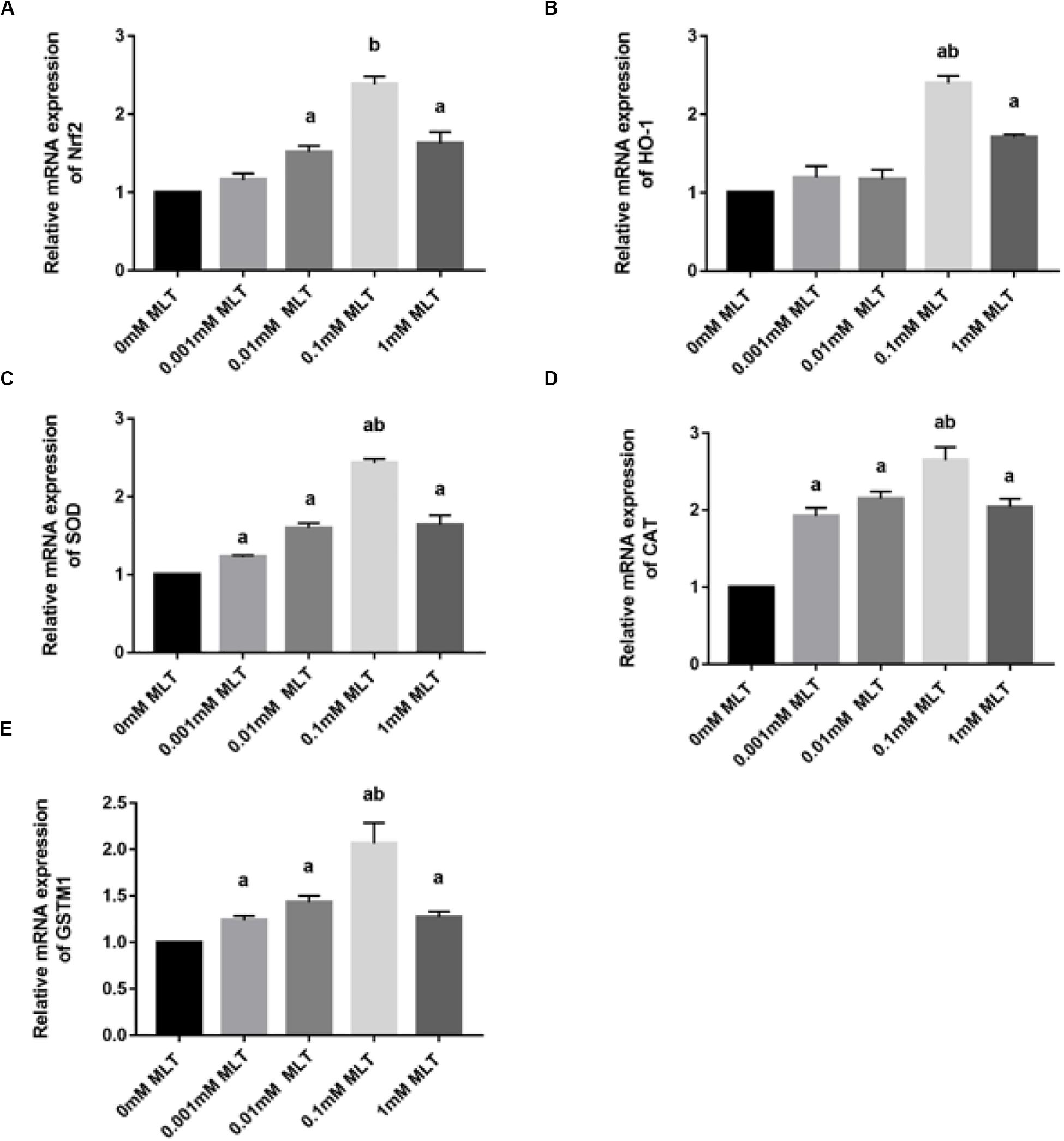
Figure 5. MLT affects Nrf2 and its related gene expressions in cryopreserved OTs. (A) Relative mRNA expressions of Nrf2 in each MLT-(un)treated group. (B) Relative mRNA expressions of HO-1 in each MLT-(un)treated group. (C) Relative mRNA expressions of SOD in each MLT-(un)treated group. (D) Relative mRNA expressions of CAT in each MLT-(un)treated group. (E) Relative mRNA expressions of GSTM1 in each MLT-(un)treated group. Data are shown as the mean ± SEM. a P < 0.05 compared with the 0-mM MLT-untreated group; b P < 0.05 compared with the MLT-treated groups.
A similar pattern of regulation was observed at the gene expression levels of Nrf2 downstream target genes including HO-1, SOD, CAT, and GSTM1 (Figures 5B–E). A striking gene upregulation was immediately observed in the MLT-treated groups (P < 0.001). Moreover, MLT at the dose of 0.1 mM, which was considered as the optimal MLT concentration, upregulated the gene expression of analyzed enzymes directly by ∼2.5 times (HO-1 and SOD, P < 0.001, Figures 5B,C) and ∼1.5 times (CAT P < 0.01, Figure 5D). However, the expression surge of GSTM1 mRNA was not as obvious as that of other Nrf2 downstream genes, although it also showed a higher level at the concentration of 1 mM MLT than other groups (Figure 5E).
MLT Cytotoxic Analysis
Safety, especially no cytotoxicity, is always one of the biggest concerns of all kinds of cryoprotectants. In order to verify whether MLT can produce a cytotoxic effect on OTs as a cryoprotective additive, the content of LDH was measured. Although the content of LDH increased slightly after adding MLT, there were no significant differences between MTL-treated groups (P > 0.05) (Figure 6).
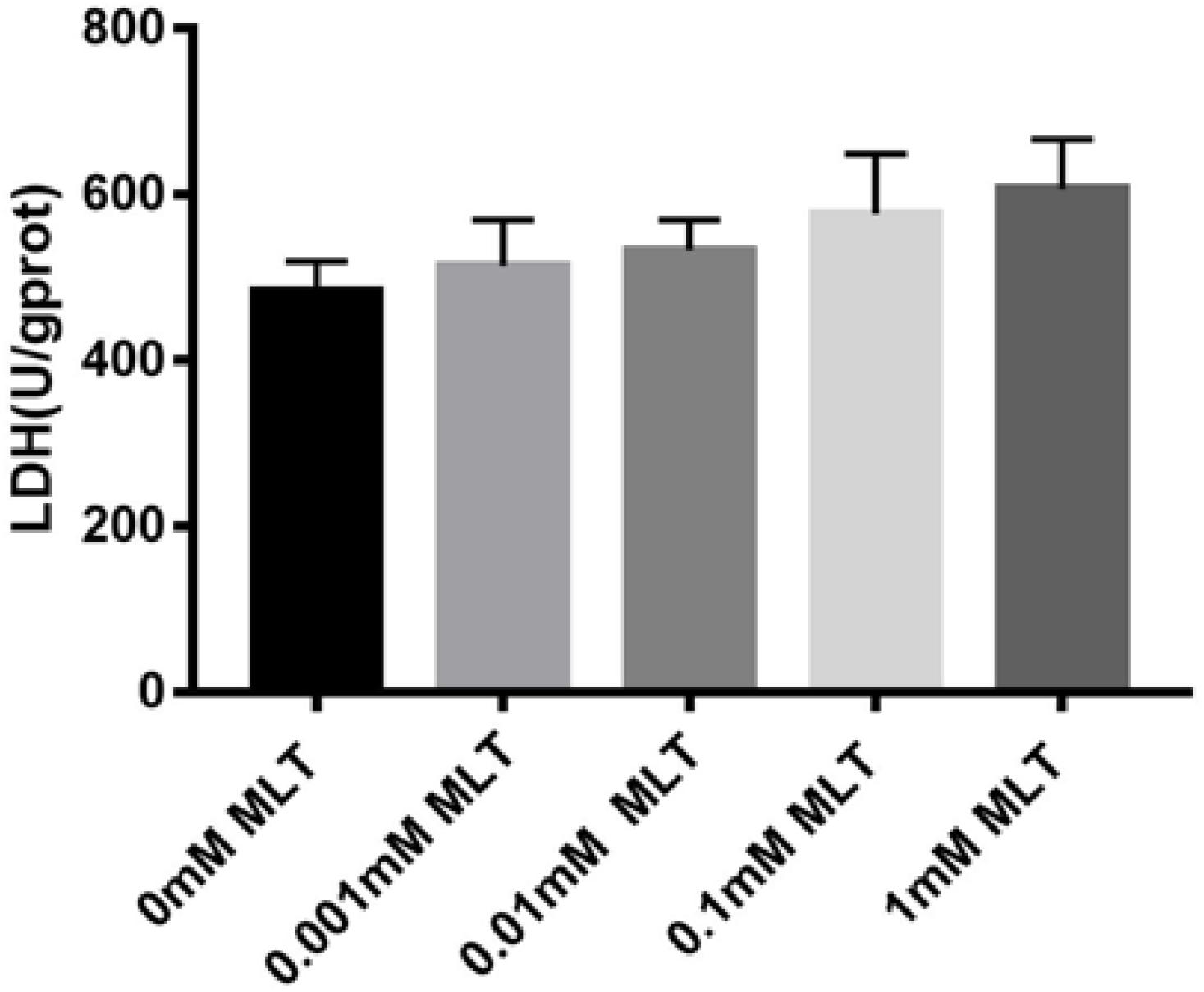
Figure 6. Lactate dehydrogenase (LDH) assay. MLT in the vitrification medium at the indicated concentrations is not cytotoxic. Data are shown as the mean ± SEM.
Discussion and Conclusion
This study is to evaluate the protective role of MLT at different concentrations in cryopreservation of OTs. Morphological changes and apoptotic rates in MLT-treated groups were compared after vitrification. We found that the 0.1-mM MLT-treated and 1-mM MLT-treated groups retained follicular morphological integrity most effectively after vitrification. Only the OTs vitrified with 0.1 mM MLT maintained a high percentage of morphological integrity comparable to fresh control. Our findings are consistent with previous reports showing that MLT-vitrificated solutions can protect the follicular morphological integrity in cryopreserved OTs and might help manage the negative effects of cryopreservation on female fertility preservation (Abir et al., 2017; Goodarzi et al., 2017).
This research found that the freeze-thaw process induced an increased apoptotic rate of follicular cells, which is in line with previous studies (Chaves et al., 2008; Won et al., 2013; Rocha et al., 2018). Studies have demonstrated the anti-apoptotic effects of some cryoprotectants, such as resveratrol and cryoprotective agent after vitrification of OTs (Won et al., 2013; Rocha et al., 2018). Notably, MLT can attenuate the stress-induced apoptotic follicular cell damage in the ovary (Barberino et al., 2017; Jang et al., 2017). Similarly, our results also showed that the numbers of intact follicles and apoptotic follicular cells were significantly reduced by MLT during the freeze-thaw processes. We also detected the level of apoptosis-related proteins in cryopreserved OTs and found that there was a decreased level of Bax (pro-apoptotic protein) and an increased level of Bcl-2 (anti-apoptotic protein), especially in the 0.1-mM MLT-treated group. Therefore, these findings above strongly support that MLT has an ideal anti-apoptotic effect.
Oxidative stress is one of the main factors that induce follicular cell apoptosis (Won et al., 2013; Barberino et al., 2017; Rocha et al., 2018; Zhang et al., 2019). MLT, as an antioxidant, has protective effects against oxidative stress and apoptosis (Reiter et al., 2016). It has also been reported that MLT, as a cryoprotectant, plays an important role in the cryopreservation of human semen (Karimfar et al., 2015; Najafi et al., 2018). However, whether MLT can protect cryopreserved OTs from oxidative damage still remains unknown.
Glutathione peroxidases catalyzes the reduction of hydrogen peroxide by GSH and thus can protect the integrity of cell membrane structure and function (Czuczejko et al., 2019). GSH is the major antioxidant sensitive to intracellular ROS (Yu et al., 2019). SOD and CAT are the first line of defense against oxidative stress by directly scavenging ROS in the defense system (Ozkan et al., 2018; Yu et al., 2019). MDA is the final product of lipid peroxidation and a biomarker indicating the level of oxidative stress. NO successively increased the oxidation stress. MLT can counteract the generation of NO by inhibiting the activity of inducible nitric oxide synthase (Mayo et al., 2005). Our results demonstrated that MLT reduced the ROS, NO, and MDA levels, meanwhile it promoted antioxidant enzyme activities (GSH, GSH-Px, T-SOD, CAT, and T-AOC), which are in line with recent studies (Deng et al., 2017; Yu et al., 2019). Therefore, MLT can not only scavenge free radicals but also promote the activity of antioxidant enzymes and prevent against oxidative stress in cryopreserved OTs.
The Nrf2 signaling pathway was further studied to investigate the protective mechanism of MLT. Nrf2 plays a significant role in regulating the expression of antioxidant genes (Lim et al., 2015; Akino et al., 2018; Khadrawy et al., 2019). In this study, MLT promoted Nrf2 mRNA expression in cryopreserved OTs, suggesting that the activation of the Nrf2 signaling pathway may be the main mechanism of MLT in protecting the cryopreserved OTs. The mechanism of MLT activating the Nrf2 signaling pathway remains to be further investigated. HO-1 is also reported to be involved in ovarian response to oxidative stress (Bergandi et al., 2014; Yan et al., 2018; Yu et al., 2019). In this study, HO-1 mRNA expression levels were found to be significantly higher in cryopreserved OTs. In addition, Nrf2 exerts the protective mechanism by regulating expressions of Nrf2 downstream target genes (including HO-1, CAT, SOD, and GSTM1) in cryopreserved OTs. Many studies found that MLT is a potent regulator of Nrf2 and HO-1 in oxidative damage (Kang et al., 2018; Niringiyumukiza et al., 2019). Therefore, it can be assumed that restraint stress can achieve oxidative stress by interfering with the Nrf2/HO-1 signaling pathway in cryopreserved OTs. However, these results may lack adequate demonstration on whether HO-1 is directly regulated by MLT or depends on Nrf2. This finding, while preliminary, suggests that the protective effects of MLT are at least partially attributable to normalization of Nrf2 and HO-1 expression to attenuate ROS produced by restraint stress.
In conclusion, MLT may have a significant protective effect on cryopreserved OTs by inhibiting oxidative stress and apoptosis, as well as activating the Nrf2 signaling pathway (Figure 7). These results provide a new method for cryopreservation of OTs in female fertility preservation.
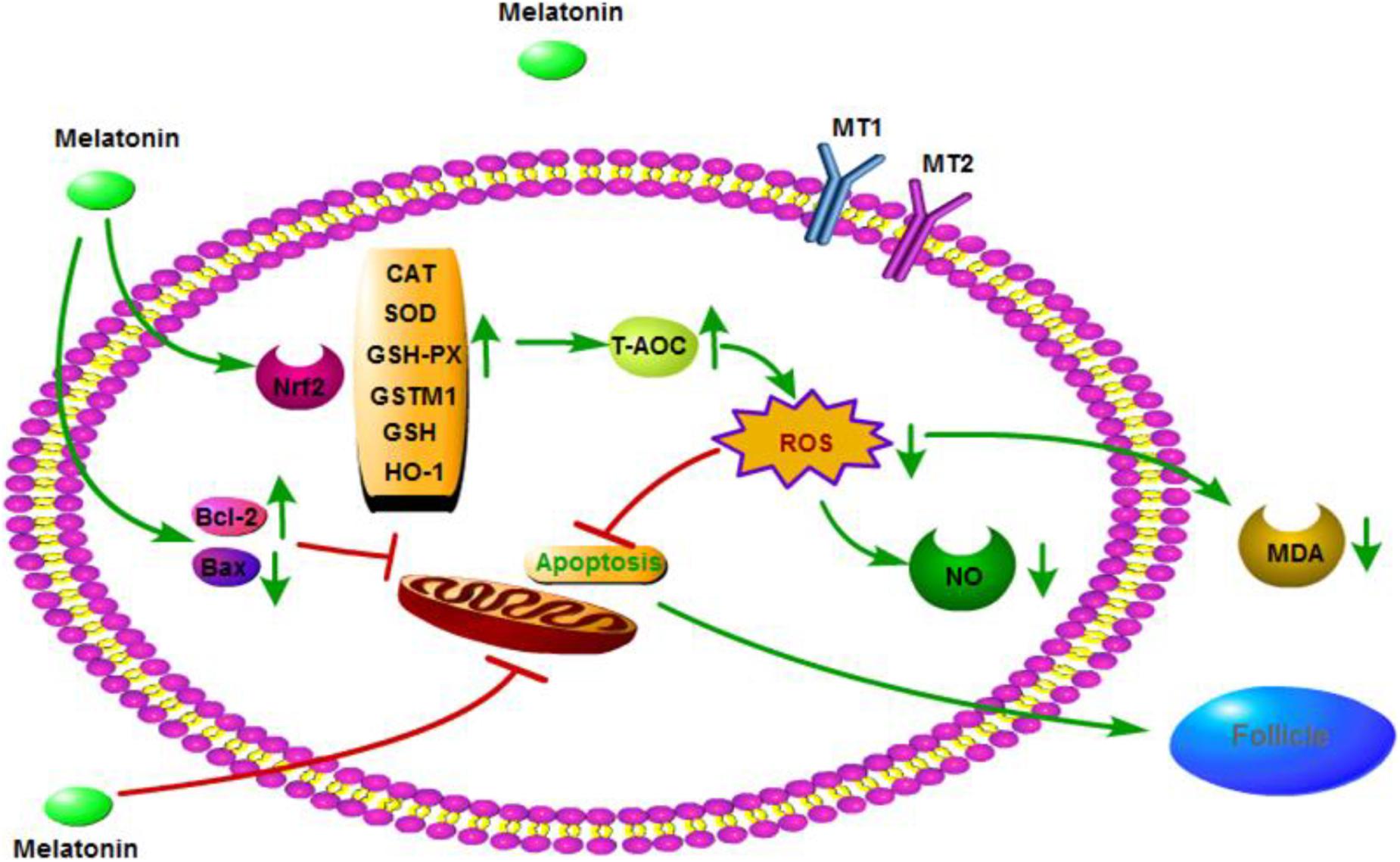
Figure 7. Mechanisms by which melatonin ameliorates oxidative stress via modulation of the Nrf2 pathway in cryopreserved OTs.
Data Availability Statement
All datasets presented in this study are included in the article/supplementary material.
Ethics Statement
The animal study was reviewed and approved by the Animal Ethics Committee of the Chengde Medical University (Chengde, China). Written informed consent was obtained from the owners for the participation of their animals in this study.
Author Contributions
TS, SD, and LC conceived and designed the experiments. TS, XL, SY, LS, and SZ performed the experiments. TS, XL, SY, and LT analyzed the data. LC and LT contributed to reagents, materials, and analysis tools. TS and LC wrote the manuscript. All authors contributed to the article and approved the submitted version.
Funding
This work was supported by grants from the NHC Key Laboratory of Family Planning and Healthy/Key Laboratory of Reproductive Medicine of Hebei Provincial (SZ-202006) and Peking University International Hospital Research Funds (No. YN2019QN13).
Conflict of Interest
The authors declare that the research was conducted in the absence of any commercial or financial relationships that could be construed as a potential conflict of interest.
Acknowledgments
The authors wish to thank SD for providing the kits and solutions. They also thank Dr. Xi Qin for help in data analysis and continuous support.
Abbreviations
CAT, catalase; GSH, glutathione; GSH-Px, glutathione peroxidases; GSTM1, glutathione S-transferase M1; HO-1, heme oxygenase-1; MDA, malondialdehyde; MLT, melatonin; NO, nitric oxide; Nrf2, nuclear factor erythroid 2-related factor 2; OTs, ovarian tissues; ROS, reactive oxygen species; SOD, superoxide dismutase.
References
Abir, R., Fisch, B., Fisher, N., Samara, N., Lerer-Serfaty, G., Magen, R., et al. (2017). Attempts to improve human ovarian transplantation outcomes of needle-immersed vitrification and slow-freezing by host and graft treatments. J. Assist. Reproduct. Genet. 34, 633–644. doi: 10.1007/s10815-017-0884-8
Agarwal, A., Aponte-Mellado, A., Premkumar, B. J., Shaman, A., and Gupta, S. (2012). The effects of oxidative stress on female reproduction: a review. Reproduct. Biol. Endocrinol. 10:49. doi: 10.1186/1477-7827-10-49
Akino, N., Wada-Hiraike, O., Terao, H., Honjoh, H., Isono, W., Fu, H., et al. (2018). Activation of Nrf2 might reduce oxidative stress in human granulosa cells. Mol. Cell Endocrinol. 470, 96–104. doi: 10.1016/j.mce.2017.10.002
Anderson, R. A., Wallace, W. H. B., and Telfer, E. E. (2017). Ovarian tissue cryopreservation for fertility preservation: clinical and research perspectives. Hum. Reproduct. Open 2017:hox001.
Barberino, R. S., Menezes, V. G., Ribeiro, A., Palheta, R. C. Jr., Jiang, X., Smitz, J. E. J., et al. (2017). Melatonin protects against cisplatin-induced ovarian damage in mice via the MT1 receptor and antioxidant activity. Biol. Reprod. 96, 1244–1255. doi: 10.1093/biolre/iox053
Bergandi, L., Basso, G., Evangelista, F., Canosa, S., Dalmasso, P., Aldieri, E., et al. (2014). Inducible nitric oxide synthase and heme oxygenase 1 are expressed in human cumulus cells and may be used as biomarkers of oocyte competence. Reproduct. Sci. (Thousand Oaks Calif.) 21, 1370–1377. doi: 10.1177/1933719114525268
Chaves, R. N., Martins, F. S., Saraiva, M. V., Celestino, J. J., Lopes, C. A., Correia, J. C., et al. (2008). Chilling ovarian fragments during transportation improves viability and growth of goat preantral follicles cultured in vitro. Reprod. Fertil Dev. 20, 640–647.
Czuczejko, J., Sielski, L, Woźniak, B., Woźniak, A., and Szewczyk-Golec, K. (2019). Melatonin supplementation improves oxidative and inflammatory state in the blood of professional athletes during the preparatory period for competitions. Free Radic. Res. 53, 198–209. doi: 10.1080/10715762.2018.1563688
Deng, S. L., Sun, T. C., Yu, K., Wang, Z. P., and Zhang, B. L. (2017). Melatonin reduces oxidative damage and upregulates heat shock protein 90 expression in cryopreserved human semen. Free Rad. Biol. Med. 113, 347–354. doi: 10.1016/j.freeradbiomed.2017.10.342
Donnez, J., and Dolmans, M. M. (2017). The ovary: from conception to death. Fertil. Steril. 108, 594–595. doi: 10.1016/j.fertnstert.2017.08.031
Gandolfi, F., Paffoni, A., Brambilla, E. P., Bonetti, S., Brevini, T., and Ragni, G. (2006). Efficiency of equilibrium cooling and vitrification procedures for the cryopreservation of ovarian tissue: comparative analysis between human and animal models. Fertil. Sterility 85(Suppl. 1), 1150–1156. doi: 10.1016/j.fertnstert.2005.08.062
Gao, J., Su, G., Liu, J., Zhang, J., Zhou, J., Liu, X., et al. (2020). Mechanisms of inhibition of excessive microglial activation by melatonin. J. Mol. Neurosci. 70, 1229–1236.
Gao, L., Zhao, Y. C., Liang, Y., Lin, X. H., Tan, Y. J., Wu, D. D., et al. (2016). The impaired myocardial ischemic tolerance in adult offspring of diabetic pregnancy is restored by maternal melatonin treatment. J. Pineal Res. 61, 340–352. doi: 10.1111/jpi.12351
Goodarzi, A., Shahneh, A. Z., Kohram, H., Sadeghi, M., and Davachi, N. D. (2017). Effect of melatonin supplementation in the long-term preservation of the sheep ovaries at different temperatures and subsequent in vitro embryo production. Theriogenology 106:265. doi: 10.1016/j.theriogenology.2017.10.009
Grynberg, M., Sonigo, C., and Santulli, P. (2018). Fertility preservation in women. N. Engl. J. Med. 378:400.
Gül, S., Gül, M., and Yigitcan, B. (2018). Melatonin preserves ovarian tissues of rats exposed to chronic TCDD: An electron microscopic approach to effects of TCDD on ovarian cells. Toxicol. Industr. Health 34, 228–236. doi: 10.1177/0748233717754174
Jang, H., Hong, K., and Choi, Y. (2017). Melatonin and fertoprotective adjuvants: prevention against premature ovarian failure during chemotherapy. Int. J. Mol. Sci. 18:1221. doi: 10.3390/ijms18061221
Jensen, A. K., Macklon, K. T., Fedder, J., Ernst, E., Humaidan, P., and Andersen, C. Y. (2017). 86 successful births and 9 ongoing pregnancies worldwide in women transplanted with frozen-thawed ovarian tissue: focus on birth and perinatal outcome in 40 of these children. J. Assist. Reprod. Genet. 34, 325–336. doi: 10.1007/s10815-016-0843-9
Kang, B., Wang, X., Xu, Q., Wu, Y., Si, X., and Jiang, D. (2018). Effect of 3-nitropropionic acid inducing oxidative stress and apoptosis of granulosa cells in geese. Biosci. Rep. 38:BSR20180274.
Karimfar, M. H., Niazvand, F., Haghani, K., Ghafourian, S., Shirazi, R., and Bakhtiyari, S. (2015). The protective effects of melatonin against cryopreservation-induced oxidative stress in human sperm. Int. J. Immunopathol. Pharmacol. 28, 69–76. doi: 10.1177/0394632015572080
Khadrawy, O., Gebremedhn, S., Salilew-Wondim, D., Taqi, M. O., Neuhoff, C., Tholen, E., et al. (2019). Endogenous and exogenous modulation of Nrf2 mediated oxidative stress response in bovine granulosa cells: potential implication for ovarian function. Int. J. Mol. Sci. 20:1635. doi: 10.3390/ijms20071635
Kleszczyński, K., Zillikens, D., and Fischer, T. W. (2016). Melatonin enhances mitochondrial ATP synthesis, reduces reactive oxygen species formation, and mediates translocation of the nuclear erythroid 2-related factor 2 resulting in activation of phase-2 antioxidant enzymes (γ-GCS, HO-1, NQO1) in ultraviolet radiation-treated normal human epidermal keratinocytes (NHEK). J. Pineal Res. 61, 187–197. doi: 10.1111/jpi.12338
Li, Z., Nickkholgh, A., Xue, Y., Bruns, H., Gross, M. L., Hoffmann, K., et al. (2009). Melatonin protects kidney grafts from ischemia/reperfusion injury through inhibition of NF-kB and apoptosis after experimental kidney transplantation. J. Pineal Res. 46, 365–372. doi: 10.1111/j.1600-079x.2009.00672.x
Lim, J., Ortiz, L., Nakamura, B. N., Hoang, Y. D., Banuelos, J., Flores, V. N., et al. (2015). Effects of deletion of the transcription factor Nrf2 and benzo [a]pyrene treatment on ovarian follicles and ovarian surface epithelial cells in mice. Reproduct. Toxicol. (Elmsford, N. Y.) 58, 24–32. doi: 10.1016/j.reprotox.2015.07.080
Mayo, J. C., Sainz, R. M., Tan, D. X., Hardeland, R., Leon, J., Rodriguez, C., et al. (2005). Anti-inflammatory actions of melatonin and its metabolites, N1-acetyl-N2-formyl-5-methoxykynuramine (AFMK) and N1-acetyl-5-methoxykynuramine (AMK), in macrophages. J. Neuroimmunol. 165, 139–149. doi: 10.1016/j.jneuroim.2005.05.002
Najafi, A., Adutwum, E., Yari, A., Salehi, E., Mikaeili, S., Dashtestani, F., et al. (2018). Melatonin affects membrane integrity, intracellular reactive oxygen species, caspase3 activity and AKT phosphorylation in frozen thawed human sperm. Cell Tissue Res. 372, 149–159. doi: 10.1007/s00441-017-2743-4
Niringiyumukiza, J. D., Cai, H., Chen, L., Li, Y., Wang, L., Zhang, M., et al. (2019). Protective properties of glycogen synthase kinase-3 inhibition against doxorubicin-induced oxidative damage to mouse ovarian reserve. Biomed. Pharmacother. Biomed. Pharmacother. 116:108963. doi: 10.1016/j.biopha.2019.108963
Olcese, J. M. (2020). Melatonin and female reproduction: an expanding universe. Front. Endocrinol. 11:85.
Ozkan, N., Ersoy, F., Ozsoy, Z., and Cakır, E. (2018). Melatonin exhibits supportive effects on oxidants and anastomotic healing during intestinal ischemia/reperfusion injury. Ulus Travma Acil Cerrahi Derg 24, 1–8.
Pang, K. C., Peri, A. J. S., Chung, H. E., Telfer, M., Elder, C. V., Grover, S., et al. (2020). Rates of fertility preservation use among transgender adolescents. JAMA Pediatr. 13:e200264.
Rajabi, Z., Khokhar, Z., and Yazdekhasti, H. (2018). The growth of preantral follicles and the impact of different supplementations and circumstances: a review study with focus on bovine and human preantral follicles. Cell. Reprogram. 20, 164–177. doi: 10.1089/cell.2017.0068
Reiter, R. J., Mayo, J. C., Tan, D. X., Sainz, R. M., Alatorre-Jimenez, M., and Qin, L. (2016). Melatonin as an antioxidant: under promises but over delivers. J. Pineal Res. 61, 253–278. doi: 10.1111/jpi.12360
Rocha, C. D., Soares, M. M., Deize, D. C. A., Júnior, J. M., Freitas Mohallem, R. F., Ribeiro Rodrigues, A. P., et al. (2018). Positive effect of resveratrol against preantral follicles degeneration after ovarian tissue vitrification. Theriogenology 114, 244–251. doi: 10.1016/j.theriogenology.2018.04.004
Tamura, H., Jozaki, M., Tanabe, M., Shirafuta, Y., Mihara, Y., Shinagawa, M., et al. (2020). Importance of melatonin in assisted reproductive technology and ovarian aging. Int. J. Mol. Sci. 21:1135. doi: 10.3390/ijms21031135
Tarín, J. J., Pérez-Albalá, S., and Cano, A. (2002). Oral antioxidants counteract the negative effects of female aging on oocyte quantity and quality in the mouse. Mol. Reprod. Dev. 61, 385–397. doi: 10.1002/mrd.10041
Van Den Heuvel-Eibrink, M. M., Van Der Kooi, A. L. F., and Wallace, W. H. B. (2018). Fertility preservation in women. N. Engl. J. Med. 378, 399–400.
Wang, H., Andoh, K., Hagiwara, H., Xiaowei, L., Kikuchi, N., Abe, Y., et al. (2001). Effect of adrenal and ovarian androgens on type 4 follicles unresponsive to FSH in immature mice. Endocrinology 142, 4930–4936. doi: 10.1210/endo.142.11.8482
Wang, Z., Ma, C., Meng, C. J., Zhu, G. Q., Sun, X. B., Huo, L., et al. (2012). Melatonin activates the Nrf2-ARE pathway when it protects against early brain injury in a subarachnoid hemorrhage model. J. Pineal Res. 53, 129–137. doi: 10.1111/j.1600-079x.2012.00978.x
Won, Y. H., Ryeol, L. J., Jaewang, L., Chul, J. B., Suk, S. C., and Hyun, K. S. (2013). Optimal vitrification protocol for mouse ovarian tissue cryopreservation: effect of cryoprotective agents and in vitro culture on vitrified–warmed ovarian tissue survival. Hum. Reprod. 29, 720–730. doi: 10.1093/humrep/det449
Yan, Z., Dai, Y., Fu, H., Zheng, Y., Bao, D., Yin, Y., et al. (2018). Curcumin exerts a protective effect against premature ovarian failure in mice. J. Mol. Endocrinol. 60, 261–271. doi: 10.1530/jme-17-0214
Yang, Z., Tang, Z., Cao, X., Xie, Q., Hu, C., Zhong, Z., et al. (2020). Controlling chronic low-grade inflammation to improve follicle development and survival. Am. J. Reprod. Immunol. 12:e13265.
Yu, K., Wang, R. X., Li, M. H., Sun, T. C., Zhou, Y. W., Li, Y. Y., et al. (2019). Melatonin reduces androgen production and upregulates heme oxygenase-1 expression in granulosa cells from PCOS patients with hypoestrogenia and hyperandrogenia. Oxidat. Med. Cell. Long. 2019:8218650.
Keywords: melatonin, ROS, Nrf2 signaling pathway, oxidative stress, cryopreserved ovarian tissue
Citation: Sun TC, Liu XC, Yang SH, Song LL, Zhou SJ, Deng SL, Tian L and Cheng LY (2020) Melatonin Inhibits Oxidative Stress and Apoptosis in Cryopreserved Ovarian Tissues via Nrf2/HO-1 Signaling Pathway. Front. Mol. Biosci. 7:163. doi: 10.3389/fmolb.2020.00163
Received: 27 April 2020; Accepted: 25 June 2020;
Published: 29 July 2020.
Edited by:
Bechan Sharma, University of Allahabad, IndiaReviewed by:
Ahmed Esmat Abdel Moneim, Helwan University, EgyptSeyithan Taysi, Gaziantep University, Turkey
Copyright © 2020 Sun, Liu, Yang, Song, Zhou, Deng, Tian and Cheng. This is an open-access article distributed under the terms of the Creative Commons Attribution License (CC BY). The use, distribution or reproduction in other forums is permitted, provided the original author(s) and the copyright owner(s) are credited and that the original publication in this journal is cited, in accordance with accepted academic practice. No use, distribution or reproduction is permitted which does not comply with these terms.
*Correspondence: Tie Cheng Sun, zufengbenpao@163.com; yxyysh@126.com; Shou Long Deng, popo84350746@163.com; Li Tian, tianli916916@126.com; Lu Yang Cheng, chengll526@126.com
†These authors have contributed equally to this work