- CNRS, UMR 8261, Université de Paris, Institut de Biologie Physico-Chimique, Paris, France
In oxygen (O2) limiting environments, numerous aerobic bacteria have the ability to shift from aerobic to anaerobic respiration to release energy. This process requires alternative electron acceptor to replace O2 such as nitrate (NO3–), which has the next best reduction potential after O2. Depending on the organism, nitrate respiration involves different enzymes to convert NO3– to ammonium (NH4+) or dinitrogen (N2). The expression of these enzymes is tightly controlled by transcription factors (TFs). More recently, bacterial small regulatory RNAs (sRNAs), which are important regulators of the rapid adaptation of microorganisms to extremely diverse environments, have also been shown to control the expression of genes encoding enzymes or TFs related to nitrate respiration. In turn, these TFs control the synthesis of multiple sRNAs. These results suggest that sRNAs play a central role in the control of these metabolic pathways. Here we review the complex interplay between the transcriptional and the post-transcriptional regulators to efficiently control the respiration on nitrate.
Introduction
Nitrate is an important nutrient for microorganisms and can be used in both assimilatory and dissimilatory pathways. Assimilatory pathways allow the incorporation of nitrogen into the organism’s biomass (DNA, proteins.). In contrast, dissimilatory nitrate reduction to ammonium (DNRA) is more a catabolic pathway leading to nitrogen excretion. In some cases, the dissimilatory process can release energy and is therefore termed “nitrate respiration.” Several metabolic pathways, such as DNRA, denitrification, and in, some bacteria, anaerobic ammonium oxidation (anammox) (reviewed in Kartal et al., 2012), use the reduction of nitrate or nitrite as the first step in respiration. The final products are NH4+ for DNRA, and N2 for the denitrification and anaerobic anammox pathways (Figure 1); however, numerous bacteria are only partial denitrifiers since the last enzyme required to convert N2O to N2 is absent (Philippot et al., 2011). These diverse respiratory nitrate reduction pathways are not necessarily present simultaneously in a given organism, but can compete for nitrate utilization. Denitrification is probably the best studied nitrate pathway in bacteria and it has been shown that incomplete denitrification can release N2O in the atmosphere, a greenhouse gas involved in global warming. Nitrate reduction also plays a key role in bacterial pathogenesis (Vázquez-Torres and Bäumler, 2016) as well as in the gut colonization by enterobacteria.
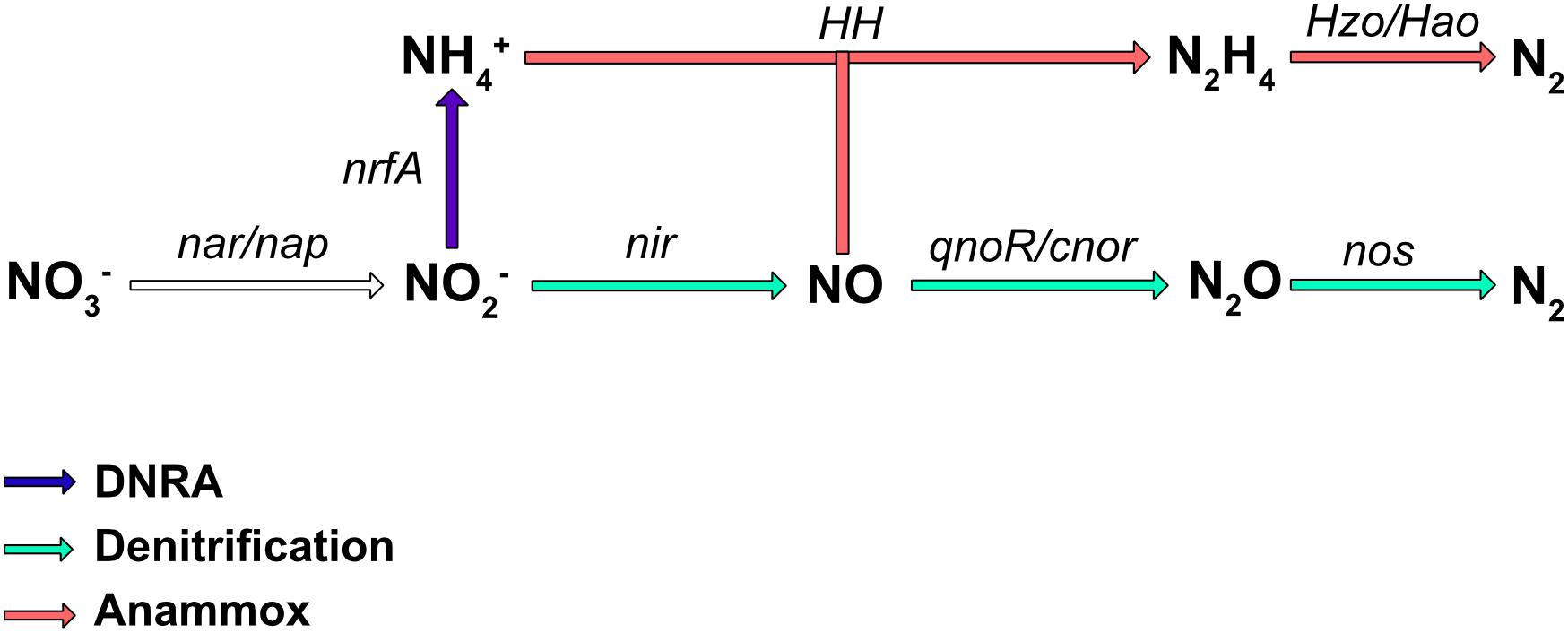
Figure 1. Biological pathways of nitrate respiration in bacteria. The major process involved in nitrate transformation to ammonium (NH4+) is the DNRA pathway (in blue), while those involved in nitrate transformation to N2 are denitrification (green), and ammonium oxidation (anammox; red). The main enzymes involved are Nar, a membrane-bound dissimilatory nitrate reductase; Nap, a periplasmic dissimilatory nitrate reductase; NirBD, a nitrite reductase; NrfA and NirK/NirS, nitrite reductases; cNor and qNor, nitric oxide reductases; NosZ, a nitrous oxide reductase; HH, hydrazine hydrolase; HAO, hydrazine oxidoreductase.
Numerous enzymes are involved in these different metabolic pathways, and the coordination of their expression is absolutely required to limit the energetic cost and avoid the accumulation of toxic compounds such as nitric oxide (NO), a free radical which has the propensity to react with other molecules (see below). To correctly regulate the expression of these genes, bacteria use several transcription factors (TFs). One of the most prominent families of transcriptional regulators found to control nitrate respiration is that of the FNR-like proteins.
As it has been shown for other important metabolic pathways, transcriptional regulation is often combined with post-transcriptional control, where non-coding small RNAs (sRNAs) play a key role. Several studies have highlighted the role of sRNAs in nitrogen metabolism and this aspect was reviewed recently (Prasse and Schmitz, 2018; Muro-Pastor and Hess, 2020). However, recent developments also pointed to an important role for sRNAs in nitrate respiration pathways, which will be addressed in this review.
We will briefly present the diversity of enzymes involved in these metabolic pathways and how the expression of these enzymes is regulated by TFs and sRNAs.
The Diversity of Enzymes Involved in Nitrate Respiration
Nitrate Reductase (Nar, Nap)
For all nitrate respiration pathways mentioned above, the first step consists of the conversion of nitrate to nitrite (NO2–) by a nitrate reductase. These enzymes use molybdenum as a co-factor. Two types of nitrate reductase have been shown to be involved in nitrate respiration: Nar localized at the membrane and Nap found in the periplasm (Figure 2). The Nar nitrate reductases are trimeric (NarG/H/I) and encoded by the NarGHJI operon. This operon is widely conserved in bacteria and is found in nitrate-ammonifying (DNRA) bacteria from Escherichia coli to Bacillus subtilis. NarI is a transmembrane protein that receives electrons from the quinone pool and anchors NarG and NarH at the membrane. The electrons are transferred from NarI, a cytochrome b quinol oxidase, to NarG via NarH. NarH and NarG contain Fe-S clusters that receive an electron and transfer it to the active site of NarG (Figure 2A). The Nar reductase couples nitrate respiration to proton translocation across the membrane, driving ATP generation. NarJ, encoded by the last gene of the operon, is a chaperone protein that plays a role in the maturation and membrane insertion of Nar. Interestingly, E. coli and Salmonella typhimurium possess a duplication of this operon (narZYWV), which seems to play a greater role in stressed cells rather than anaerobic respiration (Blasco et al., 1990; Spector et al., 1999). Three nitrate reductases (Nar1, Nar2, and Nar3) have been identified and characterized in Streptomyces coelicolor. Nar1 is induced in spores and competes with aerobic respiration for electrons from menaquinol (Falke et al., 2019). In contrast, Nar2 and Nar3 are induced by anoxic condition in the mycelium (Urem et al., 2016; Fischer et al., 2019).
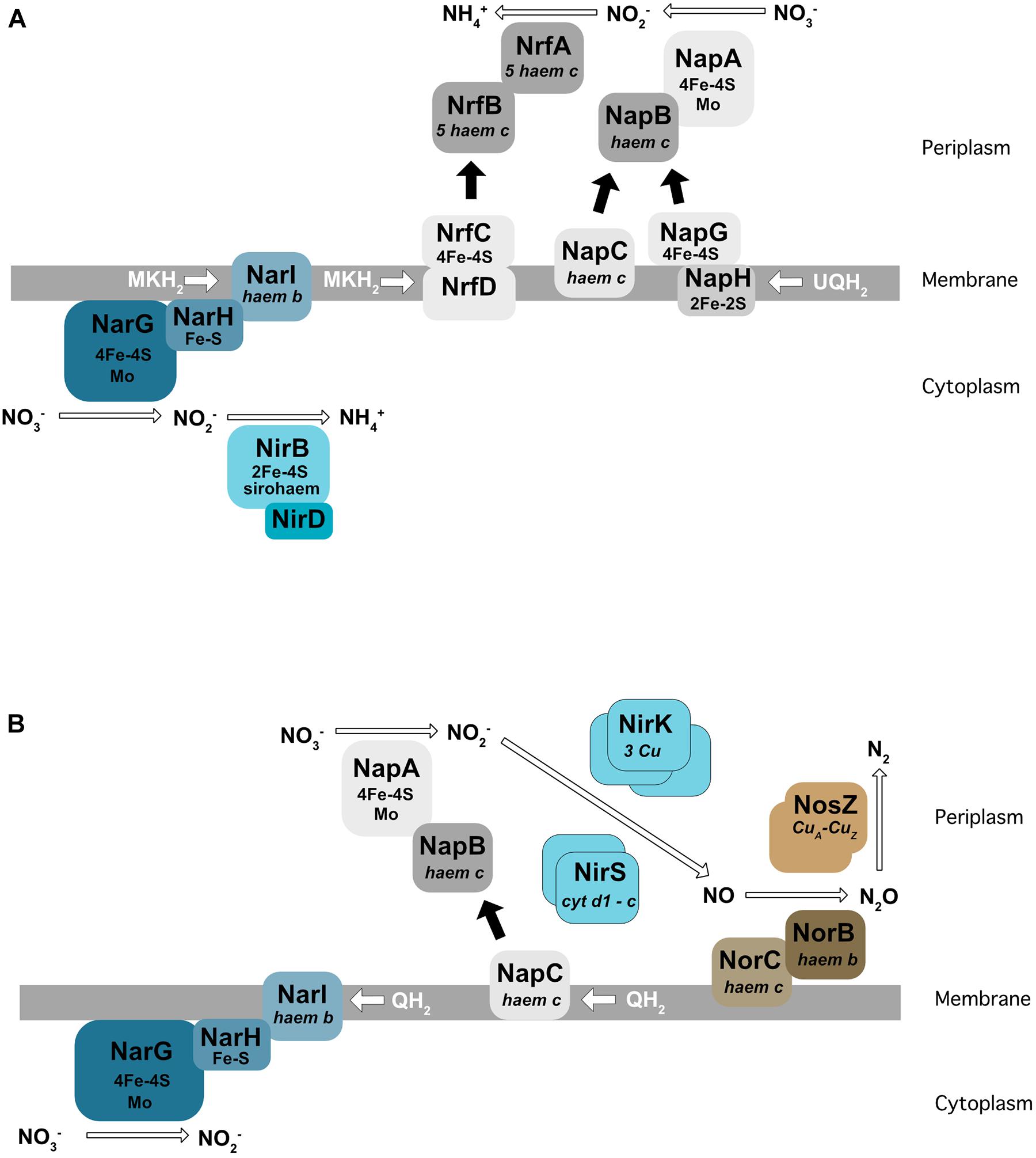
Figure 2. Enzymes involved in dissimilatory nitrate reduction to ammonium (DNRA) in E. coli (A) and in denitrification in P. denitrificans (B). Nar, membrane-bound dissimilatory nitrate reductase; Nap, periplasmic dissimilatory nitrate reductase; NirBD, a sirohaem containing nitrite reductase; NrfA, a cytochrome c nitrite reductase; NirK/NirS, cNor, and qNor, Cu-containing/cd1-type nitric oxide reductases; NosZ, a nitrous oxide reductase; QH2, MKH2, UQH2 : quinone, menaquinone, ubiquinone.
The Nap nitrate reductases are found in the periplasm of many different Gram-negative bacteria (reviewed in Richardson et al., 2007). The NapA subunit, bearing the catalytic active site, is transported across the membrane by the Tat apparatus and requires the NapD chaperone encoded in the same operon (Grahl et al., 2012). NapA receives electrons from two cytochrome c proteins, the NapC membrane protein and the NapB subunit (review by Richardson et al., 2001) (Figure 2B). In E. coli, the nap operon also encodes for a quinol-oxidizing system (napGH) composed of two Fe-S proteins: (1) NapH, a membrane-bound quinol dehydrogenase and (2) NapG, a periplasmic electron transfer adapter protein which can, similarly to NapC, deliver electrons to NapB. However, the exact function of this complex in E. coli is still unclear. The presence of both NapC and NapGH proteins in bacterial genomes is variable. Some bacteria such as Pseudomonas aeruginosa only have the napC gene and others, e.g., ε-proteobacteria such as Campylobacter jejuni or Sulfurimonas denitrificans, only have NapGH (Sievert et al., 2008; Kern and Simon, 2009; reviewed in Sparacino-Watkins et al., 2014). The nap operon also encodes NapF in E. coli, which is proposed to post-translationally modify NapA prior to its export into the periplasm (Nilavongse et al., 2006). Interestingly, this protein is absent in its close relative Salmonella. In contrast to the Nar, Nap enzymes are unable themselves to generate a proton motive force. However, Nap can play a role in nitrate respiration when associated with a proton translocating enzyme such as the NADH dehydrogenase NuoA-N (Moreno-Vivián et al., 1999). In this way, NapA has been shown to support anaerobic respiration in E. coli (Stewart et al., 2002), and in several bacteria like Bradyrhizobium japonicum (Bedmar et al., 2005), Rhodobacter sphaeroides (Liu et al., 1999), Pseudomonas sp. strain G-179 (Bedzyk et al., 1999), where the Nap enzyme has been shown to be used for the first step of denitrification rather than Nar.
Nitrite Reductase (Nir, Nrf)
The nitrite reductases ensure the reduction of nitrite to NO in denitrifying bacteria (denitrification) and into ammonium in DNRA bacteria, also known as ammonifiers (see below).
In denitrifying bacteria, two types of unrelated nitrite reductases (Nir) have been identified so far (reviewed in Moura and Moura, 2001). One, namely NirK, is an homotrimeric copper-containing enzyme which has been found in both Gram-negative and -positive bacteria (Suharti and de Vries, 2005). The second, NirS, is a homodimeric cytochrome cd1 nitrite reductase that has been characterized in Gram-negatives (Figure 2B). Electrons are delivered by the cytochrome bc1 complex. The nirS gene cluster encodes numerous additional proteins (for example the nirXISECFDLGHJN operon in Paracoccus denitrificans) necessary for the correct assembly of the active site haem d1 cofactor of NirS (reviewed in Bali et al., 2014). In contrast to nirS, the nirK gene is generally isolated, but in some cases is in an operon with the nirV gene, whose product may be required for the correct insertion of the copper center. The presence of NirK and NirS has been for a long time proposed to be mutually exclusive. However, recent genomic comparisons have showed that both enzymes can be present in the same organism (Graf et al., 2014), probably with different roles and regulation, notably in Pseudomonas stutzeri (Wittorf et al., 2018).
In the nitrate respiratory ammonifiers, such as E. coli and Salmonella enterica, NirK and NirS as well as the nitric oxide reductases (qNor and cNOR, see below) are absent, and the reduction of nitrite is performed by two classes of enzymes:
(1) A cytoplasmic sirohaem-containing nitrite reductase (NirBD), encoded by nirB and nirD (Figure 2A), with the nirC gene in the same operon probably encoding a nitrite transporter. The nirB and nirD genes are also present in the genome of B. subtilis (nasDEF). The nasDE genes encode the nitrite reductase subunits and nasF is required for nitrite reductase sirohaem cofactor formation. Interestingly, this enzyme is used for both anaerobic respiration and nitrogen assimilation in B. subtilis (Ogawa et al., 1995; Nakano et al., 1998).
(2) The periplasmic Nrf enzyme, consisting of a pentahaem cytochrome c nitrite reductase that reduces nitrite by using formate as an electron donor (Einsle et al., 2002; Figure 2A). NrfA was first identified in E. coli, but is also present in the periplasm of numerous γ-, δ-, and ε-proteobacteria. Similar to the nitrate reductase described previously, electrons are transferred from the membrane quinol dehydrogenase (NrfH or NrfCD) to the cytochrome c reductase NrfA via the small electron transfer protein NrfB (reviewed by Simon, 2002). In E. coli, the nrf operon also encodes three proteins (NrfEFG) dedicated to the attachment of the active site haem group.
Interestingly, NO is also produced by E. coli and S. enterica, despite the fact that they do not possess NirK or NirS enzymes, and is proposed to be a side-product of nitrate respiration. It has been shown that nitrite reduction to NO can be done by the NarG enzyme in Salmonella and represents the major route of NO production in this organism (Gilberthorpe and Poole, 2008).
Nitric Oxide Reductase (qNOR, cNOR)
The nitric oxide reductases reduce NO into nitrous oxide (N2O). They are part of the heme-copper oxidase family but are not able to translocate protons, in contrast to the other enzymes of this family (Bell et al., 1992; Hino et al., 2010; reviewed in Garcia-Horsman et al., 1994). Two types of NOR have been identified in Gram-negative bacteria: cNOR and qNOR, depending on the electron donor (heme c in cNOR; menaquinol in qNOR). cNOR is composed of two subunits: NorB with the catalytic site receiving electrons from a periplasmic electron donor via the NorC membrane bound cytochrome c (Figure 2B). cNORs are only found in denitrifying bacteria. Interestingly, a transient interaction between the cNORs and NirS (the cd1 nitrite reductase) has been proposed in P. denitrificans (Albertsson et al., 2019), and a similar interaction was observed in P. aeruginosa. This interaction is thought to be important for the channeling of the toxic intermediate NO. The NorCB structural subunits are encoded in an operon with other genes (norEFCBQD). The norQ and norD gene are always linked to norCB, but the norE and norF genes can be encoded separately or even be absent (Zumft, 2006). The function of these accessory proteins is not well defined. A structural study showed that even if cNOR and qNOR enzymes are not able to pump protons, the catalytic protons can be transferred from bulk water to the buried active site through a specific pathway consisting of a water channel and/or a hydrogen-bond network (Shiro, 2012).
In contrast to cNOR, the qNOR enzyme is a unique single subunit protein (NorZ) that receives electrons from quinones. The qNOR enzyme has some sequence similarities with both the NorB and the NorC proteins (Shiro, 2012). The qNOR enzymes have a wider taxonomic distribution and are found in some Archaea and non-denitrifying pathogenic bacteria, where they play a role in NO detoxification (Hendriks et al., 2000; Sousa et al., 2012).
In the Gram-positive Bacillus azotoformans, a third type of NOR was identified that binds copper (CuANOR). This enzyme receives electrons from membrane-bound cytochrome C551 (Al-Attar and De Vries, 2015) and the large subunit is similar to NorB. The small subunit has no heme c, but a CuA binding site.
Other non-respiratory enzymes have been identified that can detoxify NO. For example the flavohaemoglobin Hmp which is widespread in bacteria converts NO to NO3– (Vinogradov et al., 2013), and the flavorubredoxin NorV, with its NADH dependent oxidoreductase NorW (Gomes et al., 2002), reduces NO to NO–. Hmp and NorV are both present in E. coli but their mutation does not impair the reduction of NO, meaning that other NO reduction pathways still need to be characterized (Vine and Cole, 2011). Since these enzymes are not considered to play a role in respiration, we will not discuss them further in this review.
Nitrous Oxide Reductase (Nos)
Nitrous oxide reductase (Nos) is the last enzyme to complete denitrification by reducing N2O into dinitrogen (N2). In most denitrifying bacteria, this is a homodimeric copper-enzyme (NosZ) localized in the periplasm (Figure 2B). However, this enzyme is membrane bound in the Gram-positive bacteria B. azotoformans and Thiobacillus denitrificans (Hole et al., 1996; Suharti and de Vries, 2005). It was shown in several bacteria, such as P. denitrificans, that electron transfer to Nos is from cytochrome c. NosZ is in general found in an operon with at least 5 other genes (nosRZDFYL), encoding proteins required for its expression, maturation and maintenance (Zumft, 2006). This operon sometimes encodes supplementary genes, such as nosX or nosC. In α-, β-, and γ-proteobacteria, the NosR and NosX proteins can bind flavin mononucleotide (FMN) that might be an alternative transport pathway from the quinone pool to NosZ. The NosDFY and L proteins are involved in the maturation of NosZ, and the function of NosC is unknown.
Numerous genomes of ammonifiers also encode an atypical nitrous oxide reductase (cNosZ) (Sanford et al., 2012; Jones et al., 2013). cNosZ is a variant of the canonical NosZ enzyme, but fused to a cytochrome c domain which transports electrons to the active site (Simon et al., 2004). Moreover, cNosZ is exported to the periplasm by the Sec secretion pathway and not by the Tat (Twin Arginine translocation) pathway like the classical NosZ enzyme. The genome of Wolinella succinogenes encodes this atypical enzyme in a large operon, nosZBDGC1C2HFYL. The NosG and H are menaquinol dehydrogenases, homologous to the NapGH proteins. The 2 cytochrome c proteins (NosC1 and C2) form a putative electron transport pathway from menaquinol to cNosZ. The nosDFY genes encode a membrane-bound ABC transporter that might be important for the maturation of atypical Nos systems. Finally, NosL could be a chaperone involved in copper metallocenter assembly (Zumft, 2006).
For a long time, the denitrification and the nitrate ammonification pathways were considered to be mutually exclusive. However, recent genome sequencing data have revealed that some bacteria have all the genes required for both pathways. Shewanella loihica, for example, encodes the NrfA enzyme for the DNRA pathway, and NirK, NorB and NosZ enzymes for the denitrification (Sanford et al., 2012). Both pathways are functional in this bacterium (Yoon et al., 2015) and depend on the C/N ratio. Indeed, at low C/N ratio (i.e., electron donor-limiting growth condition) the denitrification pathway is preferred to DNRA. In contrast, at high C/N ratio (i.e., electron-acceptor limiting growth condition), ammonification is dominant (Yoon et al., 2015). Temperature and pH also influence the nitrate pathways, showing that nitrate respiration is widely connected to environmental conditions.
Some bacteria are also able to release energy by forming N2 gas from ammonium and nitrite under anoxic conditions (Figure 1). This energy pathway (anammox) seems to be limited to the Planctomycetales order. These bacteria have been found in almost all aquatic habitats where oxygen is limiting. This specific pathway requires two type of enzymes, hydrazine hydrolase (HH), which produces hydrazine from ammonium and NO, and hydrazine oxidoreductase (HZO/HAO), which allows the oxidation of hydrazine to N2 (Figure 1). Electrons are thought to be transferred to the quinone pool to ultimately generate a proton motive force.
The numerous enzymes involved in nitrate utilization pathways highlight the need for bacteria to efficiently control their expression at the transcriptional and post-transcriptional levels in response to their specific environments. This control is discussed below, with a focus on the few bacterial species where this has been studied in most detail: E. coli, S. enterica, and B. subtilis for the DNRA pathway, and P. denitrificans and P. aeruginosa for denitrification.
Transcriptional Regulators Controlling the Synthesis of Enzymes Involved in Nitrate Respiration
In bacteria that have the ability to respire on nitrate, this mode of respiration mainly depends on three parameters:
(1) Oxygen limitation
(2) The presence of nitrate (NO3–) or derived compounds such as nitrite (NO2–) or nitric oxide (NO) in the medium
(3) An appropriate donor of electrons (i.e., organic carbon compounds).
Several transcriptional regulators play a key role in integrating these signals to modulate the expression of genes related to nitrate respiration. Numerous studies have highlighted the importance of both the FNR-like global TFs controlling several metabolic pathways in order to prepare the cell to anaerobic conditions and the more specific two-component systems (TCSs) that frequently control one metabolic pathway. Most of these regulators can sense different signals like O2, NO3–, NO2– or NO (see below). FNR uses an O2-labile [4Fe-4S]2+ cluster to sense oxygen. In the absence of O2, the cluster is stable and forms a homodimer able to bind its DNA target sequences. In presence of O2, the [4Fe-4S]2+ cluster is unstable and the protein cannot dimerize, rendering it inefficient at binding its targets. It has also been demonstrated that the [4Fe-4S]2+ cluster is sensitive to NO in vitro, which also reduces the DNA-binding ability of FNR (Crack et al., 2013). Depending on the position of the FNR binding site in the promoter region, FNR activates or represses gene expression. In E. coli and B. subtilis, the FNR regulon represents more than 100 genes and its main role is to prepare the cell for anaerobic respiration (Constantinidou et al., 2006; Reents et al., 2006).
Two-component systems are typically composed of a membrane-bound histidine kinase that senses a specific stimulus and a response regulator that mediates the cellular response by modifying target gene expression. Numerous TCSs, such as NarX-NarL, are involved in nitrate respiration and will be described below. In general, they are more dedicated to nitrate respiration than FNR and their regulon includes less genes than the FNR-like regulon.
This strategy to use both global and more specific transcriptional regulators is similar in numerous bacteria, using either the DNRA or the denitrification pathway to respire on nitrate. This can provide significant advantages that include the integration of several environmental cues, e.g., O2 availability via FNR or FNR-like global regulators and the presence of alternative electron acceptors such as nitrate, via the NarX-NarL and NarQ-NarP TCSs. Some variations on this theme exist as well. For example, in B. subtilis and Streptomyces (see below), no transcriptional regulator that directly senses nitrate has been described, i.e., the regulation of the genes involved in the respiration on nitrate is not directly influenced by the presence of nitrate in the environment in this organism.
The different transcriptional regulators, the signals to which they respond, and their targets linked to nitrate respiration are described in the following sections.
Transcriptional Regulation of the DNRA Pathway
A complete review on the conservation of the transcriptional regulators involved in DNRA has been published previously (Rodionov et al., 2005). Briefly, the global transcriptional regulator FNR sensing O2, and the two nitrate-sensing NarQ-NarP and NarX-NarL TCSs have been shown to play a central role in the regulation of anaerobic respiration in E. coli and numerous γ-proteobacteria. In the presence of nitrate, NarX and NarQ phosphorylate NarL and NarP, respectively. Importantly, cross-regulation exists between these two TCSs; NarQ additionally efficiently controls the phosphorylation status of NarL, while NarX can influence NarP much more poorly (Noriega et al., 2010). Another difference between these two sensors is that NarQ is sensitive to signals other than nitrate, especially nitrite, but not NarX (Lee et al., 1999). All these transcriptional regulators (FNR, NarXL, and NarQP) are involved in controlling the synthesis of enzymes of the nitrate reduction pathway (Figure 3). More precisely, they all activate the expression of the nirBDC operon encoding nitrite reductase. The narGHJI operon is activated by NarL and FNR. E. coli and S. typhimurium genomes also encode a second cytoplasmic nitrate reductase (narZYWV), whose role is still unclear. However, in contrast to the narGHJI operon, these genes are repressed by FNR in S. typhimurium (Spector et al., 1999). The nap and the nrf genes encoding for the periplasmic nitrate- and nitrite reductase, respectively, are controlled by the NarP response regulator and by FNR (Figure 3; Jayaraman et al., 1987; Lamberg and Kiley, 2000; Browning et al., 2004). Several other targets of NarX-NarL and NarQ-NarP encode proteins involved in pathways related to nitrate respiration, e.g., NO detoxification (Filenko et al., 2005) or molybdenum attachment to enzymes such as nitrate reductase (Hasona et al., 2001). Another important transcriptional regulator involved in nitrate respiration in E. coli is NsrR. This transcriptional repressor has an NO-sensitive [Fe-S] cluster and is not only present in β- and γ-proteobacteria, but also in Streptomyces and Bacillus species (Rodionov et al., 2005; reviewed in Tucker et al., 2010). Nitrosylation of this factor inhibits its DNA-binding activity, leading to the derepression of the genes controlled by NsrR. In E. coli, NsrR has been shown to regulate 9 operons, including the nitrate- and nitrite reductase operons (nap and nrf) (Filenko et al., 2007; Figure 3). In contrast, the nrf operon is not sensitive to the mutation of nsrR in S. typhimurium (Browning et al., 2010). NsrR was also shown to regulate expression of the Nir and Nor enzymes in denitrifying bacteria such as Neisseria meningitidis (Heurlier et al., 2008). At last, it should be highlighted that enzymes of the nitrate respiration pathways are most often metalloenzymes that require redox-active cofactors, such as molybdenum, iron or copper (reviewed in Garcia-Horsman et al., 1994, Moreno-Vivián et al., 1999, Sullivan et al., 2013). Interestingly, two regulators of the E. coli nap operon are themselves dependent on such cofactors: ModE, whose activity is induced under anaerobic conditions through molybdenum binding, activates nap expression (McNicholas and Gunsalus, 2002) while IscR, containing a 2Fe-2S cluster, represses it under aerobic conditions (Giel et al., 2006). ModE is able to override the control of napF by nitrate when molybdenum is limiting (McNicholas and Gunsalus, 2002).
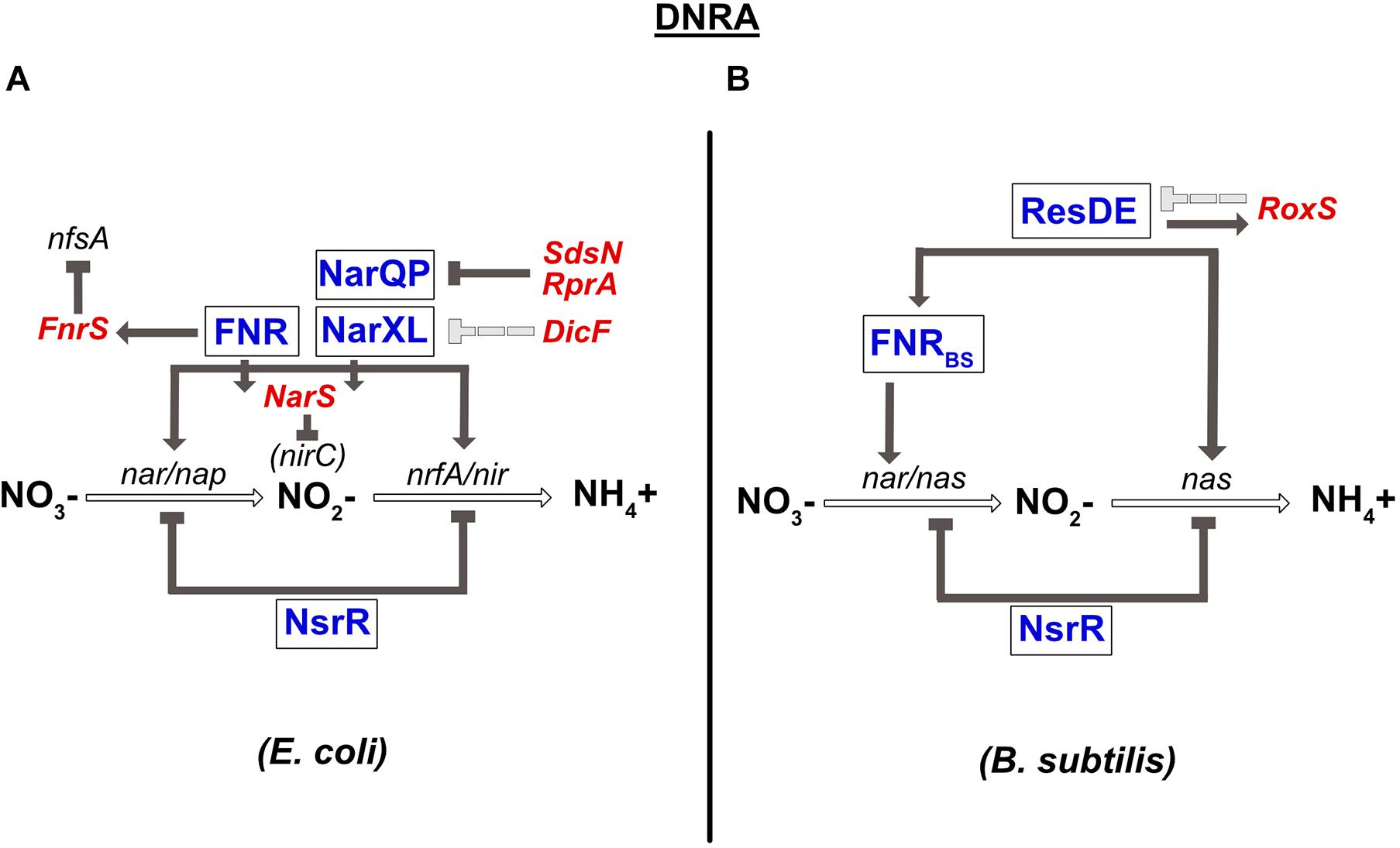
Figure 3. Regulation of dissimilatory nitrate reduction to ammonium (DNRA) in E. coli (A) and B. subtilis (B). (A) Nitrate reductase (nar/nap) and nitrite reductase (nir/nrfA) genes are co-regulated by FNR and by the two-component system NarQP for the nap, nir, and nrfA operons and NarXL for the nar and nir genes. The nar and nir operons are also repressed by the NsrR TF. narP expression is inhibited by two sRNAs, SdsN, and RprA. Expression of narXL is repressed, probably indirectly, by the DicF sRNA. In contrast, the FNR TF is responsible for fnrS and narS expression. FnrS regulates numerous genes that are dispensable during anaerobic respiration, such as nfsA, encoding an NADPH-dependent nitroreductase. The NarS sRNA is encoded at the 3′ end of the narK mRNA (encoding a transporter of nitrate and nitrite) and represses the expression of nirC, coding for a nitrite transporter. (B) Nitrate reductase gene (nar) is regulated by FNR. FNR is itself under the control of the ResDE TCS and also controls the expression of nitrite reductase (nas). NsrR inhibits the expression of the nas operon coding for nitrate and nitrite reductase. ResDE is a transcriptional activator of expression of the RoxS sRNA, which in turn negatively impacts its expression. RoxS regulates numerous genes encoding enzymes of the TCA cycle.
In Mycobacteria, NarL is also responsible for the activation of the narGHJI operon. Although this protein has 74% identity to E. coli NarL, the sensor kinase NarS is not related to NarX. Another TCS, namely DevR/DevS, was identified in Mycobacteria and responds to anaerobic conditions. DevR, the response regulator, also controls the expression of the nar genes and the nitrate transporter NarK2. Interestingly and in contrast to what is known in α- and γ-proteobacteria, the two response regulators DevR and NarL were proposed to form a heterodimer that can efficiently bind the narK2 promoter in Mycobacterium tuberculosis (Malhotra et al., 2015). Indeed, in vitro binding assays showed that DevR binding on narK2 promoter is stimulated in presence of NarL. More recently, it was shown that the PrrAB TCS, also found in α-proteobacteria (see below), induced the transcription of the DevR response regulator in Mycobacterium smegmatis and is important for ATP generation under aerobic and hypoxic conditions (Maarsingh et al., 2019).
The NarX-NarL TCS is functionally replaced by the NreABC system in Staphylococci. NreABC has the ability to sense both O2 and nitrate, with a higher impact of oxygen compared to nitrate. NreA has a nitrate-binding GAF domain that senses nitrate and NreB, considered as a functional equivalent to FNR, has a [4Fe-4S]2+ cluster that is sensitive to O2 (Müllner et al., 2008). NreB is the sensor histidine kinase and NreC the response regulator of the NreBC TCS. NreB is auto-phosphorylated in anoxic conditions, leading to the phosphorylation of NreC (NreC-P). NreC-P was shown to bind to the GC rich palindromic region of the narGHIJ, nir and narT genes (Schlag et al., 2008). The NreA protein represents an additional layer of regulation for this TCS. Indeed, in the absence of nitrate, NreA interacts with NreB and inhibits NreB kinase activity (Niemann et al., 2014; Nilkens et al., 2014).
In B. subtilis, the narGHJI operon is regulated by FNRBS (Figure 3). In contrast to E. coli FNR, the [4Fe-4S]2+ cluster of FNRBS is at the C-terminus of the protein (Reents et al., 2006). Interestingly, in this bacterium, nitrate has no direct effect on the narGHJI operon, which seems to be regulated by FNR alone. Indeed, nitrate sensors such as NarXL and NreA have not been identified in B. subtilis. However, on one hand, FNR is transcriptionally regulated by the ResDE TCS that responds to both O2 and NO (Nakano et al., 1996; Nakano and Zhu, 2001; Geng et al., 2007), and on the other, the fnr gene is co-transcribed with the narK gene encoding a nitrite extrusion protein. These results may in part be responsible for the indirect effect of nitrate on nar expression. The NsrR transcriptional repressor also plays a role in nitrate respiration in B. subtilis. The most common target of this regulator in bacteria is the hmp gene, involved in NO detoxification, as observed in B. subtilis (Kommineni et al., 2012). However, it also regulates numerous other genes, including the nitrate reductase operon (nasDEF) (Figure 3; Nakano et al., 2006).
Transcriptional Regulation of the Denitrification Pathway
The regulation of the denitrification pathway has been extensively studied in the γ-proteobacterium P. aeruginosa and in the α-proteobacterium P. denitrificans, and recently reviewed (Gaimster et al., 2018).
In P. aeruginosa, the denitrification pathway is controlled by the TCS NarXL (sensing NO3– and NO2–), two FNR like-proteins ANR and DNR, and the transcriptional regulator NosR, which is sensitive to NO and O2 (Figure 4). The narK1K2GHJI operon is activated by three transcriptional regulators, the ANR and DNR, and the NarXL TCS. In parallel, it was also shown that NarL represses expression of the nap operon during anaerobic growth (Van Alst et al., 2009). In the presence of NO, the expression of nirS, norCB and nos genes is activated by DNR (Arai et al., 1995, 2003). Moreover, ANR also controls the expression of narXL and dnr genes (Schreiber et al., 2007; Figure 4).
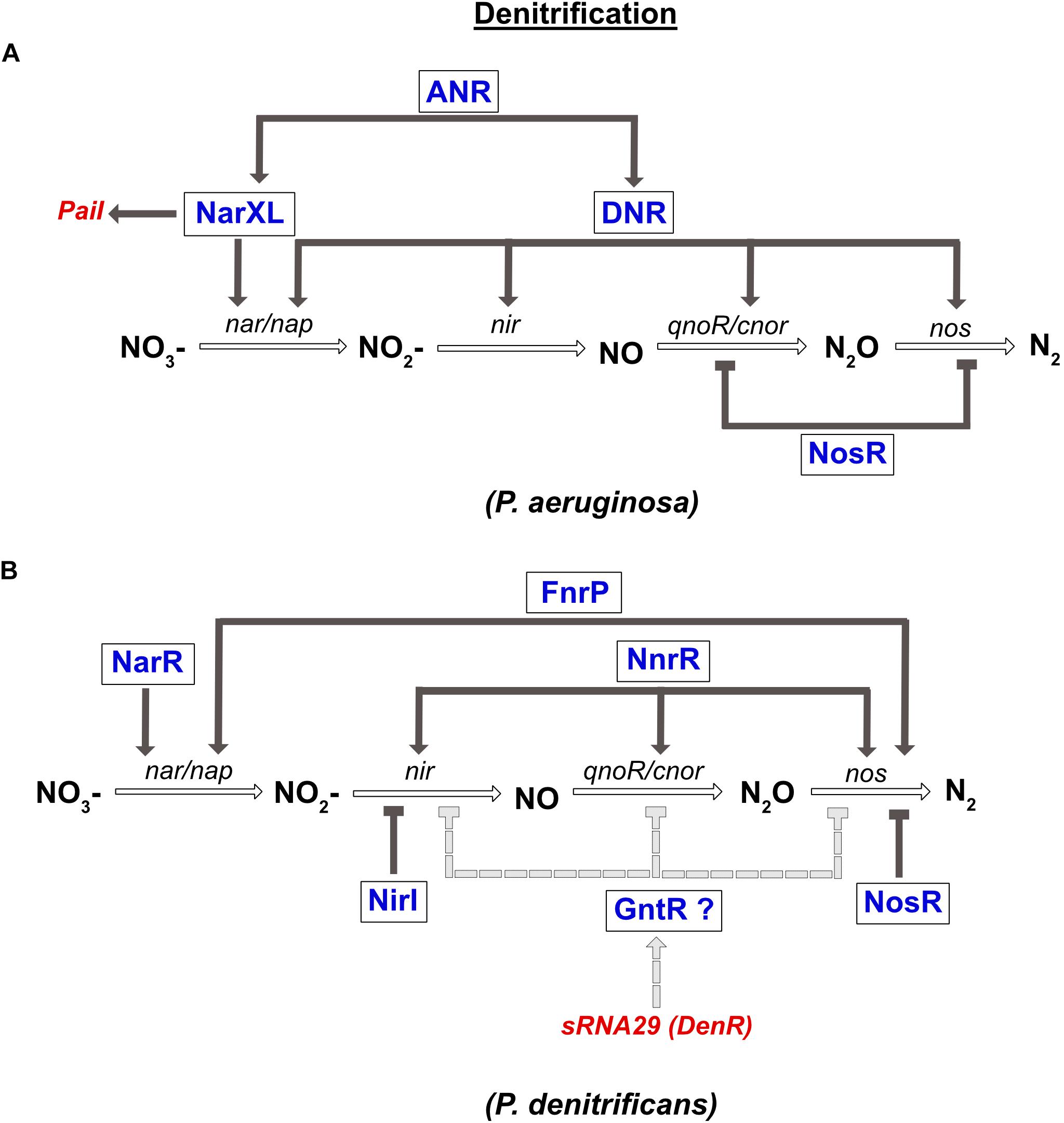
Figure 4. Regulation of denitrification in P. aeruginosa (A) and P. denitrificans (B). (A) Expression of nitrate reductase gene (nar) is directly co-regulated by the NarXL TCS and the DNR transcriptional regulator. Both regulators (NarXL and DNR) are under the control of the FNR-like regulator ANR. DNR also activates expression of the nitrite reductase gene (nir). The nitric oxide reductase (nor) and the nitrous oxide reductase (nos) genes are repressed by the NosR transcriptional repressor. Transcription of the PaiI sRNA is controlled by the NarXL TCS. (B) Expression of nitrate reductase gene (nar) is co-regulated by NarR and FnrP. Expression of nitrite reductase (nir) and nitric oxide reductase (nor) genes are activated solely by NnrR. Expression of nitrous oxide reductase gene (nos) is co-regulated by FnrP and NnrR. NirI represses the expression of nir, while NosR repressed that of nos. Moreover, the recently identified DenR (also known as sRNA-29) inhibits nir, nor and nos expression, probably indirectly via a regulator of the GntR type.
In P. denitrificans, three FNR-like proteins are involved in the regulation of denitrification: FnrP and NnrR that sense O2 and NO levels, and NarR that senses NO3– and NO2– (Lee et al., 2006; Bergaust et al., 2012; Crack et al., 2016; Figure 4). However, in contrast to FnrP, NnrR, and NarR lack the [4Fe-4S]2+ cluster. The NarR TF functionally replaces the NarXL TCS in α-proteobacteria, since no homologs have been identified so far (reviewed in Bueno et al., 2012). The narGHIJ operon is fully induced under anaerobic conditions via FnrP, and by nitrate via the NarR TF (Wood et al., 2001). The NnrR TF controls the expression of the nir, nor, and nos gene clusters (Figure 4; Saunders et al., 1999; Van Spanning et al., 1999; Bergaust et al., 2012). Finally, nos gene expression is also regulated by FnrP (Crack et al., 2016). In addition to these global regulators, two related proteins NirI and NosR, with metal ion-binding or thiol sites (CXXXCP motif), were specifically shown to regulate the expression of the nir and nos genes, respectively (Saunders et al., 1999). As mentioned above, the detrimental effect of NO is avoided by the interaction between nitric oxide reductase and nitrite reductase in P. denitrificans and P. aeruginosa. However, their co-regulation by NnrR is another way to control NO accumulation.
In the α-proteobacteria B. japonicum, Rhodobacter capsulatus, Sinorhizobium meliloti, and R. sphaeroides, similar redox sensitive TCSs have been identified, namely RegSR, RegBA, ActSR, and PrrBA, respectively. All these TCSs share a high sequence similarity in their C-terminal domain. They are involved in the regulation of different metabolic pathways, including nitrogen fixation, and can functionally replace each other (Emmerich et al., 2000). The FnrL regulator and the PrrBA TCS are important for photosynthetic gene expression during anaerobic growth of R. sphaeroides. PrrAB was also shown to induce expression of the nitrate reductase NirK, since a PrrA deletion decreases expression of the nirK-lacZ fusion (Laratta et al., 2002). Another key regulator in R. sphaeroides is the FNR-like protein NnrR, that senses NO, and that is also required for the expression of nirK, as well as that of the norCBQD operon encoding the nitric oxide reductase (Kwiatkowski and Shapleigh, 1996; Tosques et al., 1996; Laratta et al., 2002). This result shows that the NnrR regulon is indirectly controlled by the PrrAB TCS, since NO (synthetized by nirK) is necessary for NnrR function, and suggests that, in contrast to other denitrifying bacteria, R. sphaeroides uses nitrate respiration to ensure redox balancing during photosynthesis, rather than to support anaerobic growth.
In the ε-proteobacterium W. succinogenes, the nap, nrf, and cnos genes involved in nitrate respiration are upregulated in presence of nitrate, NO or N2O but not by nitrite or hydroxylamine (Kern and Simon, 2016). Three TFs, belonging to the Crp/FNR superfamily, are involved in the upregulation of these genes in specific growth condition, namely NssA, B, and C. Nss factors have an N-terminal effector domain and a C-terminal DNA binding domain. Further study will be necessary to understand how nitrate, NO and N2O are sensed by these different TFs. These TFs are homologous to NssR, previously identified in C. jejuni and involved in NO stress resistance (Elvers et al., 2005). However, in C. jejuni, NssR binding sites have not been identified upstream of the nap and nrf genes (Elvers et al., 2005).
In S. coelicolor, which possesses three nitrate reductases (Nar1, Nar2, and Nar3), the OsdRK TCS controls the expression of the narGHJI2 operon (Fischer et al., 2019). Interestingly, Nar1 and Nar3 are not under the control of this TCS, but are induced under anaerobic conditions.
Finally, in the γ-proteobacterium S. loihica PV-4, the global regulator CRP1, which is widely present in bacteria, is necessary to be able to use nitrate in the DNRA pathway, while its paralog CRP2 is required for transcription of the nitrite reductase gene nirK for denitrification (Liu et al., 2020).
Other transcriptional regulators are involved in the control of genes linked to NO detoxification, such as NorR in E. coli which regulates norV and norW and have previously been reviewed by Spiro (2007). However, we consider these transcriptional regulators as beyond the scope of this review.
In addition to such complex transcriptional control of the respiration processes, it has more recently become evident that an additional layer of post-transcriptional regulation by non-coding RNAs is also crucial in the regulation of cellular respiration. sRNAs can rapidly evolve to adapt to specific conditions (reviewed by Dutcher and Raghavan, 2018) and, not surprisingly, recent discoveries have confirmed a role for sRNAs in nitrate respiration (see also Table 1).
Small RNAs Involved in Nitrate Respiration
sRNAs Involved in the DNRA Pathway
Small RNAs involved in the respiration on nitrate have been studied in greatest detail so far in the enterobacteria E. coli or S. enterica, where a wealth of data points to the involvement of several sRNAs in the DNRA pathway. As stated above, the NarX-NarL and NarQ-NarP TCSs play key roles in the regulation of the enzymes involved in this process, and a first link between sRNAs and nitrate respiration came from the identification of sRNAs that target these regulators.
SdsN137 is one of three sRNA isoforms and it represses expression of the narP response regulator gene by pairing to the translation initiation region of this mRNA (Hao et al., 2016). Interestingly, the two other confirmed targets of the SdsN sRNAs are also involved in nitrogen metabolism, as they encode NfsA, a major NADPH-dependent nitroreductase, and HmpA, a nitric oxide dioxygenase. In agreement with the role of NfsA in governing E. coli sensitivity to nitrofuran antibiotics, SdsN was found to protect cells against these compounds (Hao et al., 2016). The same study showed that the SdsN levels respond to the stress/stationary phase sigma factor RpoS, but also change in response to different nitrogen sources (e.g., ammonium or arginine), in a NarP-dependent manner. It is still unclear, however, whether RpoS and NarP are direct regulators of sdsN gene transcription.
More recently, RprA was identified as a second sRNA that directly controls narP mRNA expression through direct base-pairing with its translation initiation region. Of note, in addition to this canonical regulation, RprA also represses narP by acting at the very 5′ end of the narP mRNA, more than 100 nucleotides upstream of the narP start codon (Brosse et al., 2021). Even though the precise mechanism for this additional control is unclear, it is most likely to be indirect, suggesting the existence of yet other narP post-transcriptional regulators. Transcription of RprA sRNA is primarily controlled by the Rcs phosphorelay that responds to envelope stress (Majdalani et al., 2002), and previously reported targets of RprA include the gene encoding the RpoS global stress response protein, and others involved in biofilm formation, conjugation, acid resistance (Majdalani et al., 2001; Mika et al., 2012; Papenfort et al., 2015; Lalaouna et al., 2018), showing that this sRNA is not just dedicated to the control of the nitrate respiration.
Changing the levels of a regulatory protein may not always lead to an effect on its regulon (Batchelor and Goulian, 2003). This is especially true in the case of response regulators that most often need to be modified by phosphorylation to be active. At least in the case of narP control by RprA or SdsN137 sRNAs, the overproduction of either sRNA is sufficient to repress the expression of the nap operon, via their effect on narP regulation, suggesting that control by these sRNAs can really impact nitrate respiration (Brosse et al., 2021).
The narL mRNA, which encodes the response regulator of the NarX-NarL TCS, is also likely subjected to post-transcriptional control by the DicF sRNA. This sRNA accumulates in low oxygen conditions and its gene is present in one copy in the commensal E. coli K-12 genome, but in four copies in the enterohemorrhagic strain 0157:H7 (EHEC) (Murashko and Lin-Chao, 2017; Melson and Kendall, 2019). DicF regulates the expression of genes important for cell division and metabolism (Tetart and Bouché, 1992; Balasubramanian et al., 2016) and enhances EHEC virulence (Melson and Kendall, 2019). narL was among the mRNAs that were differentially expressed in RNAseq analysis performed in the EHEC strain in the presence and the absence of DicF. qPCR data further confirmed that narL mRNA levels increase in the strain deleted for the four dicF genes, and showed that overproduction of any one of the four DicF sRNAs reversed this effect. Whether this is due to a direct interaction between DicF and the narL mRNA, and whether this control is conserved in other bacteria are still open questions.
Finally, there are some indications that the RyhB sRNA could also play a role in the expression of narP and/or narL mRNAs. More precisely, RyhB overproduction significantly repressed the synthesis of a narP-lacZ translational fusion in E. coli (Hao et al., 2016), while qRT-PCR assays suggested that the two Salmonella RyhB homologs, also called RfrA and RfrB, can activate narL and repress narP expression (Teixidó and Cortés, 2010). Again, precise mechanisms for these controls remain to be determined, but a convincing pairing can be predicted between RyhB and the narP mRNA, suggesting a direct interaction in this case (Hao et al., 2016).
As stated above, many enzymes involved in nitrate respiration require metal cofactors such as iron and it is thus interesting that the iron-responsive RyhB sRNA may participate in the regulation of these pathways. This is further exemplified by the regulation of the genes encoding the NirBD nitrite reductase in enterobacteria, since nirB, the first gene of the nirBDC-cysG operon, was predicted as a RyhB target using the CopraRNA pairing prediction algorithm. This interaction was validated by compensatory mutations showing that RyhB represses nirB expression in E. coli (Wright et al., 2013). Conversely, however, the effects of the two RyhB homologs in Salmonella that have been proposed to activate nirBDC expression upon nitrosative stress may be indirect (Calderón et al., 2014).
In addition to sRNAs targeting TFs of the nitrate respiration pathway such as the NarP or NarL response regulators, several sRNAs whose transcription depends on these TFs were also identified. A first example is the NarS sRNA whose levels respond to FNR and NarL TFs (Wang et al., 2020). NarS has been recently identified as an sRNA derived from the 3′ UTR of the narK mRNA, encoding a nitrate and nitrite transporter, through processing by the essential endonuclease RNase E. Transcription of narK is under the direct control of FNR and NarL, which explains how these TFs also control the accumulation of the NarS sRNA. Interestingly, NarS is a Hfq-binding sRNA that represses the expression of the nirC gene, while leaving the expression of the other cistrons of the nirBDC-cysG operon unchanged (Wang et al., 2020). As NirC is involved in nitrite uptake, it was proposed that the negative control of nirC by NarS could limit nitrite re-import under conditions of narS expression, where nitrite can be exported via NarK or reduced to ammonium.
Other cases of sRNAs whose transcription is under the control of TFs of the nitrate respiration pathway have been reported. As previously mentioned, FNR is a major regulator of gene expression in response to anaerobiosis. It regulates hundreds of genes, including genes whose products are directly related to nitrate respiration such as the nap, nir, and nrf operons in E. coli (see above). The FnrS sRNA was recognized early on as part of the FNR regulon (Durand and Storz, 2010) and is thus expressed under anaerobic conditions. Of note, fnrS transcription is also controlled by two other major TFs, ArcA (positively) and CRP (negatively), which allows further accumulation of FnrS, under anaerobic conditions and in glucose-based media, respectively (Durand and Storz, 2010). More than 30 mRNAs are repressed following FnrS pulse-overproduction; they encode proteins involved in diverse functions, such as central metabolism, energy metabolism, aerobic respiration, oxidative stress, that are dispensable under anaerobiosis. FnrS-targets include the NfsA nitroreductase, whose mRNA is also targeted by SdsN, and the CydDC glutathione transporter, important for periplasmic redox control. Interestingly, expression of the cydDC operon is additionally controlled at the transcriptional level by ArcA, NarL, and FNR, with these two latter regulators allowing induction of expression under anaerobic conditions in the presence of alternative electron acceptors, such as nitrate or nitrite (Cook et al., 1997).
Importantly, the ArcA response regulator not only contributes to FnrS synthesis, but also directly represses the transcription of ArcZ, another Hfq-dependent sRNA that targets multiple mRNAs, including rpoS, eptB, sdaC, tpx, mutS, or tolC (Papenfort et al., 2009; Mandin and Gottesman, 2010). The ArcB-ArcA TCS is activated under microaerobic conditions (Alexeeva et al., 2000) and its regulon includes many genes involved in aerobic respiration, thereby partially overlapping the FNR regulon. In addition, and in contrast to FNR, ArcB-ArcA does not directly control expression of genes of the nitrate respiration pathway. However, recent data from RNA–RNA interactome studies strongly support an interaction between the ArcZ sRNA and the napF mRNA and hence suggest an indirect role for ArcA in napF expression through the ArcZ sRNA (Melamed et al., 2016, 2020).
Transcriptional control of sRNAs by TFs involved in nitrate respiration also is true in Gram-positive bacteria. Transcription of RoxS, the only base-pairing sRNA that is conserved in B. subtilis and Staphylococcus aureus (where it is known as RsaE), is for instance directly controlled by the ResDE TCS (or its homolog SsrAB in S. aureus), allowing RoxS induction in the presence of NO (Durand et al., 2015). RoxS has many targets, several of which are involved in carbon and energy metabolism, or in amino acid transport. However, it is not yet clear whether this sRNA plays a direct role in nitrate respiration.
sRNAs Involved in the Denitrification Pathway
Although the biology of sRNAs in general has been less studied in denitrifying bacteria, it is already clear that sRNAs are involved in controlling denitrification. A recent transcriptome analysis determined the sRNA composition of P. denitrificans, the model bacterium for the study of denitrification, under different denitrifying conditions: N2O-producing or N2O-consuming during anaerobiosis, versus aerobic growth without N2O (Gaimster et al., 2016). These conditions were set-up by adding CuSO4 to the medium (N2O-consuming) or omitting it (N2O-producing), as expression of the nitrous oxide reductase NosZ, itself a copper-dependent metalloenzyme required for the last step of the denitrification pathway, depends on copper (Sullivan et al., 2013). This study allowed the identification of several sRNAs with a potential role in denitrification. Among these, the DenR sRNA (for denitrification repressor, previously known as sRNA-29) was shown later to impact denitrification by repressing the expression of several genes involved in this process, such as nirC, norBC, or nosZ, encoding nitrite reductase, subunits of nitric oxide reductase, and nitrous oxide reductase, respectively. These controls are likely indirect and mediated by DenR promoting the synthesis of a GntR-type transcriptional regulator, whose expression follows that of the sRNA, i.e., is highest in the absence of N2O and decreases as N2O levels increase (Gaimster et al., 2019). Of note, this transcriptional regulator was not previously known to control denitrification. As a result, DenR overproduction stalls denitrification at the nitrite reduction step, thereby increasing nitrite levels, while limiting production of NO and N2O.
In P. aeruginosa, another denitrifying bacterium, the 126-nt PaiI sRNA (for P. aeruginosa anaerobically induced RNA I) was identified by RNAseq as the most enriched sRNA under anoxic conditions. Further work showed that PaiI accumulates under anaerobic conditions in the presence of nitrate in an ANR- and NarXL-dependent manner (Tata et al., 2017). The presence of a close-to-consensus NarL site in the PaiI promoter sequence additionally suggests that NarL directly controls paiI transcription, while the ANR-regulation is most likely indirect via activation of NarXL by ANR (Figure 3). Interestingly, PaiI-deleted cells display defects in survival after a shift to anoxic conditions in the presence of nitrate and the activity of nitrite reductase is decreased in the absence of the sRNA (Tata et al., 2017). The molecular basis of these observations is still unclear, but these data nonetheless clearly point to a role for the PaiI sRNA in denitrification.
The PcrZ sRNA of the α-proteobacterium R. sphaeroides is another example of an sRNA responding to regulators of denitrification genes. PcrZ synthesis is induced under low oxygen conditions and this is dependent on the PrrA response regulator and the FnrL TF (Mank et al., 2012). While the presence of a consensus site for PrrA in the pcrZ promoter strongly suggests a direct regulation, this may not be the case for FnrL control (Mank et al., 2012). Both PrrA and FnrL are key regulators of R. sphaeroides anaerobiosis gene expression, and their regulons include genes for the photosynthesis apparatus. In turn, the PcrZ sRNA represses expression of several photosynthesis genes through direct pairing with their mRNAs. Interestingly, transcription of these mRNAs is itself activated by PrrA and FnrL; PcrZ thereby participates in an incoherent feed-forward loop to regulate the expression of photosynthesis genes (Mank et al., 2012). PcrZ does not appear to be directly involved in denitrification at this stage, but is more likely to play a fine-tuning role in the metabolic versatility of R. sphaeroides.
It was also recently proposed that an antisense RNA is produced from the intergenic region between the norQ and norD genes of the norECBQD operon, encoding an NO reductase in the facultative denitrifying bacterium Agrobacterium fabrum (Lecomte et al., 2020). A possible role for this antisense RNA could be to promote denitrification, since N2O production is reduced more than three-fold after inactivation of this antisense RNA. However, further studies are required to both confirm the existence of this regulatory RNA and address its precise mode of action.
Conclusion
Nitrate respiration is an important metabolic pathway, especially when oxygen is limiting, and is regulated at multiple levels. An important control of the nitrate respiration process is exerted at the transcriptional level allowing the integration of numerous environmental signals to precisely control the expression of enzymes involved in this pathway. More recent studies have shown the additional importance of post-transcriptional regulation: the involvement of sRNAs in nitrate respiration is beginning to be recognized, even though this is so far restricted to only a few model bacterial species. In addition, even in these species, their role is very likely still underestimated. Many mRNAs encoding either enzymes or TFs of the DNRA pathway were for instance significantly enriched upon Hfq co-immunoprecipitation in several studies in enterobacteria, suggesting that they could be subject to post-transcriptional control by base-pairing sRNAs as well. Furthermore, high-throughput analyses relying on the ligation of interacting RNAs following their co-immunoprecipitation with Hfq or ProQ, identified many more putative sRNAs interactions with mRNAs whose products are related to the DNRA pathway (e.g., several nap, nar, nrf, or nir genes) (Melamed et al., 2016, 2020). In addition, in S. meliloti, Hfq was found to directly interact both upstream and within the fixLJ ORF, encoding an O2–sensing TCS, and to play a role in its expression (Gao et al., 2016). This control is expected to both directly and indirectly modulate the expression of FixLJ targets, including the nif operon (nifHDK). Although it is still unknown whether fixLJ regulation by Hfq involves an sRNA or not, this is yet another illustration that the post-transcriptional control of nitrate respiration genes is far from being fully understood.
Finally, it is important to emphasize that, at least in the non-denitrifying bacteria, most studies of gene regulation by sRNAs are performed under aerobic conditions, where expression of genes for nitrate respiration may be much lower than under anaerobic conditions. There is no doubt that additional work under more favorable conditions for nitrate respiration would unravel novel sRNA regulations.
Overall, it thus appears that, as for other important metabolic pathways, nitrate respiration, and more generally nitrogen metabolism, are precisely controlled by multiple regulators acting both at the transcriptional and post-transcriptional levels. In addition to these controls of gene expression, regulation of nitrate respiration could be achieved by modulating the activity of enzymes. Indeed, it was shown that the activity of the E. coli NarGHI nitrate reductase was controlled by the dynamics of the complex, that assembles to the cell poles under anaerobic conditions (Alberge et al., 2015). As more details of these regulatory mechanisms are revealed, ideally in a large number of bacterial species, it will be interesting to determine the relative contributions of the diverse regulators involved and their respective advantages in relation to the different nitrate respiration pathways and the environmental conditions encountered by bacteria.
Author Contributions
Both authors listed have made a substantial, direct and intellectual contribution to the work, and approved it for publication.
Funding
Research in our groups is supported by the CNRS, the “Initiative d’Excellence” program from the French State (Grant “DYNAMO,” ANR-11-LABX-0011), the Agence Nationale de la Recherche (ANR grants BaRR–16-CE12-0002-01 and CoNoCo–18-CE12-0025-02 to SD), and the European Research Council (ERC) under the European Union’s Horizon 2020 Research and Innovation Programme (Grant Agreement No. 818750 to MG).
Conflict of Interest
The authors declare that the research was conducted in the absence of any commercial or financial relationships that could be construed as a potential conflict of interest.
Acknowledgments
We are grateful to Mathias Springer and Ciaran Condon for critical reading of the manuscript.
References
Al-Attar, S., and De Vries, S. (2015). An electrogenic nitric oxide reductase. FEBS Lett. 589, 2050–2057. doi: 10.1016/j.febslet.2015.06.033
Alberge, F., Espinosa, L., Seduk, F., Sylvi, L., Toci, R., Walburger, A., et al. (2015). Dynamic subcellular localization of a respiratory complex controls bacterial respiration. Elife 4, 1–14. doi: 10.7554/eLife.05357
Albertsson, I., Sjöholm, J., ter Beek, J., Watmough, N. J., Widengren, J., and Ädelroth, P. (2019). Functional interactions between nitrite reductase and nitric oxide reductase from Paracoccus denitrificans. Sci. Rep. 9, 1–12. doi: 10.1038/s41598-019-53553-z
Alexeeva, S., De Kort, B., Sawers, G., Hellingwerf, K. J., and De Mattos, M. J. T. (2000). Effects of limited aeration and of the ArcAB system on intermediary pyruvate catabolism in Escherichia coli. J. Bacteriol. 182, 4934–4940. doi: 10.1128/JB.182.17.4934-4940.2000
Arai, H., Igarashi, Y., and Kodama, T. (1995). Expression of the nir and nor genes for denitrification of Pseudomonas aeruginosa requires a novel CRP/FNR-related transcriptional regulator, DNR, in addition to ANR. FEBS Lett. 371, 73–76. doi: 10.1016/0014-5793(95)00885-D
Arai, H., Mizutani, M., and Igarashi, Y. (2003). Transcriptional regulation of the nos genes for nitrous oxide reductase in Pseudomonas aeruginosa. Microbiology 149, 29–36. doi: 10.1099/mic.0.25936-0
Balasubramanian, D., Ragunathan, P. T., Fei, J., and Vanderpool, C. K. (2016). A Prophage-Encoded Small RNA Controls Metabolism and Cell Division in Escherichia coli. mSystems 1, e00021–15. doi: 10.1128/mSystems.00021-15
Bali, S., Palmer, D. J., Schroeder, S., Ferguson, S. J., and Warren, M. J. (2014). Recent advances in the biosynthesis of modified tetrapyrroles: the discovery of an alternative pathway for the formation of heme and heme d 1. Cell. Mol. Life Sci. 71, 2837–2863. doi: 10.1007/s00018-014-1563-x
Batchelor, E., and Goulian, M. (2003). Robustness and the cycle of phosphorylation and dephosphorylation in a two-component regulatory system. Proc. Natl. Acad. Sci. U. S. A. 100, 691–696. doi: 10.1073/pnas.0234782100
Bedmar, E. J., Robles, E. F., and Delgado, M. J. (2005). The complete denitrification pathway of the symbiotic, nitrogen-fixing bacterium Bradyrhizobium japonicum. Biochem. Soc. Trans. 33, 141–144. doi: 10.1042/BST0330141
Bedzyk, L., Wang, T., and Ye, R. W. (1999). The periplasmic nitrate reductase in Pseudomonas sp. strain G-179 catalyzes the first step of denitrification. J. Bacteriol. 181, 2802–2806. doi: 10.1128/JB.181.9.2802-2806.1999
Bell, L. C., Richardson, D. J., and Ferguson, S. J. (1992). Identification of nitric oxide reductase activity in Rhodobacter capsulatus: the electron transport pathway can either use or bypass both cytochrome c2 and the cytochrome bc1 complex. J. Gen. Microbiol. 138, 437–443. doi: 10.1099/00221287-138-3-437
Bergaust, L., van Spanning, R. J. M., Frostegård, Å., and Bakken, L. R. (2012). Expression of nitrous oxide reductase in Paracoccus denitrificans is regulated by oxygen and nitric oxide through FnrP and NNR. Microbiology 158, 826–834. doi: 10.1099/mic.0.054148-0
Blasco, F., Iobbi, C., Ratouchniak, J., Bonnefoy, V., and Chippaux, M. (1990). Nitrate reductases of Escherichia coli: sequence of the second nitrate reductase and comparison with that encoded by the narGHJI operon. Mol. Gen. Genet. 222, 104–111. doi: 10.1007/BF00283030
Brosse, A., Walburger, A., Magalon, A., and Guillier, M. (2021). Synthesis of the NarP response regulator of nitrate respiration in Escherichia coli is regulated at multiple levels by Hfq and small RNAs. BioRxiv [Preprint] doi: 10.1101/2021.03.17.435884
Browning, D. F., Cole, J. A., and Busby, S. J. W. (2004). Transcription activation by remodelling of a nucleoprotein assembly: the role of NarL at the FNR-dependent Escherichia coli nir promoter. Mol. Microbiol. 53, 203–215. doi: 10.1111/j.1365-2958.2004.04104.x
Browning, D. F., Lee, D. J., Spiro, S., and Busby, S. J. W. (2010). Down-regulation of the Escherichia coli K-12 nrf promoter by binding of the NsrR nitric oxide-sensing transcription repressor to an upstream site. J. Bacteriol. 192, 3824–3828. doi: 10.1128/JB.00218-10
Bueno, E., Mesa, S., Bedmar, E. J., Richardson, D. J., and Delgado, M. J. (2012). Bacterial adaptation of respiration from oxic to microoxic and anoxic conditions: redox control. Antioxid. Redox Signal. 16, 819–852. doi: 10.1089/ars.2011.4051
Calderón, P. F., Morales, E. H., Acuña, L. G., Fuentes, D. N., Gil, F., Porwollik, S., et al. (2014). The small RNA RyhB homologs from Salmonella typhimurium participate in the response to S-nitrosoglutathione-induced stress. Biochem.Biophys. Res. Commun. 450, 641–645. doi: 10.1016/j.bbrc.2014.06.031
Constantinidou, C., Hobman, J. L., Griffiths, L., Patel, M. D., Penn, C. W., Cole, J. A., et al. (2006). A reassessment of the FNR regulon and transcriptomic analysis of the effects of nitrate, nitrite, NarXL, and NarQP as Escherichia coli K12 adapts from aerobic to anaerobic growth. J. Biol. Chem. 281, 4802–4815. doi: 10.1074/jbc.M512312200
Cook, G. M., Membrillo-Hernández, J., and Poole, R. K. (1997). Transcriptional regulation of the cydDC operon, encoding a heterodimeric ABC transporter required for assembly of cytochromes c and bd in Escherichia coli K-12: regulation by oxygen and alternative electron acceptors. J. Bacteriol. 179, 6525–6530. doi: 10.1128/jb.179.20.6525-6530.1997
Crack, J. C., Hutchings, M. I., Thomson, A. J., and Le Brun, N. E. (2016). Biochemical properties of Paracoccus denitrificans FnrP: reactions with molecular oxygen and nitric oxide. J. Biol. Inorg. Chem. 21, 71–82. doi: 10.1007/s00775-015-1326-7
Crack, J. C., Stapleton, M. R., Green, J., Thomson, A. J., and Le Brun, N. E. (2013). Mechanism of [4Fe-4S](Cys)4 cluster nitrosylation is conserved among NO-responsive regulators. J.Biol. Chem. 288, 11492–11502. doi: 10.1074/jbc.M112.439901
Durand, S., Braun, F., Lioliou, E., Romilly, C., Helfer, A. C., Kuhn, L., et al. (2015). A nitric oxide regulated small RNA controls expression of genes involved in redox homeostasis in Bacillus subtilis. PLoS Gene. 11:e1004957. doi: 10.1371/journal.pgen.1004957
Durand, S., and Storz, G. (2010). Reprogramming of anaerobic metabolism by the FnrS small RNA. Mol. Microbiol. 75, 1215–1231. doi: 10.1111/j.1365-2958.2010.07044.x
Dutcher, H. A., and Raghavan, R. (2018). Origin, Evolution, and Loss of Bacterial Small RNAs. Microbiol. Spectr. 6, 487–497. doi: 10.1128/9781683670247.ch28
Einsle, O., Messerschmidt, A., Huber, R., Kroneck, P. M. H., and Neese, F. (2002). Mechanism of the six-electron reduction of nitrite to ammonia by cytochrome c nitrite reductase. J. Am. Chem. Soc. 124, 11737–11745. doi: 10.1021/ja0206487
Elvers, K. T., Turner, S. M., Wainwright, L. M., Marsden, G., Hinds, J., Cole, J. A., et al. (2005). NssR, a member of the Crp-Fnr superfamily from Campylobacter jejuni, regulates a nitrosative stress-responsive regulon that includes both a single-domain and a truncated haemoglobin. Mol. Microbiol. 57, 735–750. doi: 10.1111/j.1365-2958.2005.04723.x
Emmerich, R., Hennecke, H., and Fischer, H. M. (2000). Evidence for a functional similarity between the two-component regulatory systems RegSR, ActSR, and RegBA (PrrBA) in α-proteobacteria. Arch. Microbiol. 174, 307–313. doi: 10.1007/s002030000207
Falke, D., Biefel, B., Haase, A., Franke, S., Fischer, M., and Gary, R. (2019). Activity of Spore-Specific Respiratory Nitrate Reductase 1 of Streptomyces coelicolor A3(2) Requires a Functional Cytochrome bcc-aa3 Oxidase Supercomplex. J. Bacteriol. 201, 1–13.
Filenko, N., Spiro, S., Browning, D. F., Squire, D., Overton, T. W., Cole, J., et al. (2007). The NsrR regulon of Escherichia coli K-12 includes genes encoding the hybrid cluster protein and the periplasmic, respiratory nitrite reductase. J. Bacteriol. 189, 4410–4417. doi: 10.1128/JB.00080-07
Filenko, N. A., Browning, D. F., and Cole, J. A. (2005). Transcriptional regulation of a hybrid cluster (prismane) protein. Biochem. Soc. Trans. 33, 195–197. doi: 10.1042/BST0330195
Fischer, M., Falke, D., Rönitz, J., Haase, A., Damelang, T., Pawlik, T., et al. (2019). Hypoxia-induced synthesis of respiratory nitrate reductase 2 of Streptomyces coelicolor A3(2) depends on the histidine kinase OsdK in mycelium but not in spores. Microbiology 165, 905–916. doi: 10.1099/mic.0.000829
Gaimster, H., Alston, M., Richardson, D. J., Gates, A. J., and Rowley, G. (2018). Transcriptional and environmental control of bacterial denitrification and N2O emissions. FEMS Microbiol. Lett. 365, 1–8. doi: 10.1093/femsle/fnx277
Gaimster, H., Chalklen, L., Alston, M., Munnoch, J. T., Richardson, D. J., Gates, A. J., et al. (2016). Genome-Wide Discovery of Putative sRNAs in Paracoccus denitrificans Expressed under Nitrous Oxide Emitting Conditions. Front. Microbiol. 7:1806. doi: 10.3389/fmicb.2016.01806
Gaimster, H., Hews, C. L., Griffiths, R., Soriano-Laguna, M. J., Alston, M., Richardson, D. J., et al. (2019). A central small RNA regulatory circuit controlling bacterial denitrification and N2O emissions. mBio 10, 1–12. doi: 10.1128/mBio.01165-19
Gao, M., Nguyen, H., González, I. S., and Teplitski, M. (2016). Regulation of fixLJ by Hfq controls symbiotically important genes in Sinorhizobium meliloti. Mol. Plant Microbe. Interact. 29, 844–853. doi: 10.1094/MPMI-09-16-0182-R
Garcia-Horsman, J. A., Barquera, B., Rumbley, J., Ma, J., and Gennis, R. B. (1994). The superfamily of heme-copper respiratory oxidases. J. Bacteriol. 176, 5587–5600. doi: 10.1128/jb.176.18.5587-5600.1994
Geng, H., Zuber, P., and Nakano, M. M. (2007). Regulation of respiratory genes by ResD-ResE signal transduction system in Bacillus subtilis. Methods Enzymol. 422, 448–464. doi: 10.1016/S0076-6879(06)22023-8
Giel, J. L., Rodionov, D., Liu, M., Blattner, F. R., and Kiley, P. J. (2006). IscR-dependent gene expression links iron-sulphur cluster assembly to the control of O2-regulated genes in Escherichia coli. Mol. Microbiol. 60, 1058–1075. doi: 10.1111/j.1365-2958.2006.05160.x
Gilberthorpe, N. J., and Poole, R. K. (2008). Nitric oxide homeostasis in Salmonella typhimurium: roles of respiratory nitrate reductase and flavohemoglobin. J. Biol. Chem. 283, 11146–11154. doi: 10.1074/jbc.M708019200
Gomes, C. M., Giuffrè, A., Forte, E., Vicente, J. B., Saraiva, L. M., Brunori, M., et al. (2002). A novel type of nitric-oxide reductase: Escherichia coli flavorubredoxin. J. Biol. Chem. 277, 25273–25276. doi: 10.1074/jbc.M203886200
Graf, D. R. H., Jones, C. M., and Hallin, S. (2014). Intergenomic Comparisons Highlight Modularity of the Denitrification Pathway and Underpin the Importance of Community Structure for N2O Emissions. PLoS One 9:e114118. doi: 10.1371/journal.pone.0114118
Grahl, S., Maillard, J., Spronk, C. A. E. M., Vuister, G. W., and Sargent, F. (2012). Overlapping transport and chaperone-binding functions within a bacterial twin-arginine signal peptide. Mol. Microbiol. 83, 1254–1267. doi: 10.1111/j.1365-2958.2012.08005.x
Hao, Y., Updegrove, T. B., Livingston, N. N., and Storz, G. (2016). Protection against deleterious nitrogen compounds: role of sigmaS-dependent small RNAs encoded adjacent to sdiA. Nucl. Acids Res. 44, 6935–6948. doi: 10.1093/nar/gkw404
Hasona, A., Self, W. T., and Shanmugam, K. T. (2001). Transcriptional regulation of the moe (molybdate metabolism) operon of Escherichia coli. Arch. Microbiol. 175, 178–188. doi: 10.1007/s002030100252
Hendriks, J., Oubrie, A., Castresana, J., Urbani, A., Gemeinhardt, S., and Saraste, M. (2000). Nitric oxide reductases in bacteria. Biochim. Biophys. Acta 1459, 266–273. doi: 10.1016/s0005-2728(00)00161-4
Heurlier, K., Thomson, M. J., Aziz, N., and Moir, J. W. B. (2008). The nitric oxide (NO)-sensing repressor NsrR of Neisseria meningitidis has a compact regulon of genes involved in NO synthesis and detoxification. J. Bacteriol. 190, 2488–2495. doi: 10.1128/JB.01869-07
Hino, T., Matsumoto, Y., Nagano, S., Sugimoto, H., Fukumori, Y., Murata, T., et al. (2010). Structural basis of biological N2O generation by bacterial nitric oxide reductase. Science 330, 1666–1670. doi: 10.1126/science.1195591
Hole, U. H., Vollack, K. U., Zumft, W. G., Eisenmann, E., Siddiqui, R. A., Friedrich, B., et al. (1996). Characterization of the membranous denitrification enzymes nitrate reductase (cytochrome cd1) and copper-containing nitrous oxide reductase from Thiobacillus denitrificans. Arch. Microbiol. 165, 55–61. doi: 10.1007/s002030050296
Jayaraman, P. S., Peakman, T. C., Busby, S. J. W., Quincey, R. V., and Cole, J. A. (1987). Location and sequence of the promoter of the gene for the NADH-dependent nitrite reductase of Escherichia coli and its regulation by oxygen, the Fnr protein and nitrite. J. Mol. Biol. 196, 781–788. doi: 10.1016/0022-2836(87)90404-9
Jones, C. M., Graf, D. R. H., Bru, D., Philippot, L., and Hallin, S. (2013). The unaccounted yet abundant nitrous oxide-reducing microbial community: a potential nitrous oxide sink. ISME J. 7, 417–426. doi: 10.1038/ismej.2012.125
Kartal, B., van Niftrik, L., Keltjens, J. T., Op, den Camp, H. J. M., and Jetten, M. S. M. (2012). Anammox-Growth Physiology, Cell Biology, and Metabolism. Adv. Micro. Physiol. 60, 211–262. doi: 10.1016/B978-0-12-398264-3.00003-6
Kern, M., and Simon, J. (2009). Electron transport chains and bioenergetics of respiratory nitrogen metabolism in Wolinella succinogenes and other Epsilonproteobacteria. Biochim. Biophys. Acta 1787, 646–656. doi: 10.1016/j.bbabio.2008.12.010
Kern, M., and Simon, J. (2016). Three transcription regulators of the Nss family mediate the adaptive response induced by nitrate, nitric oxide or nitrous oxide in Wolinella succinogenes. Environ. Microbiol. 18, 2899–2912. doi: 10.1111/1462-2920.13060
Kommineni, S., Lama, A., Popescu, B., and Nakano, M. M. (2012). Global transcriptional control by NsrR in Bacillus subtilis. J. Bacteriol. 194, 1679–1688. doi: 10.1128/JB.06486-11
Kwiatkowski, A. V., and Shapleigh, J. P. (1996). Requirement of nitric oxide for induction of genes whose products are involved in nitric oxide metabolism in Rhodobacter sphaeroides 2.4.3. J. Biol. Chem. 271, 24382–24388. doi: 10.1074/jbc.271.40.24382
Lalaouna, D., Prévost, K., Laliberté, G., Houé, V., and Massé, E. (2018). Contrasting silencing mechanisms of the same target mRNA by two regulatory RNAs in Escherichia coli. Nucl. Acids Res. 46, 2600–2612. doi: 10.1093/nar/gkx1287
Lamberg, K. E., and Kiley, P. J. (2000). FNR-dependent activation of the class II dmsA and narG promoters of Escherichia coli requires FNR-activating regions 1 and 3. Mol. Microbiol. 38, 817–827. doi: 10.1046/j.1365-2958.2000.02172.x
Laratta, W. P., Choi, P. S., Tosques, I. E., and Shapleigh, J. P. (2002). Involvement of the PrrB/PrrA two-component system in nitrite respiration in Rhodobacter sphaeroides 2.4.3: evidence for transcriptional regulation. J. Bacteriol. 184, 3521–3529. doi: 10.1128/JB.184.13.3521-3529.2002
Lecomte, S., Nesme, X., Franzino, T., Villard, C., Pivard, M., Vial, L., et al. (2020). Agrobacterium fabrum C58 involved nitrate reductase NapA and antisense RNA NorR to denitrify. FEMS Microbiol. Ecol. 97, 2–3. doi: 10.1093/femsec/fiaa233
Lee, A. I., Delgado, A., and Gunsalus, R. P. (1999). Signal-dependent phosphorylation of the membrane-bound NarX two-component sensor-transmitter protein of Escherichia coli:nitrate elicits a superior anion ligand response compared to nitrite. J. Bacteriol. 181, 5309–5316. doi: 10.1128/JB.181.17.5309-5316.1999
Lee, Y. Y., Shearer, N., and Spiro, S. (2006). Transcription factor NNR from Paracoccus denitrificans is a sensor of both nitric oxide and oxygen: isolation of nnr∗ alleles encoding effector-independent proteins and evidence for a haem-based sensing mechanism. Microbiology 152, 1461–1470. doi: 10.1099/mic.0.28796-0
Liu, H. P., Takio, S., Satoh, T., and Yamamoto, I. (1999). Involvement in denitrification of the napKEFDABC genes encoding the periplasmic nitrate reductase system in the denitrifying phototrophic bacterium Rhodobacter sphaeroides f. sp. denitrificans. Biosci. Biotechnol. Biochem. 63, 530–536. doi: 10.1271/bbb.63.530
Liu, S., Dai, J., Wei, H., Li, S., Wang, P., Zhu, T., et al. (2020). Dissimilatory Nitrate Reduction to Ammonium (DNRA) and Denitrification Pathways Are Leveraged by Cyclic AMP Receptor Protein (CRP) Paralogues Based on Electron Donor/Acceptor Limitation in Shewanella loihica PV-4. Appl. Environ. Microbiol. 87, 1–17. doi: 10.1128/AEM.01964-20
Maarsingh, J. D., Yang, S., Park, J. G., and Haydel, S. E. (2019). Comparative transcriptomics reveals PrrAB-mediated control of metabolic, respiration, energy-generating, and dormancy pathways in Mycobacterium smegmatis. BMC Genom. 20:942. doi: 10.1186/s12864-019-6105-3
Majdalani, N., Chen, S., Murrow, J., St. John, K., and Gottesman, S. (2001). Regulation of RpoS by a novel small RNA: the characterization of RprA. Mol. Microbiol. 39, 1382–1394. doi: 10.1111/j.1365-2958.2001.02329.x
Majdalani, N., Hernandez, D., and Gottesman, S. (2002). Regulation and mode of action of the second small RNA activator of RpoS translation. RprA. Mol. Microbiol. 46, 813–826. doi: 10.1046/j.1365-2958.2002.03203.x
Malhotra, V., Agrawal, R., Duncan, T. R., Saini, D. K., and Clark-Curtiss, J. E. (2015). Mycobacterium tuberculosis response regulators, DevR and NarL, interact in vivo and co-regulate gene expression during aerobic nitrate metabolism. J. Biol. Chem. 290, 8294–8309. doi: 10.1074/jbc.M114.591800
Mandin, P., and Gottesman, S. (2010). Integrating anaerobic/aerobic sensing and the general stress response through the ArcZ small RNA. EMBO J. 29, 3094–3107. doi: 10.1038/emboj.2010.179
Mank, N. N., Berghoff, B. A., Hermanns, Y. N., and Klug, G. (2012). Regulation of bacterial photosynthesis genes by the small noncoding RNA PcrZ. Proc. Natl. Acad. Sci. U. S. A. 109, 16306–16311. doi: 10.1073/pnas.1207067109
McNicholas, P. M., and Gunsalus, R. P. (2002). The molybdate-responsive Escherichia coli ModE transcriptional regulator coordinates periplasmic nitrate reductase (napFDAGHBC) operon expression with nitrate and molybdate availability. J. Bacteriol. 184, 3253–3259. doi: 10.1128/jb.184.12.3253-3259.2002
Melamed, S., Adams, P. P., Zhang, A., Zhang, H., and Storz, G. (2020). RNA-RNA Interactomes of ProQ and Hfq Reveal Overlapping and Competing Roles. Mol. Cell 77, 411–425. doi: 10.1016/j.molcel.2019.10.022
Melamed, S., Peer, A., Faigenbaum-Romm, R., Gatt, Y. E., Reiss, N., Bar, A., et al. (2016). Global Mapping of Small RNA-Target Interactions in Bacteria. Mol. cell 63, 884–897. doi: 10.1016/j.molcel.2016.07.026
Melson, E. M., and Kendall, M. M. (2019). The sRNA DicF integrates oxygen sensing to enhance enterohemorrhagic Escherichia coli virulence via distinctive RNA control mechanisms Proc. Natl. Acad. Sci. U.S.A. 116, 14210–14215. doi: 10.1073/pnas.1902725116
Mika, F., Busse, S., Possling, A., Berkholz, J., Tschowri, N., Sommerfeldt, N., et al. (2012). Targeting of csgD by the small regulatory RNA RprA links stationary phase, biofilm formation and cell envelope stress in Escherichia coli. Mol. Microbiol. 84, 51–65. doi: 10.1111/j.1365-2958.2012.08002.x
Moreno-Vivián, C., Cabello, P., Martínez-Luque, M., Blasco, R., and Castillo, F. (1999). Prokaryotic nitrate reduction: molecular properties and functional distinction among bacterial nitrate reductases. J. Bacteriol. 181, 6573–6584. doi: 10.1128/jb.181.21.6573-6584.1999
Moura, I., and Moura, J. J. G. (2001). Structural aspects of denitrifying enzymes. Curr. Opinion Chem. Biol. 5, 168–175. doi: 10.1016/S1367-5931(00)00187-3
Müllner, M., Hammel, O., Mienert, B., Schlag, S., Bill, E., and Unden, G. (2008). A PAS domain with an oxygen labile [4Fe-4S]2+ cluster in the oxygen sensor kinase NreB of Staphylococcus carnosus. Biochemistry 47, 13921–13932. doi: 10.1021/bi8014086
Murashko, O. N., and Lin-Chao, S. (2017). Escherichia coli responds to environmental changes using enolasic degradosomes and stabilized DicF sRNA to alter cellular morphology. Proc. Natl. Acad. Sci. U. S. A. 114, E8025–E8034. doi: 10.1073/pnas.1703731114 Google Scholar
Muro-Pastor, A. M., and Hess, W. R. (2020). Regulatory RNA at the crossroads of carbon and nitrogen metabolism in photosynthetic cyanobacteria. Biochim. Biophys. Acta. Gene Regul. Mech. 1863:194477. doi: 10.1016/j.bbagrm.2019.194477
Nakano, M. M., Geng, H., Nakano, S., and Kobayashi, K. (2006). The nitric oxide-responsive regulator NsrR controls ResDE-dependent gene expression. J. Bacteriol. 188, 5878–5887. doi: 10.1128/JB.00486-06
Nakano, M. M., Hoffmann, T., Zhu, Y., and Jahn, D. (1998). Nitrogen and oxygen regulation of Bacillus subtilis nasDEF encoding NADH-dependent nitrite reductase by TnrA and ResDE. J. Bacteriol. 180, 5344–5350. doi: 10.1128/JB.180.20.5344-5350.1998
Nakano, M. M., and Zhu, Y. (2001). Involvement of ResE phosphatase activity in down-regulation of ResD-controlled genes in Bacillus subtilis during aerobic growth. J. Bacteriol. 183, 1938–1944. doi: 10.1128/JB.183.6.1938-1944.2001
Nakano, M. M., Zuber, P., Glaser, P., Danchin, A., and Hulett, F. M. (1996). Two-component regulatory proteins ResD-ResE are required for transcriptional activation of fnr upon oxygen limitation in Bacillus subtilis. J. Bacteriol. 178, 3796–3802. doi: 10.1128/jb.178.13.3796-3802.1996
Niemann, V., Koch-Singenstreu, M., Neu, A., Nilkens, S., Götz, F., Unden, G., et al. (2014). The NreA protein functions as a nitrate receptor in the staphylococcal nitrate regulation system. J. Mol. Biol. 426, 1539–1553. doi: 10.1016/j.jmb.2013.12.026
Nilavongse, A., Brondijk, T. H. C., Overton, T. W., Richardson, D. J., Leach, E. R., and Cole, J. A. (2006). The NapF protein of the Escherichia coli periplasmic nitrate reductase system: demonstration of a cytoplasmic location and interaction with the catalytic subunit, NapA. Microbiology 152, 3227–3237. doi: 10.1099/mic.0.29157-0
Nilkens, S., Koch-Singenstreu, M., Niemann, V., Götz, F., Stehle, T., and Unden, G. (2014). Nitrate/oxygen co-sensing by an NreA/NreB sensor complex of Staphylococcus carnosus. Mol. Microbiol. 91, 381–393. doi: 10.1111/mmi.12464
Noriega, C. E., Lin, H.-Y., Chen, L.-L., Williams, S. B., and Stewart, V. (2010). Asymmetric cross-regulation between the nitrate-responsive NarX-NarL and NarQ-NarP two-component regulatory systems from Escherichia coli K-12. Mol. Microbiol. 75, 394–412. doi: 10.1111/j.1365-2958.2009.06987.x
Ogawa, K., Akagawa, E., Yamane, K., Sun, Z. W., LaCelle, M., Zuber, P., et al. (1995). The nasB operon and nasA gene are required for nitrate/nitrite assimilation in Bacillus subtilis. J. Bacteriol. 177, 1409–1413. doi: 10.1128/jb.177.5.1409-1413.1995
Papenfort, K., Espinosa, E., Casadesus, J., and Vogel, J. (2015). Small RNA-based feedforward loop with AND-gate logic regulates extrachromosomal DNA transfer in Salmonella. Proc. Natl. Acad. Sci. U. S. A. 112, E4772–E4781. doi: 10.1073/pnas.1507825112
Papenfort, K., Said, N., Welsink, T., Lucchini, S., Hinton, J. C., and Vogel, J. (2009). Specific and pleiotropic patterns of mRNA regulation by ArcZ, a conserved. Hfq-dependent small RNA. Mol. Microbiol. 74, 139–158. doi: 10.1111/j.1365-2958.2009.06857.x
Philippot, L., Andert, J., Jones, C. M., Bru, D., and Hallin, S. (2011). Importance of denitrifiers lacking the genes encoding the nitrous oxide reductase for N2O emissions from soil. Glob. Change Biol. 17, 1497–1504. doi: 10.1111/j.1365-2486.2010.02334.x
Prasse, D., and Schmitz, R. A. (2018). Small RNAs Involved in Regulation of Nitrogen Metabolism. Microbiol. Spect. 6, 1–17. doi: 10.1128/microbiolspec.rwr-0018-2018
Reents, H., Münch, R., Dammeyer, T., Jahn, D., and Härtig, E. (2006). The Fnr regulon of Bacillus subtilis. J. Bacteriol. 188, 1103–1112. doi: 10.1128/JB.188.3.1103-1112.2006
Richardson, D. J., Berks, B. C., Russell, D. A., Spiro, S., and Taylor, C. J. (2001). Functional, biochemical and genetic diversity of prokaryotic nitrate reductases. Cell Mol. Life Sci. 58, 165–178. doi: 10.1007/PL00000845
Richardson, D. J., Van Spanning, R. J. M., and Ferguson, S. J. (2007). “The Prokaryotic Nitrate,” in Biology of the Nitrogen, eds H. Bothe, S. J. Ferguson, and W. E. Newton (Netherlands: Elsevier), doi: 10.1016/B978-044452857-5.50003-5
Rodionov, D. A., Dubchak, I. L., Arkin, A. P., Alm, E. J., and Gelfand, M. S. (2005). Dissimilatory metabolism of nitrogen oxides in bacteria: comparative reconstruction of transcriptional networks. PLoS Comput. Biol. 1:e55. doi: 10.1371/journal.pcbi.0010055
Sanford, R. A., Wagner, D. D., Wu, Q., Chee-Sanford, J. C., Thomas, S. H., Cruz-García, C., et al. (2012). Unexpected nondenitrifier nitrous oxide reductase gene diversity and abundance in soils. Proc. Natl. Acad. Sci. U. S. A. 109, 19709–19714. doi: 10.1073/pnas.1211238109
Saunders, N. F. W., Houben, E. N. G., Koefoed, S., De Weert, S., Reijnders, W. N. M., Westerhoff, H. V., et al. (1999). Transcription regulation of the nir gene cluster encoding nitrite reductase of Paracoccus denitrificans involves NNR and NirI, a novel type of membrane protein. Mol. Microbiol. 34, 24–36. doi: 10.1046/j.1365-2958.1999.01563.x
Schlag, S., Fuchs, S., Nerz, C., Gaupp, R., Engelmann, S., Liebeke, M., et al. (2008). Characterization of the oxygen-responsive NreABC regulon of Staphylococcus aureus. J. Bacteriol. 190, 7847–7858. doi: 10.1128/JB.00905-08
Schreiber, K., Krieger, R., Benkert, B., Eschbach, M., Arai, H., Schobert, M., et al. (2007). The Anaerobic Regulatory Network Required for Pseudomonas aeruginosa Nitrate Respiration. J. Bacteriol. 189, 4310–4314. doi: 10.1128/JB.00240-07
Shiro, Y. (2012). Structure and function of bacterial nitric oxide reductases: nitric oxide reductase, anaerobic enzymes. Biochim. Biophys. Acta Bioenerg. 1817, 1907–1913. doi: 10.1016/j.bbabio.2012.03.001
Sievert, S. M., Scott, K. M., Klotz, M. G., Chain, P. S. G., Hauser, L. J., Hemp, J., et al. (2008). Genome of the Epsilonproteobacterial Chemolithoautotroph Sulfurimonas denitrificans. Appl. Environ. Microbiol. 74, 1145–1156. doi: 10.1128/AEM.01844-07
Simon, J. (2002). Enzymology and bioenergetics of respiratory nitrite ammonification. FEMS Microbiol. Rev. 26, 285–309. doi: 10.1016/S0168-6445(02)00111-0
Simon, J., Einsle, O., Kroneck, P. M. H., and Zumft, W. G. (2004). The unprecedented nos gene cluster of Wolinella succinogenes encodes a novel respiratory electron transfer pathway to cytochrome c nitrous oxide reductase. FEBS Lett. 569, 7–12. doi: 10.1016/j.febslet.2004.05.060
Sousa, F. L., Alves, R. J., Ribeiro, M. A., Pereira-Leal, J. B., Teixeira, M., and Pereira, M. M. (2012). The superfamily of heme-copper oxygen reductases: types and evolutionary considerations. Biochim. Biophys. Acta 1817, 629–637. doi: 10.1016/j.bbabio.2011.09.020
Sparacino-Watkins, C., Stolz, J. F., and Basu, P. (2014). Nitrate and periplasmic nitrate reductases. Chem. Soc. Reviews. 43, 676–706. doi: 10.1039/c3cs60249d.Nitrate
Spector, M. P., Del Portillo, F. G., Bearson, S. M. D., Mahmud, A., Magut, M., Finlay, B. B., et al. (1999). The rpoS-dependent starvation-stress response locus stiA encodes a nitrate reductase (narZYWV) required for carbon-starvation-inducible thermotolerance and acid tolerance in Salmonella typhimurium. Microbiology 145, 3035–3045. doi: 10.1099/00221287-145-11-3035
Spiro, S. (2007). Regulators of bacterial responses to nitric oxide. FEMS Microbiol. Rev. 31, 193–211. doi: 10.1111/j.1574-6976.2006.00061.x
Stewart, V., Lu, Y., and Darwin, A. J. (2002). Periplasmic nitrate reductase (NapABC enzyme) supports anaerobic respiration by Escherichia coli K-12. J. Bacteriol. 184, 1314–1323. doi: 10.1128/JB.184.5.1314-1323.2002
Suharti, and de Vries, S. (2005). Membrane-bound denitrification in the Gram-positive bacterium Bacillus azotoformans. Biochem. Soc. Trans. 33, 130–133. doi: 10.1042/BST0330130
Sullivan, M. J., Gates, A. J., Appia-Ayme, C., Rowley, G., and Richardson, D. J. (2013). Copper control of bacterial nitrous oxide emission and its impact on vitamin B12-Dependent metabolism. Proc. Natl. Acad. Sci. U. S. A. 110, 19926–19931. doi: 10.1073/pnas.1314529110
Tata, M., Amman, F., Pawar, V., Wolfinger, M. T., Weiss, S., Häussler, S., et al. (2017). The Anaerobically Induced sRNA Pail Affects Denitrification in Pseudomonas aeruginosa PA14. Front. Microbiol. 23:2312. doi: 10.3389/fmicb.2017.02312
Teixidó, L., and Cortés, P. (2010). Control by Fur of the nitrate respiration regulators NarP and NarL in Salmonella enterica. Int. Microbiol. 13, 33–39. doi: 10.2436/20.1501.01.109
Tetart, F., and Bouché, J. P. (1992). Regulation of the expression of the cell-cycle gene ftsZ by DicF antisense RNA. Division does not require a fixed number of FtsZ molecules. Mol. Microbiol 6, 615–620. doi: 10.1111/j.1365-2958.1992.tb01508.x
Tosques, I. E., Shi, J., and Shapleigh, J. P. (1996). Cloning and characterization of nnrR, whose product is required for the expression of proteins involved in nitric oxide metabolism in Rhodobacter sphaeroides 2.4.3. J. Bacteriol. 178, 4958–4964. doi: 10.1128/jb.178.16.4958-4964.1996
Tucker, N. P., Le Brun, N. E., Dixon, R., and Hutchings, M. I. (2010). There’s NO stopping NsrR, a global regulator of the bacterial NO stress response. Trends. Microbiol. 18, 149–156. doi: 10.1016/j.tim.2009.12.009
Urem, M., van Rossum, T., Bucca, G., Moolenaar, G. F., Laing, E., Świątek-Połatyńska, M. A., et al. (2016). OsdR of Streptomyces coelicolor and the Dormancy Regulator DevR of Mycobacterium tuberculosis Control Overlapping Regulons. mSystems 1, e00014–16. doi: 10.1128/mSystems.00014-16
Van Alst, N. E., Sherrill, L. A., Iglewski, B. H., and Haidaris, C. G. (2009). Compensatory periplasmic nitrate reductase activity supports anaerobic growth of Pseudomonas aeruginosa PAO1 in the absence of membrane nitrate reductase. Can J Microbiol. 55, 1133–1144. doi: 10.1139/w09-065
Van Spanning, R. J. M., Houben, E., Reijnders, W. N. M., Spiro, S., Westerhoff, H. V., and Saunders, N. (1999). Nitric oxide is a signal for NNR-mediated transcription activation in Paracoccus denitrificans. J. Bacteriol. 181, 4129–4132. doi: 10.1128/jb.181.13.4129-4132.1999
Vázquez-Torres, A., and Bäumler, A. J. (2016). Nitrate, nitrite and nitric oxide reductases: from the last universal common ancestor to modern bacterial pathogens. Curr. Opin. Microbiol. 29, 1–8. doi: 10.1016/j.mib.2015.09.002
Vine, C. E., and Cole, J. A. (2011). Nitrosative stress in Escherichia coli: reduction of nitric oxide. Biochem. Soc. Transac. 39, 213–215. doi: 10.1042/BST0390213
Vinogradov, S. N., Tinajero-Trejo, M., Poole, R. K., and Hoogewijs, D. (2013). Bacterial and archaeal globins - A revised perspective. Biochim. Biophys. Acta Proteins. Proteom. 1834, 1789–1800. doi: 10.1016/j.bbapap.2013.03.021
Wang, C., Chao, Y., Matera, G., Gao, Q., and Vogel, J. (2020). The conserved 3’ UTR-derived small RNA NarS mediates mRNA crossregulation during nitrate respiration. Nucl. Acids Res. 48, 2126–2143. doi: 10.1093/nar/gkz1168
Wittorf, L., Jones, C. M., Bonilla-Rosso, G., and Hallin, S. (2018). Expression of nirK and nirS genes in two strains of Pseudomonas stutzeri harbouring both types of NO-forming nitrite reductases. Res. Microbiol. 169, 343–347. doi: 10.1016/j.resmic.2018.04.010
Wood, N. J., Alizadeh, T., Bennett, S., Pearce, J., Ferguson, S. J., Richardson, D. J., et al. (2001). Maximal expression of membrane-bound nitrate reductase in Paracoccus is induced by nitrate via a third fnr-like regulator named NarR. J. Bacteriol. 183, 3606–3613. doi: 10.1128/JB.183.12.3606-3613.2001
Wright, P. R., Richter, A. S., Papenfort, K., Mann, M., Vogel, J., Hess, W. R., et al. (2013). Comparative genomics boosts target prediction for bacterial small RNAs. Proc. Natl Acad Sci. 110, E3487–E3496. doi: 10.1073/pnas.1303248110
Yoon, S., Sanford, R. A., and Löffler, F. E. (2015). Nitrite control over dissimilatory nitrate/nitrite reduction pathways in Shewanella loihica strain PV-4. Appl. Environ. Microbiol. 81, 3510–3517. doi: 10.1128/AEM.00688-15
Keywords: denitrification, dissimilatory nitrate reduction, two-component systems, FNR, nitrate reductase, small RNAs, Hfq
Citation: Durand S and Guillier M (2021) Transcriptional and Post-transcriptional Control of the Nitrate Respiration in Bacteria. Front. Mol. Biosci. 8:667758. doi: 10.3389/fmolb.2021.667758
Received: 14 February 2021; Accepted: 29 March 2021;
Published: 07 May 2021.
Edited by:
Björn Voß, University of Stuttgart, GermanyReviewed by:
Stephan Klähn, Helmholtz Centre for Environmental Research (UFZ), GermanyClaudio Valverde, National University of Quilmes, Argentina
Copyright © 2021 Durand and Guillier. This is an open-access article distributed under the terms of the Creative Commons Attribution License (CC BY). The use, distribution or reproduction in other forums is permitted, provided the original author(s) and the copyright owner(s) are credited and that the original publication in this journal is cited, in accordance with accepted academic practice. No use, distribution or reproduction is permitted which does not comply with these terms.
*Correspondence: Sylvain Durand, c3lsdmFpbi5kdXJhbmRAaWJwYy5mcg==; Maude Guillier, bWF1ZGUuZ3VpbGxpZXJAaWJwYy5mcg==