- 1School of Biological Sciences, University of Reading, Reading, United Kingdom
- 2The Pirbright Institute, Woking, United Kingdom
Bovine tuberculosis caused by Mycobacterium bovis, is a significant global pathogen causing economic loss in livestock and zoonotic TB in man. Several vaccine approaches are in development including reverse vaccinology which uses an unbiased approach to select open reading frames (ORF) of potential vaccine candidates, produce them as recombinant proteins and assesses their immunogenicity by direct immunization. To provide feasibility data for this approach we have cloned and expressed 123 ORFs from the M. bovis genome, using a mixture of E. coli and insect cell expression. We used a concatenated open reading frames design to reduce the number of clones required and single chain fusion proteins for protein pairs known to interact, such as the members of the PPE-PE family. Over 60% of clones showed soluble expression in one or the other host and most allowed rapid purification of the tagged bTB protein from the host cell background. The catalogue of recombinant proteins represents a resource that may be suitable for test immunisations in the development of an effective bTB vaccine.
Introduction
Bovine tuberculosis (bTB) is a major disease throughout the world and is particularly prominent in Africa and parts of Asia. While many developed countries have reduced or eliminated bTB from their cattle populations, major hotspots remain in Europe, America, Canada and New Zealand (Smith, 2012). Phylogenetic analysis of >1,000 non-redundant M. bovis genomes has suggested divergence from a last common ancestor with M. caprae ∼ 3,500 years ago with spread since aided by animal trade (Zimpel et al., 2020). Control is effected by large scale cattle testing and animals scoring positive are subsequently culled, with an acknowledged impact on sustainability and an increased risk of human infection (Chambers et al., 2014). Various control strategies have been implemented, including those with limited public appeal (Bielby et al., 2014), but it is generally accepted that control in the longer term will require the development of an efficacious cattle vaccine. Multiple potential vaccines have been described (Vordermeier and Hewinson, 2006; Kaufmann et al., 2017; McShane, 2019) but the lead contender for early use remains Bacillus Calmette-Guerin (BCG), the cornerstone of human vaccine programmes. However, many of the proteins present in the purified protein derivative (PPD) used in herd screening programs are also present in BCG leading to BCG immunised animals scoring positive, a complication if immunisation and screening occur concurrently. To overcome this, some protein markers, either missing or non-immunogenic in BCG, have been developed to differentiate between infected and vaccinated status (Whelan et al., 2010; Vordermeier et al., 2011; Srinivasan et al., 2019). An alternate approach, to develop a BCG based vaccine that does not lead to positivity in the screening test has also been investigated (Chandran et al., 2019). Although BCG derives from M. bovis, comparative genomics has revealed genomic losses in the variously passaged BCG stocks when compared to ancestral stocks (Zhang et al., 2013) suggesting that some BCG vaccine stocks may be incapable of providing suitable levels of protection unless supplemented with additional proteins representing the missing ORFs. A number of candidates have been investigated in this respect, including Ag85, ESAT-6, CFP-10 and members of the PPE family (Li et al., 2015; Dai et al., 2017; Tkachuk et al., 2017; Stylianou et al., 2018) although generally as single antigens rather than as a mixture of candidates. As a result, the case has also been made that current candidate vaccines lack diversity and that a broader range of proteins should be considered (Fletcher and Schrager, 2016).
Reverse vaccinology (Rappuoli, 2001; Serruto et al., 2012; Rappuoli et al., 2016) assumes that molecules on the cell surface of a target organism include those that may act as an effective vaccine even if they do not raise a significant serum response following natural infection. The availability of whole bacterial genomes, suitable bioinformatics and high throughput protein expression technologies allows this concept to be tested, with some notable successes (Muruato et al., 2017; Masignani et al., 2019). The feasibility of this approach is in part determined by how many and how easily bTB proteins can be expressed and purified. For Mycobacterium tuberculosis, progress in multiple protein expression has been made by the TB Structural Genomics Consortium, although the proteins selected were based primarily on their suitability as drug targets (Chim et al., 2011). To assess feasibility for proteins that might be targeted for vaccine use and to provide such proteins for testing we report a high throughput expression approach (Aricescu et al., 2006; Nettleship et al., 2010; Bird et al., 2014) to the expression of many full-length Mycobacterium bovis ORFs.
Materials and Methods
Selection of Vaccine Candidates
Candidate bTB proteins were selected by reference to lists derived from published bioinformatics approaches (e.g. (He et al., 2010; Monterrubio-López et al., 2015)) as well as current web sources (Kapopoulou et al., 2011; Dhanda et al., 2016). Throughout the study the genome of Mycobacterium bovis strain AF2122/97 was used as the reference strain. Proteins with a range of putative functions related to vaccine use were included, for example roles in virulence, predicted surface expression, previous use as a candidate vaccine and roles in the immune response and immune evasion.
Molecular Cloning
Sequences representing the candidate ORFs were ordered as custom designed double stranded DNA fragments (Integrated DNA Technologies, Belgium). Sequence predicted to encode transmembrane domains was removed and synthetic genes were codon optimised for Spodoptera frugiperda (Sf9) or E. coli to maximise expression in the relevant host. All DNA fragments were flanked with 18 base pairs of sequence homologous to the vector pTriEx 1.1 (EMD Biosciences), suitable for expression in E. coli under control of the T7 promoter or insect cells under control of the baculovirus very late P10 promoter. All clones were generated by Gibson assembly (Gibson et al., 2009). The sequence designs were such that translation initiated at the bTB ORF initiation codon and fused the C-terminus with the histidine tag present in the vector, except when otherwise indicated. The pTriEx-1.1 cloning vector was linearised by digestion with restriction enzymes XhoI and NcoI (Thermo Fisher Scientific, Paisley, United Kingdom) and 20 ng gene fragment was assembled into 50 ng linearized pTriEx-1.1 cloning vector using the NEBuilder HiFi DNA Assembly Protocol (NEB, United Kingdom). Clones were isolated following transformation of Stellar competent cells (Takara Bio, France) with confirmation by colony PCR followed by DNA sequencing.
E. Coli Expression
The default strain for protein expression in E. coli was SoluBL21 (Kim et al., 2015). Alternate hosts were E. coli T7 Express lysY (NEB) and LOBSTR (Andersen et al., 2013) which were used for low yielding constructs. Initial screening was achieved with cultures of ∼20 ml and scaled according to the outcome. Cells were grown at 37°C to an OD600 = 0.6 and induced by the addition of IPTG to 0.1 mM. After induction, growth was continued for 4 h at 37°C. Cultures were harvested by centrifugation at 4,000 × g for 15 min and disrupted for gel analysis or purification as required. Solubility was determined after lysis in 1% Triton-X detergent and gel analysis of the soluble and pellet fractions. Poor solubility was corrected, where possible, with low temperature induction (16°C) or by varying the IPTG concentration used for induction. Intractable expression was abandoned in favour of alternate constructs or the alternate host.
Recombinant Baculovirus Expression
Recombinant baculoviruses were produced by co-transfection of Sf9 cells with transfer vectors and linearised baculovirus DNA as described (Porta et al., 2013). Transfections were done in 6 well tissue culture plates at a monolayer confluency of 50%. Viruses were passaged when significant cytopathic effect was observed and viral stocks were amplified to high titre, typically 3 passages, prior to use for infection and protein detection. All viral stocks were produced in Sf9 cells. For protein expression, 100 ml suspension cultures of AoTni38 cells (Hashimoto et al., 2012) were infected at a density of 2 × 106 per ml with 10 ml of high titre (>107 pfu per ml) virus stock and the culture continued for 3 days. Cells were harvested by centrifugation and processed as described.
SDS PAGE
Protein samples were prepared using NuPAGE loading buffer (Fisher Scientific UK Ltd., United Kingdom) and heated to 98°C for 10 min before loading the gel. Proteins were separated by SDS-PAGE using 4–12% precast Tris-Glycine SDS polyacrylamide gels (Fisher Scientific UK Ltd., United Kingdom) for 30 min at 200 V. SDS-PAGE loading used the equivalent of 50 μL of bacterial culture or 5 × 104 insect cells per lane of a 10 lane, 10 cm gel. After electrophoresis, gels were subjected to either Coomassie Brilliant Blue R250 staining or transferred to a polyvinylidene difluoride (PVDF) membrane for western blot analysis.
Western Blot
Proteins were transferred electrophoretically to PVDF membranes (Immobilon®, Merck, Germany) by semi-dry transfer. Membranes were blocked with Protein-Free T20 (TBS) Blocking Buffer (Fisher Scientific UK Ltd., United Kingdom) for 1 h. All subsequent incubations and washes were done in Tris-buffer saline containing 0.1% Tween 80 (TBST). Membranes were incubated with monoclonal Anti-6X His tag® antibody conjugated to HRP (Abcam, United Kingdom) at 1:2000 dilution followed by three washes of 5 min each. The membrane was finally washed with TBS and antibody reactive bands were revealed using ECL Western Blotting Detection Reagent (Geneflow, United Kingdom) and imaged using a Syngene G-Box (Syngene, United Kingdom).
Purification
E.coli cell pellets were lysed in 10% of the original culture volume in a lysis buffer (500 mM NaCl, 20 mM Sodium phosphate buffer pH 7.4, 20 mM imidazole, 1% Triton X-100) supplemented with 1% lysozyme, 1 mM phenylmethanesulfonyl fluoride and 1 mM benzamidine hydrochloride and incubated for 10 min at 4°C. Samples were sonicated for 10 min on ice followed by centrifugation at 15,000 × g for 10 min. Infected insect cells were disrupted similarly but without lysozyme in the lysis buffer. Expressed proteins, with an affinity tag of six consecutive histidine residues, were purified by His-mag sepharose Ni beads (GE Life Sciences) using 200 µL bead slurry per preparation. Beads were incubated with the clarified lysate for a minimum of 2 h on a blood wheel before washing and elution according to the vendor’s instructions. For proteins that demonstrated a tendency to insolubility the lysis buffer also contained 34 mM SDS and the wash and elution buffers included 0.1% sodium sarkosyl (Schlager et al., 2012). For secreted proteins the clarified supernatant was adjusted to 0.5 mM nickel sulphate and used directly for IMAC pull-down. Constructs that were positive for expression but failed purification by one of these routes were abandoned.
Results
Identification and Generation of Sequences Encoding Putative Vaccine Candidates
The selection of candidates for this study was based on one or more vaccine related properties, viz. their predicted biological function (cell surface protein, virulence or adhesion), predicted immunogenicity, previous use as a candidate vaccine or sequence conservation in multiple mycobacterial genomes. From these considerations the final ORFs selected were grouped into seven loose descriptive categories (Table 1). For ORFs representing complete proteins with a predicted molecular mass of greater than ∼15 kDa, synthetic DNA was produced to the entire coding region with flanking regions that ensured a unique initiator ATG downstream of the Shine-Dalgarno sequence and fusion with a sequence encoding polyhistidine at the carboxyl terminus of the expressed protein, both features of the vector used (Figure 1A). If a signal peptide was present in the original M. bovis ORF, assessed by routine submission to the SigP server (Nielsen et al., 2019), it was changed to that of honeybee melittin, known to be efficiently processed in insect cells (Tessier et al., 1991). Sequences predicted to encode transmembrane domains were deleted. In cases where expression of the recombinant protein was either poor or, more usually, insoluble, the design was changed to incorporate an N-terminal polyhistidine sequence after the initiator codon and a stop codon prior to the vector encoded polyhistidine sequence (Figure 1A).
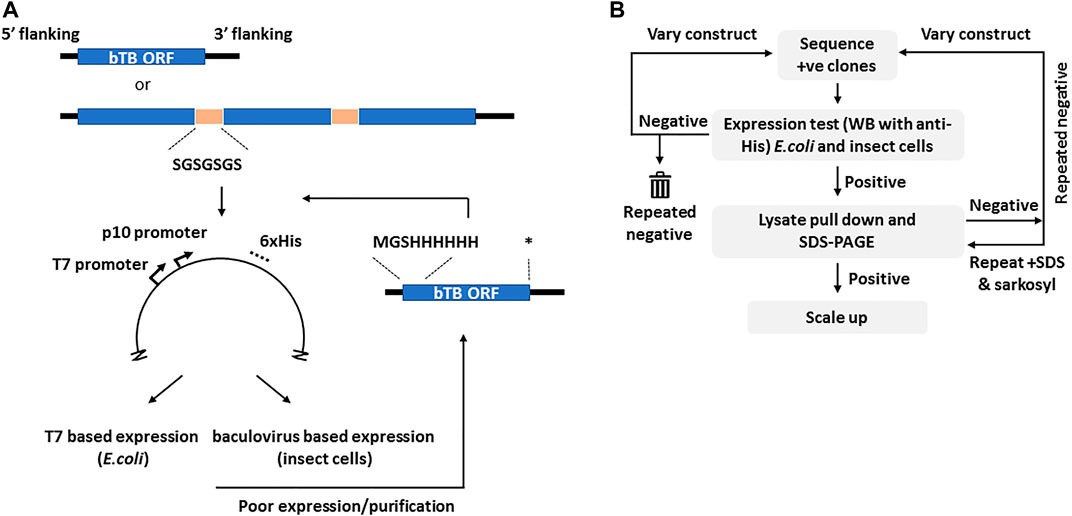
FIGURE 1. The cloning and selection strategies for the generation of the M. bovis recombinant protein atlas. Panel (A). Generic construct design showing the use of either complete or concatenated ORFs tagged at the C-terminus with polyhistidine as present in vector pTriEx1.1. In the event of poor expression the same ORFs were re-cloned with an N-terminal His tag as shown. The sequence of the flexible linker is indicated. Asterisk–stop codon. Panel (B). The protein expression and purification regimen showing the iterative nature of the process. The varied constructs are those with alternate His tags shown in (A). Initial screening was by Western blot for the His tag in all cases.
More than half of the expressed proteins that were problematic in recombinant form with a C-terminal polyhistidine were improved by this strategy, as described below. For proteins that were <15 kDa, or for proteins whose database entry did not contain an initiator methionine, for example some members of the esx family, sequences were concatenated before synthesis so that several ORFs were expressed together, connected by a Gly-Ser linker of 7 residues (Figure 1A). Similarly, for protein pairs such as the proline-proline-glutamate (PPE) and proline-glutamate (PE) complexes, the matching pair was synthesised as a single chain also connected by a flexible linker. The available crystal structures of such complexes show the C-terminus of the PPE partner lying close to the N-terminus of the paired PE (Strong et al., 2006), a distance easily spanned by the added linker. Some M. bovis ORFs were persistently difficult to express in either E. coli or insect cells and in these cases workaround strategies involved fusion with solubility tags including GFP, SUMO or the B1 domain of streptococcal protein G (GB1) (Lindhout et al., 2003; Pengelley et al., 2006; Lee et al., 2008). Some of these strategies improved expression or purification for some of the ORFs but none provided a universal route to improvement.
Protein Expression and Purification
To maximise the possibility of efficient expression of a diverse range of selected M. bovis ORFs as soluble proteins, two different expression systems were used, E. coli and recombinant baculoviruses. Screening of the clones or recombinant viruses was done in small scale cultures by Western blot with a directly conjugated antibody recognizing the polyhistidine tag (Figure 1B). The default result was a band of the molecular mass predicted for the translated protein but occasionally the band identified by the Western blot had bands additional to the predicted mass (marked in Figure 2A). We assumed smaller sizes were the result of protein degradation, with the residual C-terminal His tagged fragment being detected, whereas larger proteins were post-translationally modified or oligomeric. Notably, the concatenated PPE-PE complexes gave rise to stable products of the predicted molecular mass (marked in Figure 2B) as did the Esx concatenates (marked in Figure 2A), some of which have been reported elsewhere (e.g., (Li et al., 2016; Mearns et al., 2017)). Concatenated constructs Mb1036_Mb1129c, Mb1272_Mb2477c, Mb3070_Mb3641 and Mb3338_Mb3644c also showed predominantly single bands at the predicted molecular mass when probed with an anti-His antibody (marked in Figure 2B).
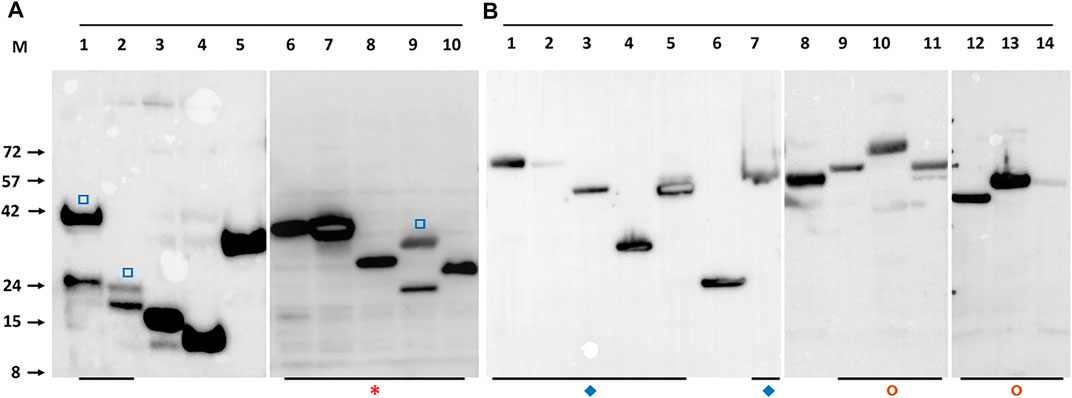
FIGURE 2. Example Western blot detection of recombinant M. bovis proteins with anti-His antibody. Panel (A). Detection of M. bovis ORFs expressed in induced E.coli. The lane identity and predicted molecular mass are: 1-Mb1228 (39 kDa), 2-Mb1916 (18 kDa), 3-Mb2410 (15 kDa), 4-Mb3441 (11 kDa), 5-Mb3412c (34 kDa), 6-EsxRST (32 kDa), 7-EsxNOQ (30 kDa), 8-EsxU (24 kDa), 9-EsxFDE (27 kDa), 10-EsxAB (21.2 kDa). Panel (B). Detection of M. bovis ORFs expressed in baculovirus infected insect cells. The lane identity and predicted molecular mass are: 1-PPE15_PE8 (66 kDa), 2-PPE20_PE15 (67 kDa), 3-PPE31_PE20 (51 kDa), 4-PPE41_PE25 (34 kDa), 5-PPE60_PE31 (53 kDa), 6-MTRA (28 kDa), 7-PPE14_PE7 (53 kDa), 8- Mb0463_Mb0674 (59 kDa), 9-Mb0854_Mb0977 (63 kDa), 10-Mb1036_Mb1129c (74 kDa), 11-Mb1272_Mb2477c (54 kDa), 12-Mb0891c (34 kDa), 13- Mb3070_Mb3641 (54 kDa), 14-Mb3338_Mb3644c (54 kDa). Open square (□) symbols indicate protein which show some breakdown as indicated by at least 2 His antibody reactive bands. Asterisk (*) indicates all Esx related proteins, most as concatenates. Diamonds (♦) indicate concatenated PPE-PE pairs. Circles (◘) other protein concatenates. M indicates the marker track, the molecular masses of which are given on the left of panel (A) in kilodaltons.
Clones positive for expression by western blot were assessed for purification by pull-down from detergent lysates using IMAC magnetic beads and the eluates assessed directly by SDS-PAGE and gel staining (Figure 3). The default outcome was a predominant band of the predicted molecular mass that also agreed with the mass identified by western blot with an anti-His antibody. The concatenated forms of the Esx proteins and many of the similarly linked PPE-PE fusions purified from the soluble fraction as single band products indicating little if any breakdown of the fusion protein at the linker junction (Figure 3B). For example, purified EsxRST and EsxU (Figure 3, panel B, lanes 7 and 8) match those blotted (Figure 2, panel A, lanes 6 and 8) and PPE-PE fusions PPE41_PE25, PPE14_PE7, PPE15_PE8 and PPE20_PE15 (Figure 3, panel B, lanes 10, 14, 16 and 17) matched the proteins identified by blot in crude lysates (Figure 2, panel B, lanes 4, 7, 1 and 2). Sequence concatenation as a viable strategy to reduce clone numbers and to produce single polypeptide versions of multimeric bTB protein complexes, therefore appears feasible, as has been shown for other proteins (reviewed in (Matsushima et al., 2008; van Rosmalen et al., 2017)). Overall, the number of recombinant proteins purified directly without any form of optimisation was ∼50% of the number of clones tested (Table 1), low expression levels or insolubility accounting for the majority of the failures.
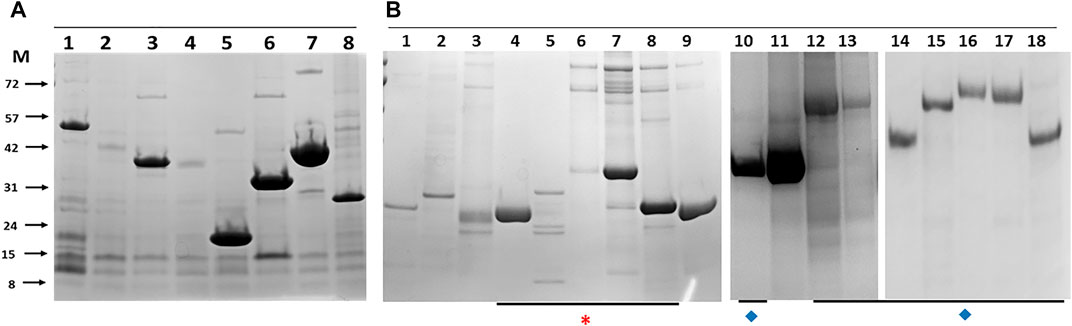
FIGURE 3. Example histidine-tagged M. bovis proteins purified by immobilised metal affinity chromatography pull down with magnetic beads from E.coli (A) and insect cells (B). The lane identity and predicted molecular masses are: Panel A, 1-Mb0704 (44 kDa), 2-Mb3070 (37 kDa), 3-Mb2054 (36 kDa), 4-Mb2002_Mb0062 (35 kDa), 5-Mb0671 (17 kDa), 6-Mb0854 (31 kDa), 7- Mb3157c_Mb3743c (37 kDa), 8-Mb0337 (26 kDa). Panel B, 1-PE5_PE32 (19.5 kDa), 2-LpqN (25 kDa), 3-LpqX (22 kDa), 4-EsxAB (21.2 kDa), 5-EsxFDE (27.3 kDa), 6-EsxNOQ (30 kDa), 7- EsxRST (32 kDa), 8-EsxU (24 kDa), 9- Mb0485 (21 kDa), 10-PPE41_PE25 (34 kDa), 11-Mb1228 (39 kDa), 12-PPE69_PE36 (46.6 kDa), 13- PPE68_PE35 (47.5 kDa), 14-PPE14_PE7 (51 kDa), 15-PPE4_PE5 (67 kDa), 16- PPE15_PE8 (66 kDa), 17- PPE20_PE15 (67 kDa), 18-PPE31_PE20 (51 kDa). Asterisk (*) indicates all Esx related proteins, most as concatenates. Diamonds (♦) indicate concatenated PPE-PE pairs. M indicates the marker track, the molecular masses of which are given on the left of panel (A) in kilodaltons.
Problematic Clones
To improve the overall recombinant bTB protein recovery rate a limited number of ORF sequences were re-cloned as N-terminal His tagged variants and purification repeated. Cell wall proteins (Mb3338 and Mb3070), conserved hypothetical (Mb0854), ribosomal protein (Mb1656), three metabolic proteins (Mb0130, Mb3652 and Mb3871), and six proteins of unknown function (Mb2898, Mb2900, Mb2656, Mb1319, Mb1228 and Mb2244) were all rescued to purified soluble proteins by this approach (Figures 4A,B). Others, such as outer membrane proteins (Mb3644, Mb1445 and Mb2653) remained unworkable. These proteins may require folding partners to be co-expressed in order to be solubilised, as was the case for the original expression trials of PPE-PE family members (Strong et al., 2006).
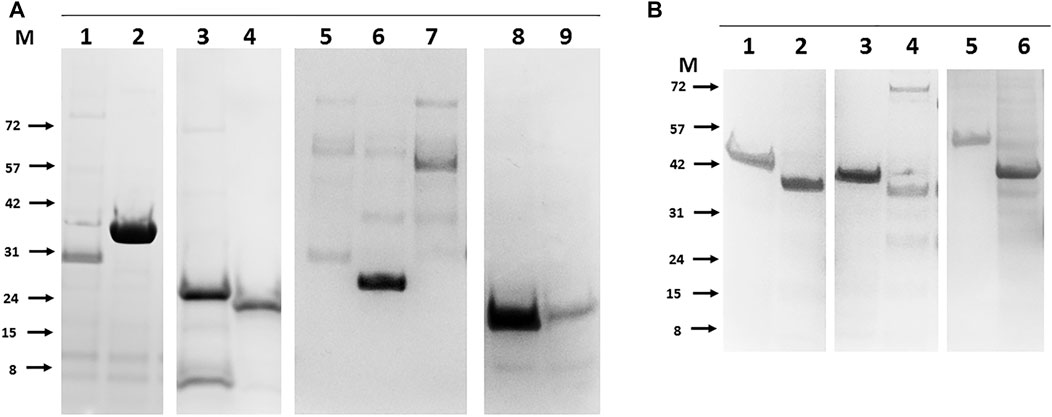
FIGURE 4. Rescue of soluble purified recombinant bTB proteins that were originally poorly expressed by alternate positioning of the His tag. Panel (A). Examples of N-terminal tagged proteins purified from E.coli. 1- Mb3338 (31 kDa), 2-Mb2656 (31 kDa), 3-Mb2898 (22 kDa), 4-Mb2900 (19 kDa), 5-Mb0130 (34 kDa), 6-Mb0854 (31 kDa), 7- Mb1656 (53 kDa), 8-Mb3652 (18 kDa), 9-Mb3871 (20 kDa). Panel (B). Examples of N-terminal tagged proteins purified from insect cells. 1- Mb0977_Mb3046c (43 kDa), 2-Mb0959 (39 kDa), 3-Mb1319 (44 kDa), 4-Mb3070 (37 kDa), 5-Mb2244 (53 kDa), 6-Mb1228 (39 kDa). Molecular weight markers (M) are labelled to the left of each panel and are in kilodaltons.
For some problematic proteins the case for their inclusion on grounds of likely candidacy as a vaccine component was such that several alternate forms of expression were assessed. EspA (Mb3646c) has been suggested to be part, with EspC, of the type VII secretion apparatus (discussed in (Ates and Brosch, 2017)) and an obvious candidate for inclusion (Chiliza et al., 2017). Recombinant EspC has been described (Lou et al., 2017; Salemi et al., 2020) and used diagnostically (Yan et al., 2018; Srinivasan et al., 2019; Villar-Hernández et al., 2020), but EspA has proven more difficult (Garces et al., 2010). EspA expression as either a C- or N-terminally His tagged protein was not successful and mutation of the single cysteine reported to drive dimer formation (Garces et al., 2010) had no effect. Fusion of EspA with EspC at either the N- or C- termini via a Gly-Ser linker did not improve expression but, of a number of enhancement tags investigated (GFP, SUMO and GB1), fusion of GFP to the N-terminus rescued detectable expression at the predicted molecular mass in both E. coli and insect cells and enabled modest levels to be purified (Figure 5A). Similarly, adding GB1 as an N- terminal fusion tag rescued expression of outer membrane protein Mb1762 and two unknown proteins, Mb1916 and Mb2410, allowing purification to reasonable levels, albeit with contaminating host derived proteins (Figure 5B). To assess if problematic expression was the result of any common feature in the proteins concerned, we inspected the AlphaFold predicted structures (Jumper et al., 2021) of the full length problematic proteins (Jumper et al., 2021) (Table 2). Of the 20 candidates analysed, only 4, Mb2244, Mb2656, Mb3652 and Mb3871 had available full length structures but in each case the proteins concerned were multimeric, some to a very high degree (Mb3871 is a 24mer), which may have limited expression here. Five proteins, Mb1656, Mb1916c, Mb2410, Mb2898 and Mb2900 had partial structures obtained with protein fragments suggesting that full length protein expression was not possible, and a further 5, Mb0130, Mb1228, Mb1319, Mb3070 and Mb3338, had fragment structures predicted on the basis of homology with other proteins whose structures have been solved. Of these 10 proteins, AlphaFold predictions suggested unstructured termini, predominantly at the N-terminus, as a likely limitation to stable protein expression. Unstructured termini, also with a predominance of unstructured N-termini, were associated with the 6 proteins (Mb0854, Mb1445, Mb1762, Mb2653, Mb3644 and Mb3646c) with no available structure, only 3 of which were recoverable here. Submission of the 20 candidate to the FuzDrop server (Hatos et al., 2022) showed a high score for likely aggregation and liquid-liquid phase separation associated with about half of the proteins for which there was either no structure or only a partial structure available (Table 2, indicated). Extensive protein engineering to reduce these scores might be necessary if these proteins were to be produced at a scale and purity required for a vaccine candidate.
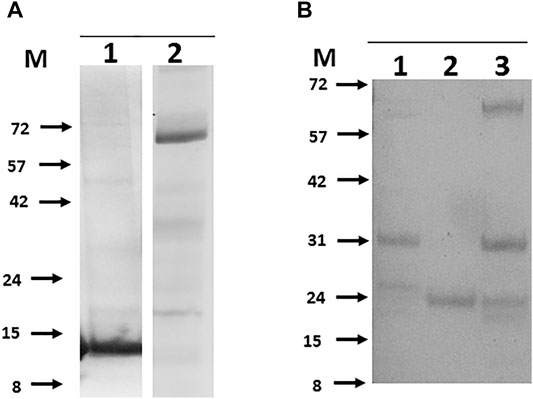
FIGURE 5. Rescued expression of problematic bTB proteins in E.coli by non-His fusion tags. Panel (A). The secretion accessory proteins EspC and EspA. 1- C-terminally His tagged EspC, 2- N-terminally GFP tagged EspA. Panel (B). 1-GB_Mb1762 (29 kDa), 2-GB_Mb1916 (25 kDa), 3-GB_Mb2410 (22 kDa) all expressed and purified as N-terminal fusions with the B1 domain of streptococcal protein G. Molecular weight markers (M) are labelled to left of each panel and are in kilodaltons.
Discussion
Bovine tuberculosis (bTB) is a significant risk to animal health and welfare with estimates of >50 million cattle infected worldwide (Waters et al., 2012). Vaccination with M. bovis BCG, which is safe and inexpensive, shows varying efficacies (Francis, 1948; Berggren, 1981; Rodrigues and Smith, 1990) but can reduce spread in cattle indicating that control via vaccination is feasible (Hope et al., 2005; Lopez-Valencia et al., 2010; Waters et al., 2012). However, as up to 80% of BCG-vaccinated cattle may react positively to the tuberculin skin test at 6 months post-vaccination (Whelan et al., 2011), alternate vaccines including new attenuated mycobacterial vaccines (Khare et al., 2007; Waters et al., 2007; Blanco et al., 2013), DNA vaccines (Maue et al., 2004; Cai et al., 2005; Skinner et al., 2005; Maue et al., 2007) and virus-vectored vaccines (Vordermeier et al., 2009; Dean et al., 2014) have been investigated. The reverse vaccinology concept has provided a new serogroup B Neisseria meningitidis vaccine, Bexsero (Masignani et al., 2019) and has been used to select vaccine candidates for Staphylococcus aureus (Oprea and Antohe, 2013), Campylobacter jejuni (Muruato et al., 2017) and Streptococcus pneumonia (Argondizzo et al., 2015) in addition to Mycobacterium tuberculosis (Monterrubio-López et al., 2015). To test the feasibility for this approach we cloned and expressed >120 ORFs encoded by the reference M. bovis AF2122/97 genome and purified more than half of them using a combination of prokaryotic and eukaryotic expression. The list includes Esx protein family members, the PE and PPE families, a number of lipoproteins and others suggested to be potential vaccine candidates, including all of the targets predicted by the VaxiJen package (Monterrubio-López et al., 2015). A useful approach to reduce the number of clones required was to concatenate shorter ORFs or family partners together via flexible linkers. The successful expression and purification of many PPE-PE complexes (PPE4-PE5, PPE68-PE35, PPE69-PE36, PPE65-PE32, PPE41-PE25, PPE17-PE11, PPE15-PE8, PPE14-PE7, PPE31-PE20, PPE20-PE15 and PPE60-PE31) linked in this way was notable and was consistent with the PPE and PE partners folding to form a stable heterodimer (Strong et al., 2006). Many current TB vaccine candidates are fusions of several preselected ORFs (reviewed in (Dockrell, 2016)) and our data suggest that PPE-PE complexes, which have been noted as potential vaccine candidates (Stylianou et al., 2018), may be suitable additions. Currently, those candidate TB vaccines that contain PPE sequences, M72 and ID93, encode only fragments (Brennan, 2017). We did not investigate post-translational modification of any expressed protein as some of these are reportedly Mycobacteria specific (van Els et al., 2014) and may not occur in either of the expression hosts used although we noted no particular association with expression outcome. For example the heparin binding hemagglutinin (HBHA), which is methylated and toxic at high levels in Mycobacteria (Delogu et al., 2004), was well expression and purified from both E. coli and insect cells. We noted some trends in the outcome of expression, e.g. recovery of soluble rpf-like proteins (Kana et al., 2008; Mukamolova et al., 2010) was successful from the baculovirus system but not from E. coli where they were insoluble, and the combination of these two systems was beneficial overall, providing a success rate for the recovery of purified soluble protein of 67%, slightly higher than the 61% success rate reported during the development of Bexsero (Serruto et al., 2012; Masignani et al., 2019) and considerably higher than the ∼33% success rate previously reported for Mycobacterial protein expression using solely E. coli (Bashiri and Baker, 2015). An AlphaFold analysis of the proteins where re-cloning with N-terminal tags benefited expression or purification revealed that unstructured termini were a common feature. A tendency to aggregation was also noted by LLPS predication software (Hatos et al., 2022) with the regions identified located in the unstructured regions. Plausibly, the improvements in expression and recoverability for some the targets that were achieved by appending an N-terminal tag stabilised an otherwise disordered structure, reducing aggregation or degradation. A GFP fusion to the sequence encoding full length EspA, where the C-terminal 100 aa of the 319 aa EspA protein sequence has no predictable structure, uniquely allowed expression and purification of this vaccine candidate. A revised HTP scheme (Figure 1) might include an AlphaFold and FuzDrop screen for disorder prior to the selection of the endpoints for translation. That some proteins scored highly for LLPS is interesting given that such regions are associated with stress and lipid homeostasis (Vendruscolo, 2022), both of which are hallmarks of TB infection (Chow and Cox, 2011). Notwithstanding these considerations, our data suggest that the production of a large library of full length bTB proteins for candidate vaccine use is feasible. Recently the protection afforded by intravenous vaccination with BCG was shown to be correlate with the IgM component of the antibody response, although no antigen specificity was reported (Divangahi et al., 2021; Irvine et al., 2021). In addition, a monoclonal antibody to a single protein, PstS1 (Mb0959 in Table 1, purified in Figure 4B), was inhibitory in a whole blood Mtb growth inhibition assay (Watson et al., 2021). Antibodies from animals immunised with proteins from the range we describe here might be used to identify other targets relevant for vaccine development against Bovine TB or the related Mycobacterium tuberculosis and Mycobacterium leprae.
Data Availability Statement
The original contributions presented in the study are included in the article/Supplementary Material, further inquiries can be directed to the corresponding author.
Author Contributions
DP, MT, DR, AH, and AW did the experimental work. BC and IJ obtained the funding, supervised the work and wrote the first draft. All authors contributed to the final manuscript.
Funding
Funding for this research was from the BBSRC grant number BB/N004698/1.
Conflict of Interest
The authors declare that the research was conducted in the absence of any commercial or financial relationships that could be construed as a potential conflict of interest.
Publisher’s Note
All claims expressed in this article are solely those of the authors and do not necessarily represent those of their affiliated organizations, or those of the publisher, the editors and the reviewers. Any product that may be evaluated in this article, or claim that may be made by its manufacturer, is not guaranteed or endorsed by the publisher.
Acknowledgments
We thank Helen McShane, Elena Stylianou, Rachel Tanner, Martin Vordermeier and Bernardo Villarreal-Ramos for constructive comments. The work was funded by the Biotechnology and Biological Sciences Research Council.
References
Andersen, K. R., Leksa, N. C., and Schwartz, T. U. (2013). OptimizedE. Coliexpression Strain LOBSTR Eliminates Common Contaminants from His˗tag Purification. Proteins 81, 1857–1861. doi:10.1002/prot.24364
Argondizzo, A. P. C., da Mota, F. F., Pestana, C. P., Reis, J. N., de Miranda, A. B., Galler, R., et al. (2015). Identification of Proteins in Streptococcus Pneumoniae by Reverse Vaccinology and Genetic Diversity of These Proteins in Clinical Isolates. Appl. Biochem. Biotechnol. 175, 2124–2165. doi:10.1007/s12010-014-1375-3
Aricescu, A. R., Assenberg, R., Bill, R. M., Busso, D., Chang, V. T., Davis, S. J., et al. (2006). Eukaryotic Expression: Developments for Structural Proteomics. Acta Crystallogr. D. Biol. Cryst. 62, 1114–1124. doi:10.1107/s0907444906029805
Ates, L. S., and Brosch, R. (2017). Discovery of the Type VII ESX-1 Secretion Needle? Mol. Microbiol. 103, 7–12. doi:10.1111/mmi.13579
Bashiri, G., and Baker, E. N. (2015). Production of Recombinant Proteins inMycobacterium Smegmatisfor Structural and Functional Studies. Protein Sci. 24, 1–10. doi:10.1002/pro.2584
Berggren, S. A. (1981). Field Experiment with BCG Vaccine in Malawi. Br. Veterinary J. 137, 88–94. doi:10.1016/s0007-1935(17)31792-x
Bielby, J., Donnelly, C. A., Pope, L. C., Burke, T., and Woodroffe, R. (2014). Badger Responses to Small-Scale Culling May Compromise Targeted Control of Bovine Tuberculosis. Proc. Natl. Acad. Sci. U.S.A. 111, 9193–9198. doi:10.1073/pnas.1401503111
Bird, L. E., Rada, H., Flanagan, J., Diprose, J. M., Gilbert, R. J. C., and Owens, R. J. (2014). Application of In-Fusion Cloning for the Parallel Construction of E. coli Expression Vectors. Methods Mol. Biol. 1116, 209–234. doi:10.1007/978-1-62703-764-8_15
Blanco, F. C., Bianco, M. V., Garbaccio, S., Meikle, V., Gravisaco, M. J., Montenegro, V., et al. (2013). Mycobacterium bovis Δmce2 Double Deletion Mutant Protects Cattle against Challenge with Virulent M. Bovis. Tuberculosis 93, 363–372. doi:10.1016/j.tube.2013.02.004
Brennan, M. J. (2017). The Enigmatic PE/PPE Multigene Family of Mycobacteria and Tuberculosis Vaccination. Infect. Immun. 85. doi:10.1128/IAI.00969-16
Cai, H., Tian, X., Hu, X. D., Li, S. X., Yu, D. H., and Zhu, Y. X. (2005). Combined DNA Vaccines Formulated Either in DDA or in Saline Protect Cattle from Mycobacterium bovis Infection. Vaccine 23, 3887–3895. doi:10.1016/j.vaccine.2005.03.025
Chambers, M. A., Carter, S. P., Wilson, G. J., Jones, G., Brown, E., Hewinson, R. G., et al. (2014). Vaccination against Tuberculosis in Badgers and Cattle: an Overview of the Challenges, Developments and Current Research Priorities in Great Britain. Veterinary Rec. 175, 90–96. doi:10.1136/vr.102581
Chandran, A., Williams, K., Mendum, T., Stewart, G., Clark, S., Zadi, S., et al. (2019). Development of a Diagnostic Compatible BCG Vaccine against Bovine Tuberculosis. Sci. Rep. 9, 17791. doi:10.1038/s41598-019-54108-y
Chiliza, T. E., Pillay, M., and Pillay, B. (2017). Identification of Unique Essential Proteins from a Mycobacterium tuberculosis F15/LAM4/KZN Phage Secretome Library. Pathog. Dis. 75. doi:10.1093/femspd/ftx001
Chim, N., Habel, J. E., Johnston, J. M., Krieger, I., Miallau, L., Sankaranarayanan, R., et al. (2011). The TB Structural Genomics Consortium: a Decade of Progress. Tuberculosis 91, 155–172. doi:10.1016/j.tube.2010.11.009
Chow, E. D., and Cox, J. S. (2011). TB Lipidomics-The Final Frontier. Chem. Biol. 18, 1517–1518. doi:10.1016/j.chembiol.2011.12.003
Dai, F. Y., Wang, J. F., Gong, X. L., and Bao, L. (2017). Immunogenicity and Protective Efficacy of Recombinant Bacille Calmette-Guerin Strains Expressing mycobacterium Antigens Ag85A, CFP10, ESAT-6, GM-CSF and IL-12p70. Hum. Vaccin Immunother. 13, 1–8. doi:10.1080/21645515.2017.1279771
Dean, G., Whelan, A., Clifford, D., Salguero, F. J., Xing, Z., Gilbert, S., et al. (2014). Comparison of the Immunogenicity and Protection against Bovine Tuberculosis Following Immunization by BCG-Priming and Boosting with Adenovirus or Protein Based Vaccines. Vaccine 32, 1304–1310. doi:10.1016/j.vaccine.2013.11.045
Delogu, G., Bua, A., Pusceddu, C., Parra, M., Fadda, G., Brennan, M. J., et al. (2004). Expression and Purification of Recombinant Methylated HBHA inMycobacterium Smegmatis. FEMS Microbiol. Lett. 239, 33–39. doi:10.1016/j.femsle.2004.08.015
Dhanda, S. K., Vir, P., Singla, D., Gupta, S., Kumar, S., and Raghava, G. P. S. (2016). A Web-Based Platform for Designing Vaccines against Existing and Emerging Strains of Mycobacterium tuberculosis. PloS one 11, e0153771. doi:10.1371/journal.pone.0153771
Divangahi, M., Javid, B., and Kaufmann, E. (2021). 100 Years of Antibody Solitude in TB. Nat. Immunol. 22 (2021), 1470–1471. doi:10.1038/s41590-021-01071-4
Dockrell, H. M. (2016). Towards New TB Vaccines: What Are the Challenges? Pathogens Dis. 74, ftw016. doi:10.1093/femspd/ftw016
Fletcher, H. A., and Schrager, L. (2016). TB Vaccine Development and the End TB Strategy: Importance and Current Status. Trans. R. Soc. Trop. Med. Hyg. 110, 212–218. doi:10.1093/trstmh/trw016
Francis, J. (1948). Bovine Tuberculosis Including a Contrast with Human Tuberculosis. Indian Med. Gazette 83, 250–251.
Garces, A., Atmakuri, K., Chase, M. R., Woodworth, J. S., Krastins, B., Rothchild, A. C., et al. (2010). EspA Acts as a Critical Mediator of ESX1-dependent Virulence in Mycobacterium tuberculosis by Affecting Bacterial Cell Wall Integrity. PLoS Pathog. 6, e1000957. doi:10.1371/journal.ppat.1000957
Gibson, D. G., Young, L., Chuang, R.-Y., Venter, J. C., Hutchison, C. A., and Smith, H. O. (2009). Enzymatic Assembly of DNA Molecules up to Several Hundred Kilobases. Nat. Methods 6, 343–345. doi:10.1038/nmeth.1318
Hashimoto, Y., Zhang, S., Zhang, S., Chen, Y.-R., and Blissard, G. W. (2012). Correction: BTI-Tnao38, a New Cell Line Derived from Trichoplusia Ni, Is Permissive for AcMNPV Infection and Produces High Levels of Recombinant Proteins. BMC Biotechnol. 12, 12. doi:10.1186/1472-6750-12-12
Hatos, A., Tosatto, S. C. E., Vendruscolo, M., and Fuxreiter, M. (2022). FuzDrop on AlphaFold: Visualizing the Sequence-dependent Propensity of Liquid-Liquid Phase Separation and Aggregation of Proteins. Nucleic acids Res. 2022, gkac386. doi:10.1093/nar/gkac386
He, Y., Xiang, Z., and Mobley, H. L. (2010). Vaxign: the First Web-Based Vaccine Design Program for Reverse Vaccinology and Applications for Vaccine Development. J. Biomed. Biotechnol. 2010, 297505. doi:10.1155/2010/297505
Hope, J. C., Thom, M. L., Villarreal-Ramos, B., Vordermeier, H. M., Hewinson, R. G., and Howard, C. J. (2005). Exposure to Mycobacterium avium Induces Low-Level Protection from Mycobacterium bovis Infection but Compromises Diagnosis of Disease in Cattle. Clin. Exp. Immunol. 141, 432–439. doi:10.1111/j.1365-2249.2005.02882.x
Irvine, E. B., O’Neil, A., Darrah, P. A., Shin, S., Choudhary, A., Li, W., et al. (2021). Robust IgM Responses Following Intravenous Vaccination with Bacille Calmette-Guérin Associate with Prevention of Mycobacterium tuberculosis Infection in Macaques. Nat. Immunol. 22, 1515–1523. doi:10.1038/s41590-021-01066-1
Jumper, J., Evans, R., Pritzel, A., Green, T., Figurnov, M., Ronneberger, O., et al. (2021). Highly Accurate Protein Structure Prediction with AlphaFold. Nature 596, 583–589. doi:10.1038/s41586-021-03819-2
Kana, B. D., Gordhan, B. G., Downing, K. J., Sung, N., Vostroktunova, G., Machowski, E. E., et al. (2008). The Resuscitation-Promoting Factors ofMycobacterium Tuberculosisare Required for Virulence and Resuscitation from Dormancy but Are Collectively Dispensable for Growthin Vitro. Mol. Microbiol. 67, 672–684. doi:10.1111/j.1365-2958.2007.06078.x
Kapopoulou, A., Lew, J. M., and Cole, S. T. (2011). The MycoBrowser Portal: a Comprehensive and Manually Annotated Resource for Mycobacterial Genomes. Tuberculosis 91, 8–13. doi:10.1016/j.tube.2010.09.006
Kaufmann, S. H. E., Weiner, J., and von Reyn, C. F. (2017). Novel Approaches to Tuberculosis Vaccine Development. Int. J. Infect. Dis. 56, 263–267. doi:10.1016/j.ijid.2016.10.018
Khare, S., Hondalus, M. K., Nunes, J., Bloom, B. R., and Garry Adams, L. (2007). Mycobacterium bovis ΔleuD Auxotroph-Induced Protective Immunity against Tissue Colonization, Burden and Distribution in Cattle Intranasally Challenged with Mycobacterium bovis Ravenel S. Vaccine 25, 1743–1755. doi:10.1016/j.vaccine.2006.11.036
Kim, H.-W., Lee, S.-Y., Park, H., and Jeon, S.-J. (2015). Expression, Refolding, and Characterization of a Small Laccase from Thermus Thermophilus HJ6. Protein Expr. Purif. 114, 37–43. doi:10.1016/j.pep.2015.06.004
Lee, C.-D., Sun, H.-C., Hu, S.-M., Chiu, C.-F., Homhuan, A., Liang, S.-M., et al. (2008). An Improved SUMO Fusion Protein System for Effective Production of Native Proteins. Protein Sci. 17, 1241–1248. doi:10.1110/ps.035188.108
Li, F., Xu, M., Qin, C., Xia, L., Xiong, Y., Xi, X., et al. (2016). Recombinant Fusion ESAT6-CFP10 Immunogen as a Skin Test Reagent for Tuberculosis Diagnosis: an Open-Label, Randomized, Two-Centre Phase 2a Clinical Trial. Clin. Microbiol. Infect. 22, 889–e16. e16. doi:10.1016/j.cmi.2016.07.015
Li, W., Li, M., Deng, G., Zhao, L., Liu, X., and Wang, Y. (2015). Prime-boost Vaccination with Bacillus Calmette Guerin and a Recombinant Adenovirus Co-expressing CFP10, ESAT6, Ag85A and Ag85B of Mycobacterium tuberculosis Induces Robust Antigen-specific Immune Responses in Mice. Mol. Med. Rep. 12, 3073–3080. doi:10.3892/mmr.2015.3770
Lindhout, D. A., Thiessen, A., Schieve, D., and Sykes, B. D. (2003). High-yield Expression of Isotopically Labeled Peptides for Use in NMR Studies. Protein Sci. 12, 1786–1791. doi:10.1110/ps.0376003
Lopez-Valencia, G., Renteria-Evangelista, T., Williams, J. d. J., Licea-Navarro, A., Mora-Valle, A. D. l., and Medina-Basulto, G. (2010). Field Evaluation of the Protective Efficacy of Mycobacterium bovis BCG Vaccine against Bovine Tuberculosis. Res. veterinary Sci. 88, 44–49. doi:10.1016/j.rvsc.2009.05.022
Lou, Y., Rybniker, J., Sala, C., and Cole, S. T. (2017). EspC Forms a Filamentous Structure in the Cell Envelope ofMycobacterium Tuberculosisand Impacts ESX-1 Secretion. Mol. Microbiol. 103, 26–38. doi:10.1111/mmi.13575
Masignani, V., Pizza, M., and Moxon, E. R. (2019). The Development of a Vaccine against Meningococcus B Using Reverse Vaccinology. Front. Immunol. 10, 751. doi:10.3389/fimmu.2019.00751
Matsushima, N., Yoshida, H., Kumaki, Y., Kamiya, M., Tanaka, T., Izumi, Y., et al. (2008). Flexible Structures and Ligand Interactions of Tandem Repeats Consisting of Proline, glycine, Asparagine, Serine, And/or Threonine Rich Oligopeptides in Proteins. Cpps 9, 591–610. doi:10.2174/138920308786733886
Maue, A. C., Waters, W. R., Palmer, M. V., Nonnecke, B. J., Minion, F. C., Brown, W. C., et al. (2007). An ESAT-6:CFP10 DNA Vaccine Administered in Conjunction with Mycobacterium bovis BCG Confers Protection to Cattle Challenged with Virulent M. Bovis. Vaccine 25, 4735–4746. doi:10.1016/j.vaccine.2007.03.052
Maue, A. C., Waters, W. R., Palmer, M. V., Whipple, D. L., Minion, F. C., Brown, W. C., et al. (2004). CD80 and CD86, but Not CD154, Augment DNA Vaccine-Induced Protection in Experimental Bovine Tuberculosis. Vaccine 23, 769–779. doi:10.1016/j.vaccine.2004.07.019
McShane, H. (2019). Insights and Challenges in Tuberculosis Vaccine Development. Lancet Respir. Med. 7, 810–819. doi:10.1016/s2213-2600(19)30274-7
Mearns, H., Geldenhuys, H. D., Kagina, B. M., Musvosvi, M., Little, F., Ratangee, F., et al. (2017). H1:IC31 Vaccination Is Safe and Induces Long-Lived TNF-α+IL-2+CD4 T Cell Responses in M. tuberculosis Infected and Uninfected Adolescents: A Randomized Trial. Vaccine 35, 132–141. doi:10.1016/j.vaccine.2016.11.023
Monterrubio-López, G. P., González-Y-Merchand, J. A., and Ribas-Aparicio, R. M. (2015). Identification of Novel Potential Vaccine Candidates against Tuberculosis Based on Reverse Vaccinology. Biomed. Res. Int. 2015, 483150. doi:10.1155/2015/483150
Mukamolova, G. V., Turapov, O., Malkin, J., Woltmann, G., and Barer, M. R. (2010). Resuscitation-promoting Factors Reveal an Occult Population of Tubercle Bacilli in Sputum. Am. J. Respir. Crit. Care Med. 181, 174–180. doi:10.1164/rccm.200905-0661oc
Muruato, L. A., Tapia, D., Hatcher, C. L., Kalita, M., Brett, P. J., Gregory, A. E., et al. (2017). Use of Reverse Vaccinology in the Design and Construction of Nanoglycoconjugate Vaccines against Burkholderia Pseudomallei. Clin. Vaccine Immunol. 24, 1–13. doi:10.1128/CVI.00206-17
Nettleship, J. E., Assenberg, R., Diprose, J. M., Rahman-Huq, N., and Owens, R. J. (2010). Recent Advances in the Production of Proteins in Insect and Mammalian Cells for Structural Biology. J. Struct. Biol. 172, 55–65. doi:10.1016/j.jsb.2010.02.006
Nielsen, H., Tsirigos, K. D., Brunak, S., and von Heijne, G. (2019). A Brief History of Protein Sorting Prediction. Protein J. 38, 200–216. doi:10.1007/s10930-019-09838-3
Oprea, M., and Antohe, F. (2013). Reverse-vaccinology Strategy for Designing T-Cell Epitope Candidates for Staphylococcus aureus Endocarditis Vaccine. Biologicals 41, 148–153. doi:10.1016/j.biologicals.2013.03.001
Pengelley, S. C., Chapman, D. C., Mark Abbott, W., Lin, H.-H., Huang, W., Dalton, K., et al. (2006). A Suite of Parallel Vectors for Baculovirus Expression. Protein Expr. Purif. 48, 173–181. doi:10.1016/j.pep.2006.04.016
Porta, C., Kotecha, A., Burman, A., Jackson, T., Ren, J., Loureiro, S., et al. (2013). Rational Engineering of Recombinant Picornavirus Capsids to Produce Safe, Protective Vaccine Antigen. PLoS Pathog. 9, e1003255. doi:10.1371/journal.ppat.1003255
Rappuoli, R., Bottomley, M. J., D’Oro, U., Finco, O., and De Gregorio, E. (2016). Reverse Vaccinology 2.0: Human Immunology Instructs Vaccine Antigen Design. J. Exp. Med. 213, 469–481. doi:10.1084/jem.20151960
Rappuoli, R. (2001). Reverse Vaccinology, a Genome-Based Approach to Vaccine Development. Vaccine 19, 2688–2691. doi:10.1016/s0264-410x(00)00554-5
Rodrigues, L. C., and Smith, P. G. (1990). Tuberculosis in Developing Countries and Methods for its Control. Trans. R. Soc. Trop. Med. Hyg. 84, 739–744. doi:10.1016/0035-9203(90)90172-b
Salemi, O., Noormohammadi, Z., Bahrami, F., Siadat, S. D., and Ajdary, S. (2020). Cloning, Expression and Purification of Espc, Espb and Espc/Espb Proteins of Mycobacterium tuberculosis ESX-1 Secretion System. Rep. Biochem. Mol. Biol. 8, 465–472.
Schlager, B., Straessle, A., and Hafen, E. (2012). Use of Anionic Denaturing Detergents to Purify Insoluble Proteins after Overexpression. BMC Biotechnol. 12, 95. doi:10.1186/1472-6750-12-95
Serruto, D., Bottomley, M. J., Ram, S., Giuliani, M. M., and Rappuoli, R. (2012). The New Multicomponent Vaccine against Meningococcal Serogroup B, 4CMenB: Immunological, Functional and Structural Characterization of the Antigens. Vaccine 30 (2), B87–B97. doi:10.1016/j.vaccine.2012.01.033
Skinner, M. A., Wedlock, D. N., de Lisle, G. W., Cooke, M. M., Tascon, R. E., Ferraz, J. C., et al. (2005). The Order of Prime-Boost Vaccination of Neonatal Calves with Mycobacterium bovis BCG and a DNA Vaccine Encoding Mycobacterial Proteins Hsp65, Hsp70, and Apa Is Not Critical for Enhancing Protection against Bovine Tuberculosis. Infect. Immun. 73, 4441–4444. doi:10.1128/iai.73.7.4441-4444.2005
Smith, N. H. (2012). The Global Distribution and Phylogeography of Mycobacterium bovis Clonal Complexes. Infect. Genet. Evol. J. Mol. Epidemiol. Evol. Genet. Infect. Dis. 12, 857–865. doi:10.1016/j.meegid.2011.09.007
Srinivasan, S., Jones, G., Veerasami, M., Steinbach, S., Holder, T., Zewude, A., et al. (2019). A Defined Antigen Skin Test for the Diagnosis of Bovine Tuberculosis. Sci. Adv. 5, eaax4899. doi:10.1126/sciadv.aax4899
Strong, M., Sawaya, M. R., Wang, S., Phillips, M., Cascio, D., and Eisenberg, D. (2006). Toward the Structural Genomics of Complexes: Crystal Structure of a PE/PPE Protein Complex from Mycobacterium tuberculosis. Proc. Natl. Acad. Sci. U.S.A. 103, 8060–8065. doi:10.1073/pnas.0602606103
Stylianou, E., Harrington-Kandt, R., Beglov, J., Bull, N., Pinpathomrat, N., Swarbrick, G. M., et al. (2018). Identification and Evaluation of Novel Protective Antigens for the Development of a Candidate Tuberculosis Subunit Vaccine. Infect. Immun. 86. doi:10.1128/IAI.00014-18
Tessier, D. C., Thomas, D. Y., Khouri, H. E., Laliberié, F., and Vernet, T. (1991). Enhanced Secretion from Insect Cells of a Foreign Protein Fused to the Honeybee Melittin Signal Peptide. Gene 98, 177–183. doi:10.1016/0378-1119(91)90171-7
Tkachuk, A. P., Gushchin, V. A., Potapov, V. D., Demidenko, A. V., Lunin, V. G., and Gintsburg, A. L. (2017). Multi-subunit BCG Booster Vaccine GamTBvac: Assessment of Immunogenicity and Protective Efficacy in Murine and guinea Pig TB Models. PloS one 12, e0176784. doi:10.1371/journal.pone.0176784
van Els, C. c. A. C. M., Corbière, V. r., Smits, K., van Gaans-van den Brink, J. A. M., Poelen, M. C. M., Mascart, F., et al. (2014). Toward Understanding the Essence of Post-Translational Modifications for the Mycobacterium tuberculosis Immunoproteome. Front. Immunol. 5, 361. doi:10.3389/fimmu.2014.00361
van Rosmalen, M., Krom, M., and Merkx, M. (2017). Tuning the Flexibility of Glycine-Serine Linkers to Allow Rational Design of Multidomain Proteins. Biochemistry 56, 6565–6574. doi:10.1021/acs.biochem.7b00902
Vendruscolo, M. (2022). Lipid Homeostasis and its Links with Protein Misfolding Diseases. Front. Mol. Neurosci. 15, 829291. doi:10.3389/fnmol.2022.829291
Villar-Hernández, R., Blauenfeldt, T., García-García, E., Muriel-Moreno, B., De Souza-Galvão, M. L., Millet, J. P., et al. (2020). Diagnostic Benefits of Adding EspC, EspF and Rv2348-B to the QuantiFERON Gold In-Tube Antigen Combination. Sci. Rep. 10, 13234. doi:10.1038/s41598-020-70204-w
Vordermeier, M., Gordon, S. V., and Hewinson, A. R. G. (2009). Antigen Mining to Define Mycobacterium bovis Antigens for the Differential Diagnosis of Vaccinated and Infected Animals: A VLA Perspective. Transbound. Emerg. Dis. 56, 240–247. doi:10.1111/j.1865-1682.2009.01070.x
Vordermeier, M., Gordon, S. V., and Hewinson, R. G. (2011). Mycobacterium bovis Antigens for the Differential Diagnosis of Vaccinated and Infected Cattle. Veterinary Microbiol. 151, 8–13. doi:10.1016/j.vetmic.2011.02.020
Vordermeier, M., and Hewinson, R. G. (2006). Development of Cattle TB Vaccines in the UK. Veterinary Immunol. Immunopathol. 112, 38–48. doi:10.1016/j.vetimm.2006.03.010
Waters, W. R., Palmer, M. V., Buddle, B. M., and Vordermeier, H. M. (2012). Bovine Tuberculosis Vaccine Research: Historical Perspectives and Recent Advances. Vaccine 30, 2611–2622. doi:10.1016/j.vaccine.2012.02.018
Waters, W. R., Palmer, M. V., Nonnecke, B. J., Thacker, T. C., Scherer, C. F. C., Estes, D. M., et al. (2007). Failure of a Mycobacterium tuberculosis ΔRD1 ΔpanCD Double Deletion Mutant in a Neonatal Calf Aerosol M. Bovis Challenge Model: Comparisons to Responses Elicited by M. Bovis Bacille Calmette Guerin. Vaccine 25, 7832–7840. doi:10.1016/j.vaccine.2007.08.029
Watson, A., Li, H., Ma, B., Weiss, R., Bendayan, D., Abramovitz, L., et al. (2021). Human Antibodies Targeting a Mycobacterium Transporter Protein Mediate Protection against Tuberculosis. Nat. Commun. 12, 602. doi:10.1038/s41467-021-20930-0
Whelan, A. O., Clifford, D., Upadhyay, B., Breadon, E. L., McNair, J., Hewinson, G. R., et al. (2010). Development of a Skin Test for Bovine Tuberculosis for Differentiating Infected from Vaccinated Animals. J. Clin. Microbiol. 48, 3176–3181. doi:10.1128/jcm.00420-10
Whelan, A. O., Coad, M., Upadhyay, B. L., Clifford, D. J., Hewinson, R. G., and Vordermeier, H. M. (2011). Lack of Correlation between BCG-Induced Tuberculin Skin Test Sensitisation and Protective Immunity in Cattle. Vaccine 29, 5453–5458. doi:10.1016/j.vaccine.2011.05.057
Yan, Z. H., Yi, L., Wei, P. J., Jia, H. Y., Wang, J., Wang, X. J., et al. (2018). Evaluation of Panels of Mycobacterium tuberculosis Antigens for Serodiagnosis of Tuberculosis. Int. J. Tuberc. Lung Dis. 22, 959–965. doi:10.5588/ijtld.18.0060
Zhang, W., Zhang, Y., Zheng, H., Pan, Y., Liu, H., Du, P., et al. (2013). Genome Sequencing and Analysis of BCG Vaccine Strains. PloS one 8, e71243. doi:10.1371/journal.pone.0071243
Keywords: bovine tuberculosis, Mycobacterium bovis, genome, open reading frame, vaccine, expression, protein purification, PPE
Citation: Paliwal D, Thom M, Hussein A, Ravishankar D, Wilkes A, Charleston B and Jones IM (2022) Towards Reverse Vaccinology for Bovine TB: High Throughput Expression of Full Length Recombinant Mycobacterium bovis Proteins. Front. Mol. Biosci. 9:889667. doi: 10.3389/fmolb.2022.889667
Received: 04 March 2022; Accepted: 06 June 2022;
Published: 11 August 2022.
Edited by:
Nikolaos E. Labrou, Agricultural University of Athens, GreeceReviewed by:
José Ángel Gutiérrez-Pabello, National Autonomous University of Mexico, MexicoHengyao Niu, Indiana University Bloomington, United States
Copyright © 2022 Paliwal, Thom, Hussein, Ravishankar, Wilkes, Charleston and Jones. This is an open-access article distributed under the terms of the Creative Commons Attribution License (CC BY). The use, distribution or reproduction in other forums is permitted, provided the original author(s) and the copyright owner(s) are credited and that the original publication in this journal is cited, in accordance with accepted academic practice. No use, distribution or reproduction is permitted which does not comply with these terms.
*Correspondence: Ian M. Jones, aS5tLmpvbmVzQHJlYWRpbmcuYWMudWs=