- 1Department of Molecular and Translational Medicine, University of Brescia, Brescia, Italy
- 2School of Medicine and Surgery, University of Milano-Bicocca, Milan, Italy
- 3Medical Department, Geriatric Unit, Azienda ULSS (Unità Locale Socio Sanitaria) 3 “Serenissima,” Venice, Italy
- 4Department of Pharmaceutical Sciences, University of Milan, Milan, Italy
- 5Aging Branch, Neuroscience Institute, National Research Council, Padua, Italy
- 6Geriatrics Section, Department of Medicine, University of Palermo, Palermo, Italy
Frailty is an aging related condition, which has been defined as a state of enhanced vulnerability to stressors, leading to a limited capacity to meet homeostatic demands. Cognitive impairment is also frequent in older people, often accompanying frailty. Age is the main independent risk factor for both frailty and cognitive impairment, and compelling evidence suggests that similar age-associated mechanisms could underlie both clinical conditions. Accordingly, it has been suggested that frailty and cognitive impairment share common pathways, and some authors proposed “cognitive frailty” as a single complex phenotype. Nevertheless, so far, no clear common underlying pathways have been discovered for both conditions. microRNAs (miRNAs) have emerged as key fine-tuning regulators in most physiological processes, as well as pathological conditions. Importantly, miRNAs have been proposed as both peripheral biomarkers and potential molecular factors involved in physiological and pathological aging. In this review, we discuss the evidence linking changes of selected miRNAs expression with frailty and cognitive impairment. Overall, miR-92a-5p and miR-532-5p, as well as other miRNAs implicated in pathological aging, should be investigated as potential biomarkers (and putative molecular effectors) of cognitive frailty.
Introduction
The greatest achievement of public healthcare in the last several decades has been the large increase in lifespan. Yet the increasing aging population has brought about new challenges to the health system, with the mounting prevalence of geriatric conditions requiring a new general healthcare system for people afflicted by physical and mental impairment (Beard et al., 2016; Howdon and Rice, 2018).
In older people, frailty and cognitive impairment are commonly found together (Fabricio et al., 2020). Frailty is a clinical syndrome with different definitions, generally referred as a state of increased vulnerability to stressors that results from a decreased physiological reserve in multiple organs and systems, leading to a limited capacity to meet homeostatic demands (Clegg et al., 2013; Proietti and Cesari, 2020). Although frailty and cognitive impairment could be considered as distinct clinical states, converging evidence has shown a close epidemiological association between these conditions (Halil et al., 2015; Kiiti Borges et al., 2019; Miyamura et al., 2019). This led to the generation of the term “cognitive frailty,” defined as a heterogeneous clinical condition characterized by the concomitant presence of both physical frailty and cognitive impairment (Kelaiditi et al., 2013).
Nevertheless, the molecular mechanisms underlying cognitive frailty are still largely unknown. microRNAs (miRNAs) are a large family of conserved small (20–22 nucleotides) non-coding RNAs involved in post-transcriptional regulation of gene expression. Each miRNA targets hundreds of transcripts mainly repressing translation or inducing mRNA degradation of target transcripts through sequence-specific binding (Mohr and Mott, 2015). Compelling evidence suggests that miRNAs are both involved in physiological/pathological processes associated with aging (Williams et al., 2017) and in the regulation of brain functions (Kumar et al., 2017a; Nampoothiri and Rajanikant, 2017). Indeed, miRNAs act in several biological functions, such as proliferation, apoptosis, cell differentiation, embryogenesis, organogenesis, signal transduction and metabolism (Alvarez-Garcia and Miska, 2005; Kloosterman and Plasterk, 2006). Thus, it should not be surprising that miRNAs were recognized as key modulators of virtually all physiological processes and, consequently, miRNAs dysregulation have been reported in a multiplicity of diseases (Figure 1; Condrat et al., 2020). In addition to their presence inside the cells, miRNAs can be found also in extracellular fluids, forming the so-called circulating miRNAs, which are supposed to be involved in cell signaling and communication (Sohel, 2016). miRNAs presence in body fluids can be due to several concomitant processes, including tissue damage, cell apoptosis and necrosis, active release in exosomes and microvesicles, or association with proteins (O’Brien et al., 2018).
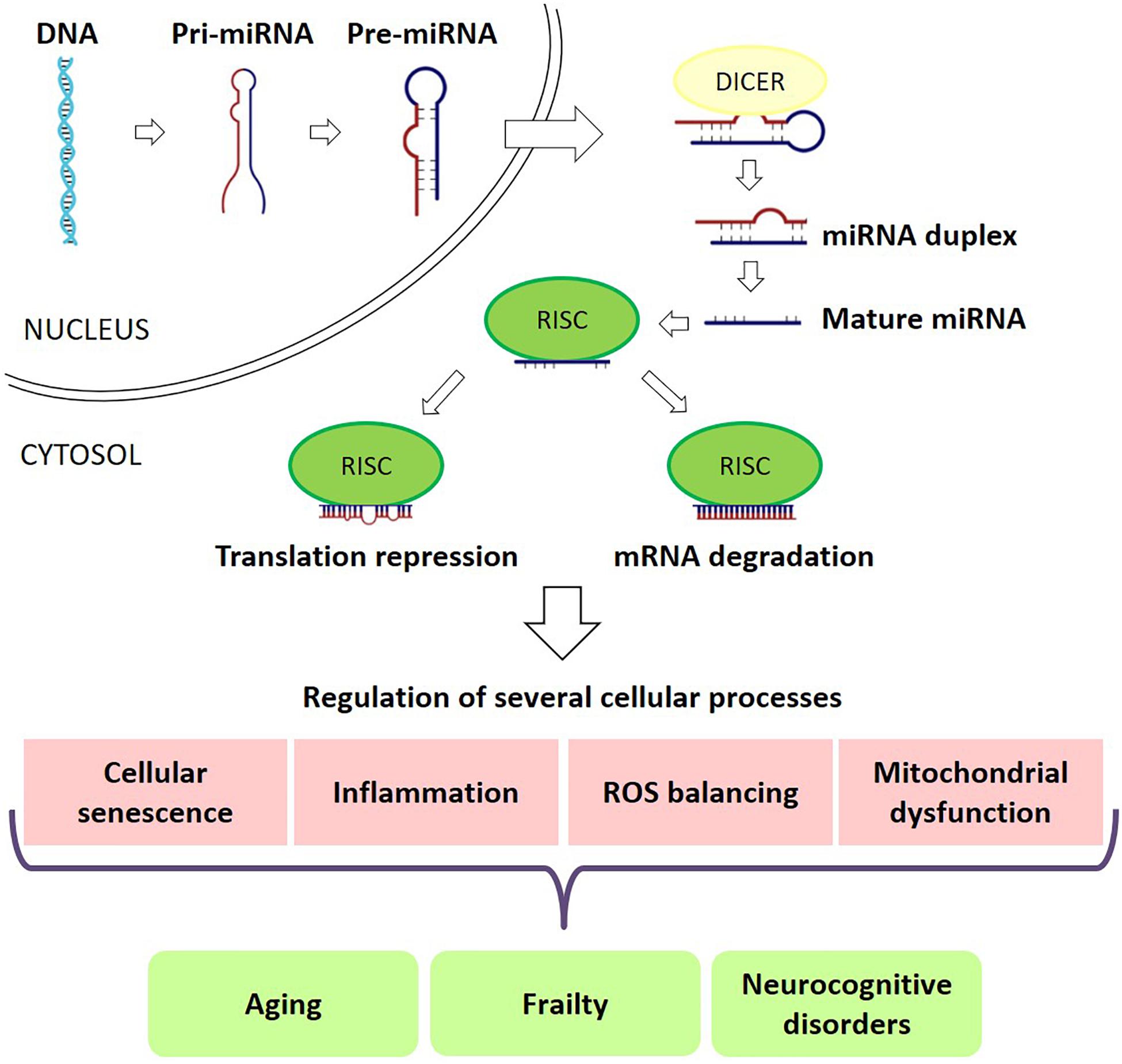
Figure 1. miRNAs in frailty and cognitive deficits. miRNAs play a major role in RNA silencing and post-transcriptional regulation of gene expression. miRNAs target hundreds of transcripts to regulate various biological pathways and processes, repressing translation or inducing mRNA degradation of target transcripts through sequence-specific binding. miRNAs are key modulators of almost all physiological processes and, consequently, miRNA dysregulation is seen in a multiplicity of diseases, including frailty and cognitive deficits.
In the present manuscript, after introducing the multiple clinical aspects and the main cellular mechanisms proposed to be associated with frailty and cognitive impairment, we designed a narrative review on the studies in which miRNAs have been proposed as peripheral circulating biomarkers for frailty or cognitive impairment, with the final aim to identify miRNAs that might be associated with cognitive frailty.
Clinical, Cellular, and Molecular Mechanisms of Frailty: The Potential Role of miRNAs
Clinical Features of Frailty
Frailty is generally considered as a geriatric syndrome, characterized by an excessive vulnerability to endogenous and exogenous stressors, due to a decrease in physiological reserves, thus leading to a high risk of developing adverse health outcomes (Clegg et al., 2013; Proietti and Cesari, 2020).
The majority of studies are based on the definition of frailty introduced by Fried and collaborators in 2001. The Fried phenotype also known as the frailty phenotype model defines frailty as a clinical syndrome in which three or more of the following criteria are present: unintentional weight loss, fatigue or self-reported exhaustion, weakness (poor grip strength), slow walking speed, and reduced or absent physical activity (Fried et al., 2001). This definition, exclusively considering the physical domain, is most frequently used for determining “physical frailty.” It should be mentioned that a key contribution to physical frailty comes from sarcopenia, defined as a progressive loss of skeletal muscle mass and strength (Ardeljan and Hurezeanu, 2021). Sarcopenia and frailty often co-exist in older patients, presenting a significant overlap of physical symptoms (Martin and Ranhoff, 2020). Indeed, sarcopenia is viewed as an essential correlate of the physical component of the frailty phenotype, although frailty can also be present in the absence of sarcopenia, suggesting the existence of several phenotypes of frailty (Davies et al., 2018).
In the same year in which Fried published the clinical criteria of physical frailty, other authors started to recognize that frailty was not exclusively characterized by physical impairments, but could be considered a more complex condition, involving other functional domains. Indeed, Rockwood and Mitnitski proposed the so-called Frailty Index (or Frailty Index of Deficit Accumulation) (Mitnitski et al., 2001), which is based on the concept that aging is a continuous process characterized by several deficits (including diseases, signs, symptoms, laboratory abnormalities, cognitive decline, and disabilities in activities of daily living), the accumulation of which may lead to frailty. Accordingly, the Frailty Index is defined as the proportion of accumulated deficits, thus representing the probability of an individual being frail (Martin and O’Halloran, 2020).
Other definitions of frailty exist, but the Fried Frailty Score and the Frailty Index are the most frequently used in clinical practice (Dent et al., 2016; Lekan et al., 2021).
More recently, a novel model of frailty has been proposed, based on a multidimensional evaluation considering the loss of harmonic interaction between multiple domains, including genetic, biological, functional, cognitive, psychological, and socio-economic dimensions, that ultimately leads to homeostatic instability (Pilotto et al., 2008, 2020). This multidimensional approach exploits the instruments of the comprehensive geriatric assessment (CGA). Operatively, CGA uses specific scales that explore functional disability, cognition, depression, nutritional status, comorbidities, number of drugs used, falls and pressure sores risk, cohabitation status, social and welfare context. This view, considering both multimorbidity and polypharmacy, allows for the evaluation of multidimensional impairment of the subject and promises to help the appropriateness of prescribing and intervention in frail older adults (Pilotto et al., 2018).
The prevalence of frailty has been assessed in many studies worldwide, although the results are highly variable, essentially depending on the definition used for indicating frailty. Overall, frailty has a prevalence estimated at around 11–16% in the population 60 years and older (Rohrmann, 2020; O’Caoimh et al., 2021). Frailty is more prevalent in women compared to men and as expected, prevalence increased with age, being the highest in subjects over 85 years (Collard et al., 2012; Rohrmann, 2020).
Cellular and Molecular Mechanisms of Frailty
In the last years, great efforts have been made to discover the molecular mechanisms underlying frailty. A gradual decrease in physiological reserve occurs with physiological aging but, in frailty, this decrease is accelerated, and homeostatic mechanisms start to fail (Clegg et al., 2013). Although lifelong accumulation of molecular and cellular damages is believed as a key element of both physiological aging and frailty, the interplay among dysfunctions in the brain, endocrine system, immune system, and skeletal muscle functions is recognized as a main factor in the development of frailty (Figure 2; Clegg et al., 2013). In the following paragraphs, we resume the main systemic and cellular processes recognized to be involved in frailty pathophysiology, including changes in the immune system, cellular senescence, and hormonal imbalance.
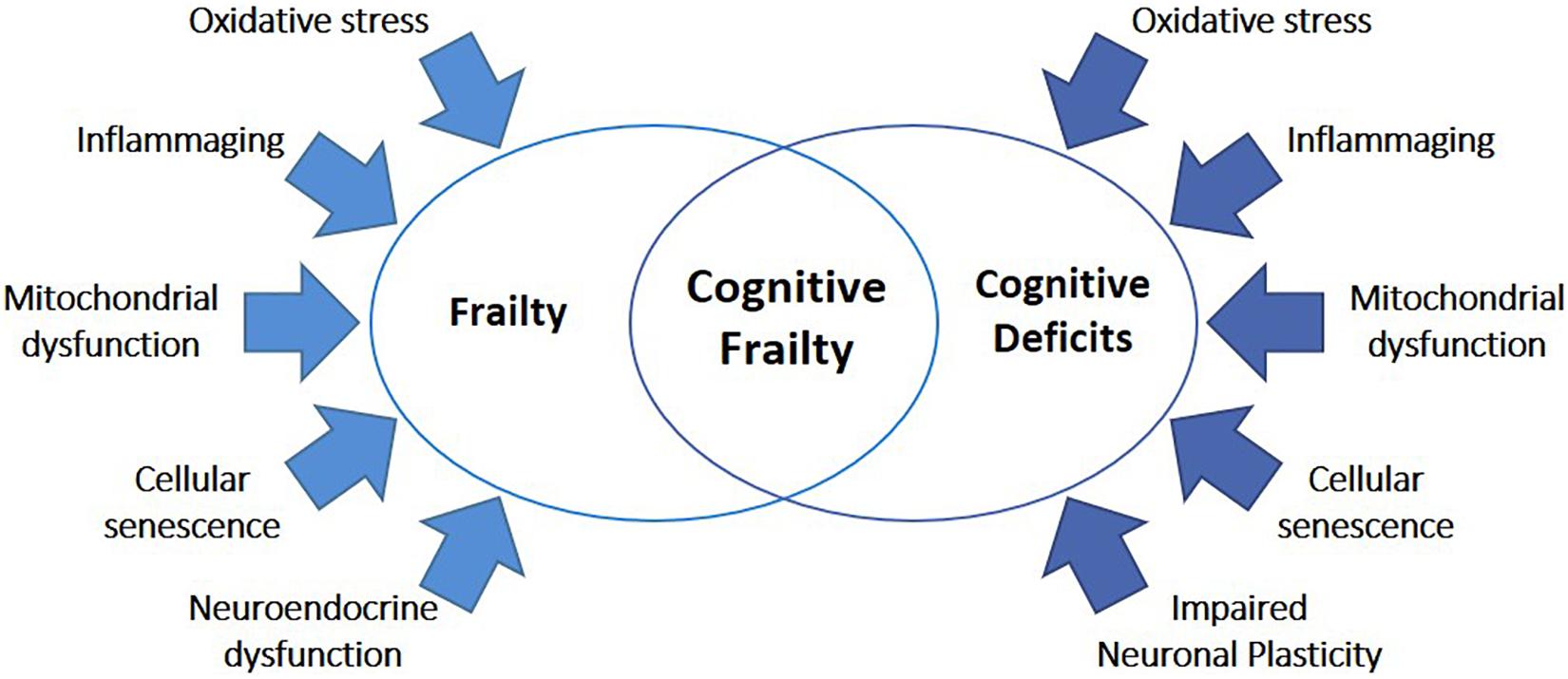
Figure 2. Common mechanisms underlying frailty and cognitive deficits. The high majority of mechanisms known to be involved in frailty were also implicated in cognitive diseases, including oxidative stress, inflammaging, mitochondrial dysfunction, cellular senescence, neuroendocrine dysfunctions, and impaired neuronal plasticity.
Changes in the Immune System and Related Musculoskeletal Consequences
Aging is associated with dramatic changes in the immune system, implying both immunosenescence (the decline in immune function with aging), and inflammaging (a state of chronic inflammation), which are considered to be main risk factors for age-related diseases (Franceschi et al., 2000; Fulop et al., 2015, 2018). Immunosenescence is characterized by altered T and B cells responses due to a modified naïve/memory cell ratio. Accumulation of memory T cells and reduction of peripheral blood naïve T cells are observed as a result of developmentally programmed thymic involution, increased serum levels of IgG and IgA, and a poor response to newly encountered microbial antigens (Pawelec, 2018). On the other hand, inflammaging is characterized by increasing circulating pro-inflammatory factors and decreasing circulatory anti-inflammatory factors (Franceschi et al., 2018). Remarkably, frail people have both immunosenescence (Lang et al., 2010) and inflammaging (Soysal et al., 2016).
The immune system plays, directly and indirectly, a role in age-associated muscle decline. Multiple immune cells have been implicated in muscle repair and regeneration, by controlling the local inflammatory responses and promoting muscle growth through releasing growth factors (Xu et al., 2020). Moreover, inflammatory cytokines have a major role in muscle homeostasis, activating muscle breakdown to generate amino acids for energy and cleave antigenic peptides. However, the overactive, insufficiently regulated inflammatory response that characterizes aging and frailty could result in loss of muscle mass and strength, with an associated reduction in functional ability (Clegg et al., 2013; Wilson et al., 2017). Accordingly, as already mentioned in the introduction, sarcopenia is considered as a key component of frailty as well as a predictor of morbidity, disability, and death in older people (Cooper et al., 2012; Nascimento et al., 2018).
Cellular Senescence
Cellular repair and regeneration are key elements in tissue homeostasis (Lazzeri et al., 2012). Aging is characterized by the loss of tissue regenerative properties and the accumulation of senescent cells, which is a defense mechanism preventing genomic instability (Bisset and Howlett, 2019). Senescent cells are non-dividing cells, highly metabolically active, that gradually acquire a secretory phenotype called senescence-associated secretory phenotype (SASP) (Cardoso et al., 2018). SASP contains a variety of factors, including proinflammatory and matrix modifying peptides, which negatively influence tissue homeostasis and regeneration (Zampino et al., 2020) and are causally linked to increased inflammaging (Korolchuk et al., 2017). SASP has also been shown to be involved in the pathogenesis of several age-related diseases and conditions, including frailty (LeBrasseur et al., 2015; Schafer et al., 2020).
Senescence is associated with dysregulated mitophagy and mitochondrial dysfunction (Chapman et al., 2019), leading to enhanced levels of reactive oxygen species (ROS), which in turn contribute to the development of senescent phenotype (Korolchuk et al., 2017), age-related diseases, and frailty (El Assar et al., 2020; Ferrucci and Zampino, 2020).
Hormonal Imbalance
During aging, hormonal axes suffer significant changes. The endocrine system is considered particularly important in frailty, because of its complex inter-relationships with the brain, immune system, and skeletal muscle (Clegg and Hassan-Smith, 2018). Anabolic hormones, such as androgens and insulin-like growth factor-1 (IGF-1), play a key role in stimulating protein synthesis, muscle growth, and insulin secretion. Strong evidence suggested that the levels of these hormones decline with age (Bisset and Howlett, 2019) and their alteration have been associated with frailty (Morley and Malmstrom, 2013). Adrenocorticotropic Hormone (ACTH) and cortisol secretion are also altered during aging and frailty leading to an impaired ability to recover from stressful stimuli in older people (Yiallouris et al., 2019). The dysregulation of multiple hormones has been proposed as one potential mechanism underlying frailty since preliminary evidence indicates that the cumulative burden of hormone deficiencies in frailty may be more important than the type of hormonal change (Bisset and Howlett, 2019).
miRNAs and Frailty
miRNAs are emerging as promising non-invasive diagnostic and prognostic biomarkers, as well as potential therapeutic agents (Vatic et al., 2020). Indeed, they could be used both to help understand physiopathological processes, and as novel therapeutic strategies allowing the simultaneous targeting of different pathways (Cardoso et al., 2018).
The study of miRNAs is a growing area of interest in the aging field. miRNAs regulate several biological events related to the aging process but are also influenced by aging processes themselves (Figure 1). At the same time, miRNAs have been consistently linked with the main systemic and cellular processes discussed above as associated with frailty. Indeed, some miRNAs, defined as “inflamma-miRs,” are involved in inflammatory pathways modulation and are differentially expressed during inflammaging (Quinn and O’Neill, 2011; Boldin and Baltimore, 2012; Olivieri et al., 2013, 2017). miRNAs play a pivotal role also in sarcopenia, regulating different aspects of muscle homeostasis (Sannicandro et al., 2019; Kinser and Pincus, 2020; Yin et al., 2020). Moreover, other miRNAs, the so-called senescence-associated miRNAs (SA-miRs) are involved in crucial biological processes of cellular senescence such as apoptosis, mitochondrial metabolism, and mitochondrial dynamics (Bu et al., 2017; Geiger and Dalgaard, 2017; Suh, 2018).
Several studies have reported differential miRNA expression between young and older individuals without discriminating for a frail phenotype (ElSharawy et al., 2012; Olivieri et al., 2012; Serna et al., 2012; Noren Hooten et al., 2013; Smith-Vikos et al., 2016) reviewed in Chen et al. (2010) and Lai et al. (2019). Conversely, to the best of our knowledge, only two studies directly evaluated changes in blood plasma miRNAs in frailty (Table 1).
Ipson and collaborators examined the changes of plasma-derived exosome miRNA profiles in frailty, comparing young, old robust, and frail individuals. They identified eight miRNAs that were enriched in frailty: miR-10a-3p, miR-92a-3p, miR-185-3p, miR-194-5p, miR-326, miR-532-5p, miR-576-5p, and miR-760 (Ipson et al., 2018). The second study evaluated the levels of three inflammation-related miRNAs (miR-21, miR-146a, and miR-223) and one miRNA related to the control of melatonin synthesis (miR-483) in plasma samples of healthy adults, older robust, and frail patients. Frail subjects had higher miR-21 levels than controls, whereas miR-223 and miR-483 levels increased in both aged groups (Rusanova et al., 2018).
Although very preliminary, these two studies identified possible novel candidate biomarkers for frailty in old age. Intriguingly, some of these miRNAs were also related to cellular mechanisms involved in frailty pathogenesis. For example, miR-21 is counted among inflamma-miRs and is known to target a variety of molecules belonging to the NF-κB/NLRP3 pathways, thus modulating the “switch on/off of inflammation (Olivieri et al., 2013, 2021). miR-10a has been involved in inflammation as well (Tahamtan et al., 2018), while expression of miR-185-3p, miR-194-5p, and miR-760 have been associated with cellular senescence and ROS production (Lee et al., 2014; Bu et al., 2017; Xu et al., 2017; Suh, 2018; Li et al., 2020; Zhang et al., 2021). miR-194-5p and miR-92a-3p were reported to regulate muscle cell homeostasis (Morton et al., 2021; Shi et al., 2021a).
Moreover, some of these frailty-related miRNAs seem to play a major role also in neurons. Indeed, miR-326 inhibits neuronal apoptosis and attenuates mitochondrial damage (He et al., 2020; Huang et al., 2021). miR-532-5p showed a neuroprotective effect reducing apoptosis, ROS production, and inflammation in cerebral ischemia-reperfusion injury (Shi et al., 2021b), and ischemic stroke (Mu et al., 2020), while mir-92a-3p, belonging to the miR-17–92 family, is a synaptic-related miRNA (Siedlecki-Wullich et al., 2021), involved in neural cells proliferation, differentiation, and maturation (Zhang et al., 2013; Xia et al., 2020).
Cognitive Impairment: The Potential Role of miRNAs
Clinical Features of Cognitive Impairment
As we age, some cognitive abilities, such as language, vocabulary, and verbal skills, remain largely unchanged but other abilities, such as conceptual reasoning, memory, and processing speed, can physiologically decline gradually over time (Harada et al., 2013). Although general knowledge and crystallized intelligence are mostly unaffected during aging, fluid intelligence, which is the ability to learn and use new information and use it to problem-solve, is more affected (Deary et al., 2009).
Cognitive disorders are a general umbrella term that describes a group of conditions characterized by impairment in cognitive abilities such as memory, problem solving, and perception (Sachdev et al., 2013). Cognitive abilities are usually assessed through the administration of specific tests, i.e., the mini-mental state examination (MMSE) (Folstein et al., 1975) and the Montreal Cognitive Assessment (MoCA) (Nasreddine et al., 2005). Among cognitive disorders, mild cognitive impairment (MCI) is increasing in attention by researchers, as demonstrated by the introduction in the DSM-5. This entity can be identified in presence of: (1) modest cognitive decline from a previous level of performance in one or more cognitive domains, greater than expected for age, without falling into the dementia range, (2) no interference with capacity for independence in everyday activities, (3) cognitive deficits not occurring exclusively in the context of a delirium, and (4) cognitive deficits not explained by another mental disorder (Ganguli, 2013; Sachdev et al., 2013). MCI affects about 3–22% of the population over the age of 65 (Stokin et al., 2015; Sanford, 2017), symptoms may remain stable for years, with some cases may revert to normality (Sachdev et al., 2013), but it is estimated that about 50% of people affected by MCI can progress to dementia, particularly Alzheimer’s disease (AD) (Gordon and Martin, 2013).
Cellular and Molecular Mechanisms Underlying Cognitive Impairment
Brain aging is the main predisposing factor for cognitive impairment (Yankner et al., 2008). As for frailty, the main mechanisms involved in cognitive disorders are also implicated in physiological aging (Figure 2). However, while a decline in cognitive features is expected during physiological aging, differently from pathological aging associated with cognitive decline, this does not result in any significant functional impairment (Schirinzi et al., 2020).
The pathophysiological mechanisms of cognitive disorders essentially comprise alterations of synaptic transmission, oxidative stress, cellular senescence, and increased inflammation.
Alterations of Synaptic Function
The maintenance of synaptic function requires the preservation of the proper synaptic structure, coordination of synaptic vesicle release and membrane excitability, and integration of retrograde signals from the postsynaptic terminal (Azpurua and Eaton, 2015). Aging is associated with physiological structural changes in the brain, including the reduction of the number and function of synapses in brain areas related to learning and memory (Burke and Barnes, 2006; Lupien et al., 2009; Cuestas Torres and Cardenas, 2020). However, beyond the physiological aging processes, more generalized synaptic deficits can induce cognitive disorders. The study of cellular mechanisms underlying cognitive impairment highlighted the role of synaptic dysfunction and synaptopathy, defined as an alteration of synaptic homeostasis leading to a high risk of degeneration and synaptic loss (Stephan et al., 2012; Skaper et al., 2017). Pathological changes identified in synaptic dysfunction include plaque and tangle formation, vascular pathologies, neurochemical deficits, cellular injury, oxidative stress, mitochondrial changes, inflammation, changes in genomic activity, disturbed protein metabolism (Stephan et al., 2012).
Oxidative Stress and Cellular Senescence
Neurons are postmitotic polarized cells with significant energy demands and mitochondria play a pivotal role in generating the ATP required to support electrochemical neurotransmission, synaptic plasticity, neural cell maintenance, and repair (Lejri et al., 2019). Defects in mitochondrial dynamics and quality control, together with inefficient mitochondrial transport and distribution in synaptic compartments, have been implicated in synaptic/neuronal degeneration and brain aging (Grimm and Eckert, 2017; Raefsky and Mattson, 2017). Apart from the production of energy, mitochondria are key modulators of brain cell survival and death by controlling calcium and redox equilibrium, producing ROS, and controlling cell apoptosis (Mattson and Arumugam, 2018). Cellular, biochemical, and molecular studies showed a clear link between oxidative stress and cognitive dysfunction during aging and age-associated neuronal diseases (Kandlur et al., 2020). Neurons are particularly vulnerable to oxidative insults: ROS may induce the activation of neuroinflammation and neuronal death, with mechanisms involving glutamate excitotoxicity, aspartate receptor signaling, and glucocorticoid receptor activation (Grimm and Eckert, 2017). Oxidative injury can alter brain plasticity, cell proliferation, neurogenesis, and synaptic neurotransmission while enhancing neuronal death and impairing normal synaptic neurotransmission (Castelli et al., 2019). Moreover, mitochondrial dysfunctions and ROS production trigger cell senescence of neurons and glial cells, which in turn contributes to changes in morphological and functional alterations associated with synaptopathy (Morley, 2018; Toricelli et al., 2021). Indeed, senescent cells secrete pro-inflammatory SASP factors and disrupt the cell-cell contacts needed for the structural and functional neuron–glial interaction that maintains neuronal homeostasis (Chinta et al., 2015).
Inflammation
The central nervous system is traditionally thought of as an immunologically privileged space, isolated from the immune system, and separated from peripheral immune cells that are unable to cross the blood-brain barrier. However, it is now accepted that there is a wide and constant bidirectional communication between the peripheral immune system and the central nervous system (Engelhardt et al., 2017). Indeed, it has been demonstrated that signals from a systemic inflammatory condition may contribute to brain immune cell population activation, which in turn may accelerate neuronal degeneration and/or cognitive decline, leading to exacerbation of a clinical condition (Perry, 2004). Although neuroinflammation serves several fundamental roles in the brain structure and function, chronic inflammation may instead cause an exaggerated response (Tangestani Fard and Stough, 2019). Resident glial cells, including microglia and astrocytes, become hyperactivated in response to inflammatory stimuli and sustain a high-level production of proinflammatory cytokines, chemokines, secondary messengers, and ROS (Shabab et al., 2017; Slota and Booth, 2019). This altered inflammatory status may contribute to the onset of cognitive impairment in older people and enhances the state of vulnerability to environmental challenges (Brivio et al., 2019).
miRNAs and Cognitive Impairment
miRNAs have been shown to play a major role in the brain as key regulators of neuronal development from neural progenitor cells, cell migration, neuronal polarization, and synapse formation (Nampoothiri and Rajanikant, 2017; Rajman and Schratt, 2017; Esteves et al., 2020). miRNAs can also modulate neuroinflammation (Thounaojam et al., 2013; Sarkar et al., 2019; Slota and Booth, 2019), formation of ROS, mitochondrial function, and cellular senescence (Bigagli et al., 2016; Konovalova et al., 2019; Catanesi et al., 2020; Figure 1). Accordingly, it has been suggested that cognitive dysfunctions in aging may be predicted by selected alterations of miRNAs expression (Danka Mohammed et al., 2017; Hernandez-Rapp et al., 2017). Recently, the involvement of miRNAs in cognitive disorders has been extensively studied, measuring their levels in different body fluids, such as plasma, serum, urine, and cerebrospinal fluid (Grasso et al., 2014; Basak et al., 2016).
Changes in miRNA expression have been correlated with cognitive performance and decline.
Kondo and collaborators examined the association between cognitive function and serum levels of six miRNAs (miR-let-7d, miR-17, miR-20a, miR-27a, miR-34a, miR-103a) in 337 Japanese subjects who had never been diagnosed with dementia. This study identified a positive correlation between the serum levels of miR-20a, miR-27a, and miR-103a and MMSE scores. Thus, low serum miR-20a, miR-27a, and miR-103a levels were significantly associated with cognitive deficits and were proposed as markers of early-stage cognitive decline (Kondo et al., 2019).
A recent study utilized machine learning approaches as a broad cognitive screening instrument to determine whether miRNAs could be proposed as blood-based biomarkers of cognitive aging (Gullett et al., 2020). Top 10 most important miRNAs for predicting total cognitive performance include miR-140-5p, miR-197-3p, miR-501-3p, miR-425-5p, miR-532-5p, miR-378a-5p, miR-411-3p, miR-181c-3p, miR-497-5p, miR-214-3p. Instead, three miRNAs (miR-140-5p, miR-197-3p, miR-501-3p) were top-ranked predictors of multiple cognitive outcomes (including fluid, crystallized, and overall cognition).
Furthermore, several studies addressed alterations of miRNA profiles in the blood of MCI patients and proposed miRNAs as specific diagnostic and/or prognostic biomarkers of MCI (reviewed in Piscopo et al., 2019). Overall, more than forty miRNAs were reported to discriminate between MCI and healthy controls in different studies, although only miR-206 was consistently found as differentially expressed in at least two reports (Piscopo et al., 2019). Specific studies on MCI patients are reported in Table 1.
Moreover, a recent meta-analysis of six microarray datasets identified 17 miRNAs as dysregulated in both MCI and AD [miR-16-5p, miR-92a-3p, miR-26b-5p, miR-106b-5p, miR-93-5p, miR-20a-5p, miR-320a, let-7a-5p, miR-484, miR-615-3p,miR-18a-3p 5, miR-7977, miR-17-5p, miR-155-5p, miR-193b-3p, miR-450a-1-3p, miR-887-5p, suggesting a key involvement in the modulation of cognitive function (Bottero and Potashkin, 2019)].
Other miRNAs were instead proposed as early biomarkers of MCI in the preclinical stage, or for prodromal AD. miRNA pairs in the miR-132 family (miR-128/miR-491-5p, miR-132/miR-491-5p, and mir-874/miR-491-5p) and the miR-134 family (miR-134/miR-370, miR-323-3p/miR-370, and miR-382/miR-370), although not differentiating MCI from AD, were proposed as predictive markers for the onset of MCI (Sheinerman et al., 2012, 2013). On the other hand, Kenny and collaborators, based on a 4-year longitudinal evaluation, found increased miR-206 levels in MCI patients at high risk of dementia (tested with the Clinical Dementia Rating, CDR) and in MCI patients with deteriorating MMSE scores. Indeed, stable MCI subjects displayed little to no change in expression over the years, while MCI patients who progressed toward dementia displayed significantly higher levels of miR-206 (Kenny et al., 2019). Moreover, while upregulation of miR-92a, miR-181c, and miR-210 levels was reported in plasma of both MCI and AD patients, the signature values in the plasma of the MCI patients that progressed to AD were found to be significantly higher than the values found in the MCI patients that did not progress to dementia (Siedlecki-Wullich et al., 2019). Altogether, these data suggest that plasma levels of miR-206, miR-92a-3p, miR-181c-5p, and miR-210-3p could be used as molecular signatures of AD progression in MCI. Finally, very recently, Qin and collaborators, identified two miRNAs, miR-6764-5p and miR-6734-3p, as remarkably upregulated in both MCI and AD subjects compared to controls (Qin et al., 2021).
miRNAs reported in at least two studies as associated with cognitive function are highlighted in blue in Table 1.
Cognitive Frailty: The Potential Role of miRNAs
Cognitive Frailty: Definitions
Several shreds of evidence demonstrated that frailty and cognitive impairment are intrinsically related, since frailty is known to increase risk of cognitive decline, and cognitive decline may increase risk of frailty and have an impact on the trajectory of frailty (as recent reviews see Kiiti Borges et al., 2019; Welstead et al., 2020; Bu et al., 2021). The concept of simultaneous presence of frailty and cognitive impairment or cognitive frailty was initially proposed in 2013 by the International Institute of Nutrition and Aging and the International Geriatrics Association (IANA), defined by the presence of physical frailty and cognitive impairment, and exclusion of concurrent dementia (Kelaiditi et al., 2013). Although the concept of cognitive frailty is well accepted and has been shown to be associated with poor outcomes, there is yet no consensus on the actual definition (Merchant et al., 2021). Indeed, multiple definitions and terminologies have been proposed, including Motoric Cognitive Risk Syndrome (MCR), defined as presence of both slow gait speed and subjective cognitive complaints and absence of concurrent dementia or mobility disability (Verghese et al., 2014), or Physio-cognitive Decline Syndrome (PCDS), defined by slowness and/or weakness and ≥ 1.5 SD below age/sex/education-matched norms in any cognitive function domain (Chen and Arai, 2020). Moreover, Ruan and collaborators proposed a new classification of cognitive frailty, in which they distinguish “reversible” from “potential reversible” cognitive frailty. Reversible cognitive frailty was defined by the presence of physical/pre-physical frailty and subjective cognitive decline and/or positive fluid and imaging biomarkers of amyloid accumulation and neurodegeneration, while potentially reversible cognitive frailty was defined by the presence of physical/pre-physical frailty and cognitive impairment (Ruan et al., 2015).
Nevertheless, recent evidence suggests that, regardless of the specific definition, cognitive frailty is a target for preventing disability and dementia through multi-domain interventions, considering physical, nutritional, cognitive as well as psychological domains, with the final aim to modify the trajectory of frailty and cognitive decline toward positive outcomes.
Even though epidemiological and clinical studies have demonstrated a close relationship between frailty and cognitive diseases, the common/concurring molecular mechanisms are still largely unknown. Nevertheless, it has been proposed that abnormalities in biological processes related to physiological aging could play a major role in both conditions (Ruan et al., 2015; Searle and Rockwood, 2015). In particular, chronic inflammation, immunosenescence, imbalanced energy metabolism, mitochondrial dysfunction, oxidative stress, and neuroendocrine dysfunctions may be all involved in cognitive frailty (Figure 2; Mulero et al., 2011; Robertson et al., 2013; Fulop et al., 2018; Sargent et al., 2018; Fabricio et al., 2020; Ma and Chan, 2020).
Putative Role of miRNAs in Cognitive Frailty
As regards the possible role of miRNAs in the pathogenesis of frailty with cognitive impairment and/or their potential use as biomarkers, to date, no studies are available considering cognitive frailty as a single condition. Furthermore, as reported above, there are only two studies analyzing changes in blood miRNAs specifically in frail subjects, while more evidence has been collected regarding cognitive impairment.
Although the limited information available makes it hard to depict a comprehensive picture of possible common miRNAs involved in both frailty and cognitive impairment, our review effort identified two miRNAs which were reported to be both differentially expressed in frail people and associated with cognitive deficits: miR-92a-3p and miR-532-5p (Table 1). Mature miR-92a-3p belongs to miR-17-92 cluster, located on chromosome 13 in the human genome. The miR-17-92 cluster, containing six miRNA precursors (miR-17, miR-18a, miR-19a, miR-20a, miR-19b-1, and miR-92a), is highly conserved among vertebrates and has fundamental roles during development (Concepcion et al., 2012; Mogilyansky and Rigoutsos, 2013). miR-92a-3p is a synaptic-related miRNA (Siedlecki-Wullich et al., 2021), involved in neural cells proliferation, differentiation, and maturation (Zhang et al., 2013; Xia et al., 2020). Intriguingly, it has been recently identified as a peripheral biomarker in different diseases, among which systemic lupus erythematosus (Kim et al., 2016), schizophrenia (Ma et al., 2018), and amyotrophic lateral sclerosis (Joilin et al., 2020). Moreover, miR-92a-3p was reported to increase ROS in mice (Gou et al., 2018), to regulates cartilage development and homeostasis (Mao et al., 2018), to participate in age-related pathophysiological processes including atherosclerosis and lipid metabolism (Loyer et al., 2014), cerebral white matter impairment (He et al., 2017), and cancer (Reis et al., 2020; Wang et al., 2021).
Mature miR-532-5p derived from pre-miR-532 which is localized on chromosome X in the human genome. miR-532-5p showed a neuroprotective effect reducing apoptosis, ROS production, and inflammation in cerebral ischemia-reperfusion injury (Shi et al., 2021b), and ischemic stroke (Mu et al., 2020). Moreover it has been implicated in inflammation (Yan et al., 2020), osteoporosis (Guo et al., 2020), as well as in tumor progression (Kim et al., 2021; Yu et al., 2021).
Conclusion
In this review, we explored the possible use of miRNAs as both potential biomarkers and molecular effectors of frailty and cognitive impairment. We discussed the evidence linking changes in circulating miRNAs expression with these clinical conditions, with the final aim of shedding light on miRNAs that might be associated with cognitive frailty.
One of the limits of this study is that evidence giving a clear mechanistic link between frailty (or cognitive impairment) and miRNAs is still missing. Moreover. to date, only two works analyzed miRNAs expression in the plasma of frail patients, as potential peripheral biomarkers of frailty (Ipson et al., 2018; Rusanova et al., 2018). No further studies have been performed to evaluate the molecular mechanisms leading to changes in miRNAs expression in frail subjects, nor analyzing a possible involvement of these miRNAs in frailty etiopathogenesis. The same could be stated for studies linking miRNAs with cognitive impairment. Nevertheless, some of the miRNAs found to be differentially expressed in the blood of frail or cognitively impaired subjects have been reported to play a key role in cellular mechanisms associated with frailty and cognitive deficits, such as cellular senescence, oxidative stress, mitochondrial dysfunction, or inflammation (Thounaojam et al., 2013; Bigagli et al., 2016; Bu et al., 2017; Suh, 2018; Tahamtan et al., 2018; Konovalova et al., 2019; Sarkar et al., 2019; Slota and Booth, 2019; Catanesi et al., 2020). This suggests that miRNAs could be considered more than peripheral biomarkers, fostering the idea that miRNAs could be mechanistically involved in the etiogenesis of both frailty and cognitive impairment.
In this context, although more studies are needed, existing literature may suggest a potential use of iR-92a-3p and miR-532-5p not only as biomarkers of cognitive frailty, but also as in the context of the study of molecular mechanisms of frailty and cognitive diseases. Besides miR-92a-3p and miR-532-5p, other miRNAs consistently implicated in cellular mechanisms underlying both frailty and cognitive dysfunction, such for instance inflamma-miRs, SA-miRs, and miRNAs regulating oxidative processes, could have potential as biomarkers and molecular effectors of cognitive frailty as well.
In conclusion, although many works have proposed miRNAs as biomarkers of frailty and cognitive decline, the study of differentially expressed miRNAs in frailty is at its infancy, and reports on cognitive frailty are still missing. The identification of selected miRNAs differentially modulated in cognitive frailty could pave the way for innovative diagnostic and prognostic strategies, which may help the clinical management of people suffering from this condition, improving their life expectancy and quality of life. Furthermore, the study of miRNAs involvement in etiological mechanisms of cognitive frailty represents a promising tool for the identification of new targets for the development of novel therapeutic approaches, thus modeling health trajectories toward positive outcomes.
Author Contributions
GC, LM, and AB: conceptualization. GC, LM, NV, and AB: writing—original draft. GC, LM, FB, AC, CF, AI, SM, MP, NV, and AB: writing—review and editing. All authors contributed to the article and approved the submitted version.
Funding
This work was supported by research grants from the Cariplo Foundation (Prog. 2014-1133 and 2017-0620).
Conflict of Interest
The authors declare that the research was conducted in the absence of any commercial or financial relationships that could be construed as a potential conflict of interest.
Publisher’s Note
All claims expressed in this article are solely those of the authors and do not necessarily represent those of their affiliated organizations, or those of the publisher, the editors and the reviewers. Any product that may be evaluated in this article, or claim that may be made by its manufacturer, is not guaranteed or endorsed by the publisher.
References
Alvarez-Garcia, I., and Miska, E. A. (2005). MicroRNA functions in animal development and human disease. Development 132, 4653–4662. doi: 10.1242/dev.02073
Azpurua, J., and Eaton, B. A. (2015). Neuronal epigenetics and the aging synapse. Front. Cell Neurosci. 9:208. doi: 10.3389/fncel.2015.00208
Basak, I., Patil, K. S., Alves, G., Larsen, J. P., and Moller, S. G. (2016). microRNAs as neuroregulators, biomarkers and therapeutic agents in neurodegenerative diseases. Cell Mol. Life Sci. 73, 811–827. doi: 10.1007/s00018-015-2093-x
Beard, J. R., Officer, A., de Carvalho, I. A., Sadana, R., Pot, A. M., Michel, J. P., et al. (2016). The World report on ageing and health: a policy framework for healthy ageing. Lancet 387, 2145–2154. doi: 10.1016/S0140-6736(15)00516-4
Bigagli, E., Luceri, C., Scartabelli, T., Dolara, P., Casamenti, F., Pellegrini-Giampietro, D. E., et al. (2016). Long-term neuroglial cocultures as a brain aging model: hallmarks of senescence, MicroRNA expression profiles, and comparison with in vivo models. J. Gerontol. A Biol. Sci. Med. Sci. 71, 50–60. doi: 10.1093/gerona/glu231
Bisset, E. S., and Howlett, S. E. (2019). The biology of frailty in humans and animals: understanding frailty and promoting translation. Aging Med. 2, 27–34. doi: 10.1002/agm2.12058
Boldin, M. P., and Baltimore, D. (2012). MicroRNAs, new effectors and regulators of NF-κB. Immunol. Rev. 246, 205–220. doi: 10.1111/j.1600-065X.2011.01089.x
Bottero, V., and Potashkin, J. A. (2019). Meta-analysis of gene expression changes in the blood of patients with mild cognitive impairment and alzheimer’s disease dementia. Int. J. Mol. Sci. 20:5403. doi: 10.3390/ijms20215403
Brivio, P., Paladini, M. S., Racagni, G., Riva, M. A., Calabrese, F., and Molteni, R. (2019). From healthy aging to frailty: in search of the underlying mechanisms. Curr. Med. Chem. 26, 3685–3701. doi: 10.2174/0929867326666190717152739
Bu, H., Wedel, S., Cavinato, M., and Jansen-Durr, P. (2017). MicroRNA regulation of oxidative stress-induced cellular senescence. Oxid. Med. Cell Longev. 2017:2398696. doi: 10.1155/2017/2398696
Bu, Z., Huang, A., Xue, M., Li, Q., Bai, Y., and Xu, G. (2021). Cognitive frailty as a predictor of adverse outcomes among older adults: a systematic review and meta-analysis. Brain Behav. 11:e01926. doi: 10.1002/brb3.1926
Burke, S. N., and Barnes, C. A. (2006). Neural plasticity in the aging brain. Nat. Rev. Neurosci. 7, 30–40. doi: 10.1038/nrn1809
Cardoso, A. L., Fernandes, A., Aguilar-Pimentel, J. A., de Angelis, M. H., Guedes, J. R., Brito, M. A., et al. (2018). Towards frailty biomarkers: candidates from genes and pathways regulated in aging and age-related diseases. Aging Res. Rev. 47, 214–277. doi: 10.1016/j.arr.2018.07.004
Castelli, V., Benedetti, E., Antonosante, A., Catanesi, M., Pitari, G., Ippoliti, R., et al. (2019). Neuronal cells rearrangement during aging and neurodegenerative disease: metabolism, oxidative stress and organelles dynamic. Front. Mol. Neurosci. 12:132. doi: 10.3389/fnmol.2019.00132
Catanesi, M., d’Angelo, M., Tupone, M. G., Benedetti, E., Giordano, A., Castelli, V., et al. (2020). MicroRNAs dysregulation and mitochondrial dysfunction in neurodegenerative diseases. Int. J. Mol. Sci. 21:5986. doi: 10.3390/ijms21175986
Chapman, J., Fielder, E., and Passos, J. F. (2019). Mitochondrial dysfunction and cell senescence: deciphering a complex relationship. FEBS Lett. 593, 1566–1579. doi: 10.1002/1873-3468.13498
Chen, L. H., Chiou, G. Y., Chen, Y. W., Li, H. Y., and Chiou, S. H. (2010). MicroRNA and aging: a novel modulator in regulating the aging network. Aging Res. Rev. 9(Suppl. 1), S59–S66. doi: 10.1016/j.arr.2010.08.002
Chen, L. K., and Arai, H. (2020). Physio-cognitive decline as the accelerated aging phenotype. Arch. Gerontol. Geriatr. 88:104051. doi: 10.1016/j.archger.2020.104051
Chinta, S. J., Woods, G., Rane, A., Demaria, M., Campisi, J., and Andersen, J. K. (2015). Cellular senescence and the aging brain. Exp. Gerontol. 68, 3–7. doi: 10.1016/j.exger.2014.09.018
Clegg, A., and Hassan-Smith, Z. (2018). Frailty and the endocrine system. Lancet Diabetes Endocrinol. 6, 743–752. doi: 10.1016/S2213-8587(18)30110-4
Clegg, A., Young, J., Iliffe, S., Rikkert, M. O., and Rockwood, K. (2013). Frailty in elderly people. Lancet 381, 752–762. doi: 10.1016/S0140-6736(12)62167-9
Collard, R. M., Boter, H., Schoevers, R. A., and Oude Voshaar, R. C. (2012). Prevalence of frailty in community-dwelling older persons: a systematic review. J. Am. Geriatr. Soc. 60, 1487–1492. doi: 10.1111/j.1532-5415.2012.04054.x
Concepcion, C. P., Bonetti, C., and Ventura, A. (2012). The microRNA-17-92 family of microRNA clusters in development and disease. Cancer J. 18, 262–267. doi: 10.1097/PPO.0b013e318258b60a
Condrat, C. E., Thompson, D. C., Barbu, M. G., Bugnar, O. L., Boboc, A., Cretoiu, D., et al. (2020). miRNAs as biomarkers in disease: latest findings regarding their role in diagnosis and prognosis. Cells 9:276. doi: 10.3390/cells9020276
Cooper, C., Dere, W., Evans, W., Kanis, J. A., Rizzoli, R., Sayer, A. A., et al. (2012). Frailty and sarcopenia: definitions and outcome parameters. Osteoporos. Int. 23, 1839–1848. doi: 10.1007/s00198-012-1913-1
Cuestas Torres, D. M., and Cardenas, F. P. (2020). Synaptic plasticity in Alzheimer’s disease and healthy aging. Rev. Neurosci. 31, 245–268. doi: 10.1515/revneuro-2019-0058
Danka Mohammed, C. P., Park, J. S., Nam, H. G., and Kim, K. (2017). MicroRNAs in brain aging. Mech. Aging Dev. 168, 3–9. doi: 10.1016/j.mad.2017.01.007
Davies, B., Garcia, F., Ara, I., Artalejo, F. R., Rodriguez-Manas, L., and Walter, S. (2018). Relationship between sarcopenia and frailty in the toledo study of healthy aging: a population based cross-sectional study. J. Am. Med. Dir. Assoc. 19, 282–286. doi: 10.1016/j.jamda.2017.09.014
Deary, I. J., Corley, J., Gow, A. J., Harris, S. E., Houlihan, L. M., Marioni, R. E., et al. (2009). Age-associated cognitive decline. Br. Med. Bull. 92, 135–152. doi: 10.1093/bmb/ldp033
Dent, E., Kowal, P., and Hoogendijk, E. O. (2016). Frailty measurement in research and clinical practice: a review. Eur. J. Intern. Med. 31, 3–10. doi: 10.1016/j.ejim.2016.03.007
Dong, H., Li, J., Huang, L., Chen, X., Li, D., Wang, T., et al. (2015). Serum MicroRNA profiles serve as novel biomarkers for the diagnosis of Alzheimer’s disease. Dis. Markers 2015:625659. doi: 10.1155/2015/625659
El Assar, M., Angulo, J., and Rodríguez-Mañas, L. (2020). Frailty as a phenotypic manifestation of underlying oxidative stress. Free Radic. Biol. Med. 149, 72–77. doi: 10.1016/j.freeradbiomed.2019.08.011
ElSharawy, A., Keller, A., Flachsbart, F., Wendschlag, A., Jacobs, G., Kefer, N., et al. (2012). Genome-wide miRNA signatures of human longevity. Aging Cell 11, 607–616. doi: 10.1111/j.1474-9726.2012.00824.x
Engelhardt, B., Vajkoczy, P., and Weller, R. O. (2017). The movers and shapers in immune privilege of the CNS. Nat. Immunol. 18, 123–131. doi: 10.1038/ni.3666
Esteves, M., Serra-Almeida, C., Saraiva, C., and Bernardino, L. (2020). New insights into the regulatory roles of microRNAs in adult neurogenesis. Curr. Opin. Pharmacol. 50, 38–45. doi: 10.1016/j.coph.2019.11.003
Fabricio, D. M., Chagas, M. H. N., and Diniz, B. S. (2020). Frailty and cognitive decline. Transl. Res. 221, 58–64. doi: 10.1016/j.trsl.2020.01.002
Ferrucci, L., and Zampino, M. (2020). A mitochondrial root to accelerated aging and frailty. Nat. Rev. Endocrinol. 16, 133–134. doi: 10.1038/s41574-020-0319-y
Folstein, M. F., Folstein, S. E., and McHugh, P. R. (1975). “Mini-mental state”. A practical method for grading the cognitive state of patients for the clinician. J. Psychiatr. Res. 12, 189–198. doi: 10.1016/0022-3956(75)90026-6
Franceschi, C., Bonafe, M., Valensin, S., Olivieri, F., De Luca, M., Ottaviani, E., et al. (2000). Inflamm-aging. An evolutionary perspective on immunosenescence. Ann. N.Y. Acad. Sci. 908, 244–254. doi: 10.1111/j.1749-6632.2000.tb06651.x
Franceschi, C., Garagnani, P., Parini, P., Giuliani, C., and Santoro, A. (2018). Inflammaging: a new immune-metabolic viewpoint for age-related diseases. Nat. Rev. Endocrinol. 14, 576–590. doi: 10.1038/s41574-018-0059-4
Fried, L. P., Tangen, C. M., Walston, J., Newman, A. B., Hirsch, C., Gottdiener, J., et al. (2001). Frailty in older adults: evidence for a phenotype. J. Gerontol. A Biol. Sci. Med. Sci. 56, M146–M156. doi: 10.1093/gerona/56.3.m146
Fulop, T., McElhaney, J., Pawelec, G., Cohen, A. A., Morais, J. A., Dupuis, G., et al. (2015). Frailty, inflammation and immunosenescence. Interdiscip. Top. Gerontol. Geriatr. 41, 26–40. doi: 10.1159/000381134
Fulop, T., Witkowski, J. M., Olivieri, F., and Larbi, A. (2018). The integration of inflammaging in age-related diseases. Semin. Immunol. 40, 17–35. doi: 10.1016/j.smim.2018.09.003
Ganguli, M. (2013). Can the DSM-5 framework enhance the diagnosis of MCI? Neurology 81, 2045–2050. doi: 10.1212/01.wnl.0000436944.01023.e5
Geiger, J., and Dalgaard, L. T. (2017). Interplay of mitochondrial metabolism and microRNAs. Cell Mol. Life Sci. 74, 631–646. doi: 10.1007/s00018-016-2342-7
Gordon, C., and Martin, D. J. (2013). Mild cognitive impairment. Expert Rev. Neurother. 13, 1247–1261. doi: 10.1586/14737175.2013.856265
Gou, L., Zhao, L., Song, W., Wang, L., Liu, J., Zhang, H., et al. (2018). Inhibition of miR-92a Suppresses Oxidative Stress and Improves Endothelial Function by Upregulating Heme Oxygenase-1 in db/db Mice. Antioxid. Redox. Signal. 28, 358–370. doi: 10.1089/ars.2017.7005
Grasso, M., Piscopo, P., Confaloni, A., and Denti, M. A. (2014). Circulating miRNAs as biomarkers for neurodegenerative disorders. Molecules 19, 6891–6910. doi: 10.3390/molecules19056891
Grimm, A., and Eckert, A. (2017). Brain aging and neurodegeneration: from a mitochondrial point of view. J. Neurochem. 143, 418–431. doi: 10.1111/jnc.14037
Gullett, J. M., Chen, Z., O’Shea, A., Akbar, M., Bian, J., Rani, A., et al. (2020). MicroRNA predicts cognitive performance in healthy older adults. Neurobiol. Aging 95, 186–194. doi: 10.1016/j.neurobiolaging.2020.07.023
Guo, X., Wei, S., Xu, F., Cai, X., Wang, H., and Ding, R. (2020). MicroRNA-532-5p is implicated in the regulation of osteoporosis by forkhead box O1 and osteoblast differentiation. BMC Musculoskelet. Disord. 21:296. doi: 10.1186/s12891-020-03317-y
Halil, M., Cemal Kizilarslanoglu, M., Emin Kuyumcu, M., Yesil, Y., and Cruz Jentoft, A. J. (2015). Cognitive aspects of frailty: mechanisms behind the link between frailty and cognitive impairment. J. Nutr. Health Aging 19, 276–283. doi: 10.1007/s12603-014-0535-z
Harada, C. N., Natelson Love, M. C., and Triebel, K. L. (2013). Normal cognitive aging. Clin. Geriatr. Med. 29, 737–752. doi: 10.1016/j.cger.2013.07.002
He, B., Chen, W., Zeng, J., Tong, W., and Zheng, P. A.-O. (2020). MicroRNA-326 decreases tau phosphorylation and neuron apoptosis through inhibition of the JNK signaling pathway by targeting VAV1 in Alzheimer’s disease. J. Cell. Physiol. 235, 480–493. doi: 10.1002/jcp.28988
He, J. R., Zhang, Y., Lu, W. J., Liang, H. B., Tu, X. Q., Ma, F. Y., et al. (2017). Age-related frontal periventricular white matter hyperintensities and miR-92a-3p are associated with early-onset post-stroke depression. Front. Aging Neurosci. 9:328. doi: 10.3389/fnagi.2017.00328
Hernandez-Rapp, J., Rainone, S., and Hebert, S. S. (2017). MicroRNAs underlying memory deficits in neurodegenerative disorders. Prog. Neuropsychopharmacol. Biol. Psychiatry 73, 79–86. doi: 10.1016/j.pnpbp.2016.04.011
Howdon, D., and Rice, N. (2018). Health care expenditures, age, proximity to death and morbidity: implications for an ageing population. J. Health Econ. 57, 60–74. doi: 10.1016/j.jhealeco.2017.11.001
Huang, Y., Wang, Y., Duan, Z., Liang, J., Xu, Y., Zhang, S., et al. (2021). Restored microRNA-326-5p inhibits neuronal apoptosis and attenuates mitochondrial damage via suppressing STAT3 in cerebral ischemia/reperfusion injury. Nanoscale Res. Lett. 16:63. doi: 10.1186/s11671-021-03520-3
Ipson, B. R., Fletcher, M. B., Espinoza, S. E., and Fisher, A. L. (2018). Identifying exosome-derived MicroRNAs as candidate biomarkers of frailty. J. Frailty Aging 7, 100–103. doi: 10.14283/jfa.2017.45
Joilin, G., Gray, E., Thompson, A. G., Bobeva, Y., Talbot, K., Weishaupt, J., et al. (2020). Identification of a potential non-coding RNA biomarker signature for amyotrophic lateral sclerosis. Brain Commun. 2:fcaa053. doi: 10.1093/braincomms/fcaa053
Kandlur, A., Satyamoorthy, K., and Gangadharan, G. (2020). Oxidative stress in cognitive and epigenetic aging: a retrospective glance. Front. Mol. Neurosci. 13:41. doi: 10.3389/fnmol.2020.00041
Kayano, M., Higaki, S., Satoh, J. I., Matsumoto, K., Matsubara, E., Takikawa, O., et al. (2016). Plasma microRNA biomarker detection for mild cognitive impairment using differential correlation analysis. Biomark. Res. 4:22. doi: 10.1186/s40364-016-0076-1
Kelaiditi, E., Cesari, M., Canevelli, M., van Kan, G. A., Ousset, P. J., Gillette-Guyonnet, S., et al. (2013). Cognitive frailty: rational and definition from an (I.A.N.A./I.A.G.G.) international consensus group. J. Nutr. Health Aging 17, 726–734. doi: 10.1007/s12603-013-0367-2
Kenny, A., McArdle, H., Calero, M., Rabano, A., Madden, S. F., Adamson, K., et al. (2019). Elevated plasma microRNA-206 levels predict cognitive decline and progression to dementia from mild cognitive impairment. Biomolecules 9:734. doi: 10.3390/biom9110734
Kiiti Borges, M., Oiring de Castro Cezar, N., Silva Santos Siqueira, A., Yassuda, M., Cesari, M., and Aprahamian, I. (2019). The relationship between physical frailty and mild cognitive impairment in the elderly: a systematic review. J. Frailty Aging 8, 192–197. doi: 10.14283/jfa.2019.29
Kim, B. S., Jung, J. Y., Jeon, J. Y., Kim, H. A., and Suh, C. H. (2016). Circulating hsa-miR-30e-5p, hsa-miR-92a-3p, and hsa-miR-223-3p may be novel biomarkers in systemic lupus erythematosus. HLA 88, 187–193. doi: 10.1111/tan.12874
Kim, M. Y., Shin, H., Moon, H. W., Park, Y. H., Park, J., and Lee, J. Y. (2021). Urinary exosomal microRNA profiling in intermediate-risk prostate cancer. Sci. Rep. 11:7355. doi: 10.1038/s41598-021-86785-z
Kinser, H. E., and Pincus, Z. (2020). MicroRNAs as modulators of longevity and the aging process. Hum. Genet. 139, 291–308. doi: 10.1007/s00439-019-02046-0
Kloosterman, W. P., and Plasterk, R. H. (2006). The diverse functions of microRNAs in animal development and disease. Dev. Cell 11, 441–450. doi: 10.1016/j.devcel.2006.09.009
Kondo, M., Yamada, H., Munetsuna, E., Yamazaki, M., Hatta, T., Iwahara, A., et al. (2019). Associations of serum microRNA-20a, –27a, and –103a with cognitive function in a Japanese population: the Yakumo study. Arch. Gerontol. Geriatr. 82, 155–160. doi: 10.1016/j.archger.2019.01.007
Konovalova, J., Gerasymchuk, D., Parkkinen, I., Chmielarz, P., and Domanskyi, A. (2019). Interplay between MicroRNAs and oxidative stress in neurodegenerative diseases. Int. J. Mol. Sci. 20:6055. doi: 10.3390/ijms20236055
Korolchuk, V. I., Miwa, S., Carroll, B., and von Zglinicki, T. (2017). Mitochondria in cell senescence: is mitophagy the weakest link? EBioMedicine 21, 7–13. doi: 10.1016/j.ebiom.2017.03.020
Kumar, S., Vijayan, M., Bhatti, J. S., and Reddy, P. H. (2017a). MicroRNAs as peripheral biomarkers in aging and age-related diseases. Prog. Mol. Biol. Transl. Sci. 146, 47–94. doi: 10.1016/bs.pmbts.2016.12.013
Kumar, S., Vijayan, M., and Reddy, P. H. (2017b). MicroRNA-455-3p as a potential peripheral biomarker for Alzheimer’s disease. Hum. Mol. Genet. 26, 3808–3822. doi: 10.1093/hmg/ddx267
Lai, W. F., Lin, M., and Wong, W. T. (2019). Tackling aging by using miRNA as a target and a tool. Trends Mol. Med. 25, 673–684. doi: 10.1016/j.molmed.2019.04.007
Lang, P. O., Mitchell, W. A., Lapenna, A., Pitts, D., and Aspinall, R. (2010). Immunological pathogenesis of main age-related diseases and frailty: role of immunosenescence. Eur. Geriatr. Med. 1, 112–121. doi: 10.1016/j.eurger.2010.01.010
Lazzeri, E., Peired, A., Ballerini, L., and Lasagni, L. (2012). “Adult stem cells in tissue homeostasis and disease,” in Current Frontiers and Perspectives in Cell Biology, ed. S. Najman (London: InTech), 379–404.
LeBrasseur, N. K., Tchkonia, T., and Kirkland, J. L. (2015). Cellular senescence and the biology of aging, disease, and frailty. Nestle Nutr. Inst. Workshop Ser. 83, 11–18. doi: 10.1159/000382054
Lee, Y. H., Kim, S. Y., and Bae, Y. S. (2014). Upregulation of miR-760 and miR-186 is associated with replicative senescence in human lung fibroblast cells. Mol. Cells 37, 620–627. doi: 10.14348/molcells.2014.0157
Lejri, I., Agapouda, A., Grimm, A., and Eckert, A. (2019). Mitochondria- and oxidative stress-targeting substances in cognitive decline-related disorders: from molecular mechanisms to clinical evidence. Oxid. Med. Cell Longev. 2019:9695412. doi: 10.1155/2019/9695412
Lekan, D. A., Collins, S. K., and Hayajneh, A. A. (2021). Definitions of frailty in qualitative research: a qualitative systematic review. J. Aging Res. 2021:6285058. doi: 10.1155/2021/6285058
Li, T., Luo, Z., Lin, S., Li, C., Dai, S., Wang, H., et al. (2020). MiR-185 targets POT1 to induce telomere dysfunction and cellular senescence. Aging 12, 14791–14807. doi: 10.18632/aging.103541
Li, W., Li, X., Xin, X., Kan, P.-C., and Yan, Y. (2016). MicroRNA-613 regulates the expression of brain-derived neurotrophic factor in Alzheimer’s disease. Biosci. Trends 10, 372–377. doi: 10.5582/bst.2016.01127
Liu, C.-G., Song, J., Zhang, Y.-Q., and Wang, P.-C. (2014a). MicroRNA-193b is a regulator of amyloid precursor protein in the blood and cerebrospinal fluid derived exosomal microRNA-193b is a biomarker of Alzheimer’s disease. Mol. Med. Rep. 10, 2395–2400. doi: 10.3892/mmr.2014.2484
Liu, C.-G., Wang, J.-L., Li, L., and Wang, P.-C. (2014b). MicroRNA-384 regulates both amyloid precursor protein and β-secretase expression and is a potential biomarker for Alzheimer’s disease. Int. J. Mol. Med. 34, 160–166. doi: 10.3892/ijmm.2014.1780
Liu, C.-G., Wang, J.-L., Li, L., Xue, L.-X., Zhang, Y.-Q., and Wang, P.-C. (2014c). MicroRNA-135a and –200b, potential Biomarkers for Alzheimer’s disease, regulate β secretase and amyloid precursor protein. Brain Res. 1583, 55–64. doi: 10.1016/j.brainres.2014.04.026
Loyer, X., Potteaux, S., Vion, A. C., Guerin, C. L., Boulkroun, S., Rautou, P. E., et al. (2014). Inhibition of microRNA-92a prevents endothelial dysfunction and atherosclerosis in mice. Circ. Res. 114, 434–443. doi: 10.1161/CIRCRESAHA.114.302213
Lupien, S. J., McEwen, B. S., Gunnar, M. R., and Heim, C. (2009). Effects of stress throughout the lifespan on the brain, behaviour and cognition. Nat. Rev. Neurosci. 10, 434–445. doi: 10.1038/nrn2639
Ma, J., Shang, S., Wang, J., Zhang, T., Nie, F., Song, X., et al. (2018). Identification of miR-22-3p, miR-92a-3p, and miR-137 in peripheral blood as biomarker for schizophrenia. Psychiatry Res. 265, 70–76. doi: 10.1016/j.psychres.2018.03.080
Ma, L., and Chan, P. (2020). Understanding the physiological links between physical frailty and cognitive decline. Aging Dis. 11, 405–418. doi: 10.14336/AD.2019.0521
Mao, G., Zhang, Z., Hu, S., Zhang, Z., Chang, Z., Huang, Z., et al. (2018). Exosomes derived from miR-92a-3p-overexpressing human mesenchymal stem cells enhance chondrogenesis and suppress cartilage degradation via targeting WNT5A. Stem Cell Res. Ther. 9:247. doi: 10.1186/s13287-018-1004-0
Martin, F. C., and O’Halloran, A. M. (2020). Tools for assessing frailty in older people: general concepts. Adv. Exp. Med. Biol. 1216, 9–19. doi: 10.1007/978-3-030-33330-0_2
Martin, F. C., and Ranhoff, A. H. (2020). “Frailty and sarcopenia,” in Orthogeriatrics: The Management of Older Patients with Fragility Fractures, eds P. Falaschi and D. Marsh (Cham: Springer), 53–65.
Mattson, M. P., and Arumugam, T. V. (2018). Hallmarks of brain aging: adaptive and pathological modification by metabolic states. Cell Metab. 27, 1176–1199. doi: 10.1016/j.cmet.2018.05.011
Merchant, R. A., Chan, Y. H., Hui, R. J. Y., Tsoi, C. T., Kwek, S. C., Tan, W. M., et al. (2021). Motoric cognitive risk syndrome, physio-cognitive decline syndrome, cognitive frailty and reversibility with dual-task exercise. Exp. Gerontol. 150:111362. doi: 10.1016/j.exger.2021.111362
Mitnitski, A. B., Mogilner, A. J., and Rockwood, K. (2001). Accumulation of deficits as a proxy measure of aging. ScientificWorldJournal 1, 323–336. doi: 10.1100/tsw.2001.58
Miyamura, K., Fhon, J. R. S., Bueno, A. A., Fuentes-Neira, W. L., Silveira, R., and Rodrigues, R. A. P. (2019). Frailty syndrome and cognitive impairment in older adults: systematic review of the literature. Rev. Lat. Am. Enfermagem. 27:e3202. doi: 10.1590/1518-8345.3189.3202
Mogilyansky, E., and Rigoutsos, I. (2013). The miR-17/92 cluster: a comprehensive update on its genomics, genetics, functions and increasingly important and numerous roles in health and disease. Cell Death Differ. 20, 1603–1614. doi: 10.1038/cdd.2013.125
Mohr, A. M., and Mott, J. L. (2015). Overview of microRNA biology. Semin. Liver Dis. 35, 3–11. doi: 10.1055/s-0034-1397344
Morley, J. E. (2018). An overview of cognitive impairment. Clin. Geriatr. Med. 34, 505–513. doi: 10.1016/j.cger.2018.06.003
Morley, J. E., and Malmstrom, T. K. (2013). Frailty, sarcopenia, and hormones. Endocrinol. Metab. Clin. N. Am. 42, 391–405. doi: 10.1016/j.ecl.2013.02.006
Morton, S. U., Sefton, C. R., Zhang, H., Dai, M., Turner, D. L., Uhler, M. D., et al. (2021). microRNA-mRNA profile of skeletal muscle differentiation and relevance to congenital myotonic dystrophy. Int. J. Mol. Sci. 22:2692. doi: 10.3390/ijms22052692
Mu, J., Cheng, X., Zhong, S., Chen, X., and Zhao, C. (2020). Neuroprotective effects of miR-532-5p against ischemic stroke. Metab. Brain Dis. 35, 753–763. doi: 10.1007/s11011-020-00544-z
Mulero, J., Zafrilla, P., and Martinez-Cacha, A. (2011). Oxidative stress, frailty and cognitive decline. J. Nutr. Health Aging 15, 756–760. doi: 10.1007/s12603-011-0130-5
Nagaraj, S., Laskowska-Kaszub, K., Dȩbski, K. J., Wojsiat, J., Da̧browski, M., Gabryelewicz, T., et al. (2017). Profile of 6 microRNA in blood plasma distinguish early stage Alzheimer’s disease patients from non-demented subjects. Oncotarget 8, 16122–16143. doi: 10.18632/oncotarget.15109
Nampoothiri, S. S., and Rajanikant, G. K. (2017). Decoding the ubiquitous role of microRNAs in neurogenesis. Mol. Neurobiol. 54, 2003–2011. doi: 10.1007/s12035-016-9797-2
Nascimento, C. M., Ingles, M., Salvador-Pascual, A., Cominetti, M. R., Gomez-Cabrera, M. C., and Viña, J. (2018). Sarcopenia, frailty and their prevention by exercise. Free Radic. Biol. Med. 132, 42–49. doi: 10.1016/j.freeradbiomed.2018.08.035
Nasreddine, Z. S., Phillips, N. A., Bedirian, V., Charbonneau, S., Whitehead, V., Collin, I., et al. (2005). The montreal cognitive assessment, MoCA: a brief screening tool for mild cognitive impairment. J. Am. Geriatr. Soc. 53, 695–699. doi: 10.1111/j.1532-5415.2005.53221.x
Noren Hooten, N., Fitzpatrick, M., Wood, W. H. III, De, S., Ejiogu, N., Zhang, Y., et al. (2013). Age-related changes in microRNA levels in serum. Aging 5, 725–740. doi: 10.18632/aging.100603
O’Brien, J., Hayder, H., Zayed, Y., and Peng, C. (2018). Overview of MicroRNA biogenesis, mechanisms of actions, and circulation. Front. Endocrinol. 9:402. doi: 10.3389/fendo.2018.00402
O’Caoimh, R., Sezgin, D., O’Donovan, M. R., Molloy, D. W., Clegg, A., Rockwood, K., et al. (2021). Prevalence of frailty in 62 countries across the world: a systematic review and meta-analysis of population-level studies. Age Aging 50, 96–104. doi: 10.1093/aging/afaa219
Olivieri, F., Capri, M., Bonafe, M., Morsiani, C., Jung, H. J., Spazzafumo, L., et al. (2017). Circulating miRNAs and miRNA shuttles as biomarkers: perspective trajectories of healthy and unhealthy aging. Mech. Aging Dev. 165, 162–170. doi: 10.1016/j.mad.2016.12.004
Olivieri, F., Prattichizzo, F., Giuliani, A., Matacchione, G., Rippo, M. R., Sabbatinelli, J., et al. (2021). miR-21 and miR-146a: the microRNAs of inflammaging and age-related diseases. Aging Res. Rev. 70:101374. doi: 10.1016/j.arr.2021.101374
Olivieri, F., Rippo, M. R., Procopio, A. D., and Fazioli, F. (2013). Circulating inflamma-miRs in aging and age-related diseases. Front. Genet. 4:121. doi: 10.3389/fgene.2013.00121
Olivieri, F., Spazzafumo, L., Santini, G., Lazzarini, R., Albertini, M. C., Rippo, M. R., et al. (2012). Age-related differences in the expression of circulating microRNAs: miR-21 as a new circulating marker of inflammaging. Mech. Aging Dev. 133, 675–685. doi: 10.1016/j.mad.2012.09.004
Pawelec, G. (2018). Age and immunity: what is “immunosenescence”? Exp. Gerontol. 105, 4–9. doi: 10.1016/j.exger.2017
Perry, V. H. (2004). The influence of systemic inflammation on inflammation in the brain: implications for chronic neurodegenerative disease. Brain Behav. Immun. 18, 407–413. doi: 10.1016/j.bbi.2004.01.004
Pilotto, A., Ferrucci, L., Franceschi, M., D’Ambrosio, L. P., Scarcelli, C., Cascavilla, L., et al. (2008). Development and validation of a multidimensional prognostic index for one-year mortality from comprehensive geriatric assessment in hospitalized older patients. Rejuvenation Res. 11, 151–161. doi: 10.1089/rej.2007.0569
Pilotto, A., Polidori, M. C., Veronese, N., Panza, F., Arboretti Giancristofaro, R., Pilotto, A., et al. (2018). Association of antidementia drugs and mortality in community-dwelling frail older patients with dementia: the role of mortality risk assessment. J. Am. Med. Dir. Assoc. 19, 162–168. doi: 10.1016/j.jamda.2017.08.017
Pilotto, A., Veronese, N., Siri, G., Bandinelli, S., Tanaka, T., Cella, A., et al. (2020). Association between the multidimensional prognostic index and mortality over 15 years of follow-up in the inchianti study. J. Gerontol. A Biol. Sci. Med. Sci. 76, 1678–1685. doi: 10.1093/gerona/glaa237
Piscopo, P., Lacorte, E., Feligioni, M., Mayer, F., Crestini, A., Piccolo, L., et al. (2019). MicroRNAs and mild cognitive impairment: a systematic review. Aging Res. Rev. 50, 131–141. doi: 10.1016/j.arr.2018.11.005
Proietti, M., and Cesari, M. (2020). Frailty: what is it? Adv. Exp. Med. Biol. 1216, 1–7. doi: 10.1007/978-3-030-33330-0_1
Qin, H., Hu, C., Zhao, X., Tian, M., and Zhu, B. (2021). Usefulness of candidate mRNAs and miRNAs as biomarkers for mild cognitive impairment and Alzheimer’s disease. Int. J. Neurosci. [Epub ahead of print]. doi: 10.1080/00207454.2021.1886098
Quinn, S. R., and O’Neill, L. A. (2011). A trio of microRNAs that control Toll-like receptor signalling. Int. Immunol. 23, 421–425. doi: 10.1093/intimm/dxr034
Raefsky, S. M., and Mattson, M. P. (2017). Adaptive responses of neuronal mitochondria to bioenergetic challenges: roles in neuroplasticity and disease resistance. Free Radic. Biol. Med. 102, 203–216. doi: 10.1016/j.freeradbiomed.2016.11.045
Rajman, M., and Schratt, G. (2017). MicroRNAs in neural development: from master regulators to fine-tuners. Development 144, 2310–2322. doi: 10.1242/dev.144337
Reis, P. P., Drigo, S. A., Carvalho, R. F., Lopez Lapa, R. M., Felix, T. F., Patel, D., et al. (2020). Circulating miR-16-5p, miR-92a-3p, and miR-451a in plasma from lung cancer patients: potential application in early detection and a regulatory role in tumorigenesis pathways. Cancers 12:2071. doi: 10.3390/cancers12082071
Robertson, D. A., Savva, G. M., and Kenny, R. A. (2013). Frailty and cognitive impairment–a review of the evidence and causal mechanisms. Aging Res. Rev. 12, 840–851. doi: 10.1016/j.arr.2013.06.004
Rohrmann, S. (2020). Epidemiology of frailty in older people. Adv. Exp. Med. Biol. 1216, 21–27. doi: 10.1007/978-3-030-33330-0_3
Ruan, Q., Yu, Z., Chen, M., Bao, Z., Li, J., and He, W. (2015). Cognitive frailty, a novel target for the prevention of elderly dependency. Aging Res. Rev. 20, 1–10. doi: 10.1016/j.arr.2014.12.004
Rusanova, I., Diaz-Casado, M. E., Fernandez-Ortiz, M., Aranda-Martinez, P., Guerra-Librero, A., Garcia-Garcia, F. J., et al. (2018). Analysis of plasma MicroRNAs as predictors and biomarkers of aging and frailty in humans. Oxid. Med. Cell Longev. 2018:7671850. doi: 10.1155/2018/7671850
Sachdev, P. S., Lipnicki, D. M., Crawford, J., Reppermund, S., Kochan, N. A., Trollor, J. N., et al. (2013). Factors predicting reversion from mild cognitive impairment to normal cognitive functioning: a population-based study. PLoS One 8:e59649. doi: 10.1371/journal.pone.0059649
Sanford, A. M. (2017). Mild cognitive impairment. Clin. Geriatr. Med. 33, 325–337. doi: 10.1016/j.cger.2017.02.005
Sannicandro, A. J., Soriano-Arroquia, A., and Goljanek-Whysall, K. (2019). Micro(RNA)-managing muscle wasting. J. Appl. Physiol. 127, 619–632. doi: 10.1152/japplphysiol.00961.2018
Sargent, L., Nalls, M., Starkweather, A., Hobgood, S., Thompson, H., Amella, E. J., et al. (2018). Shared biological pathways for frailty and cognitive impairment: a systematic review. Aging Res. Rev. 47, 149–158. doi: 10.1016/j.arr.2018.08.001
Sarkar, S. N., Russell, A. E., Engler-Chiurazzi, E. B., Porter, K. N., and Simpkins, J. W. (2019). MicroRNAs and the genetic nexus of brain aging, neuroinflammation, neurodegeneration, and brain trauma. Aging Dis. 10, 329–352. doi: 10.14336/AD.2018.0409
Schafer, M. J., Zhang, X., Kumar, A., Atkinson, E. J., Zhu, Y., Jachim, S., et al. (2020). The senescence-associated secretome as an indicator of age and medical risk. JCI Insight 5:e133668. doi: 10.1172/jci.insight.133668
Schirinzi, T., Canevelli, M., Suppa, A., Bologna, M., and Marsili, L. (2020). The continuum between neurodegeneration, brain plasticity, and movement: a critical appraisal. Rev. Neurosci. 31, 723–742. doi: 10.1515/revneuro-2020-0011
Searle, S. D., and Rockwood, K. (2015). Frailty and the risk of cognitive impairment. Alzheimers Res. Ther. 7:54. doi: 10.1186/s13195-015-0140-3
Serna, E., Gambini, J., Borras, C., Abdelaziz, K. M., Belenguer, A., Sanchis, P., et al. (2012). Centenarians, but not octogenarians, up-regulate the expression of microRNAs. Sci. Rep. 2:961. doi: 10.1038/srep00961
Shabab, T., Khanabdali, R., Moghadamtousi, S. Z., Kadir, H. A., and Mohan, G. (2017). Neuroinflammation pathways: a general review. Int. J. Neurosci. 127, 624–633. doi: 10.1080/00207454.2016.1212854
Sheinerman, K. S., Tsivinsky, V. G., Abdullah, L., Crawford, F., and Umansky, S. R. (2013). Plasma microRNA biomarkers for detection of mild cognitive impairment: biomarker validation study. Aging 5, 925–938. doi: 10.18632/aging.100624
Sheinerman, K. S., Tsivinsky, V. G., Crawford, F., Mullan, M. J., Abdullah, L., and Umansky, S. R. (2012). Plasma microRNA biomarkers for detection of mild cognitive impairment. Aging 4, 590–605. doi: 10.18632/aging.100486
Shi, Y., Mao, X., Cai, M., Hu, S., Lai, X., Chen, S., et al. (2021a). miR-194-5p negatively regulates the proliferation and differentiation of rabbit skeletal muscle satellite cells. Mol. Cell. Biochem. 476, 425–433. doi: 10.1007/s11010-020-03918-0
Shi, Y., Yi, Z., Zhao, P., Xu, Y., and Pan, P. (2021b). MicroRNA-532-5p protects against cerebral ischemia-reperfusion injury by directly targeting CXCL1. Aging 13, 11528–11541. doi: 10.18632/aging.202846
Siedlecki-Wullich, D., Catala-Solsona, J., Fabregas, C., Hernandez, I., Clarimon, J., Lleo, A., et al. (2019). Altered microRNAs related to synaptic function as potential plasma biomarkers for Alzheimer’s disease. Alzheimers Res. Ther. 11:46. doi: 10.1186/s13195-019-0501-4
Siedlecki-Wullich, D. A.-O., Miñano-Molina, A. A.-O., and Rodríguez-Álvarez, J. (2021). microRNAs as early biomarkers of alzheimer’s disease: a synaptic perspective. Cells 10:113. doi: 10.3390/cells10010113
Skaper, S. D., Facci, L., Zusso, M., and Giusti, P. (2017). Synaptic plasticity, dementia and alzheimer disease. CNS Neurol. Disord. Drug Targets 16, 220–233. doi: 10.2174/1871527316666170113120853
Slota, J. A., and Booth, S. A. (2019). MicroRNAs in neuroinflammation: implications in disease pathogenesis, biomarker discovery and therapeutic applications. Noncoding RNA 5:35. doi: 10.3390/ncrna5020035
Smith-Vikos, T., Liu, Z., Parsons, C., Gorospe, M., Ferrucci, L., Gill, T. M., et al. (2016). A serum miRNA profile of human longevity: findings from the Baltimore Longitudinal Study of Aging (BLSA). Aging 8, 2971–2987. doi: 10.18632/aging.101106
Sohel, M. H. (2016). Extracellular/Circulating MicroRNAs: release mechanisms, functions and challenges. Achiev. Life Sci. 10, 175–186. doi: 10.1016/j.als.2016.11.007
Soysal, P., Stubbs, B., Lucato, P., Luchini, C., Solmi, M., Peluso, R., et al. (2016). Inflammation and frailty in the elderly: a systematic review and meta-analysis. Aging Res. Rev. 31, 1–8. doi: 10.1016/j.arr.2016.08.006
Stephan, B. C., Hunter, S., Harris, D., Llewellyn, D. J., Siervo, M., Matthews, F. E., et al. (2012). The neuropathological profile of mild cognitive impairment (MCI): a systematic review. Mol. Psychiatry 17, 1056–1076. doi: 10.1038/mp.2011.147
Stokin, G. B., Krell-Roesch, J., Petersen, R. C., and Geda, Y. E. (2015). Mild neurocognitive disorder: an old wine in a new bottle. Harv. Rev. Psychiatry 23, 368–376. doi: 10.1097/HRP.0000000000000084
Suh, N. (2018). MicroRNA controls of cellular senescence. BMB Rep. 51, 493–499. doi: 10.5483/BMBRep.2018.51
Tahamtan, A., Teymoori-Rad, M., Nakstad, B., and Salimi, V. (2018). Anti-inflammatory MicroRNAs and their potential for inflammatory diseases treatment. Front. Immunol. 9:1377. doi: 10.3389/fimmu.2018.01377
Tangestani Fard, M., and Stough, C. (2019). A review and hypothesized model of the mechanisms that underpin the relationship between inflammation and cognition in the elderly. Front. Aging Neurosci. 11:56. doi: 10.3389/fnagi.2019.00056
Thounaojam, M. C., Kaushik, D. K., and Basu, A. (2013). MicroRNAs in the brain: it’s regulatory role in neuroinflammation. Mol. Neurobiol. 47, 1034–1044. doi: 10.1007/s12035-013-8400-3
Toricelli, M., Pereira, A. A. R., Souza Abrao, G., Malerba, H. N., Maia, J., Buck, H. S., et al. (2021). Mechanisms of neuroplasticity and brain degeneration: strategies for protection during the aging process. Neural Regen. Res. 16, 58–67. doi: 10.4103/1673-5374.286952
Vatic, M., von Haehling, S., and Ebner, N. (2020). Inflammatory biomarkers of frailty. Exp. Gerontol. 133:110858. doi: 10.1016/j.exger.2020.110858
Verghese, J., Annweiler, C., Ayers, E., Barzilai, N., Beauchet, O., Bennett, D. A., et al. (2014). Motoric cognitive risk syndrome: multicountry prevalence and dementia risk. Neurology 83, 718–726. doi: 10.1212/WNL.0000000000000717
Wang, L., Cui, M., Qu, F., Cheng, D., Yu, J., Tang, Z., et al. (2021). MiR-92a-3p promotes the malignant progression of hepatocellular carcinoma by mediating the PI3K/AKT/mTOR signaling pathway. Curr. Pharm. Des. 27, 3244–3250. doi: 10.2174/1381612827666210612054156
Wang, T., Chen, K., Li, H., Dong, S., Su, N., Liu, Y., et al. (2015). The feasibility of utilizing plasma MiRNA107 and BACE1 messenger RNA gene expression for clinical diagnosis of amnestic mild cognitive impairment. J. Clin. Psychiatry 76, 135–141. doi: 10.4088/JCP.13m08812
Welstead, M., Jenkins, N. D., Russ, T., Luciano, M., and Muniz-Terrera, G. (2020). A systematic review of frailty trajectories: their shape and influencing factors. Gerontologist [Epub ahead of print]. doi: 10.1093/geront/gnaa061
Williams, J., Smith, F., Kumar, S., Vijayan, M., and Reddy, P. H. (2017). Are microRNAs true sensors of aging and cellular senescence? Aging Res. Rev. 35, 350–363. doi: 10.1016/j.arr.2016.11.008
Wilson, D., Jackson, T., Sapey, E., and Lord, J. M. (2017). Frailty and sarcopenia: the potential role of an aged immune system. Aging Res. Rev. 36, 1–10. doi: 10.1016/j.arr.2017.01.006
Xia, X., Wang, Y., and Zheng, J. C. (2020). The microRNA-17 ~ 92 family as a key regulator of neurogenesis and potential regenerative therapeutics of neurological disorders. Stem Cell Rev. Rep. [Epub ahead of print]. doi: 10.1007/s12015-020-10050-5
Xie, B., Zhou, H., Zhang, R., Song, M., Yu, L., Wang, L., et al. (2015). Serum miR-206 and miR-132 as potential circulating biomarkers for mild cognitive impairment. J. Alzheimers Dis. 45, 721–731. doi: 10.3233/JAD-142847
Xu, S., Zhang, B., Zhu, Y., Huang, H., Yang, W., Huang, H., et al. (2017). miR-194 functions as a novel modulator of cellular senescence in mouse embryonic fibroblasts. Cell Biol. Int. 41, 249–257. doi: 10.1002/cbin.10715
Xu, W., Wong, G., Hwang, Y. Y., and Larbi, A. (2020). The untwining of immunosenescence and aging. Semin. Immunopathol. 42, 559–572. doi: 10.1007/s00281-020-00824-x
Yan, X., Zeng, D., Zhu, H., Zhang, Y., Shi, Y., Wu, Y., et al. (2020). MiRNA-532-5p regulates CUMS-induced depression-like behaviors and modulates LPS-induced proinflammatory cytokine signaling by targeting STAT3. Neuropsychiatr. Dis. Treat. 16, 2753–2764. doi: 10.2147/NDT.S251152
Yang, T. T., Liu, C. G., Gao, S. C., Zhang, Y., and Wang, P. C. (2018). The serum exosome derived MicroRNA-135a, –193b, and –384 were potential Alzheimer’s disease biomarkers. Biomed. Environ. Sci. 31, 87–96. doi: 10.3967/bes2018.011
Yankner, B. A., Lu, T., and Loerch, P. (2008). The aging brain. Annu. Rev. Pathol. 3, 41–66. doi: 10.1146/annurev.pathmechdis.2.010506.092044
Yiallouris, A., Tsioutis, C., Agapidaki, E., Zafeiri, M., Agouridis, A. P., Ntourakis, D., et al. (2019). Adrenal aging and its implications on stress responsiveness in humans. Front. Endocrinol. 10:54. doi: 10.3389/fendo.2019.00054
Yin, J., Qian, Z., Chen, Y., Li, Y., and Zhou, X. (2020). MicroRNA regulatory networks in the pathogenesis of sarcopenia. J. Cell. Mol. Med. 24, 4900–4912. doi: 10.1111/jcmm.15197
Yu, B., Zhou, S., Liang, H., Ye, Q., and Wang, Y. (2021). Development and validation of a novel circulating miRNA-based diagnostic score for early detection of hepatocellular carcinoma. Dig. Dis. Sci. [Epub ahead of print]. doi: 10.1007/s10620-021-07031-0
Zampino, M., Ferrucci, L., and Semba, R. D. (2020). Biomarkers in the path from cellular senescence to frailty. Exp. Gerontol. 129:110750. doi: 10.1016/j.exger.2019.110750
Zhang, Q., Wu, X., and Yang, J. (2021). miR-194-5p protects against myocardial ischemia/reperfusion injury via MAPK1/PTEN/AKT pathway. Ann. Transl. Med. 9:654. doi: 10.21037/atm-21-807
Zhang, Y., Ueno, Y., Liu, X. S., Buller, B., Wang, X., Chopp, M., et al. (2013). The MicroRNA-17-92 cluster enhances axonal outgrowth in embryonic cortical neurons. J. Neurosci. 33, 6885–6894. doi: 10.1523/JNEUROSCI.5180-12.2013
Keywords: frailty, cognitive frailty, biomarkers, miRNA–microRNA, cognitive impairment, MCI (mild cognitive impairment)
Citation: Carini G, Musazzi L, Bolzetta F, Cester A, Fiorentini C, Ieraci A, Maggi S, Popoli M, Veronese N and Barbon A (2021) The Potential Role of miRNAs in Cognitive Frailty. Front. Aging Neurosci. 13:763110. doi: 10.3389/fnagi.2021.763110
Received: 23 August 2021; Accepted: 19 October 2021;
Published: 12 November 2021.
Edited by:
David Facal, University of Santiago de Compostela, SpainReviewed by:
Zuyun Liu, Zhejiang University, ChinaQingwei Ruan, Fudan University, China
Elzbieta Bobrowicz-Campos, University of Coimbra, Portugal
Copyright © 2021 Carini, Musazzi, Bolzetta, Cester, Fiorentini, Ieraci, Maggi, Popoli, Veronese and Barbon. This is an open-access article distributed under the terms of the Creative Commons Attribution License (CC BY). The use, distribution or reproduction in other forums is permitted, provided the original author(s) and the copyright owner(s) are credited and that the original publication in this journal is cited, in accordance with accepted academic practice. No use, distribution or reproduction is permitted which does not comply with these terms.
*Correspondence: Alessandro BarbonYWxlc3NhbmRyby5iYXJib25AdW5pYnMuaXQ=