- 1Department of Biochemistry, Stellenbosch University, Stellenbosch, South Africa
- 2Department of Biochemistry, Midlands State University, Gweru, Zimbabwe
- 3Department of Biochemistry and Microbiology, University of Venda, Thohoyandou, South Africa
The coronavirus disease (COVID-19) caused by a coronavirus identified in December 2019 has caused a global pandemic. COVID-19 was declared a pandemic in March 2020 and has led to more than 6.3 million deaths. The pandemic has disrupted world travel, economies, and lifestyles worldwide. Although vaccination has been an effective tool to reduce the severity and spread of the disease there is a need for more concerted approaches to fighting the disease. COVID-19 is characterised as a severe acute respiratory syndrome . The severity of the disease is associated with a battery of comorbidities such as cardiovascular diseases, cancer, chronic lung disease, and renal disease. These underlying diseases are associated with general cellular stress. Thus, COVID-19 exacerbates outcomes of the underlying conditions. Consequently, coronavirus infection and the various underlying conditions converge to present a combined strain on the cellular response. While the host response to the stress is primarily intended to be of benefit, the outcomes are occasionally unpredictable because the cellular stress response is a function of complex factors. This review discusses the role of the host stress response as a convergent point for COVID-19 and several non-communicable diseases. We further discuss the merits of targeting the host stress response to manage the clinical outcomes of COVID-19.
1 Introduction
In December 2019, a novel Severe Acute Respiratory Syndrome Corona Virus 2 (SARS-CoV2) was found to be the cause of the Coronavirus disease (COVID-19) outbreak. SARS-CoV2 spread rapidly worldwide, resulting in a pandemic that started in March of 2020 (World Health Organization, 2020). To date, it has infected over 556 million people and caused more than 6.3 million deaths globally (https://www.worldometers.info/coronavirus/). The pandemic has also negatively impacted international travel, trade, education and social interactions across the globe. Coronaviruses (CoVs) have caused three 21st century outbreaks of SARS-related diseases in humans, namely: SARS-CoV of 2004, Middle East Respiratory Syndrome (MERS-CoV) of 2012 and the current SARS-CoV2 of 2019 (Zhang T. et al., 2020; Zhu et al., 2020). SARS-CoV2 is part of the β-coronavirus genus which shares 79% sequence identity with SARS-CoV and 50% with MERS-CoV (Wang Y. et al., 2020). The clinical features of COVID-19 are flu-like symptoms, including nasal congestion, sore throat and dry cough, which can lead to severe pneumonia (Zhu et al., 2020). Severe cases of COVID-19 infection have been reported to be associated with comorbidities such as chronic respiratory and cardiovascular diseases, diabetes, hypertension, and cancer. In these cases, the main causes of death are shock, respiratory and multiple organ failures. This review will mainly focus on the host stress response process to SARS-CoV2 infection and discuss the roles of host cell stress pathways in regulating the progression of the various comorbidities associated with the infection. We further discuss the therapeutic potential of targeting these processes.
2 SARS-CoV2 as a cellular stressor
SARS-CoV2 entry into host cells is an important step in viral infectivity and pathogenesis. To facilitate entry, the viral surface-exposed spike glycoprotein (S) attaches to the host cell receptors. The S protein has a receptor-binding domain (RBD), which contains a cleavage site where it is first preactivated with a proprotein convertase furin. Once it is processed, the RBD binds to the human angiotensin-converting enzyme 2 (ACE2) receptor on the host cells (Zhang H. et al., 2020). Studies have shown that SARS-CoV2 utilises the same ACE2 receptor as SARS-CoV to enter the host cell via its S protein (Hoffmann et al., 2020). The RBD is generally believed to facilitate receptor-mediated endocytosis for viral entry into the host cells (Petersen et al., 2020). However, several other mechanisms of viral entry have been proposed (Figure 1). These mechanisms include: A. The canonical clathrin-mediated endocytosis pathway, which is thought to be ACE2 receptor-dependent and pH-sensitive (Milewska et al., 2018; Yang and Shen, 2020); B. The non-canonical caveolae independent endocytosis pathway (Szczepanski et al., 2018), which involves anchored lipid rafts and micropinocytosis (Glebov, 2020); C. Flotillin-1-associated endocytosis; D. Clathrin-independent carrier (CLIC)/glycosylphosphatidylinositol-anchored protein-enriched endosomal compartment (GEEC) endocytosis and E. Macropinocytosis (Glebov, 2020). These various entry pathways are thought to be utilised by the virus depending on the target cell types. It has been reported that more than one mechanism of host entry could be employed by SARS-CoV2 (Yang and Shen, 2020).
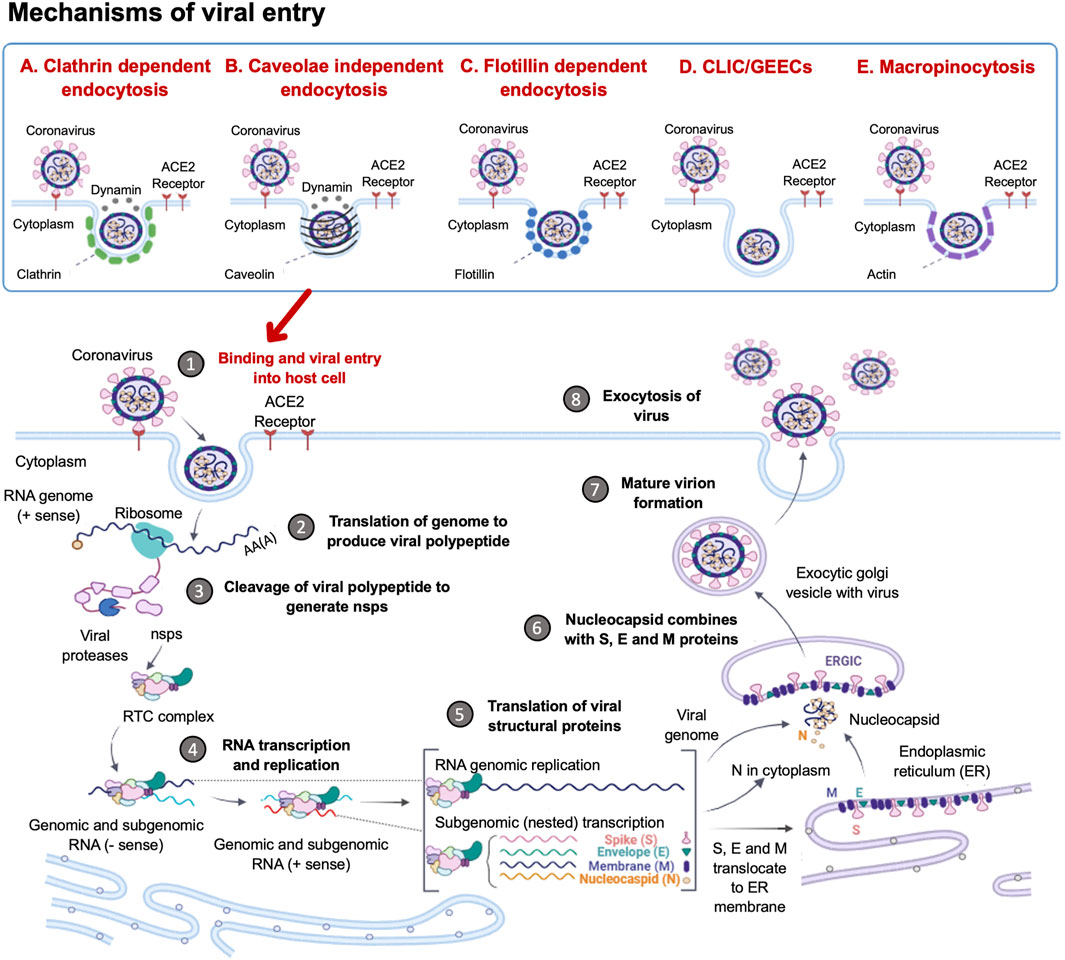
FIGURE 1. Coronavirus Life Cycle in the Host Cell. The potential mechanisms of viral entry into the host cell include canonical Clathrin-dependent endocytosis and non-canonical pathways such as: Caveolae-independent endocytosis, Flotillin-dependent endocytosis, CLIC/GEEC endocytosis and Macropinocytosis. After viral entry, the replication of the coronavirus in the target cell is initiated. The RNA genome is uncoated, which allows for the initiation of translation using host ribosomes to produce viral polypeptides. These polyproteins are cleaved by proteases to produce non-structural proteins (NSPs), which are responsible for the formation of the replication-transcription complex (RTC). The RTC facilitates the production of genomic and sub-genomic RNA (-sense and + sense) copies. Following the sub-genomic (nested) transcription, viral structural proteins are produced: spike (S), small envelope (E), membrane (M) and nucleocapsid (N) proteins. The ER facilitates the translation of these viral structural proteins and subsequent embedding on the ER membrane. The nucleocapsids assemble in the cytoplasm and bud off to the Endoplasmic Reticulum-Golgi Intermediate Compartment (ERGIC), where they combine with the structural proteins. The accumulation of viral material causes swelling of the Golgi-apparatus, which results in the formation of smooth structures of virions budding off as enveloped smooth vesicles containing the newly acquired envelopes. These mature virions are released through exocytosis. (Figure created using https://biorender.com/).
Following the entry of the virus into the host cell via an endosome, lysosomal proteases facilitate viral uncoating to release the RNA genome in the cytoplasm. When the released positive-sense RNA genome is translated at the ribosome to produce viral polypeptides and structural proteins. This is followed by the transcription of the viral genes with subsequent packaging in the nucleocapsids (Figure 1). In the first event, the positive-sense RNA genome is translated into two large viral polyproteins, namely: PP1a and PP1b, that are encoded from approximately two-thirds of the viral genome (Chen et al., 2020; Hidalgo et al., 2021). These viral polyproteins are cleaved by viral encoded chymotrypsin-like proteases (3CLpro) and papain-like proteases, to yield 16 non-structural proteins (NSP1-16) (Andersen et al., 2020; Uddin et al., 2020). The second event is facilitated by NSPs that form the replication-transcription complex (RTC) which facilitates the transcription and replication of the remaining one-third of sub-genomic mRNAs encoding for the four main structural proteins, namely: spike (S) receptor binding, envelope (E), membrane (M) and nucleocapsid (N) (Gordon et al., 2020; Kim et al., 2020). Thereafter, the structural proteins are embedded onto the Endoplasmic Reticulum-Golgi Intermediate Compartment (ERGIC) or on the Endoplasmic Reticulum (ER). These newly synthesised genomic RNA molecules and proteins accumulate to form the nucleocapsid. The modified ERGIC containing the viral structural proteins, buds off the ER and encloses the nucleocapsid to form a mature virion (Klumperman et al., 1994). The new virions are prepared for secretion through exocytosis via the Golgi apparatus. During this process, the ER membranes are depleted and the cell protein folding machinery is hijacked to process these viral proteins. These events exert stress upon the infected cell. Interestingly, it has previously been established that the stress-induced expression of heat shock proteins (Hsps) in Drosophila restricts viral infectivity (Merkling et al., 2015).
3 Overview of stress proteins
Stress proteins (SPs) are a set of molecular chaperones, whose expression is upregulated in response to cellular stress. These SPs are involved in cytoprotection by facilitating protein folding and unfolding, protein activation and the assembly of protein complexes. Cellular stress response modulates pathways that stimulate cell survival or cell death and the dysregulation thereof. For this reason, cell stress response is implicated in various human diseases including cardiovascular diseases, neurodegenerative diseases, cancer and some other infectious diseases. Hsps, along with the predominantly ER localised Protein Disulphide Isomerases (PDI) (Wan et al., 2020), constitute key components of the cellular stress response machinery. Hsps are generally classified into several different families based on their average molecular sizes in kDa as well as sequence conservation. They fall within the following key groupings: Hsp110, Hsp100, Hsp90, Hsp70, Hsp60, Hsp40 (also known as J domain proteins; JDP) and lastly, small Hsps (sHsps) (Figure 2). Due to their central role, Hsps are implicated in several cellular pathways and are inherently linked to various pathologies (Favatier et al., 1997; Edkins et al., 2018).
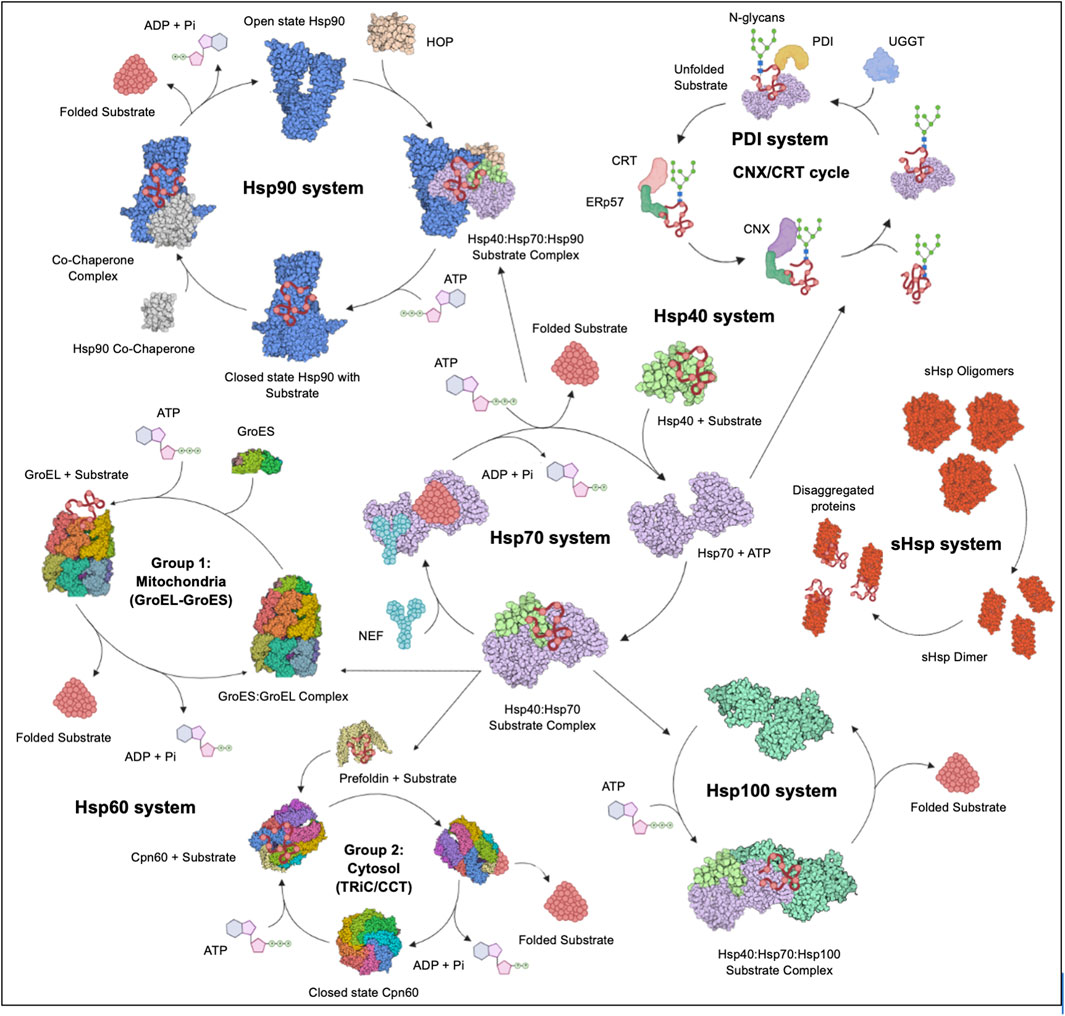
FIGURE 2. The Hsp chaperone system. The Hsp40 chaperone system recruits nascent substrate proteins and transports them to the Hsp70 folding system. Thereafter, the folded clients are transferred over to the Hsp90 system for activation or assistance to form multiple protein complexes. The more complex substrates are brought to the Hsp60 (GroEL) and TRiC systems. Unfolded proteins are transferred to the Hsp100 system for disaggregation in cooperation with the sHsp system. The CRT and CNX recruit Hsp folded glycoproteins to PDIs for further folding and disulphide bond stabilisation. Figure adapted from the HSP information resource database (http://pdslab.biochem.iisc.ernet.in/hspir/index.php).
3.1 The Hsp100 protein family
In humans, there are six Hsp100 members with diverse functions. Two members exhibit protein disaggregation capabilities, while the other four members are proteases (represented by caseinolytic protease (Clp)). Structurally, Hsp100s are grouped into two groups, type 1 and type 2. Type 1 refers to members with two AAA+ ATPases domains, namely the NBD 1 and NBD2. These domains are characterised by the presence of two walker motifs (1 and 2) and a middle domain between the NBDs (Zolkiewski et al., 2012). Hsp104/ClpB, ClpA, ClpC and Hsp78 constitute the type I cluster. The type 2members of the Hsp100 members, ClpP and Hsv, possess a single NBD2 but lack the middle domain. Hsp100 proteins have been implicated in neurodegenerative diseases and other protein-folding-related diseases. Their implication in these pathologies is based on their role in suppressing and reversing protein aggregation (Ferrari et al., 2018). As a disaggregase, Hsp100 occurs in complex with Hsp70, Hsp110 and Hsp40 (Kaimal et al., 2017; Lin et al., 2022). This highlights that while the functions of the various Hsps are unique, they also cooperate to manage cellular stress.
3.2 The Hsp90 protein family
Five human Hsp90s are localised within the cytosol, ER and mitochondria. The three cytosolic paralogs include: the stress-inducible α-Hsp90 (HSP90AA2/HSPC2), the truncated chimeric Hsp90 (HSP90AAA1/HSPC1) and the housekeeping β-isoform (HSPB1/HSPC3) (Chen et al., 2005). The ER and mitochondria host the 94 kDa glucose-regulated protein (Grp94/HSPC4) and the tumour necrosis factor receptor-associated protein-1 (TRAP 1/HSPC5) respectively (Kampinga et al., 2009). Structurally, these proteins share a conserved domain architecture that comprises the N-terminal ATPase domain, the middle domain with substrate binding capability and the C-terminal dimerization domain (Jackson, 2012). Hsp90s are ATP-dependent molecular chaperones that play a central role in protein homeostasis (Obermann et al., 1998; Chakraborty and Edkins, 2021). The function of Hsp90 is regulated by several co-chaperones (Bachman et al., 2018). In the ADP-bound state, the clients/substrates are recruited into an early complex consisting of the Hsp70/Hsp40/Hsp-interacting protein (HIP) and the Hsp90/Hsp70-organising protein (Hop) (Luengo et al., 2019). ATP hydrolysis is activated by the Hsp90 ATPase activator 1 (AHA1; Oroz et al., 2019). Following nucleotide exchange, the Hsp90 forms a mature complex with co-chaperones p23, p50, cell division cycle 37 (cdc37) and immunophilins (Biebl et al., 2020). Furthermore, post-translational modification of Hsp90 through phosphorylation (Xu et al., 2019) and acetylation (Mollapour and Neckers, 2012), regulates its functional specificity (Prodromou, 2016). Host Hsp90 substrates that are associated with the possible uptake of viruses include those responsible for transcription, translation, mitochondrial function, kinetochore assembly, centrosome function and maintenance of the cell cycle (Lubkowska et al., 2021). Hsp90 also facilitates membrane trafficking and membrane deformability during the release of exosomes (Lauwers et al., 2018). There is a wide array of Hsp90 clients, most of which are molecules involved in signal transduction. Hsp90 facilitates the conformational maturation of several of its client. In addition, Hsp90 is also involved in the ordered assembly and stabilisation of subunits of multiprotein complexes (Makhnevych and Houry, 2012). This highlights a possible central role of this chaperone in viral replication within the host. To this end, inhibitors of Hsp90 are of interest as possible therapies against COVID-19 (Ramos and Ayinde, 2021; Wyler et al., 2021; Biancatelli et al., 2022).
3.3 The Hsp70 family
At least seventeen members of the Hsp70 family of chaperones are found in humans. Hsp70s are grouped into two subfamilies: canonical members (Hsp70) and non-canonical members (Hsp110) subgroup. There are 13 canonical Hsp70s which resemble the prokaryotic Hsp70, represented by E. coli DnaK. They include the cytosolic Hsp70-1/Hsp72/HSPA1A, HSPA1B/Hsp70-2, HSPA1L/Hum70t, HSPA2, Hsp70B/HSPA6, HspA7/Hsp70-7, Hcs70/HSPA8, HSPA12A/FLJ13874, HSP112B/RP23-32L15.1, HSPA13/Stch and HspA14/Hsp70-4; the ER localised Grp78/Bip/HSPA5 and the mitochondrial localised mortalin/GRP75/HSPA9. Hsp110 members include, the cytosolic Hsp105/HSPH1, Apg-2/Hsp110/HSPH2, Apg-1/HSPH3 and the ER localised Grp170/HSPH4 (Easton et al., 2000; Kampinga et al., 2009; Chakafana and Shonhai, 2021). Structurally, Hsp70s are composed of an N-terminal nucleotide-binding domain (NBD) that exhibits ATPase activity and a C- terminal substrate-binding domain (SBD). The Hsp110 subfamily displays a similar domain architecture, however, they have a long acidic insertion in the SBD, making them larger members (Oh et al., 1999; Chakafana et al., 2019). Hsp110 functions as a chaperone, whilst also facilitating nucleotide exchange for its canonical Hsp70 counterparts (Dragovic et al., 2006; Andreasson et al., 2008). The Hsp70 chaperone plays a central role in the folding of nascent polypeptides released from the ribosomes. It also refolds misfolded proteins and as well as facilitates the assembly of multiprotein complexes (Figure 2). In addition, Hsp70 also cooperates with Hsp90 and Hsp60 to facilitate the maturation of some of its clients (Luengo et al., 2019; Wang et al., 2022).
The primary co-chaperone of Hsp70s are the Hsp40 proteins (JDP). Hsp40s are composed of a conserved J domain that facilitates the interaction with Hsp70, activating ATP hydrolysis by the latter (Cintron and Toft, 2006; Kampinga et al., 2009). Hsp40s are also known to recruit substrates to Hsp70 and are therefore called substrate scanners (Fan et al., 2003). Hsp70 has a high affinity for substrates in the ADP-bound state. To facilitate substrate release, the nucleotide exchange factors (NEFs), such as Hsp110, exchange ADP for ATP (Dragovic et al., 2006; Alderson et al., 2016). These functions contrast with HIP, which locks Hsp70 in the ADP-bound state (Nollen et al., 2001). Therefore, the action of NEFs and HIP determines the substrate residency time on Hsp70, which influences substrate fate. Hsp70 was found to be one of the distinct biomarkers circulating in COVID-19 ICU cases (Fraser et al., 2020). Considering the cytoprotective role of Hsp70, it has been proposed that the periodic fever conditions associated with COVID-19 infections, may benefit the host by stimulating the expression of this chaperone (Guihur et al., 2020).
The ER-based chaperones have widespread functions in nearly every stage of protein processing (Figure 3). During protein import into the ER lumen, newly synthesised polypeptides that emerge from the ribosomes, are recognized by the signal recognition particle (SRP), which transports these proteins to the ER membrane for translocation via the Sec61 channel (Haßdenteufel et al., 2018; Jomaa et al., 2022). The ER-resident Hsp70 (Grp78/BiP), binds incoming peptides and actively threads them into the ER lumen (Craig, 2018; Haßdenteufel et al., 2018). BiP and the ER-resident Hsp40 (ERdj5), play a role in the post-translational insertion of proteins into the ER membrane (Araki and Nagata, 2012). They also facilitate the processing of aggregated membrane proteins, by earmarking them for degradation. Additionally, ER chaperones also facilitate the export of proteins from the ER through their involvement in the ERGIC pathway (Ito and Nagata, 2019). Irreparably misfolded proteins are destroyed by autophagy or redirected to the ER-associated degradation (ERAD) pathway for destruction in the proteasomes located in the cytosol (Oikonomou and Hendershot, 2020; Braakman and Hebert, 2021). This pathway involves the ER-resident chaperones such as ERdj5, BiP and Grp94, which bind and target substrates for degradation (Adams et al., 2019).
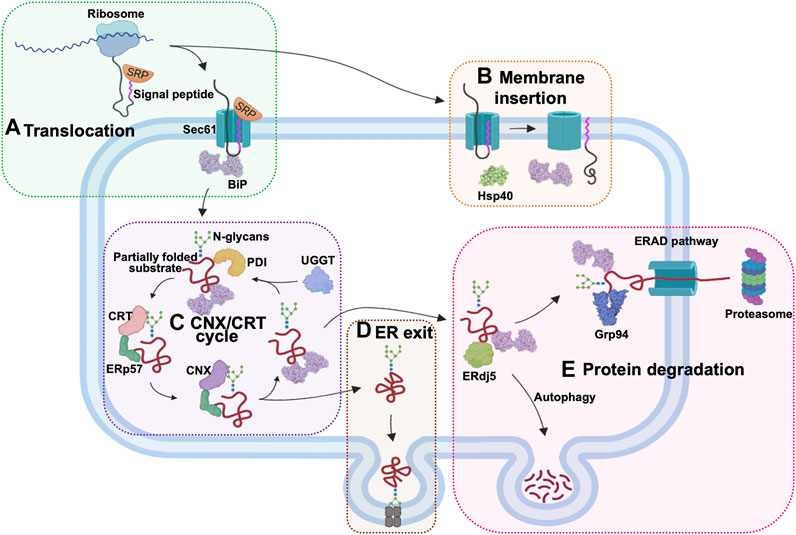
FIGURE 3. The role of ER chaperones in proteostasis. The ER-localised chaperones have numerous roles in this cellular organelle. (A) ER chaperones enable the translocation of proteins into the ER. Newly synthesized proteins are targeted by the signal recognition particle (SRP) as they emerge from the ribosomes. The SRP-bound protein enters the ER through the Sec61 translocon protein channel and is bound by Hsp70 (Grp78/BiP) inside the ER lumen for active import. (B) Chaperones also facilitate post-translational ER membrane insertion of proteins. (C) The CNX/CRT chaperone system facilitates proper glycosylation of proteins targeted for various cellular compartments. (D) ER chaperones are involved in processing the exit of the proteins via the ERGIC pathway. (E) Proteins that are not properly folded are bound by ERdj5, BiP, and Grp94 in the ER and channelled for degradation by autophagy or through the ERAD pathway in the cytosol.
3.4 The Hsp60 chaperonins
Hsp60 proteins are ATP-dependent chaperonins and are classified into two main groups, namely, type 1 and type 2 (Okamoto et al., 2017; Ishida et al., 2018). Type 1 chaperonins are mainly found in the mitochondria of eukaryotes and in the cytoplasm of prokaryotes (GroEL in E. coli). This class of chaperonins form a 7-member ring back-to-back complex with a central core, which then requires Hsp10 (GroES in E. coli) to close the core, functioning as a lid (Enriquez et al., 2017). The type 2 chaperonins, which include the cytosolic TCP1-ring complex (TRiC), are not well studied but are found in the archaeal chromosome and eukaryotic cytosol. They form a similar dimerization of the 8-9 protomer complexes to make 16–18 subunits joined end to end, forming a barrel structure with a central core (Ishida et al., 2018). Similar to type 1 chaperonins, the central core is closed by the Hsp10 protein. Some Hsp60s escape the mitochondria and translocate to the circulatory system, where they are known to induce proinflammatory cytokines. For this reason, Hsp60 is implicated in hypertension and is therefore thought to aggravate COVID-19 related complications (Jakovac, 2020).
3.5 Small heat shock proteins
In humans, there are eleven members of the small heat shock protein (sHsp) family of chaperones. They include HSPB1/Hsp25, HSPB2/Hsp27, HSPB3/Hspl27, HSPB4/crystallin α-A, HSPB5/crystallin α-B, HSPB6/Hsp20, HSPB7/cvHsp, HSPB8/Hsp22, HSPB9/FLJ27437, HSPB10/ODF1 and HSPB11/Hsp16.2 (Kampinga et al., 2009). Structurally, sHsps have a conserved α-crystallin domain, sandwiched with a variable N-terminal region and the C-terminal regions. These sHsps exhibit holdase chaperone activity which is ATP-independent. They function in complexes to minimise protein unfolding and serve as holdases (Haslbeck et al., 2019). The most studied sHsps are the Hsp27, crystallin α-A and crystallin α-B. Of these, only Hsp27 has been implicated in COVID-19 pathology (Wendt et al., 2021).
3.6 Protein disulphide isomerases
Protein Disulphide Isomerases (PDIs) are oxidoreductases that catalyse the enzymatic reduction and isomerization of disulphide bridge formation (Appenzeller-Herzog and Ellgaard, 2008). In eukaryotes, almost a third of the cellular proteome contains disulphide bonds (Mahmood et al., 2021). In the ER, the PDIs stabilise and promote the folding of client proteins into three-dimensional structures (Kranz et al., 2017). In humans, there are 19 PDIs localised in the ER (Ellgaard and Ruddock, 2005). Generally, the PDIs are characterised by the CXXC active site motif, where the cysteine residues take part in the exchange of disulphide bond formation to stabilise client proteins. PDIs are also involved in ER protein degradation (ERAD) and calcium level regulation (Kramer et al., 2001; Riemer et al., 2011). The most studied PDI is PDIA3 (ERp57/Grp58), which is comprised of the canonical four thioredoxin domain structure of the a-b-b-a domain organisation. In general, the a-domains of PDIs contain the catalytic CXXC active site motif, which can exhibit thiol-sulphate reductase, oxidase or isomerase activity (Darby and Creighton, 1995; Chichiarelli et al., 2022). The b-domains bind substrates with high affinity to facilitate isomerization (Klappa et al., 1998). The PDIA3 b-domains do not directly interact with substrate proteins but rather indirectly through their association with lectins, calreticulin (CRT) and calnexin (CNX) (Oliver et al., 1999; Molinari et al., 2004). The CRT and CNX recruit glycoproteins to PDIA3 for correct folding and disulphide bond stabilisation. If the PDI fails to achieve a competent fold, the substrate protein undergoes re-glycosylation by the glucose:glycoprotein:glucosyl transferase (UGGT) to repeat the cycle (Kozlov and Gehring, 2020; Mahmood et al., 2021). Therefore, PDIs play an essential role in protein disulphide bond formation and protein glycosylation quality control mechanisms that are thought to be essential for SARS-CoV2 protein maturation (Fu et al., 2020). Abnormalities in these protein quality control systems in all cell organelles, have severe consequences for the cell and have been implicated in several diseases (Parakh and Atkin, 2015; Chamberlain and Anathy, 2020). Protein folding aberrances are at the centre of diseases such as cancer, neurodegenerative disorders, metabolic diseases, and infections (Gámez et al., 2018).
4 COVID-19 susceptibility profiles
SARS-CoV2 pathogenesis is mainly exacerbated by underlying cellular stress, which is more pronounced in patients with comorbidities such as diabetes, cardiovascular disease, hypertension and obesity among others (Sanyaolu et al., 2020). Generally, viral infections are associated with inflammation, a hallmark of COVID-19 pathology (Varga et al., 2020), which further puts strain on the protein folding system (Kuppalli and Rasmussen, 2020; McGonagle et al., 2020). The role of Hsps in immunomodulation is well established and appears to be a function of their circulating levels (Zininga et al., 2018). Thus, the expression profiles of these proteins in various disease conditions could serve as biomarkers of disease severity and patient outcomes (Table 1).
4.1 Chronic lung diseases
Chronic lung diseases include a wide array of diseases such as asthma, Chronic Obstructive Pulmonary Diseases (COPD), Interstitial Lung Diseases (ILD), cystic fibrosis, lung cancer and chronic pneumonia to name a few (Cottin, 2013; Celli and Wedzicha, 2019). Chronic lung diseases cause excessive inflammation, immune dysregulation, and impaired repair processes, which ultimately leads to tissue damage and diminished organ function (Meikle et al., 2021). Chronic inflammation is a prominent symptom of chronic lung diseases and causes elevated levels of reactive oxygen species (ROS) in cells, resulting in oxidative stress (Hulgan et al., 2003; Sharif-Askari et al., 2021). Excessive ROS may stimulate prolonged inflammatory responses and signalling cascades that damage cells, which may lead to apoptosis (Chatterjee, 2016; Ivanov et al., 2017).
Hsp70 is variably expressed depending on the nature of the conditions affecting the lungs. The elevated expression of Hsp70 associated with asthma may trigger either pro- or anti-inflammatory pathways, due to its diverse immunomodulating effects (Shevchenko et al., 2020). Hsp70 and Hsp27 expression levels were elevated in lung tissues of patients with COPD, when compared to healthy controls, and were correlated to disease severity (Dong et al., 2013; Zimmermann et al., 2020). In Idiopathic Pulmonary Fibrosis (IPF), one of the common interstitial lung diseases, Hsp70s were observed to be downregulated in response to an increase in the profibrotic molecules, IGFBP5 (insulin-like growth factor-binding protein 5) or (TGF β1) transforming growth factor-β1 (Sellares et al., 2019). Thus, Hsp70 suppression perpetuates fibrosis development in the human fibroblasts. Hsps alleviate oxidative stress through their active roles in the refolding of damaged proteins and PDIs are important for maintaining a redox balance inside the cells suppressing the development of pulmonary fibrosis (Parakh and Atkin, 2015; Marinova et al., 2020; Tanguy et al., 2021).
One of the major complications of COVID-19 is the development of acute respiratory distress syndrome (ARDS), a condition that tremendously impairs the ability of the lungs to absorb oxygen (Meikle et al., 2021). In a 2007 study, lung injury was induced in rats, whereafter the rats were administered with adenoviral vectors expressing Hsp70 proteins (Weiss et al., 2007). It was observed that Hsp70 limited NF-ĸB activation, which in turn limited the proteasomal degradation of IĸBα. Indeed, Hsp70 expression is reportedly elevated in ARDS (Alreshidi et al., 2021), and is known to inhibit intracellular proteasomal degradation (Ryu and Ha, 2020). It was observed that Hsp70 limited NF-ĸB activation, which in turn limited the proteasomal degradation of IĸB kinase signalosome, thereby suppressing inflammation (Weiss et al., 2007). Thus, by suppressing inflammation, Hsp70 expression may indirectly regulate COVID-19 pathology (Sharif-Askari et al., 2021). Additionally, it was reported that several oxidative stress genes are upregulated during Coronavirus infection and expression of these genes is thus likely induced by SARS-CoV2 (Sharif-Askari et al., 2021). Hsp70 being a central player in preventing the accumulation of oxidative stress, might be similarly affected by SARS-CoV2. Although not yet experimentally confirmed, there might be a link between the dysregulation of Hsp70 and other stress proteins and the severity of SARS-CoV2 infection.
4.2 Chronic kidney disease
Chronic Kidney Disease (CKD) is a condition characterised by glomerulosclerosis and interstitial fibrosis (Musiał and Zwolińska, 2011). CKD is commonly a result of stress inflicted on hepatocytes from various sources such as, uremic toxins, pro-inflammatory molecules, reactive oxygen species, pro-apoptotic molecules, infectious agents, and dialysis (Nayak Rao, 2016). Several studies reported that the level of Hsp72 expression was upregulated in patients with CKD (Musial and Zwolińska, 2011; Lebherz-Eichinger et al., 2012; Morales-Buenrostro et al., 2014). The presence of uremic toxic may cause increased expression of Hsp72, which has been shown to inhibit the proliferation and apoptosis of renal tubular cells, resulting in reduced renal fibrosis (Pan et al., 2020). Chronic kidney damage is also thought to be associated with noxious conditions where upregulated Hsp72 suppresses apoptosis (Rao, 2016). Although research regarding the role of Hsps in CKD is limited, more work on the role of these proteins in renal dialysis has been conducted. It has been observed that renal ischemia-reperfusion injury (IRI), incurred during renal dialysis, resulted in an induction of Hsp72 (43-fold increase) and Hsp27 (12-fold increase) (Nayak Rao, 2016). Additionally, Hsp70 offers protective properties from renal IRI that include, cytoskeletal stabilization, anti-inflammatory effects, anti-apoptotic properties, and influence over the stimulation of regulatory T-cells (Nayak Rao, 2016). These functions of Hsp70 and sHsp are potentially important in reducing further complications upon the onset of SARS-CoV2 (ERA-EDTA Council et al., 2021). Furthermore, elevated levels of Hsp90 observed in CKD patients are associated with increased oxidative stress and inflammation (Musial and Zwolińska, 2011).
4.3 Obesity
Obesity is a metabolic syndrome generally linked to the increased severity of several non-communicable diseases such as, type 2 diabetes mellitus (T2DM), cardiovascular diseases (CVD) and certain cancers (Blüher, 2019). The accumulation of adipose tissue and the increases in energy inputs associated with obesity often triggers chronic inflammation in the fatty tissues (Sell et al., 2017). This inflammation results in an increase in proinflammatory cytokines, both locally and systemically (Lehr et al., 2012; Sell et al., 2012; Tiss et al., 2014). The role of Hsps in inflammation in obese individuals is not well established, as conflicting results have been reported. For example, increased levels of Hsp60, Hsp72, Hsp90, Hsp70 and Grp94 released from adipocytes under stressful conditions have been shown to act as adipokines, linking their expression to obesity and chronic inflammation (Märker et al., 2012; Tiss et al., 2014; Sell et al., 2017). Conversely, individuals with obesity and insulin resistance were reported to exhibit suppressed heat shock response (HSR) activity which by extension results in reduced Hsps expression, as insulin signalling is essential to HSR activity (Di Naso et al., 2015; de Lemos Muller et al., 2018; Bruxel et al., 2019). This contrasts with normal inflammatory conditions where the HSR upregulates Hsp production, which counteracts the inflammatory response (Singh and Hasday, 2013; Zininga et al., 2018; Krause et al., 2020). The decreased HSR activity in obese individuals could be responsible for the dysregulated inflammation and negative prognosis in individuals infected with SARS-CoV-2 (Krause et al., 2020). Unlike the other Hsps, Hsp40 expression is decreased in obese individuals. A study reported that normal levels of Hsp40 were restored upon exercise, suggesting a possible role for this protein in the regulation of insulin resistance and thus mitigating against obesity (Tiss et al., 2014).
4.4 Diabetes
The metabolic disorder, T2DM, is characterised by the dysregulation of insulin production and activity, leading to chronically elevated levels of sugar in the blood. The disruption of insulin production associated with diabetes, in turn, disrupts the insulin signalling pathway which is a crucial part of the HSR system. Insulin inhibits the activity of the glycogen synthase kinase-3β (GSK-3), which suppresses the activation of HSF-1, abrogating its interaction with heat shock elements, an important step in regulating transcription of HSP genes (Bijur et al., 2000). Without insulin to inhibit the activity of GSK-3, HSR activity is downregulated and so is the expression of Hsps. Therefore, patients with diabetes are more susceptible to severe infections as they are unable to regulate the resulting inflammation, culminating in further complications (Krause et al., 2020). This partially explains the increased case fatality in individuals infected with COVID-19 when compared to non-diabetic patients (Xue et al., 2020). T2DM patients display dysregulated levels of Hsp60, Hsp70 and Hsp72, which contribute to inflammation and insulin resistance and vascular complications (Khadir et al., 2018; Zilaee and Shirali, 2016; Amawi et al., 2019).
4.5 Cardiovascular diseases
Cardiovascular diseases (CVD) are characterised by cellular stress, in which a collection of cardioprotective Hsps are released in the heart (Henderson and Pockley, 2012; Ranek Mark et al., 2018). Several Hsps including Hsp27, Hsp60, Hsp70 and Hsp90, are secreted and released at different rates during coronary stress (Jaroszyński et al., 2018; Duan et al., 2020; Krishnan-Sivadoss et al., 2021). A high secretion of Hsp27 has been shown to offer some cardioprotection, whilst low Hsp27 serum levels, especially in older patients, has been associated with carotid atherosclerosis and oxidative stress. This leads to an increased risk of cardiovascular disease and sudden cardiovascular death (Jaroszyński et al., 2018). Upon cardiac injury, Hsp60 is released into the extracellular fluid, where it activates the body’s innate immunity through the induction of a proinflammatory state in the heart. The subsequent increase in the production of the tumour necrosis factor, TNF-α, facilitates apoptosis and thus attributes to the progression of heart failure (Duan et al., 2020).
Upregulated Hsp60 expression has been found in atherosclerotic lesions and has increased the risk of atherosclerosis (Grundtman et al., 2011). In addition, the cross-reactivity of the immune system with autologous Hsp60 and Hsp70 results in T-cell adhesion to endothelial cells and the initial inflammatory response of atherosclerosis (Rodriguez-Iturbe et al., 2019; Duan et al., 2020). Consequently, autoantibodies produced against either Hsp60 or Hsp70 were reported to exacerbate atherosclerosis (Schett et al., 1997; Stocker and Keaney, 2004; Wick et al., 2014) and hypertension (Rodriguez-Iturbe et al., 2019; Romagnoli et al., 2020). Therefore, Hsp60 and Hsp70 are intricately involved in the development and progression of atherosclerosis and subsequent complications in other diseases. Notably, there are increasing reports linking the induction of Hsp70, Hsp90 and co-chaperones to heart failure (Ranek Mark et al., 2018; Rodriguez-Iturbe and Johnson, 2018).
Patients who become infected with SARS-CoV2, whilst having underlying cardiovascular conditions, have a higher risk of developing a severe infection, myocarditis, and blood clots, which increases the chance of death (Huang et al., 2020; Srivastava, 2020). This is due to a combination of the effects of a viral infection, coupled with the stress caused by the underlying cardiovascular conditions (Srivastava, 2020). Consequently, the intricate involvement of Hsps in several cardiovascular diseases most likely influences the severity of COVID-19 in these patients. Hsp60 appears to be the most studied Hsp implicated in cardiovascular diseases and COVID-19 (Jakovac, 2020). For example, one study hypothesized that the high levels of Hsp60 present in the plasma of hypertensive patients contribute to the cytokine release syndrome (Song et al., 2020). This is the main mechanism responsible for the third hyperinflammatory phase of COVID-19, which often leads to heart failure (Romagnoli et al., 2020). SARS-CoV2 also causes substantial tissue damage, which can result in the release of intracellular Hsp60 into the plasma. Subsequently, this causes an increase in pre-existing Hsp60 levels and could result in systemic hyper inflammation, causing damage to multiple organs (Romagnoli et al., 2020). It was also found that Hsp60 levels in the plasma positively correlate to acute lung injury and systemic inflammatory responses in patients with no prior pulmonary trauma (Pespeni et al., 2005). Although more research is required to fully understand the role of Hsps in heart failure, there is a common consensus that these proteins have important therapeutic and diagnostic considerations in COVID-19.
4.6 Cancer
Cancer is a disease state during which abnormal cells grow rapidly and uncontrollably, such that they have harmful effects on tissues and organs. Cancerous cell propagation is highly dependent on stress proteins to assist in the folding of improperly folded and mutated proteins, for their continued dysregulated growth and development (Wu et al., 2017a; reviewed in Chakafana and Shonhai, 2021). The underlying causes and mechanisms involved in Hsp expression are not fully understood. For example, Hsp27 and Hsp70 expression is downregulated in neuroblastoma and renal cancer respectively (Wu P. et al., 2017). Conversely, in hepatocellular carcinoma (HCC), the upregulation of Hsp27 plays a cytoprotective role in preventing cancerous cell apoptosis by interfering with the proteins in the apoptotic pathways (Guo et al., 2009; Wang et al., 2015). Cancer cells exploit the’ cytoprotective function of Hsp70 to sustain themselves (Giri et al., 2017). In HCC and lung cancer, it was observed that both Hsp90 and Hsp70 expression levels were upregulated, leading to proliferation and metastasis of cancerous cells (Leng et al., 2012; Cedrés et al., 2018; Sun et al., 2018; Jiang and Shen, 2020). Cell survival was facilitated through cytochrome c inhibition, regulation of extracellular signal-regulated kinase (ERK), phosphorylation of protein kinase B (Akt) and degradation of apoptotic components (Guo et al., 2009; Wang et al., 2015). Therefore, the upregulated expression levels of Hsp27, Hsp70 and Hsp90, increase the invasion and metastasis of some cancerous cells (Katsogiannou et al., 2014; Zhou et al., 2015; Saha and Anirvan, 2020; Wan et al., 2020). Furthermore, the Hsp70 and Hsp60 proteins present on the surface of cancer cells are implicated in immunomodulation, as they bind and activate immune cells and antibodies (Burgio et al., 2021). Thus, this could explain the possible link between cancer and COVID-19 susceptibility. This association is thought to be due to the dysregulated immune system in both cancer and COVID-19 patients (Zong et al., 2021).
5 Cellular stress drives heat shock protein expression
Cells that are exposed to stress respond by upregulating some of their Hsp stress proteins (Samali and Orrenius, 1998; Richter et al., 2010). The levels of upregulation are dependent on the type of stress, as the first response for cells is to facilitate post-translational modification (PTM). PTMs on Hsps modulate the chaperone efficiency and enable it to deal with increased demand due to increased protein unfolding. For example, Hsp90 phosphorylation and Hsp70 acetylation have been shown to increase their chaperone activities (Xu et al., 2012; Park et al., 2017). Continued stress stimuli activate the HSR, which is mainly modulated by HSF1 (Sarkar and Roy, 2017). In its inactive state, the HSF is bound to an HSP (Figure 4A). When an HSF is activated, it dissociates from the Hsp and undergoes phosphorylation and oligomerization in the cytosol, after which it translocates into the nucleus (Xu, 2012). The HSF binds to the HSE, located in the promoter regions of HSP genes, which in turn activates the increased expression of the Hsps (Kmiecik and Mayer, 2022).
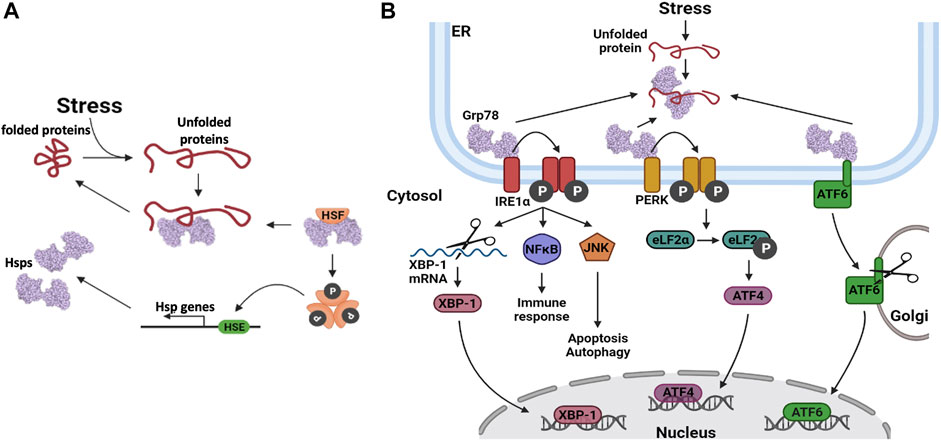
FIGURE 4. Heat shock protein expression regulation. (A) Upregulation of Hsp gene transcription. When unfolded proteins accumulate in the cell, Hsps dissociate from their bound heat shock factors (HSF). This frees up the Hsps to assist in protein folding and it also activates the HSF. The HSFs are phosphorylated, after which they oligomerize. The HSFs bind to heat shock elements (HSE) in the promoter regions of Hsp genes, thereby enhancing their transcription. (B) Hsp gene transcription is upregulated via three distinct pathways involving three distinct transcription factors (TFs); Inositol requiring enzyme 1α (IRE1α), protein kinase RNA-like endoplasmic reticulum kinase (PERK) protein and activating transcription factor 6 (ATF6).
The ER is also an important organelle in cell stress response as it houses a protein folding machinery. In the wake of external stress sources, the ER-resident Hsps are known to transverse and dissociate from their substrates to become available for the suppression of protein aggregation. Grp78 and Grp94 are central players in the ER protein refolding machinery, and they often cooperate to achieve their chaperone goals (Zhu and Lee, 2015). However, if these proteins are overwhelmed, the unfolded protein response (UPR) is activated. During this event, Hsp expression is upregulated, and cytosolic protein translation is halted to limit the peptide load in the ER (Marzec et al., 2012; Hetz and Papa, 2018). Terminally misfolded proteins that cannot be rescued are channelled to the ERAD pathway for degradation (Oikonomou and Hendershot, 2020; Ninagawa et al., 2021). It should be noted that the PDIs and their associated systems are also responsive to stress as they control proper disulphide bond formation and can also catalyse reverse reactions. In addition, they also distinguish properly and erroneously glycosylated proteins, towards channelling the latter to the ERAD for degradation.
The UPR stress response is activated and regulated via three distinct pathways which enable the expression of UPR related genes (Figure 4B). These three pathways are initiated by the dissociation of Grp78 from the client protein, in response to elevated levels of unfolded proteins in the ER lumen (Lukas et al., 2019). In the Inositol requiring enzyme 1α (IRE1α) pathway, IRE1α undergoes autophosphorylation, followed by oligomerization which facilitates its association with downstream signalling molecules (Hetz et al., 2006; Sano and Reed 2013). Thus, IRE1α interacts with c-Jun N-terminal kinase (JNK) to activate autophagy and with the nuclear factor NFĸB, to activate inflammatory signalling pathways that regulate the release of cytokines and chemokines (Sano and Reed, 2013). IRE1α also has endoribonuclease activity, which is utilized to alternatively splice the X-box binding protein 1 (XBP-1) mRNA, yielding the XBP-1 transcription factor. XBP-1 translocates to the cell nucleus where it activates the transcription of Grp78 and other proteins that are inherent to the ERAD pathway (Kitamura, 2008). The second pathway involves the protein kinase, RNA-like endoplasmic reticulum kinase (PERK). When PERK is released from Grp78, autophosphorylation of PERK is triggered, which in turn phosphorylates the translation elongation initiation factor 2α (eLF2α) (Schönthal, 2012). The subsequent inactivation of eLF2α, relieves ER stress by preventing additional protein synthesis and thereby decreasing the number of proteins that require folding. Transcription factor 4 (ATF4) is translocated to the nucleus, where it activates the expression of numerous stress regulating and pro-apoptotic genes (Lukas et al., 2019). The third pathway involves activating transcription factor 6 (ATF6). Upon dissociation from Grp78, this protein is transported to the Golgi apparatus, where it is proteolytically cleaved. The mature ATF6 protein enters the nucleus where it activates the expression of various UPR and ERAD pathway proteins (Sano and Reed, 2013; Lukas et al., 2019). These events are implicated in the viral life cycle as the coronavirus replication cycle induces ER stress (Sureda et al., 2020). As such, the patient’s UPR/ER stress response may be a predictor of the SARS-CoV2 antiviral response.
6 Heat shock protein upregulation could facilitate both viral cellular uptake and replication
Viral proteins, like human proteins, require host chaperones for the folding and assembly of complex viral core particles (Xiao et al., 2010). The upregulation of these host molecular chaperones thus facilitates viral replication (Table 2). Hsps are important in the replication of virtually all viruses including, DNA viruses, both positive and negative sense RNA genomes and double-stranded RNA viruses (Song et al., 2010; Wan et al., 2020). Due to limited data on the role of Hsps on SARS-CoV2 infection, we highlight some of the important pathways from other unrelated viruses that may use unique protein folding systems to draw similarities in COVID-19 pathogenesis. Generally, viral protein homeostasis presents a distinct set of clients for cellular protein folding machinery. As such, viral replication is subject to the folding capacity of the host cell due to three main factors. Firstly, the limited genomes of viruses entail that the viral proteins are multifunctional, and as such, require structurally complex proteins that are solely dependent on chaperones for folding (McBride et al., 2014). Secondly, many cytopathic viruses produce copious amounts of viral proteins within a short time (Oualikene et al., 2000), which places a huge protein folding burden on the host cell. Thirdly, viral capsid precursors are more prone to aggregation and misfolding due to their complexity as they are made of at least a thousand identical subunits (Rossmann, 1984; Geller et al., 2012; Cheng and Brooks, 2013). Several RNA viruses replicate with minimal proofreading and produce several mutant viral proteins during infection, which require a robust host chaperone system to fold into functional forms. In general, CoV manipulate the host chaperone system to render the cells more conducive for their replication. For example, the SARS CoVs E-protein has been implicated in suppressing the stress response in host cells upon infection (DeDiego et al., 2007). It has also been reported that SARS-CoVs structural proteins S, 6, 3a and 8a, induce ER stress response (Ye et al., 2008; Fung et al., 2014; Shi et al., 2019).
6.1 Viral entry
Several viruses interact with Hsps as auxiliary receptors to enter host cells through the clathrin-mediated endocytosis pathway. Hsp70 is commonly implicated as an auxiliary receptor (Figure 5), as is observed in the host cell entry of zika virus; a process that is facilitated by extracellular Hsp70 (Pujhari et al., 2019). The ER Hsp70 homology, Grp78, has been reported to facilitate the invasion of host cells by several viruses, amongst them, the Japanese encephalitis virus (Nain et al., 2017) and SARS-CoV2 (Ha et al., 2020; Carlos et al., 2021). The SBD of Grp78 positioned on the surface of African green monkey kidney epithelial Vero cells, was reported to recognize the S protein RBD of the SARS CoV2 to facilitate viral entry into these cells. In addition, the upregulated expression of Grp78 was associated with the surface expression of the ACE2 receptors in SARS-CoV2 patients (Sabirli et al., 2021). The interaction of the ACE2 receptors with Grp78 required both the NBD and SBD (Carlos et al., 2021). These findings suggest that the full-length human Grp78 protein and possibly its functional partners Grp94, Hsp40 and PDIs could be targeted to reduce SARS-CoV2 entry into host cells and to combat the ensuing viral infection.
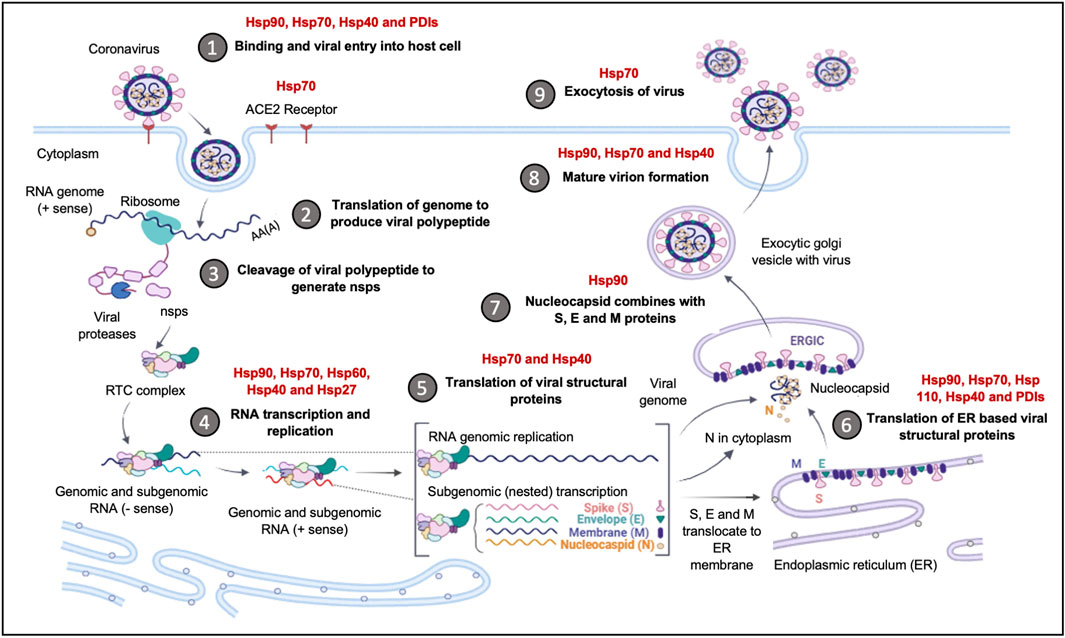
FIGURE 5. The upregulation of Hsps facilitates Coronavirus invasion and replication. The Hsps implicated in the Coronavirus replication cycle are highlighted. The binding and entry of the viral particle is achieved through the ACE-2 receptor, with Hsp90, Hsp70, Hsp40 and PDI, acting as auxiliary receptors. Upon viral entry and initial translation and transcription, Hsp40, Hsp70, Hsp60 and Hsp27, are recruited to facilitate in viral protein maturation. The folding of structural proteins is assisted by Hsp70 and Hsp90. The ER-resident chaperones, Grp94, Grp78, ERDnaJ and PDIs, facilitate the folding and insertion of viral proteins onto the ERGIC membrane. The formation of the nucleocapsid complex is overseen by Hsp90, while viral exocytosis requires the cooperation of Hsp90, Hsp70 and Hsp40. Image created with Biorender.
6.2 Virus replication
The central role of Hsp90 in protein complex formation is thought to function as a catalyst for the replication of invading viruses (Wan et al., 2020). Hsp90 acts as a buffer, preventing deleterious folding outcomes of mutated viral proteins (Blagosklonny et al., 1996; Jaeger and Whitesell, 2019). As such, its role in facilitating fold of mutation prone viral proteins is apparent. Both Hsp40 and Hsp70 are virtually involved in virtually all the stages of viral infection in the host cell, as they modulate viral entry, replication, gene expression, and virion assembly and release (Table 2). In addition, the Hsp40 protein, DnaJB6 is also involved in immunomodulation of the foot-and-mouth-disease virus (FDV) infection (Zhang et al., 2019), and it is therefore plausible that these Hsps play a role in viral replication.
6.3 Viral protein maturation and viral assembly
Hsp70/Hsp90 proteins are the main drivers of protein folding within host cells and, inadvertently, they also facilitate the folding of invader viral proteins, enabling them to attain functional states. Protein folding occurs mainly in the cytosol of the infected cell and the Hsp40 chaperones are involved in the recruitment of the viral proteins from the ribosomes. Several of the replication steps of the Coronavirus, such as translation, takes place on the ER membranes (Knoops et al., 2008). Some of the viral proteins are thus, translocated across the ER membrane during translation, while others remain in the cytosol for folding, after which they are recruited to the ER for virion assembly. A distinct set of chaperones control the folding processes of the proteins within the ER lumen. The SARS-CoV2 structural proteins S, 6, 3a and 8a, require folding inside the ER lumen. The increased load of viral proteins in the ER disrupts the ER protein folding machinery, which induces ER stress (Fung et al., 2014; Shi et al., 2019). SARS-COV2 viruses are so dependent on the integrity of the ER folding machinery for their own replication, the virus inhibits the ER stress cascade mechanisms by the activation of the XBP-1 mediated pathway of the UPR, thereby preventing apoptosis. This is potentially mediated by the E protein; a 76 amino acid protein that oligomerises to form an ion conductive pore in the ER membranes, which restores the Ca2+ imbalance generated during ER stress (Torres et al., 2006). Viral proteins heavily depend on Hsp90 for their folding, assembly, and maturation, which partly accounts for the induction of Hsp90 expression (Ha et al., 2020).
7 Role of heat shock proteins in inflammation
SARS-CoVs cause ARDS (Reizine et al., 2021), hemophagocytic syndrome (Yang et al., 2021), lymphoid depletion (Silverstein et al., 2022) and skeletal muscle fibre necrosis (Suh et al., 2021), which are all consequences of elevated levels of pro-inflammatory cytokines. Although predominantly expressed and found intracellularly, Hsp90 and Hsp70 were reportedly found in the extracellular space surrounding both stressed and non-stressed cells (Tsutsumi and Neckers, 2007). Surface exposed Hsp90 is thought to be involved in immunomodulation and facilitates the internalisation of viruses such as dengue virus (DENV), infectious bursal disease virus (IBDV) and influenza A virus (Reyes-del Valle et al., 2005; Cabrera-Hernandez et al., 2007; Wang X. et al., 2020). In line with recent evidence, SARS-CoV2 has been linked to the stimulation of stress proteins (Gadotti et al., 2021) which may potentially facilitate virus internalisation and immunomodulation. SARS-CoV2 infection potentially utilises molecular mimicry to imitate host cell surface receptors facilitating entry and evading the host immune response (Angileri et al., 2020; Cappello, 2020; Cappello et al., 2020; Hightower and Santoro, 2020; Kasperkiewicz, 2021). The molecular mimicry of human cell surface-expressed molecules could result in the expression of antibodies that cross-react with human proteins. This culminates in an autoimmune response from the host (Burgio et al., 2021). Hsps are some of the molecules that share epitopes with microbial counterparts and are often the targets for molecular mimicry (Burgio et al., 2021). It was reported that some Hsps share both immunogenic and antigenic epitopes with SARS-CoV2 viral proteins (Marino Gammazza et al., 2020). This partly explains the cause of an increase in autoimmunity cases in SARS-CoV2 patients.
The cytokine storm that results from a SARS-CoV2 infection is the major cause of lung damage resulting in mortality (Song et al., 2020). A cytokine storm refers to the excessive production of proinflammatory cytokines such as interleukins 1 and 6 (IL-1 and IL-6), tumour necrosis factor α (TNF-α) and interferon -y (Huang et al., 2005; Copaescu et al., 2020). The release of these cytokines triggers a flood of immune cells to the infection site, including T cells, macrophages, and neutrophils, among others. This excessive immune response results in tissue and organ damage, which may ultimately lead to organ failure and, by extension, untimely death (Ragab et al., 2020).
Hsps facilitate the presentation of antigens by the major histocompatibility complex (MHC-1) on the surface of coronavirus infected cells, for clearance by the NK and CD8+T cell subsets (Moseley, 2000; Furuta and Eguchi, 2020; Neukirch et al., 2020). Hsp90 was shown to not only function as chaperone, but also as an antigen-presenting molecule during lymphocytic choriomeningitis viral infection (Basta et al., 2005). In addition, Hsp90 immunomodulates the host response to Sendai virus infection by regulating the activation of interferon regulatory factor 3 and TBK-1 stabilization of Sendai virus (Yang et al., 2009). Generally, Hsps are upregulated to assist in the assembly and folding of numerous immune system antigen-recognition proteins. These include molecules such as immunoglobulins, T-cell receptors and components of the MHC (Zugel and Kaufmann, 1999). Furthermore, the ER-resident Grp94, BiP and calnexin, are involved in the assembly of antibody light and heavy chains in the ER lumen (Zugel and Kaufmann, 1999). It has also been suggested that Hsps may take part in antigen delivery to MHC proteins, due to their promiscuity in binding a wide range of similar peptides (Zininga et al., 2018). These diverse roles Hsps make them important immune modulators that promote viral infection.
8 Conclusion and future perspectives
Hsp90 plays a central role in cellular protein quality control. Inhibition of the Hsp90 system affects various signalling pathways, making it an attractive drug target in viral infections. Additionally, the high mutation rates of RNA viruses further support targeting Hsp90 as an antiviral candidate. In many viral infections, Hsp90 was shown to be an important chaperone that functions in facilitating viral protein folding, replication, transport, and assembly (Geller et al., 2013; Geller et al., 2018; Wyler et al., 2021). Most Hsp90 inhibitors were initially identified as anticancer agents and some of these are being repurposed for use against viral infections (Ramos and Ayinde, 2021; Zhao et al., 2022). Hsp90 inhibitors are potential broad action antivirals, as they act on several viruses by blocking their replication and stimulating apoptosis in infected host cells. It was recently reported that Hsp90 inhibitors prevented endothelial barrier damage in lung tissues and reduced SARS-CoV2 replication (Kubra et al., 2020; Lubkowska et al., 2021; Wyler et al., 2021). Another study hypothesised that Hsp70 and Hsp90 proteins potentially bind to the ACE2 receptors, masking the receptors and inhibiting binding to SARS-CoV2 viruses, resulting in reduced viral host cell entry (Alreshidi et al., 2021). These Hsp90 inhibitors exhibiting potent antiviral activity were reportedly effective at lower concentrations compared to the dosages required in cancer treatments. Therefore, there is merit in targeting the Hsp system, as the shorter acute nature of viral infection implies the use of much less total dosage compared to that required to treat cancer. Taken together, the use of Hsp inhibitors offers promising prospects as combinational treatments in controlling COVID-19.
Author contributions
Conceptualization, TZ; writing-original draft preparation, CC, MS and TZ; writing-review and editing, CC, MS, VM, AS, and TZ; supervision, TZ; funding acquisition, TZ. All authors have read and agreed to the published version of the manuscript.
Funding
This work is based on the research supported in part by the Department of Science and Technology/National Research Foundation (NRF) of South Africa (Grant numbers 129401 and 145,405) awarded to TZ, (Grant number 131724) awarded to MLS and (Grant number 140943) awarded to CC.
Acknowledgments
The authors would like to acknowledge Stellenbosch University Sub-Committee B and the ECAD for support to TZ. We thank Thomas Tipih and Jared Reece Prinsloo for their critical reading of this manuscript.
Conflict of interest
The authors declare that the research was conducted in the absence of any commercial or financial relationships that could be construed as a potential conflict of interest.
Publisher’s note
All claims expressed in this article are solely those of the authors and do not necessarily represent those of their affiliated organizations, or those of the publisher, the editors and the reviewers. Any product that may be evaluated in this article, or claim that may be made by its manufacturer, is not guaranteed or endorsed by the publisher.
References
Adams, B. M., Oster, M. E., and Hebert, D. N. (2019). Protein quality control in the endoplasmic reticulum. Protein J. 38, 317–329. doi:10.1007/s10930-019-09831-w
Agostini, I., Popov, S., Li, J., Dubrovsky, L., Hao, T., and Bukrinsky, M. (2000). Heat-shock protein 70 can replace viral protein R of HIV-1 during nuclear import of the viral preintegration complex. Exp. Cell Res. 259 (2), 398–403. doi:10.1006/excr.2000.4992
Alderson, T. R., Kim, J. H., and Markley, J. L. (2016). Dynamical structures of Hsp70 and hsp70-hsp40 complexes. Structure 24 (7), 1014–1030. doi:10.1016/j.str.2016.05.011
Alreshidi, F. S., Ginawi, I. A., Hussain, M. A., and Arif, J. M. (2021). Piperaquine- and aspirin- mediated protective role of Hsp70 and Hsp90 as modes to strengthen the natural immunity against potent SARS-CoV-2. Biointerface Res. Appl. Chem. 11, 12364–12379. doi:10.33263/BRIAC114.1236412379
Amawi, K. F., Al-Mazari, I. S., Alsarhan, A., Alhamad, H. Q. M., and Alkhatib, A. J. (2019). Diabetes upregulates the expression of HSP90 and downregulates HSP70 in the liver of diabetic rats. Comp. Clin. Path. 28 (2), 473–478. doi:10.1007/s00580-019-02902-5
Andersen, K. G., Rambaut, A., Lipkin, W. I., Holmes, E. C., and Garry, R. F. (2020). The proximal origin of SARS-CoV-2. Nat. Med. 26 (4), 450–452. doi:10.1038/s41591-020-0820-9
Andreasson, C., Fiaux, J., Rampelt, H., Mayer, M. P., and Bukau, B. (2008). Hsp110 is a nucleotide-activated exchange factor for Hsp70. J. Biol. Chem. 283 (14), 8877–8884. doi:10.1074/jbc.M710063200
Angileri, F., Angileri, F., Légaré, S., Gammazza, A. M., de Macario, E. C., Macario, A. J., et al. (2020). Is molecular mimicry the culprit in the autoimmune haemolytic anaemia affecting patients with COVID-19? Br. J. Haematol. 190, e92–e93. doi:10.1111/bjh.16883
Ano, Y., Sakudo, A., Kimata, T., Uraki, R., Sugiura, K., and Onodera, T. (2010). Oxidative damage to neurons caused by the induction of microglial NADPH oxidase in encephalomyocarditis virus infection. Neurosci. Lett. 469 (1), 39–43. doi:10.1016/j.neulet.2009.11.040
Appenzeller-Herzog, C., and Ellgaard, L. (2008). The human PDI family: Versatility packed into a single fold. Biochim. Biophys. Acta 1783 (4), 535–548. doi:10.1016/j.bbamcr.2007.11.010
Araki, K., and Nagata, K. (2012). Protein folding and quality control in the ER. Cold Spring Harb. Perspect. Biol. 4, a015438. doi:10.1101/cshperspect.a015438
Archer, A. E., Von Schulze, A. T., and Geiger, P. C. (2018). Exercise, heat shock proteins and insulin resistance. Philos. Trans. R. Soc. Lond. B Biol. Sci. 373 (1738), 20160529. doi:10.1098/rstb.2016.0529
Bachman, A. B., Keramisanou, D., Xu, W., Beebe, K., Moses, M. A., Vasantha Kumar, M. V., et al. (2018). Phosphorylation induced cochaperone unfolding promotes kinase recruitment and client class-specific Hsp90 phosphorylation. Nat. Commun. 9 (1), 265. doi:10.1038/s41467-017-02711-w
Bartz, S. R., Pauza, C. D., Ivanyi, J., Jindal, S., Welch, W. J., and Malkovsky, M. (1994). An Hsp60 related protein is associated with purified HIV and SIV. J. Med. Primatol. 23 (2-3), 151–154. doi:10.1111/j.1600-0684.1994.tb00116.x
Basta, S., Stoessel, R., Basler, M., van den Broek, M., and Groettrup, M. (2005). Cross-presentation of the long-lived lymphocytic choriomeningitis virus nucleoprotein does not require neosynthesis and is enhanced via heat shock proteins. J. Immunol. 175 (2), 796–805. doi:10.4049/jimmunol.175.2.796
Batra, J., Tripathi, S., Kumar, A., Katz, J. M., Cox, N. J., Lal, R. B., et al. (2016). Human Heat shock protein 40 (Hsp40/DnaJB1) promotes influenza A virus replication by assisting nuclear import of viral ribonucleoproteins. Sci. Rep. 6 (1), 19063. doi:10.1038/srep19063
Bi, S., Hong, P. W., Lee, B., and Baum, L. G. (2011). Galectin-9 binding to cell surface protein disulfide isomerase regulates the redox environment to enhance T-cell migration and HIV entry. Proc. Natl. Acad. Sci. U. S. A. 108 (26), 10650–10655. doi:10.1073/pnas.1017954108
Biancatelli, R. M. L. C., Solopov, P. A., Gregory, B., Khodour, Y., and Catravas, J. D. (2022). HSP90 inhibitors modulate SARS-CoV-2 spike protein subunit 1-induced human pulmonary microvascular endothelial activation and barrier dysfunction. Front. Physiol. 13, 812199. doi:10.3389/fphys.2022.812199
Biebl, M. M., Riedl, M., and Buchner, J. (2020). Hsp90 Co-chaperones form plastic genetic networks adapted to client maturation. Cell Rep. 32 (8), 108063. doi:10.1016/j.celrep.2020.108063
Bijur, G. N., De Sarno, P., and Jope, R. S. (2000). Glycogen synthase kinase-3beta facilitates staurosporine- and heat shock-induced apoptosis protection by lithium. J. Biol. Chem. 275 (11), 7583–7590. doi:10.1074/jbc.275.11.7583
Blagosklonny, M. V., Toretsky, J., Bohen, S., and Neckers, L. (1996). Mutant conformation of p53 translated in vitro or in vivo requires functional HSP90. Proc. Natl. Acad. Sci. U. S. A. 93 (16), 8379–8383. doi:10.1073/pnas.93.16.8379
Blüher, M. (2019). Obesity: Global epidemiology and pathogenesis. Nat. Rev. Endocrinol. 15 (5), 288–298. doi:10.1038/s41574-019-0176-8
Braakman, I., and Hebert, D. N. (2021). Protein folding in the endoplasmic reticulum. Cold Spring Harb. Perspect. Biol. 2013, a013201–18. doi:10.1101/cshperspect.a013201
Bruxel, M. A., Tavares, A. M. V., Neto, L. D. Z., de Souza Borges, V., Schroeder, H. T., Bock, P. M., et al. (2019). Chronic whole-body heat treatment relieves atherosclerotic lesions, cardiovascular and metabolic abnormalities, and enhances survival time restoring the anti-inflammatory and anti-senescent heat shock response in mice. Biochimie 156, 33–46. doi:10.1016/j.biochi.2018.09.011
Burgio, S., Conway de Macario, E., Macario, A. J., and Cappello, F. (2021). SARS-CoV-2 in patients with cancer: Possible role of mimicry of human molecules by viral proteins and the resulting anti-cancer immunity. Cell Stress Chaperones 26 (4), 611–616. doi:10.1007/s12192-021-01211-7
Cabrera-Hernandez, A., Thepparit, C., Suksanpaisan, L., and Smith, D. R. (2007). Dengue virus entry into liver (HepG2) cells is independent of hsp90 and hsp70. J. Med. Virol. 79 (4), 386–392. doi:10.1002/jmv.20786
Cao, M., Wei, C., Zhao, L., Wang, J., Jia, Q., Wang, X., et al. (2014). DnaJA1/Hsp40 is co-opted by influenza A virus to enhance its viral RNA polymerase activity. J. Virol. 88 (24), 14078–14089. doi:10.1128/JVI.02475-14
Cappello, F., Cappello, F., Marino Gammazza, A., Dieli, F., Conway de Macario, E., and Macario, A. J. (2020). Does SARS-CoV-2 trigger stress-induced autoimmunity by molecular mimicry? A hypothesis. J. Clin. Med. 9 (7), E2038. doi:10.3390/jcm9072038
Cappello, F. (2020). COVID-19 and molecular mimicry: The Columbus’ egg? J. Clin. Neurosci. 77, 246. doi:10.1016/j.jocn.2020.05.015
Carlos, A. J., Ha, D. P., Yeh, D. W., Van Krieken, R., Tseng, C. C., Zhang, P., et al. (2021). The chaperone GRP78 is a host auxiliary factor for SARS-CoV-2 and GRP78 depleting antibody blocks viral entry and infection. J. Biol. Chem. 296, 100759. doi:10.1016/j.jbc.2021.100759
Cedrés, S., Felip, E., Cruz, C., Martinez de Castro, A., Pardo, N., Navarro, A., et al. (2018). Activity of HSP90 inhibiton in a metastatic lung cancer patient with a germline BRCA1 mutation. J. Natl. Cancer Inst. 110 (8), 914–917. doi:10.1093/jnci/djy012
Celli, B. R., and Wedzicha, J. A. (2019). Update on clinical aspects of chronic obstructive pulmonary disease. N. Engl. J. Med. 381, 1257–1266. doi:10.1056/NEJMra1900500
Chakafana, G., and Shonhai, A. (2021). The role of non-canonical Hsp70s (Hsp110/Grp170) in cancer. Cells 10 (2), 254. doi:10.3390/cells10020254
Chakafana, G., Zininga, T., and Shonhai, A. (2019). Comparative structure-function features of Hsp70s of Plasmodium falciparum and human origins. Biophys. Rev. 11 (4), 591–602. doi:10.1007/s12551-019-00563-w
Chakraborty, A., and Edkins, A. L. (2021). HSP90 as a regulator of extracellular matrix dynamics. Biochem. Soc. Trans. 49 (6), 2611–2625. doi:10.1042/BST20210374
Chamberlain, N., and Anathy, V. (2020). Pathological consequences of the unfolded protein response and downstream protein disulphide isomerases in pulmonary viral infection and disease. J. Biochem. 167 (2), 173–184. doi:10.1093/jb/mvz101
Chatterjee, S. (2016). “Chapter two - oxidative stress, inflammation, and disease,” in Oxidative stress and biomaterials. Editors T. Dziubla, and D. A. Butterfield (Academic Press), 35–58.
Chattopadhyay, S., Mukherjee, A., Patra, U., Bhowmick, R., Basak, T., Sengupta, S., et al. (2017). Tyrosine phosphorylation modulates mitochondrial chaperonin Hsp60 and delays rotavirus NSP4-mediated apoptotic signaling in host cells. Cell. Microbiol. 19 (3), e12670. doi:10.1111/cmi.12670
Chen, B., Piel, W. H., Gui, L., Bruford, E., and Monteiro, A. (2005). The HSP90 family of genes in the human genome: Insights into their divergence and evolution. Genomics 86 (6), 627–637. doi:10.1016/j.ygeno.2005.08.012
Chen, J., Song, S., Liu, Y., Liu, D., Lin, Y., Ge, S., et al. (2018). Autoreactive T cells to citrullinated HSP90 are associated with interstitial lung disease in rheumatoid arthritis. Int. J. Rheum. Dis. 21 (7), 1398–1405. doi:10.1111/1756-185X.13316
Chen, Y.-J., Chen, Y.-H., Chow, L.-P., Tsai, Y.-H., Chen, P.-H., Huang, C.-Y. F., et al. (2010). Heat shock protein 72 is associated with the hepatitis C virus replicase complex and enhances viral RNA replication. J. Biol. Chem. 285 (36), 28183–28190. doi:10.1074/jbc.M110.118323
Chen, Y., Liu, Q., and Guo, D. (2020). Emerging coronaviruses: Genome structure, replication, and pathogenesis. J. Med. Virol. 92 (4), 418–423. doi:10.1002/jmv.25681
Cheng, S., and Brooks, C. L. (2013). Viral capsid proteins are segregated in structural fold space. PLoS Comput. Biol. 9 (2), e1002905. doi:10.1371/journal.pcbi.1002905
Chiang, Y., Sheng, W.-H., Shao, P.-L., Chi, Y.-H., Chen, Y.-M., Huang, S.-W., et al. (2014). Large isoform of mammalian relative of dnaj is a major determinant of human susceptibility to HIV-1 infection. EBioMedicine 284, 126–132. doi:10.1016/j.ebiom.2014.10.002
Chichiarelli, S., Altieri, F., Paglia, G., Rubini, E., Minacori, M., and Eufemi, M. (2022). ERp57/PDIA3: New insight. Cell. Mol. Biol. Lett. 27, 12. doi:10.1186/s11658-022-00315-x
Choi, Y. J., Park, J. Y., Lee, H. S., Suh, J., Song, J. Y., Byun, M. K., et al. (2021). Effect of asthma and asthma medication on the prognosis of patients with COVID-19. Eur. Respir. J. 57 (3), 2002226. doi:10.1183/13993003.02226-2020
Cintron, N. S., and Toft, D. (2006). Defining the requirements for Hsp40 and Hsp70 in the Hsp90 chaperone pathway. J. Biol. Chem. 281 (36), 26235–26244. doi:10.1074/jbc.M605417200
Connor, J. H., McKenzie, M. O., Parks, G. D., and Lyles, D. S. (2007). Antiviral activity and RNA polymerase degradation following Hsp90 inhibition in a range of negative strand viruses. Virology 362 (1), 109–119. doi:10.1016/j.virol.2006.12.026
Copaescu, A., Smibert, O., Gibson, A., Phillips, E. J., and Trubiano, J. A. (2020). The role of IL-6 and other mediators in the cytokine storm associated with SARS-CoV-2 infection. J. Allergy Clin. Immunol. 146 (3), 518–534. doi:10.1016/j.jaci.2020.07.001
Cottin, V. (2013). Interstitial lung disease. Eur. Respir. Rev. 22, 26–32. doi:10.1183/09059180.00006812
Craig, E. A. (2018). Hsp70 at the membrane: Driving protein translocation. BMC Biol. 16, 11. doi:10.1186/s12915-017-0474-3
Darby, N. J., and Creighton, T. E. (1995). Functional properties of the individual thioredoxin-like domains of protein disulfide isomerase. Biochemistry 34 (37), 11725–11735. doi:10.1021/bi00037a009
Das, S., Laxminarayana, S. V., Chandra, N., Ravi, V., and Desai, A. (2009). Heat shock protein 70 on Neuro2a cells is a putative receptor for Japanese encephalitis virus. Virology 385 (1), 47–57. doi:10.1016/j.virol.2008.10.025
de Lemos Muller, C. H., Rech, A., Botton, C. E., Schroeder, H. T., Bock, P. M., Farinha, J. B., et al. (2018). Heat-induced extracellular HSP72 release is blunted in elderly diabetic people compared with healthy middle-aged and older adults, but it is partially restored by resistance training. Exp. Gerontol. 111, 180–187. doi:10.1016/j.exger.2018.07.014
DeDiego, M. L., Alvarez, E., Almazán, F., Rejas, M. T., Lamirande, E., Roberts, A., et al. (2007). A severe acute respiratory syndrome coronavirus that lacks the E gene is attenuated in vitro and in vivo. J. Virol. 81 (4), 1701–1713. doi:10.1128/JVI.01467-06
Di Naso, F. C., Rosa Porto, R., Sarubbi Fillmann, H., Maggioni, L., Vontobel Padoin, A., Jacques Ramos, R., et al. (2015). Obesity depresses the anti-inflammatory HSP70 pathway, contributing to NAFLD progression. Obesity 23 (1), 120–129. doi:10.1002/oby.20919
Diwaker, D., Mishra, K. P., Ganju, L., and Singh, S. B. (2015). Protein disulfide isomerase mediates dengue virus entry in association with lipid rafts. Viral Immunol. 28 (3), 153–160. doi:10.1089/vim.2014.0095
Dobmeyer, T. S., Findhammer, S., Dobmeyer, J. M., Klein, S. A., Raffel, B., Hoelzer, D., et al. (1997). Ex vivo induction of apoptosis in lymphocytes is mediated by oxidative stress: Role for lymphocyte loss in HIV infection. Free Radic. Biol. Med. 22 (5), 775–785. doi:10.1016/s0891-5849(96)00403-0
Doms, R. W., and Trono, D. (2000). The plasma membrane as a combat zone in the HIV battlefield. Genes Dev. 14 (21), 2677–2688. doi:10.1101/gad.833300
Dong, J., Guo, L., Liao, Z., Zhang, M., Zhang, M., Wang, T., et al. (2013). Increased expression of heat shock protein 70 in chronic obstructive pulmonary disease. Int. Immunopharmacol. 17 (3), 885–893. doi:10.1016/j.intimp.2013.09.003
Dong, Q., Men, R., Dan, X., Chen, Y., Li, H., Chen, G., et al. (2018). Hsc70 regulates the IRES activity and serves as an antiviral target of enterovirus A71 infection. Antivir. Res. 150, 39–46. doi:10.1016/j.antiviral.2017.11.020
Dragovic, Z., Broadley, S. A., Shomura, Y., Bracher, A., and Hartl, F. U. (2006). Molecular chaperones of the Hsp110 family act as nucleotide exchange factors of Hsp70s. EMBO J. 25 (11), 2519–2528. doi:10.1038/sj.emboj.7601138
Duan, Y., Tang, H., Mitchell-Silbaugh, K., Fang, X., Han, Z., and Ouyang, K. (2020). Heat shock protein 60 in cardiovascular physiology and diseases. Front. Mol. Biosci. 7, 73. doi:10.3389/fmolb.2020.00073
Easton, D. P., Kaneko, Y., and Subjeck, J. R. (2000). The Hsp110 and Grp170 stress proteins: Newly recognized relatives of the Hsp70s’, Cell stress and chaperones. Cell Stress Chaperones 5 (4), 276–290. doi:10.1379/1466-1268(2000)005<0276:thagsp>2.0.co;2
Edkins, A. L., Price, J. T., Pockley, A. G., and Blatch, G. L. (2018). Heat shock proteins as modulators and therapeutic targets of chronic disease: An integrated perspective. Philos. Trans. R. Soc. Lond. B Biol. Sci. 373 (1738), 20160521. doi:10.1098/rstb.2016.0521
Ellgaard, L., and Ruddock, L. W. (2005). The human protein disulphide isomerase family: Substrate interactions and functional properties. EMBO Rep. 6 (1), 28–32. doi:10.1038/sj.embor.7400311
Enriquez, A. S., Rojo, H. M., Bhatt, J. M., Molugu, S. K., Hildenbrand, Z. L., and Bernal, R. A. (2017). The human mitochondrial Hsp60 in the APO conformation forms a stable tetradecameric complex. Cell Cycle 16 (13), 1309–1319. doi:10.1080/15384101.2017.1321180
ERA-EDTA CouncilERACODA Working Group Fliser, D., Fouque, D., Goumenos, D., Massy, Z. A., et al. (2021). Chronic kidney disease is a key risk factor for severe COVID-19: A call to action by the ERA-EDTA. Nephrol. Dial. Transpl. 36 (1), 87–94. PMCID: PMC7771976. doi:10.1093/ndt/gfaa314
Fan, C.-Y., Lee, S., and Cyr, D. M. (2003). Mechanisms for regulation of Hsp70 function by Hsp40. Cell Stress Chaperones 8 (4), 309–316. doi:10.1379/1466-1268(2003)008<0309:mfrohf>2.0.co;2
Fang, D., Haraguchi, Y., Jinno, A., Soda, Y., Shimizu, N., and Hoshino, H. (1999). Heat shock cognate protein 70 is a cell fusion-enhancing factor but not an entry factor for human T-cell lymphotropic virus type I. Biochem. Biophys. Res. Commun. 261 (2), 357–363. doi:10.1006/bbrc.1999.1028
Favatier, F., Bornman, L., Hightower, L. E., Günther, E., and Polla, B. S. (1997). Variation in hsp gene expression and hsp polymorphism: Do they contribute to differential disease susceptibility and stress tolerance. Cell Stress Chaperones 2 (3), 141–155. doi:10.1379/1466-1268(1997)002<0141:vihgea>2.3.co;2
Ferrari, L., Geerts, W. J., van Wezel, M., Kos, R., Konstantoulea, A., van Bezouwen, L. S., et al. (2018). Human chaperones untangle fibrils of the Alzheimer protein Tau. Biorxiv, 426650. doi:10.1101/426650
Fislová, T., Thomas, B., Graef, K. M., and Fodor, E. (2010). Association of the influenza virus RNA polymerase subunit PB2 with the host chaperonin CCT. J. Virol. 84 (17), 8691–8699. doi:10.1128/JVI.00813-10
Fraser, D. D., Cepinskas, G., Slessarev, M., Martin, C., Daley, M., Miller, M. R., et al. (2020). Novel outcome biomarkers identified with targeted proteomic analyses of plasma from critically ill coronavirus disease 2019 patients. Crit. Care Explor. 2 (6), e0189. doi:10.1097/CCE.0000000000000189
Fu, J., Gao, J., Liang, Z., and Yang, D. (2020). PDI-regulated disulfide bond formation in protein folding and biomolecular assembly. Molecules 26 (1), 171. doi:10.3390/molecules26010171
Fung, T. S., Huang, M., and Liu, D. X. (2014). Coronavirus-induced ER stress response and its involvement in regulation of coronavirus–host interactions. Virus Res. 194, 110–123. doi:10.1016/j.virusres.2014.09.016
Furuta, K., and Eguchi, T. (2020). “Roles of HSP on antigen presentation,” in Heat shock proteins in human diseases (Cham: Springer), 275–280.
Gadotti, A. C., Lipinski, A. L., Vasconcellos, F. T., Marqueze, L. F., Cunha, E. B., Campos, A. C., et al. (2021). Susceptibility of the patients infected with Sars-Cov2 to oxidative stress and possible interplay with severity of the disease. Free Radic. Biol. Med. 165, 184–190. doi:10.1016/j.freeradbiomed.2021.01.044
Gallina, A., Hanley, T. M., Mandel, R., Trahey, M., Broder, C. C., Viglianti, G. A., et al. (2002). Inhibitors of protein-disulfide isomerase prevent cleavage of disulfide bonds in receptor-bound glycoprotein 120 and prevent HIV-1 entry. J. Biol. Chem. 277 (52), 50579–50588. doi:10.1074/jbc.M204547200
Gámez, A., Yuste-Checa, P., Brasil, S., Briso-Montiano, Á., Desviat, L. R., Ugarte, M., et al. (2018). Protein misfolding diseases: Prospects of pharmacological treatment. Clin. Genet. 93 (3), 450–458. doi:10.1111/cge.13088
García-Dorival, I., Wu, W., Armstrong, S. D., Barr, J. N., Carroll, M. W., Hewson, R., et al. (2016). Elucidation of the cellular interactome of Ebola virus nucleoprotein and identification of therapeutic targets. J. Proteome Res. 15 (12), 4290–4303. doi:10.1021/acs.jproteome.6b00337
Geller, R., Andino, R., and Frydman, J. (2013). Hsp90 inhibitors exhibit resistance-free antiviral activity against respiratory syncytial virus. PloS One 8 (2), e56762. doi:10.1371/journal.pone.0056762
Geller, R., Pechmann, S., Acevedo, A., Andino, R., and Frydman, J. (2018). Hsp90 shapes protein and RNA evolution to balance trade-offs between protein stability and aggregation. Nat. Commun. 9 (1), 1781. doi:10.1038/s41467-018-04203-x
Geller, R., Taguwa, S., and Frydman, J. (2012). Broad action of Hsp90 as a host chaperone required for viral replication. Biochim. Biophys. Acta 1823 (3), 698–706. doi:10.1016/j.bbamcr.2011.11.007
Geller, R., Vignuzzi, M., Andino, R., and Frydman, J. (2007). Evolutionary constraints on chaperone-mediated folding provide an antiviral approach refractory to development of drug resistance. Genes Dev. 21 (2), 195–205. doi:10.1101/gad.1505307
Gerayeli, F. V., Milne, S., Cheung, C., Li, X., Yang, C. W. T., Tam, A., et al. (2021). COPD and the risk of poor outcomes in COVID-19: A systematic review and meta-analysis. EClinicalMedicine 33, 100789. doi:10.1016/j.eclinm.2021.100789
Giri, B., Sethi, V., Modi, S., Garg, B., Banerjee, S., Saluja, A., et al. (2017). Heat shock protein 70 in pancreatic diseases: Friend or foe. J. Surg. Oncol. 116 (1), 114–122. doi:10.1002/jso.24653
Glebov, O. O. (2020). Understanding SARS-Co-V2 endocytosis for COVID-19 drug repurposing. FEBS J. 287 (17), 3664–3671. doi:10.1111/febs.15369
Gordon, D. E., Jang, G. M., Bouhaddou, M., Xu, J., Obernier, K., White, K. M., et al. (2020). A SARS-CoV-2 protein interaction map reveals targets for drug repurposing. Nature 583 (7816), 459–468. doi:10.1038/s41586-020-2286-9
Graef, K. M., Vreede, F. T., Lau, Y. F., McCall, A. W., Carr, S. M., Subbarao, K., et al. (2010). The PB2 subunit of the influenza virus RNA polymerase affects virulence by interacting with the mitochondrial antiviral signaling protein and inhibiting expression of beta interferon. J. Virol. 84 (17), 8433–8445. doi:10.1128/JVI.00879-10
Grundtman, C., Kreutmayer, S. B., Almanzar, G., Wick, M. C., and Wick, G. (2011). Heat shock protein 60 and immune inflammatory responses in atherosclerosis. Arterioscler. Thromb. Vasc. Biol. 31 (5), 960–968. doi:10.1161/ATVBAHA.110.217877
Guihur, A., Rebeaud, M. E., Fauvet, B., Tiwari, S., Weiss, Y. G., and Goloubinoff, P. (2020). Moderate fever cycles as a potential mechanism to protect the respiratory system in COVID-19 patients. Front. Med. 7, 564170. doi:10.3389/fmed.2020.564170
Guo, K., Kang, N. X., Li, Y., Sun, L., Gan, L., Cui, F. J., et al. (2009). Regulation of HSP27 on NF-kappaB pathway activation may be involved in metastatic hepatocellular carcinoma cells apoptosis. BMC Cancer 9 (1), 100–110. doi:10.1186/1471-2407-9-100
Ha, D. P., Van Krieken, R., Carlos, A. J., and Lee, A. S. (2020). The stress-inducible molecular chaperone GRP78 as potential therapeutic target for coronavirus infection. J. Infect. 81 (3), 452–482. doi:10.1016/j.jinf.2020.06.017
Haslbeck, M., Weinkauf, S., and Buchner, J. (2019). Small heat shock proteins: Simplicity meets complexity. J. Biol. Chem. 294 (6), 2121–2132. doi:10.1074/jbc.REV118.002809
Haßdenteufel, S., Johnson, N., Paton, A. W., Paton, J. C., High, S., and Zimmermann, R. (2018). Chaperone-mediated Sec61 channel gating during ER import of small precursor proteins overcomes Sec61 inhibitor-reinforced energy barrier. Cell Rep. 23, 1373–1386. doi:10.1016/j.celrep.2018.03.122
Henderson, B., and Pockley, A. G. (2012). Proteotoxic stress and circulating cell stress proteins in the cardiovascular diseases. Cell Stress Chaperones 17 (3), 303–311. doi:10.1007/s12192-011-0318-y
Hetz, C., Bernasconi, P., Fisher, J., Lee, A. H., Bassik, M. C., Antonsson, B., et al. (2006). Proapoptotic BAX and BAK modulate the unfolded protein response by a direct interaction with IRE1alpha. Science 312 (5773), 572–576. doi:10.1126/science.1123480
Hetz, C., and Papa, F. R. (2018). The unfolded protein response and cell fate control. Mol. Cell 69, 169–181. doi:10.1016/j.molcel.2017.06.017
Hidalgo, P., Valdés, M., and González, R. A. (2021). Molecular biology of coronaviruses: An overview of virus-host interactions and pathogenesis. Bol. Med. Hosp. Infant. Mex. 78 (1), 41–58. doi:10.24875/BMHIM.20000249
Hightower, L. E., and Santoro, M. G. (2020). Coronaviruses and stress: From cellular to global. Cell Stress Chaperones 25 (5), 701–705. doi:10.1007/s12192-020-01155-4
Hirayama, E., Atagi, H., Hiraki, A., and Kim, J. (2004). Heat shock protein 70 is related to thermal inhibition of nuclear export of the influenza virus ribonucleoprotein complex. J. Virol. 78 (3), 1263–1270. doi:10.1128/jvi.78.3.1263-1270.2004
Hoffmann, M., Kleine-Weber, H., Schroeder, S., Krüger, N., Herrler, T., Erichsen, S., et al. (2020). SARS-CoV-2 cell entry depends on ACE2 and TMPRSS2 and is blocked by a clinically proven protease inhibitor. Cell 181 (2), 271–280. e8. doi:10.1016/j.cell.2020.02.052
Huang, C., Wang, Y., Li, X., Ren, L., Zhao, J., Hu, Y., et al. (2020). Clinical features of patients infected with 2019 novel coronavirus in Wuhan, China. Lancet 395 (10223), 497–506. doi:10.1016/S0140-6736(20)30183-5
Huang, K. J., Su, I. J., Theron, M., Wu, Y. C., Lai, S. K., Liu, C. C., et al. (2005). An interferon-γ-related cytokine storm in SARS patients. J. Med. Virol. 75 (2), 185–194. doi:10.1002/jmv.20255
Hulgan, T., Morrow, J., D'Aquila, R. T., Raffanti, S., Morgan, M., Rebeiro, P., et al. (2003). Oxidant stress is increased during treatment of human immunodeficiency virus infection. Clin. Infect. Dis. 37, 1711–1717. doi:10.1086/379776
Ikebe, E., Kawaguchi, A., Tezuka, K., Taguchi, S., Hirose, S., Matsumoto, T., et al. (2014). A novel HSP90 inhibitor, 17-DMAG, induces Tax down-regulation and its oral administration to ATL-model mice intervenes against the infiltration property of the ATL-like lymphocytes and provides extended survival period. Retrovirology 11 (1), P44. doi:10.1186/1742-4690-11-S1-P44
Ishida, R., Okamoto, T., Motojima, F., Kubota, H., Takahashi, H., Tanabe, M., et al. (2018). Physicochemical properties of the mammalian molecular chaperone HSP60. Int. J. Mol. Sci. 19 (2), 489. doi:10.3390/ijms19020489
Ito, S., and Nagata, K. (2019). Roles of the endoplasmic reticulum‐resident, collagen-specific molecular chaperone Hsp47 in vertebrate cells and human disease. J. Biol. Chem. 294 (6), 2133–2141. doi:10.1074/jbc.TM118.002812
Ivanov, A. V., Bartosch, B., and Isaguliants, M. G. (2017). Oxidative stress in infection and consequent disease. Oxid. Med. Cell. Longev. 2017, 3496043. doi:10.1155/2017/3496043
Jackson, S. E. (2012). “Hsp90: Structure and function,” in Molecular chaperones (Springer), 155–240.
Jaeger, A. M., and Whitesell, L. (2019). HSP90: Enabler of cancer adaptation. Annu. Rev. Cancer Biol. 3, 275–297. doi:10.1146/annurev-cancerbio-030518-055533
Jakovac, H. (2020). COVID-19 and hypertension: Is the HSP60 culprit for the severe course and worse outcome? Am. J. Physiol. Heart Circ. Physiol. 319 (4), H793–H796. doi:10.1152/ajpheart.00506.2020
Jaroszyński, A., Jaroszyńska, A., Zaborowski, T., Drelich-Zbroja, A., Zapolski, T., and Dabrowski, W. (2018). Serum heat shock protein 27 levels predict cardiac mortality in hemodialysis patients. BMC Nephrol. 19, 359. doi:10.1186/s12882-018-1157-1
Jiang, Q., and Shen, X. (2020). Research progress of heat shock protein 90 and hepatocellular carcinoma. Int. J. Clin. Med. 11 (2), 43–52. doi:10.4236/ijcm.2020.112005
Jomaa, A., Gamerdinger, M., Hsieh, H. H., Wallisch, A., Chandrasekaran, V., Ulusoy, Z., et al. (2022). Mechanism of signal sequence handover from NAC to SRP on ribosomes during ER-protein targeting. Science 375 (6583), 839–844. doi:10.1126/science.abl6459
Kaimal, J. M., Kandasamy, G., Gasser, F., and Andréasson, C. (2017). Coordinated Hsp110 and Hsp104 activities power protein disaggregation in Saccharomyces cerevisiae. Mol. Cell. Biol. 37 (11), e00027–17. doi:10.1128/MCB.00027-17
Kampinga, H. H., Hageman, J., Vos, M. J., Kubota, H., Tanguay, R. M., Bruford, E. A., et al. (2009). Guidelines for the nomenclature of the human heat shock proteins. Cell Stress Chaperones 14 (1), 105–111. doi:10.1007/s12192-008-0068-7
Kang, S. M., Kim, J.-H., Lee, W., Kim, G.-W., Lee, K.-H., Choi, K.-Y., et al. (2009). Interaction of hepatitis C virus core protein with Hsp60 triggers the production of reactive oxygen species and enhances TNF-α-mediated apoptosis. Cancer Lett. 279 (2), 230–237. doi:10.1016/j.canlet.2009.02.003
Karlas, A., Machuy, N., Shin, Y., Pleissner, K.-P., Artarini, A., Heuer, D., et al. (2010). Genome-wide RNAi screen identifies human host factors crucial for influenza virus replication. Nature 463 (7282), 818–822. doi:10.1038/nature08760
Kasperkiewicz, M. (2021). Covid-19, heat shock proteins, and autoimmune bullous diseases: A potential link deserving further attention. Cell Stress Chaperones 26 (1), 1–2. doi:10.1007/s12192-020-01180-3
Katoh, H., Kubota, T., Kita, S., Nakatsu, Y., Aoki, N., Mori, Y., et al. (2015). Heat shock protein 70 regulates degradation of the mumps virus phosphoprotein via the ubiquitin-proteasome pathway. J. Virol. 89 (6), 3188–3199. doi:10.1128/JVI.03343-14
Katsogiannou, M., Andrieu, C., and Rocchi, P. (2014). Heat shock protein 27 phosphorylation state is associated with cancer progression. Front. Genet. 5, 346. doi:10.3389/fgene.2014.00346
Khachatoorian, R., Ganapathy, E., Ahmadieh, Y., Wheatley, N., Sundberg, C., Jung, C. L., et al. (2014). The NS5A-binding heat shock proteins HSC70 and HSP70 play distinct roles in the hepatitis C viral life cycle. Virology 454, 118–127. doi:10.1016/j.virol.2014.02.016
Khachatoorian, R., Ruchala, P., Waring, A., Jung, C. L., Ganapathy, E., Wheatley, N., et al. (2015). Structural characterization of the HSP70 interaction domain of the hepatitis C viral protein NS5A. Virology 475, 46–55. doi:10.1016/j.virol.2014.10.011
Khadir, A., Kavalakatt, S., Cherian, P., Warsame, S., Abubaker, J. A., Dehbi, M., et al. (2018). Physical exercise enhanced heat shock protein 60 expression and attenuated inflammation in the adipose tissue of human diabetic obese. Front. Endocrinol. 9, 16. doi:10.3389/fendo.2018.00016
Kim, D., Lee, J. Y., Yang, J. S., Kim, J. W., Kim, V. N., and Chang, H. (2020). The architecture of SARS-CoV-2 transcriptome. Cell 181 (4), 914–921. doi:10.1016/j.cell.2020.04.011
Kitamura, M. (2008). Endoplasmic reticulum stress in the kidney. Clin. Exp. Nephrol. 12, 317–325. doi:10.1007/s10157-008-0060-7
Klappa, P., uddock, L. W., Darby, N. J., and Freedman, R. B. (1998). The b′ domain provides the principal peptide-binding site of protein disulfide isomerase but all domains contribute to binding of misfolded proteins. EMBO J. 17 (4), 927–935. doi:10.1093/emboj/17.4.927
Klumperman, J., Locker, J. K., Meijer, A., Horzinek, M. C., Geuze, H. J., and Rottier, P. J. (1994). Coronavirus M proteins accumulate in the Golgi complex beyond the site of virion budding. J. Virol. 68 (10), 6523–6534. doi:10.1128/JVI.68.10.6523-6534.1994
Kmiecik, S. W., and Mayer, M. P. (2022). Molecular mechanisms of heat shock factor 1 regulation. Trends biochem. Sci. 47, 218–234. doi:10.1016/j.tibs.2021.10.004
Knobil, K., Choi, A. M., Weigand, G. W., and Jacoby, D. B. (1998). Role of oxidants in influenza virus-induced gene expression. Am. J. Physiol. 274 (1), L134–L142. doi:10.1152/ajplung.1998.274.1.L134
Knoops, K., Kikkert, M., Van Den Worm, , Sjoerd, H. E., van der Meer, Y., Koster, A. J., et al. (2008). SARS-coronavirus replication is supported by a reticulovesicular network of modified endoplasmic reticulum. PLoS Biol. 6 (9), e226. doi:10.1371/journal.pbio.0060226
Ko, S. H., Liau, Y. J., Chi, Y. H., Lai, M. J., Chiang, Y. P., Lu, C. Y., et al. (2019). Interference of DNAJB6/MRJ isoform switch by morpholino inhibits replication of HIV-1 and RSV. Mol. Ther. Nucleic Acids 14, 251–261. doi:10.1016/j.omtn.2018.12.001
Korenaga, M., Wang, T., Li, Y., Showalter, L. A., Chan, T., Sun, J., et al. (2005). Hepatitis C virus core protein inhibits mitochondrial electron transport and increases reactive oxygen species (ROS) production. J. Biol. Chem. 280 (45), 37481–37488. doi:10.1074/jbc.M506412200
Kozlov, G., and Gehring, K. (2020). Calnexin cycle–structural features of the ER chaperone system. FEBS J. 287 (20), 4322–4340. doi:10.1111/febs.15330
Kramer, B., Ferrari, D. M., Klappa, P., Pohlmann, N., and Soling, H. D. (2001). Functional roles and efficiencies of the thioredoxin boxes of calcium-binding proteins 1 and 2 in protein folding. Biochem. J. 357 (1), 83–95. doi:10.1042/0264-6021:3570083
Kranz, P., Neumann, F., Wolf, A., Classen, F., Pompsch, M., Ocklenburg, T., et al. (2017). PDI is an essential redox-sensitive activator of PERK during the unfolded protein response (UPR). Cell Death Dis. 8 (8), e2986e2986. doi:10.1038/cddis.2017.369
Krause, M., Gerchman, F., and Friedman, R. (2020). Coronavirus infection (SARS-CoV-2) in obesity and diabetes comorbidities: Is heat shock response determinant for the disease complications? Diabetol. Metab. Syndr. 12 (1), 63. doi:10.1186/s13098-020-00572-w
Krishnan-Sivadoss, I., Mijares-Rojas, I. A., Villarreal-Leal, R. A., Torre-Amione, G., Knowlton, A. A., and Guerrero-Beltrán, C. E. (2021). Heat shock protein 60 and cardiovascular diseases: An intricate love-hate story. Med. Res. Rev. 41, 29–71. doi:10.1002/med.21723
Kubra, K.-T., Uddin, M. A., Akhter, M. S., and Barabutis, N. (2020). Hsp90 inhibitors induce the unfolded protein response in bovine and mice lung cells. Cell. Signal. 67, 109500. doi:10.1016/j.cellsig.2019.109500
Kumar, P., Gaur, P., Kumari, R., and Lal, S. K. (2019). Influenza A virus neuraminidase protein interacts with Hsp90, to stabilize itself and enhance cell survival. J. Cell. Biochem. 120 (4), 6449–6458. doi:10.1002/jcb.27935
Kuppalli, K., and Rasmussen, A. L. (2020). A glimpse into the eye of the COVID-19 cytokine storm. EBioMedicine 55, 102789. doi:10.1016/j.ebiom.2020.102789
Lauwers, E., Wang, Y. C., Gallardo, R., Van der Kant, R., Michiels, E., Swerts, J., et al. (2018). Hsp90 mediates membrane deformation and exosome release. Mol. Cell 71 (5), 689–702. doi:10.1016/j.molcel.2018.07.016
Lebherz-Eichinger, D., Ankersmit, H. J., Hacker, S., Hetz, H., Kimberger, O., Schmidt, E. M., et al. (2012). HSP27 and HSP70 serum and urine levels in patients suffering from chronic kidney disease. Clin. Chim. Acta. 413 (1-2), 282–286. doi:10.1016/j.cca.2011.10.010
Lee, J. H., Gao, J., Kosinski, P. A., Elliman, S. J., Hughes, T. E., Gromada, J., et al. (2013). Heat shock protein 90 (HSP90) inhibitors activate the heat shock factor 1 (HSF1) stress response pathway and improve glucose regulation in diabetic mice. Biochem. Biophys. Res. Commun. 430 (3), 1109–1113. doi:10.1016/j.bbrc.2012.12.029
Lee, T. G., Tang, N., Thompson, S., Miller, J., and Katze, M. G. (1994). The 58, 000-dalton cellular inhibitor of the interferon-induced double-stranded RNA-activated protein kinase (PKR) is a member of the tetratricopeptide repeat family of proteins. Mol. Cell. Biol. 14 (4), 2331–2342. doi:10.1128/mcb.14.4.2331
Lehr, S., Hartwig, S., and Sell, H. (2012). Adipokines: A treasure trove for the discovery of biomarkers for metabolic disorders. Proteomics. Clin. Appl. 6 (1–2), 91–101. doi:10.1002/prca.201100052
Leng, A. M., Liu, T., Yang, J., Cui, J. F., Li, X. H., Zhu, Y. N., et al. (2012). The apoptotic effect and associated signalling of HSP90 inhibitor 17-DMAG in hepatocellular carcinoma cells. Cell Biol. Int. 36 (10), 893–899. doi:10.1042/CBI20110473
Leone, G., Coffey, M. C., Gilmore, R., Duncan, R., Maybaum, L., and Lee, P. W. (1996). C-terminal trimerization, but not N-terminal trimerization, of the reovirus cell attachment protein is a posttranslational and Hsp70/ATP-dependent process. J. Biol. Chem. 271 (14), 8466–8471. doi:10.1074/jbc.271.14.8466
Liao, S. L., Raung Sl Fau - Chen, C.-J., and Chen, C. J. (2002). Japanese encephalitis virus stimulates superoxide dismutase activity in rat glial cultures. Neurosci. Lett. 324 (2), 133–136. doi:10.1016/s0304-3940(02)00236-7
Lin, J., Shorter, J., and Lucius, A. L. (2022). AAA+ proteins: One motor, multiple ways to work. Biochem. Soc. Trans. 50 (2), 895–906. doi:10.1042/BST20200350
Ling, S., Luo, M., Jiang, S., Liu, J., Ding, C., Zhang, Q., et al. (2018). Cellular Hsp27 interacts with classical swine fever virus NS5A protein and negatively regulates viral replication by the NF-κB signaling pathway. Virology 518, 202–209. doi:10.1016/j.virol.2018.02.020
Lubkowska, A., Pluta, W., Strońska, A., and Lalko, A. (2021). Role of heat shock proteins (HSP70 and HSP90) in viral infection. Int. J. Mol. Sci. 22 (17), 9366. doi:10.3390/ijms22179366
Luengo, T. M., Mayer, M. P., and Rüdiger, S. G. D. (2019). The Hsp70–Hsp90 chaperone cascade in protein folding. Trends Cell Biol. 29 (2), 164–177. doi:10.1016/j.tcb.2018.10.004
Lukas, J., Pospech, J., Oppermann, C., Hund, C., Iwanov, K., Pantoom, S., et al. (2019). Role of endoplasmic reticulum stress and protein misfolding in disorders of the liver and pancreas. Adv. Med. Sci. 64, 315–323. doi:10.1016/j.advms.2019.03.004
Luo, J., Rizvi, H., Preeshagul, I. R., Egger, J. V., Hoyos, D., Bandlamudi, C., et al. (2020). COVID-19 in patients with lung cancer. Ann. Oncol. 31 (10), 1386–1396. doi:10.1016/j.annonc.2020.06.007
Macejak, D. G., and Sarnow, P. (1992). Association of heat shock protein 70 with enterovirus capsid precursor P1 in infected human cells. J. Virol. 66 (3), 1520–1527. doi:10.1128/JVI.66.3.1520-1527.1992
Mahmood, F., Xu, R., Awan, M. U. N., Song, Y., Han, Q., Xia, X., et al. (2021). PDIA3: Structure, functions and its potential role in viral infections. Biomed. Pharmacother. 143, 112110. doi:10.1016/j.biopha.2021.112110
Makhnevych, T., and Houry, W. A. (2012). The role of Hsp90 in protein complex assembly. Biochim. Biophys. Acta 1823 (3), 674–682. doi:10.1016/j.bbamcr.2011.09.001
Manzoor, R., Kuroda, K., Yoshida, R., Tsuda, Y., Fujikura, D., Miyamoto, H., et al. (2014). Heat shock protein 70 modulates influenza A virus polymerase activity. J. Biol. Chem. 289 (11), 7599–7614. doi:10.1074/jbc.M113.507798
Marino Gammazza, A., Légaré, S., Lo Bosco, G., Fucarino, A., Angileri, F., Conway de Macario, E., et al. (2020). Human molecular chaperones share with SARS-CoV-2 antigenic epitopes potentially capable of eliciting autoimmunity against endothelial cells: Possible role of molecular mimicry in COVID-19. Cell Stress Chaperones 25 (5), 737–741. doi:10.1007/s12192-020-01148-3
Marinova, M., Solopov, P., Dimitropoulou, C., Colunga Biancatelli, R. M., and Catravas, J. D. (2020). Post-treatment with a heat shock protein 90 inhibitor prevents chronic lung injury and pulmonary fibrosis, following acute exposure of mice to HCl. Exp. Lung Res. 46 (6), 203–216. doi:10.1080/01902148.2020.1764148
Märker, T., Sell, H., Zilleßen, P., Glöde, A., Kriebel, J., Ouwens, D. M., et al. (2012). Heat shock protein 60 as a mediator of adipose tissue inflammation and insulin resistance. Diabetes 61 (3), 615–625. doi:10.2337/db10-1574
Marzec, L., Zdrojewski, Z., Liberek, T., Bryl, E., Chmielewski, M., Witkowski, J. M., et al. (2009). Expression of Hsp72 protein in chronic kidney disease patients. Scand. J. Urol. Nephrol. 43 (5), 400–408. doi:10.3109/00365590903089489
Marzec, M., Eletto, D., and Argon, Y. (2012). GRP94: An HSP90-like protein specialized for protein folding and quality control in the endoplasmic reticulum. Biochim. Biophys. Acta 1823, 774–787. doi:10.1016/j.bbamcr.2011.10.013
McBride, R., Van Zyl, M., and Fielding, B. C. (2014). The coronavirus nucleocapsid is a multifunctional protein. Viruses 6 (8), 2991–3018. doi:10.3390/v6082991
McGonagle, D., O'Donnell, J. S., Sharif, K., Emery, P., and Bridgewood, C. (2020). Immune mechanisms of pulmonary intravascular coagulopathy in COVID-19 pneumonia. Lancet Rheumatol. 2 (7), e437–e445. doi:10.1016/S2665-9913(20)30121-1
Meikle, C. K. S., Creeden, J. F., McCullumsmith, C., and Worth, R. G. (2021). SSRIs: Applications in inflammatory lung disease and implications for COVID-19. Neuropsychopharmacol. Rep. 41, 325–335. doi:10.1002/npr2.12194
Melville, M. W., Hansen, W. J., Freeman, B. C., Welch, W. J., and Katze, M. G. (1997). The molecular chaperone hsp40 regulates the activity of P58IPK, the cellular inhibitor of PKR. Proc. Natl. Acad. Sci. U. S. A. 94 (1), 97–102. doi:10.1073/pnas.94.1.97
Merkling, S. H., Overheul, G. J., van Mierlo, J. T., Arends, D., Gilissen, C., and van Rij, R. P. (2015). The heat shock response restricts virus infection in Drosophila. Sci. Rep. 5 (1), 12758. doi:10.1038/srep12758
Milewska, A., Nowak, P., Owczarek, K., Szczepanski, A., Zarebski, M., Hoang, A., et al. (2018). Entry of human coronavirus NL63 into the cell. J. Virol. 92 (3), e01933–17. doi:10.1128/JVI.01933-17
Mittal, S., and Rajala, M. S. (2020). Heat shock proteins as biomarkers of lung cancer. Cancer Biol. Ther. 21 (6), 477–485. doi:10.1080/15384047.2020.1736482
Molinari, M., Eriksson, K. K., Calanca, V., Galli, C., Cresswell, P., Michalak, M., et al. (2004). Contrasting functions of calreticulin and calnexin in glycoprotein folding and ER quality control. Mol. Cell 13 (1), 125–135. doi:10.1016/s1097-2765(03)00494-5
Mollapour, M., and Neckers, L. (2012). Post-translational modifications of Hsp90 and their contributions to chaperone regulation. Biochim. Biophys. Acta 1823 (3), 648–655. doi:10.1016/j.bbamcr.2011.07.018
Momose, F., Naito, T., Yano, K., Sugimoto, S., Morikawa, Y., and Nagata, K. (2002). Identification of Hsp90 as a stimulatory host factor involved in influenza virus RNA synthesis. J. Biol. Chem. 277 (47), 45306–45314. doi:10.1074/jbc.M206822200
Morales-Buenrostro, L. E., Salas-Nolasco, O. I., Barrera-Chimal, J., Casas-Aparicio, G., Irizar-Santana, S., Perez-Villalva, R., et al. (2014). Hsp72 is a novel biomarker to predict acute kidney injury in critically ill patients. PloS one 9 (10), e109407. doi:10.1371/journal.pone.0109407
Moseley, P. (2000). Stress proteins and the immune response. Immunopharmacology 48 (3), 299–302. doi:10.1016/s0162-3109(00)00227-7
Musial, K., Szprynger, K., Szczepańska, M., and Zwolińska, D. (2010). The heat shock protein profile in children with chronic kidney disease. Perit. Dial. Int. 30 (2), 227–232. doi:10.3747/pdi.2008.00153
Musiał, K., and Zwolińska, D. (2011). Heat shock proteins in chronic kidney disease. Pediatr. Nephrol. 26, 1031–1037. doi:10.1007/s00467-010-1709-5
Nain, M., Mukherjee, S., Karmakar, S. P., Paton, A. W., Paton, J. C., Abdin, M. Z., et al. (2017). GRP78 is an important host factor for Japanese encephalitis virus entry and replication in mammalian cells. J. Virol. 91 (6), e02274–16. doi:10.1128/JVI.02274-16
Nayak Rao, S. (2016). The role of heat shock proteins in kidney disease. J. Transl. Int. Med. 4, 114–117. doi:10.1515/jtim-2016-0034
Nelson, E. V., Pacheco, J. R., Hume, A. J., Cressey, T. N., Deflubé, L. R., Ruedas, J. B., et al. (2017). An RNA polymerase II-driven Ebola virus minigenome system as an advanced tool for antiviral drug screening. Antivir. Res. 146, 21–27. doi:10.1016/j.antiviral.2017.08.005
Neukirch, L., Fougeroux, C., Andersson, A. M. C., and Holst, P. J. (2020). The potential of adenoviral vaccine vectors with altered antigen presentation capabilities. Expert Rev. Vaccines 19 (1), 25–41. doi:10.1080/14760584.2020.1711054
Ninagawa, S., George, G., and Mori, K. (2021). Mechanisms of productive folding and endoplasmic reticulum-associated degradation of glycoproteins and non-glycoproteins. Biochim. Biophys. Acta. Gen. Subj. 1865, 129812–129820. doi:10.1016/j.bbagen.2020.129812
Nollen, E. A. A., Kabakov, A. E., Brunsting, J. F., Kanon, B., Höhfeld, J., and Kampinga, H. H. (2001). Modulation of in vivo HSP70 chaperone activity by hip and bag-1. J. Biol. Chem. 276 (7), 4677–4682. doi:10.1074/jbc.M009745200
Obermann, W. M. J., Sondermann, H., Russo, A. A., Pavletich, N. P., and Hartl, F. U. (1998). In vivo function of Hsp90 is dependent on ATP binding and ATP hydrolysis. J. Cell Biol. 143 (4), 901–910. doi:10.1083/jcb.143.4.901
Oglesbee, M. J., Kenney, H., Kenney, T., and Krakowka, S. (1993). Enhanced production of morbillivirus gene-specific RNAs following induction of the cellular stress response in stable persistent infection. Virology 192 (2), 556–567. doi:10.1006/viro.1993.1072
Oh, H. J., Easton, D., Murawski, M., Kaneko, Y., and Subjeck, J. R. (1999). The chaperoning activity of hsp110 identification of functional domains by use of targeted deletions. J. Biol. Chem. 274 (22), 15712–15718. doi:10.1074/jbc.274.22.15712
Oikonomou, C., and Hendershot, L. M. (2020). Disposing of misfolded ER proteins: A troubled substrate's way out of the ER. Mol. Cell. Endocrinol. 500, 110630–30. doi:10.1016/j.mce.2019.110630
Okamoto, T., Yamamoto, H., Kudo, I., Matsumoto, K., Odaka, M., Grave, E., et al. (2017). HSP60 possesses a GTPase activity and mediates protein folding with HSP10. Sci. Rep. 7 (1), 16931. doi:10.1038/s41598-017-17167-7
Olagnier, D., Peri, S., Steel, C., van Montfoort, N., Chiang, C., Beljanski, V., et al. (2014). Cellular oxidative stress response controls the antiviral and apoptotic programs in dengue virus-infected dendritic cells. PLoS Pathog. 10 (12), e1004566. doi:10.1371/journal.ppat.1004566
Oliveira, A. P., Simabuco, F. M., Tamura, R. E., Guerrero, M. C., Ribeiro, P. G., Libermann, T. A., et al. (2013). Human respiratory syncytial virus N, P and M protein interactions in HEK-293T cells. Virus Res. 177 (1), 108–112. doi:10.1016/j.virusres.2013.07.010
Oliver, J. D., Roderick, H. L., Llewellyn, D. H., and High, S. (1999). ERp57 functions as a subunit of specific complexes formed with the ER lectins calreticulin and calnexin. Mol. Biol. Cell 10 (8), 2573–2582. doi:10.1091/mbc.10.8.2573
Oroz, J., Blair, L. J., and Zweckstetter, M. (2019). Dynamic Aha1 co-chaperone binding to human Hsp90. Protein Sci. 28 (9), 1545–1551. doi:10.1002/pro.3678
Oualikene, W., Lamoureux, L., Weber, J. M., and Massie, B. (2000). Protease-deleted adenovirus vectors and complementing cell lines: Potential applications of single-round replication mutants for vaccination and gene therapy. Hum. Gene Ther. 11 (9), 1341–1353. doi:10.1089/10430340050032438
Padwad, Y. S., Mishra, K. P., Jain, M., Chanda, S., Karan, D., and Ganju, L. (2009). RNA interference mediated silencing of Hsp60 gene in human monocytic myeloma cell line U937 revealed decreased dengue virus multiplication. Immunobiology 214 (6), 422–429. doi:10.1016/j.imbio.2008.11.010
Pan, Z., Wu, Q., Xie, Z., Wu, Q., Tan, X., and Wang, X. (2020). Upregulation of HSP72 attenuates tendon adhesion by regulating fibroblast proliferation and collagen production via blockade of the STAT3 signaling pathway. Cell. Signal. 71, 109606. doi:10.1016/j.cellsig.2020.109606
Parakh, S., and Atkin, J. D. (2015). Novel roles for protein disulphide isomerase in disease states: A double edged sword? Front. Cell Dev. Biol. 3, 30. doi:10.3389/fcell.2015.00030
Parissi, V., Calmels, C., De Soultrait, V. R., Caumont, A., Fournier, M., Chaignepain, S., et al. (2001). Functional interactions of human immunodeficiency virus type 1 integrase with human and yeast HSP60. J. Virol. 75 (23), 11344–11353. doi:10.1128/JVI.75.23.11344-11353.2001
Park, Y. H., Seo, J. H., Park, J., Lee, H. S., and Kim, K. (2017). Hsp70 acetylation prevents caspase-dependent/independent apoptosis and autophagic cell death in cancer cells. Int. J. Oncol. 51, 573–578. doi:10.3892/ijo.2017.4039
Pespeni, M., Mackersie, R. C., Lee, H., Morabito, D., Hodnett, M., Howard, M., et al. (2005). Serum levels of Hsp60 correlate with the development of acute lung injury after trauma. J. Surg. Res. 126 (1), 41–47. doi:10.1016/j.jss.2005.01.012
Petersen, O. H., Gerasimenko, O. V., and Gerasimenko, J. V. (2020). Endocytic uptake of SARS-CoV-2: the critical roles of pH, Ca2+, and NAADP. Function 1 (1), zqaa003. doi:10.1093/function/zqaa003
Prodromou, C. (2016). Mechanisms of Hsp90 regulation. Biochem. J. 473 (16), 2439–2452. doi:10.1042/BCJ20160005
Pujhari, S., Brustolin, M., Macias, V. M., Nissly, R. H., Nomura, M., Kuchipudi, S. V., et al. (2019). Heat shock protein 70 (Hsp70) mediates Zika virus entry, replication, and egress from host cells. Emerg. Microbes Infect. 8 (1), 8–16. doi:10.1080/22221751.2018.1557988
Ragab, D., Salah Eldin, H., Taeimah, M., Khattab, R., and Salem, R. (2020). The COVID-19 cytokine storm; what we know so far. Front. Immunol. 11, 1446. doi:10.3389/fimmu.2020.01446
Ramos, C. H., and Ayinde, K. S. (2021). Are Hsp90 inhibitors good candidates against Covid-19? Curr. Protein Pept. Sci. 22 (3), 192–200. doi:10.2174/1389203721666201111160925
Ranek Mark, J., Stachowski Marisa, J., Kirk Jonathan, A., and Willis Monte, S. (2018). The role of heat shock proteins and co-chaperones in heart failure. Philos. Trans. R. Soc. Lond. B Biol. Sci. 373 (1738), 20160530. doi:10.1098/rstb.2016.0530
Rao, S. N. (2016). The role of heat shock proteins in kidney disease. J. Transl. Int. Med. 4 (3), 114–117. doi:10.1515/jtim-2016-0034
Rathore, A. P., Haystead, T., Das, P. K., Merits, A., Ng, M. L., and Vasudevan, S. G. (2014). Chikungunya virus nsP3 & nsP4 interacts with HSP-90 to promote virus replication: HSP-90 inhibitors reduce CHIKV infection and inflammation in vivo. Antivir. Res. 103, 7–16. doi:10.1016/j.antiviral.2013.12.010
Reizine, F., Lesouhaitier, M., Gregoire, M., Pinceaux, K., Gacouin, A., Maamar, A., et al. (2021). SARS-CoV-2-induced ARDS associates with MDSC expansion, lymphocyte dysfunction, and arginine shortage. J. Clin. Immunol. 41 (3), 515–525. doi:10.1007/s10875-020-00920-5
Reyes-del Valle, J., Chávez-Salinas, S., Medina, F., and Del Angel, R. M. (2005). Heat shock protein 90 and heat shock protein 70 are components of dengue virus receptor complex in human cells. J. Virol. 79 (8), 4557–4567. doi:10.1128/JVI.79.8.4557-4567.2005
Richter, K., Haslbeck, M., and Buchner, J. (2010). The heat shock response: Life on the verge of death. Mol. Cell 40, 253–266. doi:10.1016/j.molcel.2010.10.006
Riemer, J., Hansen, H. G., Appenzeller-Herzog, C., Johansson, L., and Ellgaard, L. (2011). Identification of the PDI-family member ERp90 as an interaction partner of ERFAD. PloS One 6 (2), e17037. doi:10.1371/journal.pone.0017037
Rodriguez-Iturbe, B., and Johnson, R. J. (2018). Heat shock proteins and cardiovascular disease. Physiol. Int. 105 (1), 19–37. doi:10.1556/2060.105.2018.1.4
Rodriguez-Iturbe, B., Lanaspa, M. A., and Johnson, R. J. (2019). The role of autoimmune reactivity induced by heat shock protein 70 in the pathogenesis of essential hypertension. Br. J. Pharmacol. 176 (12), 1829–1838. doi:10.1111/bph.14334
Romagnoli, S., Peris, A., De Gaudio, A. R., and Geppetti, P. (2020). SARS-CoV-2 and COVID-19: From the bench to the bedside. Physiol. Rev. 100 (4), 1455–1466. doi:10.1152/physrev.00020.2020
Rossmann, M. G. (1984). Constraints on the assembly of spherical virus particles. Virology 134 (1), 1–11. doi:10.1016/0042-6822(84)90267-8
Ryu, H. H., and Ha, S. H. (2020). HSP70 interacts with Rheb, inhibiting mTORC1 signaling. Biochem. Biophys. Res. Commun. 533 (4), 1198–1203. doi:10.1016/j.bbrc.2020.07.053
Sabirli, R., Koseler, A., Goren, T., Turkcuer, I., and Kurt, O. (2021). High GRP78 levels in covid-19 infection: A case-control study. Life Sci. 265, 118781. doi:10.1016/j.lfs.2020.118781
Sagara, Y., Ishida, C., Inoue, Y., Shiraki, H., and Maeda, Y. (1998). 71-kilodalton heat shock cognate protein acts as a cellular receptor for syncytium formation induced by human T-cell lymphotropic virus type 1. J. Virol. 72 (1), 535–541. doi:10.1128/JVI.72.1.535-541.1998
Saha, A., and Anirvan, P. (2020). Cancer progression in COVID-19: Integrating the roles of renin angiotensin aldosterone system, angiopoietin-2, heat shock protein-27 and epithelial mesenchymal transition. Ecancermedicalscience 14, 1099. doi:10.3332/ecancer.2020.1099
Saksela, K. (1997). HIV-1 Nef and host cell protein kinases. Front. Biosci. 2 (4), 606–618. doi:10.2741/a217
Samali, A., and Orrenius, S. (1998). Heat shock proteins: Regulators of stress response and apoptosis. Cell Stress Chaperones 3, 228–236. doi:10.1379/1466-1268(1998)003<0228:hspros>2.3.co;2
Sano, R., and Reed, J. C. (2013). ER stress-induced cell death mechanisms. Biochim. Biophys. Acta 1833, 3460–3470. doi:10.1016/j.bbamcr.2013.06.028
Sanyaolu, A., Okorie, C., Marinkovic, A., Patidar, R., Younis, K., Desai, P., et al. (2020). Comorbidity and its impact on patients with COVID-19. SN Compr. Clin. Med. 2, 1069–1076. doi:10.1007/s42399-020-00363-4
Sarkar, S., and Roy, S. (2017). A mini review on heat shock proteins (hsps): Special emphasis on heat shock protein70 (hsp70). BN Seal. J. Sci. 9, 129–138.
Schett, G., Metzler, B., Kleindienst, R., Moschèn, I., Hattmannsdorfer, R., Wolf, H., et al. (1997). Salivary anti-hsp65 antibodies as a diagnostic marker for gingivitis and a possible link to atherosclerosis. Int. Arch. Allergy Immunol. 114 (3), 246–250. doi:10.1159/000237675
Schönthal, A. H. (2012). Endoplasmic reticulum stress: its role in disease and novel prospects for therapy. Scientifica 2012, 857516. doi:10.6064/2012/857516
Sell, H., Habich, C., and Eckel, J. (2012). Adaptive immunity in obesity and insulin resistance. Nat. Rev. Endocrinol. 8 (12), 709–716. doi:10.1038/nrendo.2012.114
Sell, H., Poitou, C., Habich, C., Bouillot, J. L., Eckel, J., and Clément, K. (2017). Heat shock protein 60 in obesity: Effect of bariatric surgery and its relation to inflammation and cardiovascular risk. Obesity 25 (12), 2108–2114. doi:10.1002/oby.22014
Sellares, J., Veraldi, K. L., Thiel, K. J., Cárdenes, N., Alvarez, D., Schneider, F., et al. (2019). Intracellular heat shock protein 70 deficiency in pulmonary fibrosis. Am. J. Respir. Cell Mol. Biol. 60 (6), 629–636. doi:10.1165/rcmb.2017-0268OC
Sharif-Askari, N. S., Sharif-Askari, F. S., Mdkhana, B., Alsayed, H. A. H., Alsafar, H., Alrais, Z. F., et al. (2021). Upregulation of oxidative stress gene markers during SARS-COV-2 viral infection. Free Radic. Biol. Med. 172, 688–698. doi:10.1016/j.freeradbiomed.2021.06.018
Sharma, K., Tripathi, S., Ranjan, P., Kumar, P., Garten, R., Deyde, V., et al. (2011). Influenza A virus nucleoprotein exploits Hsp40 to inhibit PKR activation. PLoS One 6 (6), e20215. doi:10.1371/journal.pone.0020215
Shevchenko, M., Servuli, E., Albakova, Z., Kanevskiy, L., and Sapozhnikov, A. (2020). The role of heat shock protein 70 kDa in asthma. J. Asthma Allergy 13, 757–772. doi:10.2147/JAA.S288886
Shi, C.-S., Nabar, N. R., Huang, N. N., and Kehrl, J. H. (2019). SARS-Coronavirus Open Reading Frame-8b triggers intracellular stress pathways and activates NLRP3 inflammasomes. Cell Death Discov. 5 (1), 101. doi:10.1038/s41420-019-0181-7
Simmons, A., Aluvihare, V., and McMichael, A. (2001). Nef triggers a transcriptional program in T cells imitating single-signal T cell activation and inducing HIV virulence mediators. Immunity 14 (6), 763–777. doi:10.1016/s1074-7613(01)00158-3
Singh, I. S., and Hasday, J. D. (2013). Fever, hyperthermia and the heat shock response. Int. J. Hyperth. 29 (5), 423–435. doi:10.3109/02656736.2013.808766
Silverstein, N. J., Wang, Y., Manickas-Hill, Z., Carbone, C., Dauphin, A., Boribong, B. P., et al. (2022). Innate lymphoid cells and COVID-19 severity in SARS-CoV-2 infection. elife 11, e74681. doi:10.7554/eLife.74681
Skolnik, K., and Ryerson, C. J. (2016). Unclassifiable interstitial lung disease: A review. Respirology 21 (1), 51–56. doi:10.1111/resp.12568
Song, H., Moseley, P. L., Lowe, S. L., and Ozbun, M. A. (2010). Inducible heat shock protein 70 enhances HPV31 viral genome replication and virion production during the differentiation-dependent life cycle in human keratinocytes. Virus Res. 147 (1), 113–122. doi:10.1016/j.virusres.2009.10.019
Song, P., Li, W., Xie, J., Hou, Y., and You, C. (2020). Cytokine storm induced by SARS-CoV-2. Clin. Chim. Acta. 509, 280–287. doi:10.1016/j.cca.2020.06.017
Srivastava, K. (2020). Association between COVID-19 and cardiovascular disease. Int. J. Cardiol. Heart Vasc. 29, 100583. doi:10.1016/j.ijcha.2020.100583
Stocker, R., and Keaney, J. F. (2004). Role of oxidative modifications in Atherosclerosis. Physiol. Rev. 84 (4), 1381–1478. doi:10.1152/physrev.00047.2003
Storkanova, H., Oreska, S., Spiritovic, M., Hermankova, B., Pavelka, K., Vencovsky, J., et al. (2018). SAT0493 Plasma levels of hsp90 are increased in interstitial lung disease and skin fibrosis in patients with systemic sclerosis. Ann. Rheum. Dis. 73 (6), 1215–1222.
Suh, J., Mukerji, S. S., Collens, S. I., Padera, R. F., Pinkus, G. S., Amato, A. A., et al. (2021). Skeletal muscle and peripheral nerve histopathology in COVID-19. Neurology 97 (8), e849–e858. doi:10.1212/WNL.0000000000012344
Sun, M., Yu, Z., Ma, J., Pan, Z., Lu, C., and Yao, H. (2017). Down-regulating heat shock protein 27 is involved in porcine epidemic diarrhea virus escaping from host antiviral mechanism. Vet. Microbiol. 205, 6–13. doi:10.1016/j.vetmic.2017.04.031
Sun, Y., Huang, Y.-H., Huang, F.-Y., Mei, W.-L., Liu, Q., Wang, C.-C., et al. (2018). 3'-epi-12β-hydroxyfroside, a new cardenolide, induces cytoprotective autophagy via blocking the Hsp90/Akt/mTOR axis in lung cancer cells. Theranostics 8, 2044–2060. doi:10.7150/thno.23304
Sureda, A., Alizadeh, J., Nabavi, S. F., Berindan-Neagoe, I., Cismaru, C. A., Jeandet, P., et al. (2020). Endoplasmic reticulum as a potential therapeutic target for Covid-19 infection management? Eur. J. Pharmacol. 882, 173288. doi:10.1016/j.ejphar.2020.173288
Swaroop, S., Mahadevan, A., Shankar, S. K., Adlakha, Y. K., and Basu, A. (2018). HSP60 critically regulates endogenous IL-1β production in activated microglia by stimulating NLRP3 inflammasome pathway. J. Neuroinflammation 15 (1), 177. doi:10.1186/s12974-018-1214-5
Szczepanski, A., Owczarek, K., Milewska, A., Baster, Z., Rajfur, Z., Mitchell, J. A., et al. (2018). Canine respiratory coronavirus employs caveolin-1-mediated pathway for internalization to HRT-18G cells. Vet. Res. 49 (1), 55. doi:10.1186/s13567-018-0551-9
Taguwa, S., Yeh, M. T., Rainbolt, T. K., Nayak, A., Shao, H., Gestwicki, J. E., et al. (2019). Zika virus dependence on host Hsp70 provides a protective strategy against infection and disease. Cell Rep. 26 (4), 906–920. doi:10.1016/j.celrep.2018.12.095
Tanguy, J., Pommerolle, L., Garrido, C., Kolb, M., Bonniaud, P., Goirand, F., et al. (2021). Extracellular heat shock proteins as therapeutic targets and biomarkers in fibrosing interstitial lung diseases. Int. J. Mol. Sci. 22 (17), 9316. doi:10.3390/ijms22179316
Tiss, A., Khadir, A., Abubaker, J., Abu-Farha, M., Al-Khairi, I., Cherian, P., et al. (2014). Immunohistochemical profiling of the heat shock response in obese non-diabetic subjects revealed impaired expression of heat shock proteins in the adipose tissue. Lipids Health Dis. 13, 106. doi:10.1186/1476-511X-13-106
Torres, J., Parthasarathy, K., Lin, X., Saravanan, R., Kukol, A., and Liu, D. X. (2006). Model of a putative pore: the pentameric α-helical bundle of SARS coronavirus E protein in lipid bilayers. Biophys. J. 91 (3), 938–947. doi:10.1529/biophysj.105.080119
Triantafilou, K., Fradelizi, D., Wilson, K., and Triantafilou, M. (2002). GRP78, a coreceptor for coxsackievirus A9, interacts with major histocompatibility complex class I molecules which mediate virus internalization. J. Virol. 76 (2), 633–643. doi:10.1128/jvi.76.2.633-643.2002
Tsou, Y. L., Lin, Y. W., Chang, H. W., Lin, H. Y., Shao, H. Y., Yu, S. L., et al. (2013). Heat shock protein 90: Role in enterovirus 71 entry and assembly and potential target for therapy. PLoS One 8 (10), e77133. doi:10.1371/journal.pone.0077133
Tsutsumi, S., and Neckers, L. (2007). Extracellular heat shock protein 90: A role for a molecular chaperone in cell motility and cancer metastasis. Cancer Sci. 98 (10), 1536–1539. doi:10.1111/j.1349-7006.2007.00561.x
Uddin, M., Mustafa, F., Rizvi, T. A., Loney, T., Al Suwaidi, H., Al-Marzouqi, A. H. H., et al. (2020). SARS-CoV-2/COVID-19: Viral genomics, epidemiology, vaccines, and therapeutic interventions. Viruses 12 (5), 526. doi:10.3390/v12050526
Ujino, S., Yamaguchi, S., Shimotohno, K., and Takaku, H. (2009). Heat-shock protein 90 is essential for stabilization of the hepatitis C virus nonstructural protein NS3. J. Biol. Chem. 284 (11), 6841–6846. doi:10.1074/jbc.M806452200
Varga, Z., Flammer, A. J., Steiger, P., Haberecker, M., Andermatt, R., Zinkernagel, A. S., et al. (2020). Endothelial cell infection and endotheliitis in COVID-19. Lancet 395 (10234), 1417–1418. doi:10.1016/S0140-6736(20)30937-5
Vasconcelos, D. Y., Cai, X. H., and Oglesbee, M. J. (1998). Constitutive overexpression of the major inducible 70 kDa heat shock protein mediates large plaque formation by measles virus. J. Gen. Virol. 79 (9), 2239–2247. doi:10.1099/0022-1317-79-9-2239
Vashist, S., Urena, L., Gonzalez-Hernandez, M. B., Choi, J., de Rougemont, A., Rocha-Pereira, J., et al. (2015). Molecular chaperone Hsp90 is a therapeutic target for noroviruses. J. Virol. 89 (12), 6352–6363. doi:10.1128/JVI.00315-15
Vega-Almeida, T. O., Salas-Benito, M., Nova-Ocampo, D., Ascensión, M., del Angel, R. M., and Salas-Benito, J. S. (2013). Surface proteins of C6/36 cells involved in dengue virus 4 binding and entry. Arch. Virol. 158 (6), 1189–1207. doi:10.1007/s00705-012-1596-0
Wan, Q., Song, D., Li, H., and He, M. L. (2020). Stress proteins: The biological functions in virus infection, present and challenges for target-based antiviral drug development. Signal Transduct. Target. Ther. 5 (1), 125–140. doi:10.1038/s41392-020-00233-4
Wang, C., Zhang, Y., Guo, K., Wang, N., Jin, H., Liu, Y., et al. (2015). Heat shock proteins in hepatocellular carcinoma: Molecular mechanism and therapeutic potential. Int. J. Cancer 138 (8), 1824–1834. doi:10.1002/ijc.29723
Wang, F., Qiu, Y., Zhang, H. M., Hanson, P., Ye, X., Zhao, G., et al. (2017). Heat shock protein 70 promotes coxsackievirus B3 translation initiation and elongation via Akt-mTORC1 pathway depending on activation of p70S6K and Cdc2. Cell. Microbiol. 19 (7), e12725. doi:10.1111/cmi.12725
Wang, M., Dong, Q., Wang, H., He, Y., Chen, Y., Zhang, H., et al. (2016). Oblongifolin M, an active compound isolated from a Chinese medical herb Garcinia oblongifolia, potently inhibits enterovirus 71 reproduction through downregulation of ERp57. Oncotarget 7 (8), 8797–8808. doi:10.18632/oncotarget.7122
Wang, R. Y. L., Huang, Y. R., Chong, K. M., Hung, C. Y., Ke, Z. L., and Chang, R. Y. (2011). DnaJ homolog Hdj2 facilitates Japanese encephalitis virus replication. Virol. J. 8 (1), 471–477. doi:10.1186/1743-422X-8-471
Wang, R. Y. R., Noddings, C. M., Kirschke, E., Myasnikov, A. G., Johnson, J. L., and Agard, D. A. (2022). Structure of hsp90–hsp70–hop–GR reveals the Hsp90 client-loading mechanism. Nature 601 (7893), 460–464. doi:10.1038/s41586-021-04252-1
Wang, X., Zheng, T., Lin, L., Zhang, Y., Peng, X., Yan, Y., et al. (2020b). Influenza a virus induces autophagy by its hemagglutinin binding to cell surface heat shock protein 90AA1. Front. Microbiol. 11, 566348. doi:10.3389/fmicb.2020.566348
Wang, Y., Grunewald, M., and Perlman, S. (2020a). Coronaviruses: An updated overview of their replication and pathogenesis. Methods Mol. Biol. 2203, 1–29. doi:10.1007/978-1-0716-0900-2_1
Waxman, L., Whitney, M., Pollok, B. A., Kuo, L. C., and Darke, P. L. (2001). Host cell factor requirement for hepatitis C virus enzyme maturation. Proc. Natl. Acad. Sci. U. S. A. 98 (24), 13931–13935. doi:10.1073/pnas.241510898
Weiss, Y. G., Bromberg, Z., Raj, N., Raphael, J., Goloubinoff, P., Ben-Neriah, Y., et al. (2007). Enhanced heat shock protein 70 expression alters proteasomal degradation of IkappaB kinase in experimental acute respiratory distress syndrome. Crit. Care Med. 35 (9), 2128–2138. doi:10.1097/01.ccm.0000278915.78030.74
Wendt, R., Lingitz, M. T., Laggner, M., Mildner, M., Traxler, D., Graf, A., et al. (2021). Clinical relevance of elevated soluble ST2, HSP27 and 20S proteasome at hospital admission in patients with COVID-19. Biology 10 (11), 1186. doi:10.3390/biology10111186
Wick, G., Jakic, B., Buszko, M., Wick, M. C., and Grundtman, C. (2014). The role of heat shock proteins in atherosclerosis. Nat. Rev. Cardiol. 11 (9), 516–529. doi:10.1038/nrcardio.2014.91
World Health Organization (2020). Novel coronavirus (2019-nCoV): Situation report, 3. Available at: https://reliefweb.int/report/china/novel-coronavirus-2019-ncov-situation-report-3-23-january-2020.
Wu, J., Liu, T., Rios, Z., Mei, Q., Lin, X., and Cao, S. (2017a). Heat shock proteins and cancer. Trends Pharmacol. Sci. 38 (3), 226–256. doi:10.1016/j.tips.2016.11.009
Wu, P., Chang, Y., and Pan, C. (2017b). High expression of heat shock proteins and heat shock factor-1 distinguishes an aggressive subset of clear cell renal cell carcinoma. Histopathology 71 (5), 711–718. doi:10.1111/his.13284
Wyler, E., Mösbauer, K., Franke, V., Diag, A., Gottula, L. T., Arsiè, R., et al. (2021). Transcriptomic profiling of SARS-CoV-2 infected human cell lines identifies HSP90 as target for COVID-19 therapy. Iscience 24 (3), 102151. doi:10.1016/j.isci.2021.102151
Xiao, A., Wong, J., and Luo, H. (2010). Viral interaction with molecular chaperones: Role in regulating viral infection. Arch. Virol. 155 (7), 1021–1031. doi:10.1007/s00705-010-0691-3
Xu, T., Lin, Z., Wang, C., Li, Y., Xia, Y., Zhao, M., et al. (2019). Heat shock protein 70 as a supplementary receptor facilitates enterovirus 71 infections in vitro. Microb. Pathog. 128, 106–111. doi:10.1016/j.micpath.2018.12.032
Xu, W., Mollapour, M., Prodromou, C., Wang, S., Scroggins, B. T., Palchick, Z., et al. (2012). Dynamic tyrosine phosphorylation modulates cycling of the Hsp90-P50CDC37-AHA1 chaperone machine. Mol. Cell 47, 434–443. doi:10.1016/j.molcel.2012.05.015
Xue, T., Li, Q., Zhang, Q., Lin, W., Weng, J., Li, L., et al. (2020). Blood glucose levels in elderly subjects with type 2 diabetes during Covid-19 outbreak: A retrospective study in a single center (3/31/2020). Available at SSRN: https://ssrn.com/abstract=3566198.
Yang, D., de la Rosa, G., Tewary, P., and Oppenheim, J. J. (2009). Alarmins link neutrophils and dendritic cells. Trends Immunol. 30 (11), 531–537. doi:10.1016/j.it.2009.07.004
Yang, K., Xing, M. Y., Jiang, L. Y., Cai, Y. P., Yang, L. L., Xie, N. N., et al. (2021). Infection-associated hemophagocytic syndrome in critically ill patients with COVID-19. Curr. Med. Sci. 41 (1), 39–45. doi:10.1007/s11596-021-2315-4
Yang, N., and Shen, H. M. (2020). Targeting the endocytic pathway and autophagy process as a novel therapeutic strategy in COVID-19. Int. J. Biol. Sci. 16 (10), 1724–1731. doi:10.7150/ijbs.45498
Ye, Z., Wong, C. K., Li, P., and Xie, Y. (2008). A SARS-CoV protein, ORF-6, induces caspase-3 mediated, ER stress and JNK-dependent apoptosis. Biochim. Biophys. Acta 1780 (12), 1383–1387. doi:10.1016/j.bbagen.2008.07.009
Yi, Z., Yuan, Z., Rice, C. M., and MacDonald, M. R. (2012). Flavivirus replication complex assembly revealed by DNAJC14 functional mapping. J. Virol. 86 (21), 11815–11832. doi:10.1128/JVI.01022-12
Zhang, H., Penninger, J. M., Li, Y., Zhong, N., and Slutsky, A. S. (2020b). Angiotensin-converting enzyme 2 (ACE2) as a SARS-CoV-2 receptor: Molecular mechanisms and potential therapeutic target. Intensive Care Med. 46 (4), 586–590. doi:10.1007/s00134-020-05985-9
Zhang, T., Wu, Q., and Zhang, Z. (2020a). Probable pangolin origin of SARS-CoV-2 associated with the COVID-19 outbreak. Curr. Biol. 30 (7), 1346–1351. doi:10.1016/j.cub.2020.03.022
Zhang, W., Yang, F., Zhu, Z., Yang, Y., Wang, Z., Cao, W., et al. (2019). Cellular DNAJA3, a novel VP1-interacting protein, inhibits foot-and-mouth disease virus replication by inducing lysosomal degradation of VP1 and attenuating its antagonistic role in the beta interferon signaling pathway. J. Virol. 93 (13), e00588–19. doi:10.1128/JVI.00588-19
Zhao, Y., Xiao, D., Zhang, L., Song, D., Chen, R., Li, S., et al. (2022). HSP90 inhibitors 17-AAG and VER-82576 inhibit porcine deltacoronavirus replication in vitro. Vet. Microbiol. 265, 109316. doi:10.1016/j.vetmic.2021.109316
Zilaee, M., and Shirali, S. (2016). Heat shock proteins and diabetes. Can J. Diabetes 40 (6), 594–602. doi:10.1016/j.jcjd.2016.05.016
Zhou, X., Zhang, C. Z., Lu, S. X., Chen, G. G., Li, L. Z., Liu, L. L., et al. (2015). miR-625 suppresses tumour migration and invasion by targeting IGF2BP1 in hepatocellular carcinoma. Oncogene 34 (8), 965–977. doi:10.1038/onc.2014.35
Zhu, G., and Lee, A. S. (2015). Role of the unfolded protein response, GRP78 and GRP94 in organ homeostasis. J. Cell. Physiol. 230, 1413–1420. doi:10.1002/jcp.24923
Zhu, N., Zhang, D., Wang, W., Li, X., Yang, B., Song, J., et al. (2020). A novel coronavirus from patients with pneumonia in China, 2019. N. Engl. J. Med. 382, 727–733. doi:10.1056/NEJMoa2001017
Zimmermann, M., Traxler, D., Bekos, C., Simader, E., Mueller, T., Graf, A., et al. (2020). Heat shock protein 27 as a predictor of prognosis in patients admitted to hospital with acute COPD exacerbation. Cell Stress Chaperones 25 (1), 141–149. doi:10.1007/s12192-019-01057-0
Zininga, T., Ramatsui, L., and Shonhai, A. (2018). Heat shock proteins as immunomodulants. Molecules 23 (11), 2846. doi:10.3390/molecules23112846
Zolkiewski, M., Zhang, T., and Nagy, M. (2012). Aggregate reactivation mediated by the Hsp100 chaperones. Arch. Biochem. Biophys. 520 (1), 1–6. doi:10.1016/j.abb.2012.01.012
Zong, Z., Wei, Y., Ren, J., Zhang, L., and Zhou, F. (2021). The intersection of COVID-19 and cancer: Signaling pathways and treatment implications. Mol. Cancer 20 (1), 76–19. doi:10.1186/s12943-021-01363-1
Keywords: SARS–CoV–2, COVID-19, cell stress responses, heat shock proteins, stress proteins, drug target
Citation: Caillet C, Stofberg ML, Muleya V, Shonhai A and Zininga T (2022) Host cell stress response as a predictor of COVID-19 infectivity and disease progression. Front. Mol. Biosci. 9:938099. doi: 10.3389/fmolb.2022.938099
Received: 06 May 2022; Accepted: 15 July 2022;
Published: 11 August 2022.
Edited by:
Xolani Henry Makhoba, University of Fort Hare, South AfricaReviewed by:
Ofentse Jacob Pooe, University of KwaZulu-Natal, South AfricaJeffersson Leandro Jimenez Restrepo, University of São Paulo, Brazil
Copyright © 2022 Caillet, Stofberg, Muleya, Shonhai and Zininga. This is an open-access article distributed under the terms of the Creative Commons Attribution License (CC BY). The use, distribution or reproduction in other forums is permitted, provided the original author(s) and the copyright owner(s) are credited and that the original publication in this journal is cited, in accordance with accepted academic practice. No use, distribution or reproduction is permitted which does not comply with these terms.
*Correspondence: Tawanda Zininga, dHppbmluZ2FAc3VuLmFjLnph