Effects of Sex, Age, and Apolipoprotein E Genotype on Brain Ceramides and Sphingosine-1-Phosphate in Alzheimer’s Disease and Control Mice
- 1Department of Internal Medicine, Erasmus University Medical Center, Rotterdam, Netherlands
- 2Department of Psychiatry and Neuropsychology, School for Mental Health and Neuroscience, Maastricht University, Maastricht, Netherlands
- 3Department of Molecular Cell Biology and Immunology, Amsterdam Neuroscience, VU Medical Center, Amsterdam UMC, Amsterdam, Netherlands
- 4Department of Neurology, University Hospital Bonn, Venusberg Campus, Bonn, Germany
- 5Department of Clinical Chemistry, Erasmus University Medical Center, Rotterdam, Netherlands
- 6Leiden Institute of Chemistry, Leiden University, Leiden, Netherlands
Apolipoprotein ε4 (APOE)4 is a strong risk factor for the development of Alzheimer’s disease (AD) and aberrant sphingolipid levels have been implicated in AD. We tested the hypothesis that the APOE4 genotype affects brain sphingolipid levels in AD. Seven ceramides and sphingosine-1-phosphate (S1P) were quantified by LC-MSMS in hippocampus, cortex, cerebellum, and plasma of <3 months and >5 months old human APOE3 and APOE4-targeted replacement mice with or without the familial AD (FAD) background of both sexes (145 animals). APOE4 mice had higher Cer(d18:1/24:0) levels in the cortex (1.7-fold, p = 0.002) than APOE3 mice. Mice with AD background showed higher levels of Cer(d18:1/24:1) in the cortex than mice without (1.4-fold, p = 0.003). S1P levels were higher in all three brain regions of older mice than of young mice (1.7-1.8-fold, all p ≤ 0.001). In female mice, S1P levels in hippocampus (r = −0.54 [−0.70, −0.35], p < 0.001) and in cortex correlated with those in plasma (r = −0.53 [−0.71, −0.32], p < 0.001). Ceramide levels were lower in the hippocampus (3.7–10.7-fold, all p < 0.001), but higher in the cortex (2.3–12.8-fold, p < 0.001) of female than male mice. In cerebellum and plasma, sex effects on individual ceramides depended on acyl chain length (9.5-fold lower to 11.5-fold higher, p ≤ 0.001). In conclusion, sex is a stronger determinant of brain ceramide levels in mice than APOE genotype, AD background, or age. Whether these differences impact AD neuropathology in men and women remains to be investigated.
Introduction
Alzheimer’s disease (AD) is the most common cause of late-onset dementia with a prevalence of approximately 50 million cases worldwide (Prince et al., 2013). It is a progressive neurodegenerative disorder characterized by a gradual loss of memory and other cognitive functions. Less than 3% of AD is early onset, caused by mutations including those in the APP, PS1, or PS2 genes (Kang et al., 1987; Levy-Lahad et al., 1995; Sherrington et al., 1995). There is no single cause for late-onset, sporadic AD, but important risk factors are age and being female (Liesinger et al., 2018). A major genetic risk factor for sporadic AD is the ε4 allele of the APOE gene encoding for apolipoprotein (Apo)E4 in comparison to the other APOE isoforms, ε2 and ε3 (Corder et al., 1993; Strittmatter et al., 1993; Zhang and Hong, 2015). Compared to the general population, individuals heterozygous for APOE4 have a ∼3-fold higher risk of developing AD, and homozygous APOE4 individuals have a ∼15-fold increased risk (Chartier-Harlin et al., 1994; Farrer et al., 1997; Kloske and Wilcock, 2020). How ApoE4 affects AD development remains to be clarified. Besides genome wide associations studies have identified APOE as a longevity gene, with APOE4 being associated with lower odds for a long live (Partridge Nature 2018, 561, 45–56 and Deelen Nature comm 2019, 10:3669).
ApoE is best known for its role in peripheral lipid trafficking, and there is evidence supporting a similar role for ApoE in the brain (Holtzman et al., 2012). Glial cells, which are the predominant source of brain ApoE, secrete it associated with lipids as high density lipoprotein-like particles (Abildayeva et al., 2006; Mahley, 2016). Brain lipid homeostasis is strictly regulated (Bjorkhem and Meaney, 2004; Strazielle and Ghersi-Egea, 2013). Imbalances in brain (sphingo)lipid homeostasis are associated with intellectual disability and with neurodegenerative disease (Liu et al., 1998; Haughey et al., 2010; Crivelli et al., 2020a) and possibly also with AD. In mice, deletion of Apoe or replacement with human APOE4 leads to a dysfunctional cerebrovascular unit (Mulder et al., 2001; Bell et al., 2012), which may affect trafficking of lipids, including sphingolipids, across the blood–brain barrier. Such a disturbance in brain lipid homeostasis by APOE4 may accelerate the pathogenesis of AD. Presently, the knowledge on trafficking of sphingolipids across the blood–brain barrier is limited (Zimmermann et al., 2001).
Alterations in brain and plasma sphingolipid homeostasis have been observed in patients with cognitive impairment and with AD (Mielke et al., 2010a,b, 2014, 2017; Martinez Martinez and Mielke, 2017; Crivelli et al., 2020a). Sphingolipids consist of a sphingosine backbone and can have various head groups and an acyl chain that differs in length. Besides their role as plasma membrane components (Olsen and Faergeman, 2017), sphingolipids are involved in neuronal plasticity (Wheeler et al., 2009), neurogenesis (Schwarz and Futerman, 1997; Olsen and Faergeman, 2017), and inflammation (Hannun, 1994; van Echten-Deckert et al., 2014). The sphingolipid acyl chain length is an important determinant of their function. Sphingolipids with long chains (C16:0) increase apoptosis, while very-long chains (C22:0-C24:0/C24:1) offer partial protection from apoptosis (Park and Park, 2015). In addition, the ratio between saturated (i.e., C24:0) and unsaturated (i.e., C24:1) acyl chains affects plasma membrane properties, thereby affecting signal transduction, membrane fusion, and cellular integrity (Pinto et al., 2008; Lazzarini et al., 2015).
Ceramides are the central hub of sphingolipid metabolism and are derived via de novo synthesis or from the degradation of more complex sphingolipids. Low ceramide levels promote neuronal cell growth, development, survival and division (Schwarz and Futerman, 1997; Brann et al., 1999; Mullen et al., 2012), while high levels may cause (neuronal) cell death (Jana et al., 2009; Mullen and Obeid, 2012; Czubowicz and Strosznajder, 2014). Sphingosine-1-phosphate (S1P) is formed in a reversible process from ceramide. S1P is an important signaling molecule that regulates cell survival, differentiation and immunity (van Echten-Deckert et al., 2014). The balance between ceramides and S1P is considered a major determinant of cell survival and death (Van Brocklyn and Williams, 2012).
The effect of APOE4 on cholesterol and phospholipid homeostasis in the brain has been reported, but little attention has been paid, so far, to its relation with sphingolipid homeostasis. In patients with late-onset AD, APOE4 was associated with higher ceramide levels in brain, but this was not observed in healthy controls (Bandaru et al., 2009; Couttas et al., 2018). Minor differences in total brain ceramide levels were found between APOE4, APOE3, and APOE2 knock-in mice (Sharman et al., 2010; den Hoedt et al., 2016). Therefore, we aimed at investigating the modulatory effect of APOE genotype on brain sphingolipid homeostasis, in the context of the development of AD pathology. To this end, we assessed brain and plasma ceramide and S1P profiles in APOE4 and APOE3 transgenic mice with or without five familial AD mutations (E4FAD or E3FAD; K670N/M671L, I716V, and V717I in the APP gene and M146L and L286V in the PS1 gene) (Tai et al., 2011; Youmans et al., 2012a,b). The mice with the FAD mutations develop an AD phenotype, including Aβ accumulation, neuroinflammation, and cognitive impairment, from as early as 4 months of age (Youmans et al., 2012b; Tai et al., 2017). Therefore, sphingolipids were analyzed in different brain regions of mice younger than 3 months and older than 5 months. As sex potentially modulates AD incidence (Jorm and Jolley, 1998; Fratiglioni et al., 2000; Liesinger et al., 2018) and pathology (Maynard et al., 2006; Schafer et al., 2007), both female and male mice were included in the analyses.
Materials and Methods
Animals
This study was not pre-registered. Transgenic APOE3-targeted replacement (TR), E3FAD-TR (APOE3-TR mice with 5xFAD mutations), APOE4-TR, and E4FAD-TR (APOE4-TR mice with 5xFAD mutations) mice were purchased from Dr. Mary Jo LaDu (University of Illinois at Chicago) and have been fully characterized by Oakley et al. (2006) and Youmans et al. (2012a,b). Colonies were maintained at Maastricht University. Young [<3 months, (2.1–2.6 months old)] and older [>5 months (5.4–14.3 months old)] male and female mice of all four genotypes were included in this study. An alternative analysis excluding 3 animals 5 months and 3 animals 14 months to narrow age range to 7–12 was carried out and the data is reported in Supplementary Information 1. Exclusion of these 6 animals did not affect the results qualitatively. All female mice were breeders. Animals were housed socially on a reverse 12-h day-night cycle under standardized environmental conditions (ambient temperature 20 ± 1°C; humidity 40–60%, background noise, cage enrichment) at the central animal facility of Maastricht University and had ad libitum access to food and water. All experiments were approved by the Animal Welfare Committee of Maastricht University and were performed according to Dutch federal regulations for animal protection (DEC 2015-002).
No sample size calculation was performed prior to the experiments, but based on previous studies on sphingolipids in mice we aimed at 10 mice per group (Barrier et al., 2010; den Hoedt et al., 2016). The in total 145 mice were divided in groups consisting of 7–10 animals for brain analysis and of 4–10 animals for plasma analysis (Table 1). Animals were sacrificed by CO2 inhalation in the morning (09:00 – 12:00 h) and subsequent decapitation. Blood was collected in a Microvette® CB 300 LH tube (order no. 16.443, Sarstedt group, Etten-Leur, Netherlands) and subsequently centrifuged (2,000 g, 4°C, 10 min) to isolate plasma, which was stored at −80°C until analysis. From all animals the brain was removed, cut through the midline sagittal section, snap frozen in liquid nitrogen, and stored at −80°C until analysis. Before sphingolipid analysis, brain hemispheres were dissected into cortex, hippocampus, and cerebellum on ice and samples were powdered on dry ice and stored at −80°C until analysis.
Sphingolipid Analysis
Group allocation of experimental animals was unknown to the experimenter prior to sphingolipid analysis.
Lipid Extraction
Sphingolipids were extracted as described (de Wit et al., 2016, 2019). In short, frozen tissue samples were weighed and homogenized in cold Millipore water (MQ, 18.2 MΩ cm filter) from a Milli-Q® PF Plus system (Merck Millipore B.V., Amsterdam, Netherlands). To 10 μL tissue homogenates and plasma samples, the internal standards Cer(d18:1/17:0), Cer(d17:0/24:1), and S1P(d18:1)-D7 were added (10 μL of 2, 2 and 0.2 μg/mL in methanol, respectively; IS: Avanti Polar Lipids, Alabaster, AL, United States; methanol: Merck Millipore B.V.). After addition of 10 μL of 10% TEA solution [triethylamine (10/90, v/v) in methanol/dichloromethane (DCM) (50/50, v/v); TEA: Merck Millipore B.V., DCM: Merck Millipore B.V.]. Lipids were extracted with 450 μL methanol/DCM (50/50, v/v). Samples were vortexed and incubated under constant agitation for 30 min at 4°C followed by centrifugation at 18,500 g for 20 min at 4°C (Hettich mikro 200R, Geldermalsen, Netherlands). Supernatants were transferred to glass vials, freeze dried and reconstituted in 100 μL methanol prior to liquid chromatography-tandem mass spectrometry (LC-MSMS).
Liquid Chromatography-Tandem Mass Spectrometry Analysis
An LC-30A autosampler (Shimadzu, Kyoto, Japan) injected 10 μL brain lipid extracts or 5 μL plasma lipid extracts into a Shimadzu HPLC system (Shimadzu) equipped with a Kinetex C8 column (50 mm × 2.1 mm, 2.6 μm, 00B-4497-AN, Phenomenex, Maarssen, Netherlands) at 30°C. After washing with 95% mobile phase A [MQ/methanol (50/50, v/v) containing 1.5 mM ammonium formate and 0.1% formic acid] and 5% mobile phase B (methanol containing 1 mM ammonium formate and 0.1% formic acid) for 2 min, elution was performed by a linear gradient from 95% mobile phase A and 5% mobile phase B to 7% mobile phase A and 93% mobile phase B in 5.5 min, which was held for 4.5 min. After 10 min the column was flushed with 99% mobile phase B for 2 min followed by a 2 min re-equilibration. The flow rate was set at 0.25 mL/min and total run time was 14 min. The effluent was directed to a Sciex Qtrap 5500 quadruple mass spectrometer (AB Sciex Inc., Thornhill, ON, Canada) and analyzed in positive ion mode following electrospray ionization using multiple reaction monitoring. Detailed LC-MS/MS settings for each sphingolipid species are given in Supplementary Table 1.
We quantified S1P and the seven most abundant ceramide species for which standards were commercially available. Nine-point calibration curves were constructed by plotting analyte to internal standard peak area ratios versus the corresponding analyte concentration for Cer(d18:1/14:0), Cer(d18:1/16:0), Cer(d18:1/18:0), Cer(d18:1/20:0), Cer(d18:1/22:0), Cer(d18:1/24:1), Cer(d18:1/24:0), and S1P(d18:1) (all Avanti polar lipids). Correlation coefficients (R2) were >0.99. Sphingolipid levels were determined from these standard curves based on sphingolipid species acyl chain length. Instrument control and quantification of spectral data was performed using MultiQuant software (AB Sciex Inc.). Brain sphingolipid levels were normalized to mg tissue weight and plasma sphingolipid levels to mL plasma used for analysis.
Statistical Analyses
All outcome parameters were analyzed with IBM SPSS Statistics version 24.0. Group allocation of all experimental animals was known prior to statistical analysis. For sphingolipid parameters Z-values were calculated and individual values that corresponded to a Z-value that deviated more than 4 from the center were considered outliers. Of all data points, 0.84% were excluded as outliers. Normal distribution of the data was confirmed by the Shapiro–Wilk test.
Four main parameters, APOE genotype, FAD mutations, age, and sex, determined to which group mice were assigned, with a total of sixteen groups. The interaction between these four main parameters was analyzed by a generalized linear model to assess whether a combination of these four parameters affected the S1P and ceramide levels and ceramide distribution differently than the individual parameters. Relative ceramide levels were calculated by dividing the level of the individual species by the sum of all the variants measured. Univariate analysis was used to assess the effects of the four parameters on S1P(d18:1), while multivariate analysis was used to assess the effects on the individual ceramide species. The Benjamini–Hochberg procedure (false discover rate = 0.05) was used to correct for multiple testing (Benjamini and Hochberg, 1995). Pearson’s test was used to assess the correlation between sphingolipid levels in plasma and brain regions in female or in male mice.
Results
For the present study, 5xFAD mice were cross-bred with APOE3-TR and APOE4-TR mice to obtain the E3FAD and E4FAD mice. To confirm an AD phenotype in the E3FAD and E4FAD mice (Tai et al., 2011; Youmans et al., 2012a,b) Aβ deposition was determined in the hippocampus (Youmans et al., 2012b) by enzyme-linked immunoassay (Crivelli et al., 2021) as described in Supplementary Information 2. Tris-buffered saline (TBS) soluble, TBS-1% Triton-X (TBS-T) soluble, and formic acid (FA) soluble Aβ depositions were detectable in the hippocampus of the E3FAD and E4FAD mice. Older mice showed a higher extent of Aβ depositions than young mice, irrespective of APOE genotype or sex (all p < 0.001; Supplementary Figure 1).
Overall Effect of APOE4 Genotype, Familial Alzheimer’s Disease Mutations, Age, and Sex on Sphingosine-1-Phosphate and Ceramide Levels in Brain and in Plasma
The effect of APOE genotype, FAD mutations, age, and sex on S1P and ceramide levels in the hippocampus, cortex, cerebellum and plasma of mice is shown in Figures 1–3 (individual ceramides in Supplementary Figures 2–8). Figure 1 shows that S1P levels in all brain regions tended to be higher in older than in younger mice. Total ceramide levels were mostly affected by sex (Figure 2). In the hippocampus, total ceramide levels tended to be lower in female than in male mice, while in the cortex they tended to be higher. When examining the overall differences in total and specific ceramide and S1P levels in all groups, we found no significant interaction between the four independent parameters, APOE genotype, FAD mutations, age, and sex (Supplementary Tables 2, 3). This allowed us to assess the effect of each parameter separately and independently and hence only the main effects of APOE genotype, FAD mutations, age, and sex are displayed below (Figure 3).
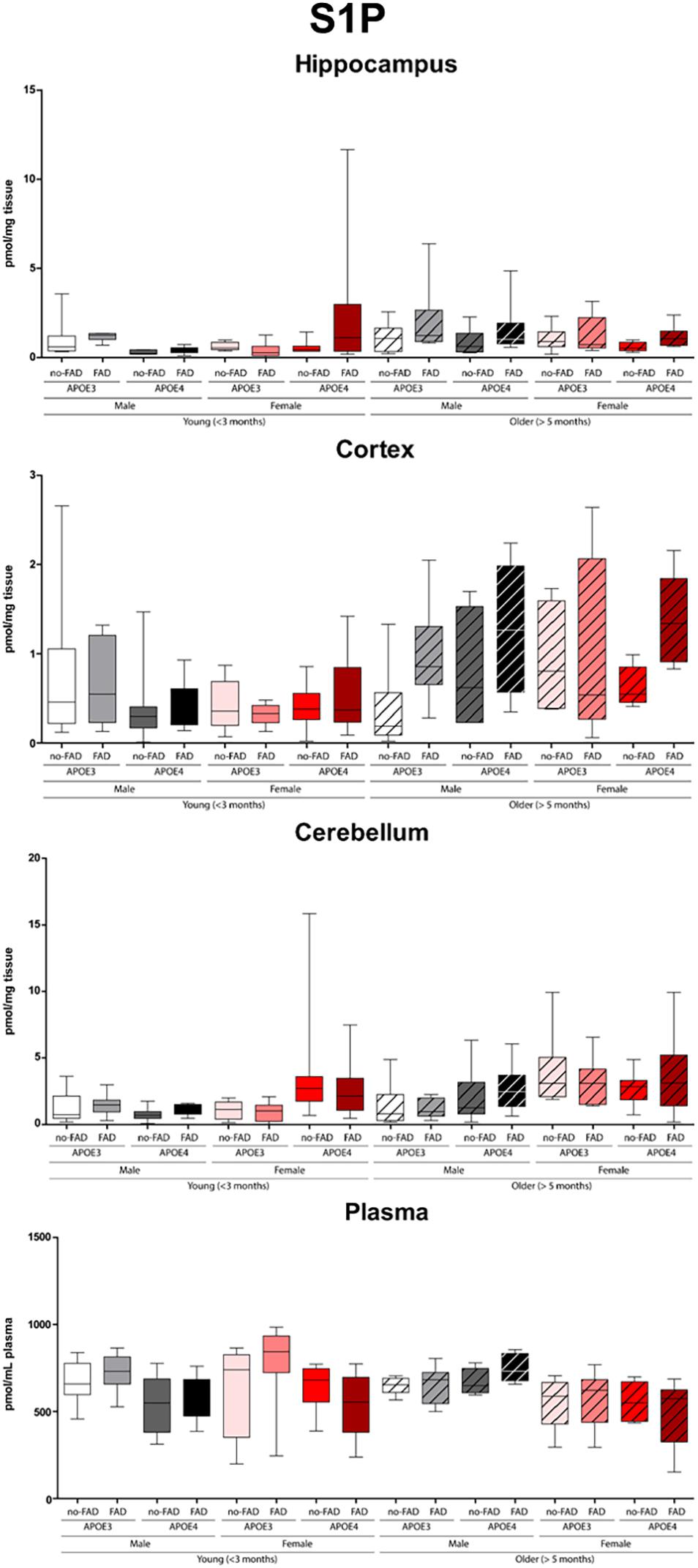
Figure 1. Sphingosine-1-phosphate (S1P) levels in hippocampus, cortex, cerebellum, and plasma. Data are provided as median (line, box dimensions 25th–75th percentile, whiskers min–max) in pmol/mg tissue or pmol/mL plasma (n = 7–10 mice per group for brain samples and n = 4–10 per group for plasma samples).
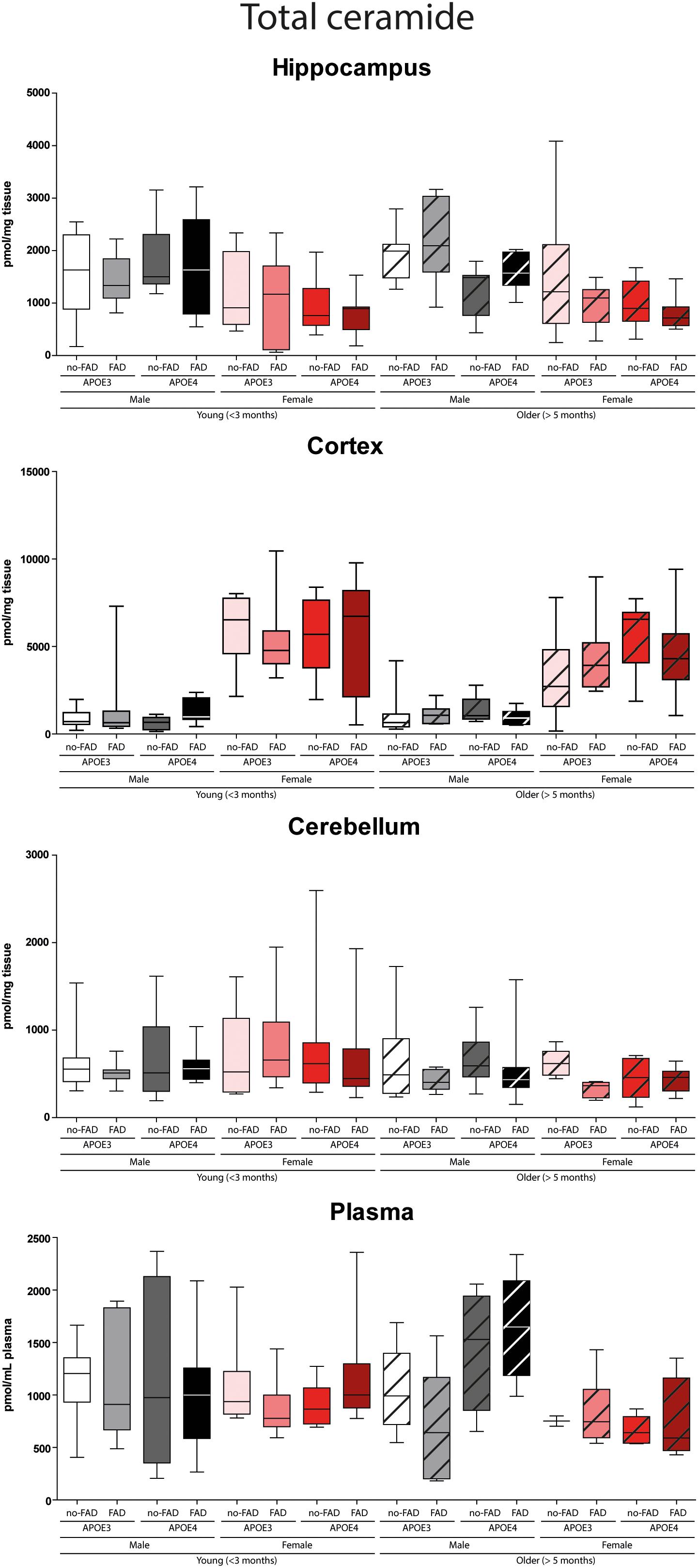
Figure 2. Total determined ceramide levels in hippocampus, cortex, cerebellum, and plasma. Data are given as median (line, box dimensions 25th–75th percentile, whiskers min–max) in pmol/mg tissue or pmol/mL plasma (n = 7–10 mice per group for brain samples and n = 4–10 per group for plasma samples).
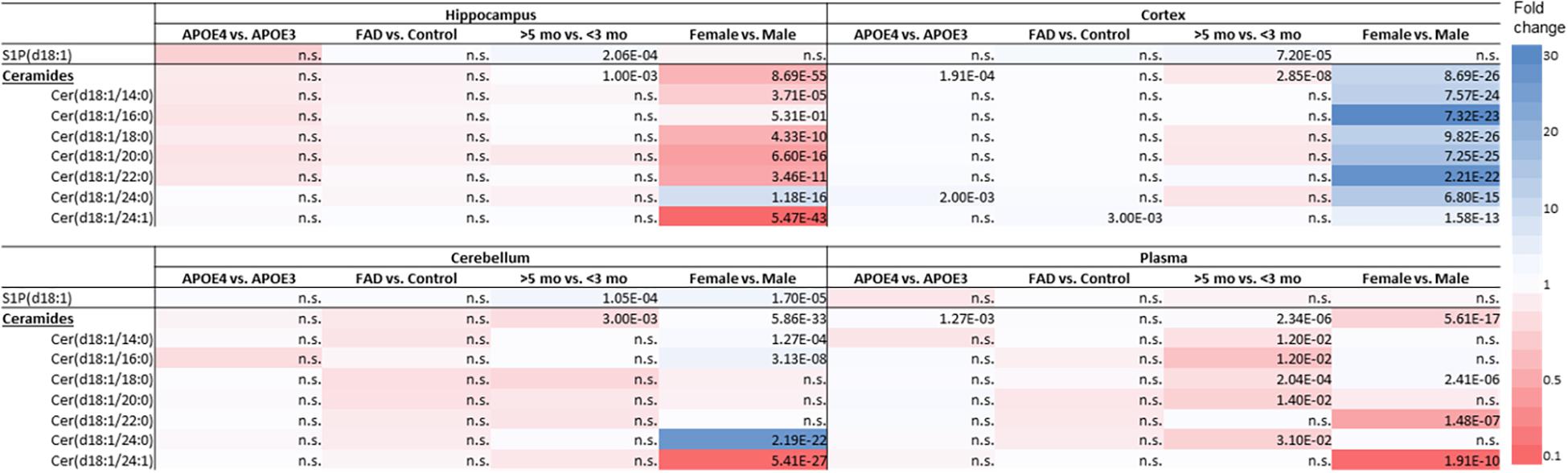
Figure 3. Effect of APOE genotype (APOE4 vs. APOE3), familial AD mutations (FAD vs. non-FAD), age (>5 mo vs. <3 mo), and sex (♂ vs. ♀) on sphingolipid levels in hippocampus, cortex, cerebellum and in plasma of mice. Color-scale indicates the differences in sphingolipid levels; blue indicates up to 30-fold higher levels and red indicates up to 10-fold lower sphingolipid levels. P-values of significant differences after correction for multiple testing are indicated in the table, n.s., non-significant (n = 45–76 mice per group).
Limited Effects of APOE Genotype on Levels of Sphingosine-1-Phosphate and Ceramide in Brain and in Plasma
APOE4 compared to APOE3 showed limited impact on few sphingolipids in brain and in plasma (Figure 3). S1P(d18:1) levels were not affected by APOE genotype. Ceramide levels in the cortex were higher in APOE4 than in APOE3 carriers (1.1-fold, p < 0.001). When analyzing individual ceramides, levels of Cer(d18:1/24:0) exclusively were significantly higher in the cortex of APOE4 than of APOE3 carriers (1.7-fold, p = 0.002). APOE4 compared to APOE3 mice also displayed higher levels of total ceramide levels in plasma (1.1-fold, p = 0.001), mostly due to higher levels of Cer(d18:1/20:0) (1.4-fold, p = 0.012).
Limited Effects of Familial Alzheimer’s Disease Mutations on Sphingolipid Profiles in Brain and Plasma
Familial Alzheimer’s disease mutations hardly affected sphingolipids in brain or in plasma (Figure 3). While mice with APOE4 display higher levels of Cer(d18:1/24:0), mice with FAD mutations displayed higher levels of Cer(d18:1/24:1) in the cortex than in mice without the FAD mutations (1.4-fold, p = 0.003).
Limited Effects of Age on Sphingolipid Profiles in Brain and Plasma
Older mice displayed higher levels of S1P(d18:1) in the hippocampus, cortex, and cerebellum than younger mice (1.7 – 1.8-fold, all p < 0.001), while there were no differences in plasma (Figure 3). On the other hand, total cortex and cerebellum ceramide levels were lower in older than in young mice (1.1 – 1.2-fold, all p ≤ 0.003), which could not be attributed to individual ceramide species. Total ceramide levels in plasma were also lower in older than in young mice (1.1-fold, p < 0.001), which was due to lower levels of most of the individual ceramides (1.6 – 4.4-fold, all p ≤ 0.012).
Effects of Sex on Sphingolipid Profiles in Brain and Plasma
In the cerebellum S1P(d18:1) levels were higher in female than in male mice (1.9-fold, p < 0.001), while levels did not differ in the hippocampus, cortex, and in plasma (Figure 3).
Total ceramide levels in the hippocampus were lower in female than in male mice (4.3-fold, p < 0.001). In line, levels of five out of seven individual ceramide species were lower in female than in male mice (2.7 – 9.7-fold, all p < 0.001), whereas Cer(d18:1/24:0) levels were fourfold higher (p < 0.001). In contrast with the findings in the hippocampus, total ceramide levels in cortex were higher in female than in male mice (5.1-fold, p < 0.001), as were all individual ceramide species (2.3 – 12.8-fold, all p < 0.001). Also in the cerebellum total ceramide levels were slightly, but significantly, higher in female than in male mice (1.05-fold, p < 0.001), due to three out of seven individual ceramide species (1.3 – 11.5-fold, all p < 0.001), whereas Cer(d18:1/24:1) was 9.5-fold lower (p < 0.001). In line with findings in the hippocampus, total ceramide levels in plasma, were lower in female than in male mice (3.4-fold, p < 0.001), mostly due to lower Cer(d18:1/22:0) and Cer(d18:1/24:1) levels (6.2 – 9.5-fold, both p < 0.001). However, Cer(d18:1/18:0) levels were higher (1.3-fold, p < 0.001).
Effect of APOE4 Genotype, Familial Alzheimer’s Disease Mutations, Age, and Sex on Ceramide Acyl Chain Length Distribution
As ceramide acyl chain composition can affect sphingolipid function we examined the acyl chain length distribution in further detail. The effects of APOE genotype, FAD mutations, age and sex on ceramide acyl chain length distribution were in line with the data on the absolute levels (Supplementary Figure 9).
Cortex and Hippocampus Sphingosine-1-Phosphate Levels Correlate With Plasma Levels in Female Mice
In female mice, plasma S1P(d18:1) levels negatively associated with those in the cortex (r = −0.53, [95%CI: −0.71, −0.32], p < 0.001) and hippocampus (r = −0.54, [95%CI: −0.70, −0.35], p < 0.001), regardless of age, APOE genotype and presence of FAD mutations. In male mice, no correlation was found between S1P or ceramide levels in plasma and any of the brain regions analyzed.
Validation of Effects of APOE4 Genotype, Age, and Sex on Sphingosine-1-Phosphate and Ceramide Levels in a Small Cohort of Mice
To confirm our findings that sex greatly affected ceramide and to a smaller extent S1P levels in the hippocampus and cortex of our mice we reanalyzed brain tissues from a selection of mice (n = 20 per region) by a different extraction, sample preparation, and LC-MSMS method. S1P levels were determined as previously described (Mirzaian et al., 2016). Total ceramide levels were determined by microwave-induced deacylation followed by quantification of the sphingoid base (Mirzaian et al., 2017). The latter method somewhat overestimates total ceramide levels since some of the sulfatides also lose their sulfate group during deacylation. These results showed qualitatively similar results to our study with total ceramide levels being lower in the hippocampus and higher in the cortex of female than of male mice (Table 2). No effect of APOE genotype or age on ceramide levels was found, whereas S1P levels in the hippocampus were lower in the APOE4 mice than in APOE3 mice.
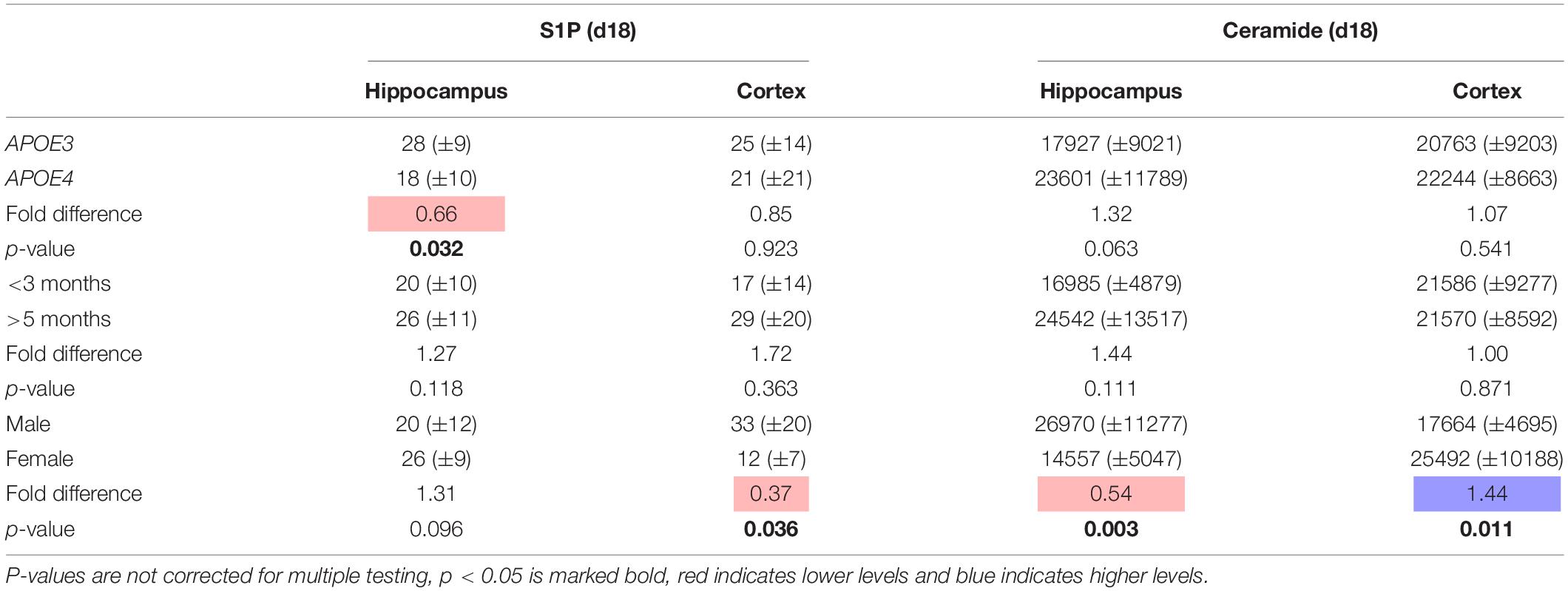
Table 2. Effect of APOE genotype (APOE4 vs. APOE3), age (>5 months vs. <3 months), and sex (female vs. male) on S1P and ceramide levels (mean ± sd pmol/mg protein) in hippocampus and cortex of a selection of mice to validate study results (n = 20).
Discussion
The data of this explorative study revealed that APOE genotype, FAD mutations, and age affect overall brain and plasma sphingolipids to a very limited extent. Unexpectedly, sex notably affected ceramide levels, e.g., with ceramide levels being in the hippocampus and higher in the cortex of female than of male mice. The limited effect of APOE genotype on sphingolipid levels, even in AD mice, might be due to the fact that the mice in our study were relatively younger (5.4–14.3 months, only 3 mice ≥ 10 months) than in previously published papers. Previously, we found APOE4 knock-in mice > 15 months old to display lower ceramide levels in the brain than wild-type mice (den Hoedt et al., 2016) suggesting an effect of APOE genotype on sphingolipids may become apparent with increasing age or that human APOE3 affects ceramide differently from mouse apoE. The observed and externally validated profound effects of sex on sphingolipids may provide an avenue to further explore sex-specific mechanisms contributing to disease progression in men and women with Alzheimer’s disease.
Although, the ε4 allele of the APOE gene is long known to be the strongest genetic risk factor for the development of late-onset AD, the underlying mechanisms contributing to disease progression remain to be established. Differences in lipidation of ApoE4 and ApoE3 secreted by astrocytes have been detected in AD (Verghese et al., 2013; Grimm et al., 2017), with potential consequences for the clearance of Aß from the brain. So far, no major effects of APOE genotype on brain lipids, such as sterols, phospholipids, fatty acids or ceramides have been observed (Mulder et al., 1998; Martins et al., 2006; Sharman et al., 2010; Lim et al., 2014). Our data show minor modulatory effects of the APOE genotype on overall sphingolipid homeostasis. Interestingly, only Cer(d18:1/24:0) levels were significantly higher in the cortex of APOE4 mice irrespective of sex, age, and FAD mutations. Higher levels of Cer(d18:1/24:0) were also observed in the brain of AD patients (Cutler et al., 2004a). Increased levels of intracellular Cer(d18:1/24:0) have been found to induce apoptosis in cultured neutrophils (Seumois et al., 2007), but Cutler et al. (2004a) could not detect any direct link with apoptosis. It cannot be excluded that the increased Cer(d18:1/24:0) levels observed in the cortex of the APOE4 mice in our study contribute, via inducing apoptosis, to the neuronal loss that is a prominent pathological feature of AD. With aging this may further exacerbate the neurodegenerative processes.
Minor effects of the FAD mutations on brain sphingolipids were observed. Our observation that mice with the FAD mutations show significantly higher levels of Cer(d18:1/24:1) in the cortex than mice without the mutations is in line with previously reported data of the APP(SL)/PS1 knock-in AD mouse model (Barrier et al., 2010). Ceramides have been suggested to play a role in neuroinflammatory processes occurring in neurodegenerative diseases like AD. In reactive astrocytes of patients with late-onset AD, frontotemporal lobar dementia, and capillary cerebral amyloid angiopathy, high ceramide levels and an increased expression of ceramide synthase 5, the enzyme responsible for Cer(d18:1/16:0) production, were observed (van Doorn et al., 2012; de Wit et al., 2016, 2019). Additionally, in individuals with a parental history of late-onset AD, cerebral spinal fluid Cer(d18:1/18:0) levels correlated with Aβ and T-tau levels (Mielke et al., 2014). On the other hand high serum ceramide levels, especially Cer(d18:1/16:0) and Cer(d18:1/24:0), were also observed to be associated with the risk of developing sporadic late-onset AD (Mielke et al., 2012). Although we observed only Cer(d18:1/24:1) levels increased because of the FAD mutations on brain ceramide levels, these effects are in line with the proposed role of ceramides in the pathogenesis of AD.
Effects of aging on sphingolipid profiles have previously been reported. Age-related increases in ceramide levels and decrease in S1P were detected in the hippocampus of cognitively normal individuals of 65 years or older (Cutler et al., 2004b; Couttas et al., 2018). An accumulation upon aging of ceramide in the cortex and hippocampus has also been reported in wild-type mice and rats (Cutler et al., 2004b; Durani et al., 2017; Vozella et al., 2017), suggesting that these changes reflect normal aging processes. In contrast, we observed a modest increase in S1P and a modest decrease in ceramides in all brain regions upon maturation of the mice, irrespective of APOE genotype, FAD mutations, and sex. However, the previously reported increase in ceramides were detected in mice that were almost twice as old as the eldest mice in this study, which may explain the lack of such a difference. Interestingly, S1P levels were significantly higher in the brain regions of older than of younger mice. S1P has been suggested to modulate synaptic strength (Kanno et al., 2010), brain inflammation, and cerebrovascular integrity (Chua et al., 2020). Upon aging, the increased S1P levels in combination with higher Aß42 levels may deteriorate synaptic function and blood–brain barrier integrity during the progression of AD. However, the origin of the S1P needs to be further investigated, because region-specific differences in sphingolipid metabolism have been observed (Blot et al., 2021). In plasma, S1P levels were reported to be higher in females compared to males in response to estradiol starting at a relatively young age (Guo et al., 2014). Yet with aging and menopause S1P plasma levels were downregulated (Guo et al., 2014). In our study, we could not reproduce these findings probably because of small samples size or because of the fact that the older females were employed as breeders. During pregnancy the levels of estradiol fluctuate (Bai et al., 2020), with possible consequences for S1P regulation (Guo et al., 2014).
So far reports on sex-specific effects on sphingolipid profiles in the brain of mice are limited. Sex-specific differences in sphingolipid levels in the cortex of APPSL/PS1 mice, but not in PS1 mice have been reported (Barrier et al., 2010). Female APPSL/PS1 mice display lower levels of saturated fatty acid ceramides [i.e., Cer(d18:1/24:0)], and higher levels of unsaturated fatty acid ceramides [Cer(d18:1/24:1)], than male mice in the cortex at the age of 3 and 6 months (Barrier et al., 2010). In contrast with these data, we found statistically relevant higher levels of saturated fatty acid ceramides and lower levels of unsaturated fatty acid ceramides in the cortex of female as compared to male mice. This discrepancy might be due to the different backgrounds of the mice (Casas et al., 2004). Moreover, it has to be noticed that our female mice were former breeders.
Sex-dependent effects on ceramide levels have also been reported for human hippocampus, where ceramide levels correlated with age in men exclusively (Couttas et al., 2018). In addition, the elevated plasma ceramide levels in (menopausal) women, as compared to men, without cognitive impairment negatively correlated with estradiol levels (Mielke et al., 2015; Vozella et al., 2019). Estradiol was found to decrease hypothalamic ceramide levels and thereby endoplasmatic reticulum stress in female rats (Gonzalez-Garcia et al., 2018), which is in line with the lower ceramide levels we observed in the hippocampus of female compared to male mice. Estradiol was also found to modulate plasma membrane lipid rafts, highly enriched in ceramides and other sphingolipids and where the amyloidogenic processing of APP takes place (Cordy et al., 2006). The reduction of estradiol associated with menopause could contribute to the development of AD via a modulatory effect on lipid raft composition (Marin and Diaz, 2018). The differences in sphingolipids between sexes might provide insight into metabolism-related differences between men and women that may contribute to the development of AD and underline the importance of the use of both sexes in future studies.
Notably, we found ceramide levels to be higher in the cortex than in the hippocampus. This may have critical implications when designing drugs to control ceramide levels in the brain (Giles et al., 2017). In fact, the response to the ceramide modulators may be different depending on brain region or even cell type (Fitzner et al., 2020).
There are several limitations in this study. First, the AD model used reflects familial (early-onset) AD, whereas APOE4 is a genetic risk factor for sporadic late-onset AD and most studies reporting a link between sphingolipid levels and cognitive decline were performed in patients with sporadic late-onset AD (Mielke et al., 2010a, 2011; Mielke and Haughey, 2012). Although, the FAD mouse is a model for familial AD, similar to sporadic AD the mice display Aβ deposition, neuroinflammation, and cognitive impairment (Tai et al., 2017; Youmans et al., 2012b). Secondly, although the older mice (>5 months) did show increased Aß levels, our mice were relatively young. Senescence effects in mice are generally not observed before the age of 10 months when they are considered middle aged (Jonas, 2007; Dutta and Sengupta, 2016). We did observe changes in sphingolipid levels in relatively young mice depending on FAD mutations, APOE4 genotype, or sex which may suggest these contribute to the later development of AD. Thirdly, due to the nature of the study and the four parameters that determined the sixteen different groups there were relatively few mice per individual group, especially considering plasma analysis. However, the very limited interaction effects between APOE genotype, FAD mutations, age, and sex allowed us to address their effect on S1P and ceramide levels as independent parameters in groups of sufficient size (n = 45–76 per group). The sex-specific findings were externally validated further strengthening our findings. Finally, our analysis comprised a selected number of sphingolipids, e.g., S1P and seven ceramides. Though the analysis of additional sphingolipids, such as hexosylceramides and sulfatides, is undoubtedly interesting in association to FAD mutation and age (Crivelli et al., 2020b), we here focused on S1P and ceramide as they are important signaling sphingolipids and commercial MS standards were available for these lipids. Brain sphingosine levels were below the detection limit of our LC-MSMS setup and were therefore not reported.
Conclusion
Our data shows very limited effects of APOE genotype on very-long chain ceramides [Cer(d18:1/24:0)], which might represent one of the early signs of neuroinflammation that may worsen with aging. Unexpectedly, sex was found to profoundly affect ceramide levels in plasma and in brain in particular in the cortex and hippocampus. A role for sex hormones needs further investigation. If and how these brain ceramide profiles affect the pathogenesis of AD differently in men and women remains to be examined. S1P levels in the brain increased with aging and in female mice S1P levels in cortex and hippocampus negatively correlate with levels in plasma. Therefore, plasma S1P might be of interest for future investigation as proxy for alterations in brain sphingolipid metabolism, and to explore if these are related to the progression of neurodegenerative processes.
Data Availability Statement
The raw data supporting the conclusions of this article will be made available by the authors, without undue reservation.
Ethics Statement
The animal study was reviewed and approved by Animal Welfare Committee of Maastricht University.
Author Contributions
SH: methodology, formal analysis, investigation, data curation, writing – original draft, and visualization. SC, ML, JS, and MM-D: methodology, resources, and writing – review and editing. FL: methodology, investigation, and writing – review and editing. HV: conceptualization, writing – review and editing, and funding acquisition. JW: conceptualization and writing – review and editing, and funding acquisition. ES: writing – review and editing. AV: methodology and writing – original draft. PM-M: conceptualization, methodology, resources, writing – review and editing, and funding acquisition. MTM: conceptualization, methodology, writing – original draft, supervision, project administration, and funding acquisition. All authors contributed to the article and approved the submitted version.
Funding
This work was supported by grants to SH, SC, JW, HV, PM-M, and MTM from ZonMw Memorabel program (projectnr: 733050105). PM-M was also supported by the International Foundation for Alzheimer Research (ISAO) (projectnr: 14545).
Conflict of Interest
The authors declare that the research was conducted in the absence of any commercial or financial relationships that could be construed as a potential conflict of interest.
Publisher’s Note
All claims expressed in this article are solely those of the authors and do not necessarily represent those of their affiliated organizations, or those of the publisher, the editors and the reviewers. Any product that may be evaluated in this article, or claim that may be made by its manufacturer, is not guaranteed or endorsed by the publisher.
Supplementary Material
The Supplementary Material for this article can be found online at: https://www.frontiersin.org/articles/10.3389/fnagi.2021.765252/full#supplementary-material
References
Abildayeva, K., Jansen, P. J., Hirsch-Reinshagen, V., Bloks, V. W., Bakker, A. H., Ramaekers, F. C., et al. (2006). 24(S)-hydroxycholesterol participates in a liver X receptor-controlled pathway in astrocytes that regulates apolipoprotein E-mediated cholesterol efflux. J. Biol. Chem. 281, 12799–12808. doi: 10.1074/jbc.m601019200
Bai, J., Qi, Q. R., Li, Y., Day, R., Makhoul, J., Magness, R. R., et al. (2020). Estrogen Receptors and Estrogen-Induced Uterine Vasodilation in Pregnancy. Int. J. Mol. Sci. 21:4349. doi: 10.3390/ijms21124349
Bandaru, V. V., Troncoso, J., Wheeler, D., Pletnikova, O., Wang, J., Conant, K., et al. (2009). ApoE4 disrupts sterol and sphingolipid metabolism in Alzheimer’s but not normal brain. Neurobiol. Aging 30, 591–599. doi: 10.1016/j.neurobiolaging.2007.07.024
Barrier, L., Ingrand, S., Fauconneau, B., and Page, G. (2010). Gender-dependent accumulation of ceramides in the cerebral cortex of the APP(SL)/PS1Ki mouse model of Alzheimer’s disease. Neurobiol. Aging 31, 1843–1853. doi: 10.1016/j.neurobiolaging.2008.10.011
Bell, R. D., Winkler, E. A., Singh, I., Sagare, A. P., Deane, R., Wu, Z., et al. (2012). Apolipoprotein E controls cerebrovascular integrity via cyclophilin A. Nature 485, 512–516. doi: 10.1038/nature11087
Benjamini, Y., and Hochberg, Y. (1995). Controlling the False Discovery Rate - a Practical and Powerful Approach to Multiple Testing. J. R. Statist. Soc. Ser. B Methodol. 57, 289–300. doi: 10.1111/j.2517-6161.1995.tb02031.x
Bjorkhem, I., and Meaney, S. (2004). Brain cholesterol: long secret life behind a barrier. Arterioscler. Thromb. Vasc. Biol. 24, 806–815. doi: 10.1161/01.atv.0000120374.59826.1b
Blot, F. G. C., Krijnen, W. H. J. J., Hoedt, S. D., Osörio, C., White, J. J., Mulder, M. T., et al. (2021). Sphingolipid metabolism governs Purkinje cell patterned degeneration in Atxn1[82Q]/+ mice. Proc. Natl. Acad. Sci. U. S. A. 118:e2016969118. https://doi.org/10.1073/pnas.2016969118
Brann, A. B., Scott, R., Neuberger, Y., Abulafia, D., Boldin, S., Fainzilber, M., et al. (1999). Ceramide signaling downstream of the p75 neurotrophin receptor mediates the effects of nerve growth factor on outgrowth of cultured hippocampal neurons. J. Neurosci. 19, 8199–8206. doi: 10.1523/jneurosci.19-19-08199.1999
Casas, C., Sergeant, N., Itier, J. M., Blanchard, V., Wirths, O., van der Kolk, N., et al. (2004). Massive CA1/2 neuronal loss with intraneuronal and N-terminal truncated Abeta42 accumulation in a novel Alzheimer transgenic model. Am. J. Pathol. 165, 1289–1300. doi: 10.1016/s0002-9440(10)63388-3
Chartier-Harlin, M. C., Parfitt, M., Legrain, S., Perez-Tur, J., Brousseau, T., Evans, A., et al. (1994). Apolipoprotein E, epsilon 4 allele as a major risk factor for sporadic early and late-onset forms of Alzheimer’s disease: analysis of the 19q13.2 chromosomal region. Hum. Mol. Genet. 3, 569–574. doi: 10.1093/hmg/3.4.569
Chua, X. Y., Ho, L. T. Y., Xiang, P., Chew, W. S., Lam, B. W. S., Chen, C. P., et al. (2020). Preclinical and Clinical Evidence for the Involvement of Sphingosine 1-Phosphate Signaling in the Pathophysiology of Vascular Cognitive Impairment. Neuromolecular. Med. 23, 47–67. doi: 10.1007/s12017-020-08632-0
Corder, E. H., Saunders, A. M., Strittmatter, W. J., Schmechel, D. E., Gaskell, P. C., Small, G. W., et al. (1993). Gene dose of apolipoprotein E type 4 allele and the risk of Alzheimer’s disease in late onset families. Science 261, 921–923. doi: 10.1126/science.8346443
Cordy, J. M., Hooper, N. M., and Turner, A. J. (2006). The involvement of lipid rafts in Alzheimer’s disease. Mol. Membr. Biol. 23, 111–122. doi: 10.1080/09687860500496417
Couttas, T. A., Kain, N., Tran, C., Chatterton, Z., Kwok, J. B., and Don, A. S. (2018). Age-Dependent Changes to Sphingolipid Balance in the Human Hippocampus are Gender-Specific and May Sensitize to Neurodegeneration. J. Alzheimers Dis. 63, 503–514. doi: 10.3233/jad-171054
Crivelli, S. M., Giovagnoni, C., Visseren, L., Scheithauer, A. L., de Wit, N., den Hoedt, S., et al. (2020a). Sphingolipids in Alzheimer’s disease, how can we target them? Adv. Drug Deliv. Rev. 159, 214–231. doi: 10.1016/j.addr.2019.12.003
Crivelli, S. M., Luo, Q., Stevens, J. A. A., Giovagnoni, C., van Kruining, D., Bode, G., et al. (2021). CERTL reduces C16 ceramide, amyloid-beta levels, and inflammation in a model of Alzheimer’s disease. Alzheimers Res. Ther. 13:45.
Crivelli, S. M., van Kruining, D., Luo, Q., Stevens, J. A. A., Giovagnoni, C., Paulus, A., et al. (2020b). Ceramide analog [18F]F-HPA-12 detects sphingolipid disbalance in the brain of Alzheimer’s disease transgenic mice by functioning as a metabolic probe. Sci. Rep. 2020:19354.
Cutler, R. G., Haughey, N. J., Tammara, A., McArthur, J. C., Nath, A., Reid, R., et al. (2004a). Dysregulation of sphingolipid and sterol metabolism by ApoE4 in HIV dementia. Neurology 63, 626–630.
Cutler, R. G., Kelly, J., Storie, K., Pedersen, W. A., Tammara, A., Hatanpaa, K., et al. (2004b). Involvement of oxidative stress-induced abnormalities in ceramide and cholesterol metabolism in brain aging and Alzheimer’s disease. Proc. Natl. Acad. Sci. U S A. 101, 2070–2075. doi: 10.1073/pnas.0305799101
Czubowicz, K., and Strosznajder, R. (2014). Ceramide in the molecular mechanisms of neuronal cell death. The role of sphingosine-1-phosphate. Mol. Neurobiol. 50, 26–37. doi: 10.1007/s12035-013-8606-4
de Wit, N. M., den Hoedt, S., Martinez-Martinez, P., Rozemuller, A. J., Mulder, M. T., and de Vries, H. E. (2019). Astrocytic ceramide as possible indicator of neuroinflammation. J. Neuroinflamm. 16, 48.
de Wit, N. M., Snkhchyan, H., den Hoedt, S., Wattimena, D., de Vos, R., Mulder, M. T., et al. (2016). Altered Sphingolipid Balance in Capillary Cerebral Amyloid Angiopathy. J. Alzheimers Dis. 60, 795–807. doi: 10.3233/jad-160551
den Hoedt, S., Janssen, C. I., Astarita, G., Piomelli, D., Leijten, F. P., Crivelli, S. M., et al. (2016). Pleiotropic Effect of Human ApoE4 on Cerebral Ceramide and Saturated Fatty Acid Levels. J. Alzheimers Dis. 60, 769–781.
Durani, L. W., Hamezah, H. S., Ibrahim, N. F., Yanagisawa, D., Makpol, S., Damanhuri, H. A., et al. (2017). Age-related changes in the metabolic profiles of rat hippocampus, medial prefrontal cortex and striatum. Biochem. Biophys. Res. Commun. 493, 1356–1363. doi: 10.1016/j.bbrc.2017.09.164
Dutta, S., and Sengupta, P. (2016). Men and mice: Relating their ages. Life Sci. 152, 244–248. doi: 10.1016/j.lfs.2015.10.025
Farrer, L. A., Cupples, L. A., Haines, J. L., Hyman, B., Kukull, W. A., Mayeux, R., et al. (1997). Effects of age, sex, and ethnicity on the association between apolipoprotein E genotype and Alzheimer disease. A meta-analysis. APOE and Alzheimer Disease Meta Analysis Consortium. JAMA 278, 1349–1356.
Fitzner, D., Bader, J. M., Penkert, H., Bergner, C. G., Su, M., Weil, M. T., et al. (2020). Cell-Type- and Brain-Region-Resolved Mouse Brain Lipidome. Cell Rep. 32:108132. doi: 10.1016/j.celrep.2020.108132
Fratiglioni, L., Launer, L. J., Andersen, K., Breteler, M. M., Copeland, J. R., Dartigues, J. F., et al. (2000). Incidence of dementia and major subtypes in Europe: A collaborative study of population-based cohorts. Neurologic Diseases in the Elderly Research Group. Neurology 54, S10–S15.
Giles, C., Takechi, R., Mellett, N. A., Meikle, P. J., Dhaliwal, S., and Mamo, J. C. (2017). Differential regulation of sphingolipid metabolism in plasma, hippocampus, and cerebral cortex of mice administered sphingolipid modulating agents. J. Neurochem. 141, 413–422.
Gonzalez-Garcia, I., Contreras, C., Estevez-Salguero, A., Ruiz-Pino, F., Colsh, B., Pensado, I., et al. (2018). Estradiol Regulates Energy Balance by Ameliorating Hypothalamic Ceramide-Induced ER Stress. Cell Rep. 25, 413–423e415.
Grimm, M. O. W., Michaelson, D. M., and Hartmann, T. (2017). Omega-3 fatty acids, lipids, and apoE lipidation in Alzheimer’s disease: a rationale for multi-nutrient dementia prevention. J. Lipid Res. 58, 2083–2101. doi: 10.1194/jlr.r076331
Guo, S., Yu, Y., Zhang, N., Cui, Y., Zhai, L., Li, H., et al. (2014). Higher level of plasma bioactive molecule sphingosine 1-phosphate in women is associated with estrogen. Biochim. Biophys. Acta 1841, 836–846. doi: 10.1016/j.bbalip.2014.02.005
Hannun, Y. A. (1994). The sphingomyelin cycle and the second messenger function of ceramide. J. Biol. Chem. 269, 3125–3128. doi: 10.1016/s0021-9258(17)41834-5
Haughey, N. J., Bandaru, V. V., Bae, M., and Mattson, M. P. (2010). Roles for dysfunctional sphingolipid metabolism in Alzheimer’s disease neuropathogenesis. Biochim. Biophys. Acta 1801, 878–886. doi: 10.1016/j.bbalip.2010.05.003
Holtzman, D. M., Herz, J., and Bu, G. (2012). Apolipoprotein E and apolipoprotein E receptors: normal biology and roles in Alzheimer disease. Cold Spring Harb. Perspect. Med. 2:a006312. doi: 10.1101/cshperspect.a006312
Jana, A., Hogan, E. L., and Pahan, K. (2009). Ceramide and neurodegeneration: susceptibility of neurons and oligodendrocytes to cell damage and death. J. Neurol. Sci. 278, 5–15. doi: 10.1016/j.jns.2008.12.010
Jorm, A. F., and Jolley, D. (1998). The incidence of dementia: a meta-analysis. Neurology 51, 728–733. doi: 10.1212/wnl.51.3.728
Kang, J., Lemaire, H. G., Unterbeck, A., Salbaum, J. M., Masters, C. L., Grzeschik, K. H., et al. (1987). The precursor of Alzheimer’s disease amyloid A4 protein resembles a cell-surface receptor. Nature 325, 733–736. doi: 10.1038/325733a0
Kanno, T., Nishizaki, T., Proia, R. L., Kajimoto, T., Jahangeer, S., Okada, T., et al. (2010). Regulation of synaptic strength by sphingosine 1-phosphate in the hippocampus. Neuroscience 171, 973–980. doi: 10.1016/j.neuroscience.2010.10.021
Kloske, C. M., and Wilcock, D. M. (2020). The Important Interface Between Apolipoprotein E and Neuroinflammation in Alzheimer’s Disease. Front. Immunol. 11:754. doi: 10.3389/fimmu.2020.00754
Lazzarini, A., Macchiarulo, A., Floridi, A., Coletti, A., Cataldi, S., Codini, M., et al. (2015). Very-long-chain fatty acid sphingomyelin in nuclear lipid microdomains of hepatocytes and hepatoma cells: can the exchange from C24:0 to C16:0 affect signal proteins and vitamin D receptor? Mol. Biol. Cell 26, 2418–2425. doi: 10.1091/mbc.e15-04-0229
Levy-Lahad, E., Wasco, W., Poorkaj, P., Romano, D. M., Oshima, J., Pettingell, W. H., et al. (1995). Candidate gene for the chromosome 1 familial Alzheimer’s disease locus. Science 269, 973–977. doi: 10.1126/science.7638622
Liesinger, A. M., Graff-Radford, N. R., Duara, R., Carter, R. E., Hanna Al-Shaikh, F. S., Koga, S., et al. (2018). Sex and age interact to determine clinicopathologic differences in Alzheimer’s disease. Acta Neuropathol. 136, 873–885. doi: 10.1007/s00401-018-1908-x
Lim, W. L., Martins, I. J., and Martins, R. N. (2014). The involvement of lipids in Alzheimer’s disease. J. Genet. Genomics 41, 261–274. doi: 10.1016/j.jgg.2014.04.003
Liu, Y., Peterson, D. A., and Schubert, D. (1998). Amyloid beta peptide alters intracellular vesicle trafficking and cholesterol homeostasis. Proc. Natl. Acad. Sci. U S A. 95, 13266–13271. doi: 10.1073/pnas.95.22.13266
Mahley, R. W. (2016). Central Nervous System Lipoproteins: ApoE and Regulation of Cholesterol Metabolism. Arterioscler. Thromb. Vasc. Biol. 36, 1305–1315. doi: 10.1161/ATVBAHA.116.307023
Marin, R., and Diaz, M. (2018). Estrogen Interactions With Lipid Rafts Related to Neuroprotection. Impact of Brain Ageing and Menopause. Front. Neurosci. 12:128. doi: 10.3389/fnins.2018.00128
Martinez Martinez, P., and Mielke, M. M. (2017). Sphingolipids in Alzheimer’s Disease and Related Disorders. J. Alzheimers Dis. 60, 753–756. doi: 10.3233/JAD-170735
Martins, I. J., Hone, E., Foster, J. K., Sunram-Lea, S. I., Gnjec, A., Fuller, S. J., et al. (2006). Apolipoprotein E, cholesterol metabolism, diabetes, and the convergence of risk factors for Alzheimer’s disease and cardiovascular disease. Mol. Psychiatry 11, 721–736. doi: 10.1038/sj.mp.4001854
Maynard, C. J., Cappai, R., Volitakis, I., Cherny, R. A., Masters, C. L., Li, Q. X., et al. (2006). Gender and genetic background effects on brain metal levels in APP transgenic and normal mice: implications for Alzheimer beta-amyloid pathology. J. Inorg. Biochem. 100, 952–962. doi: 10.1016/j.jinorgbio.2006.02.010
Mielke, M. M., and Haughey, N. J. (2012). Could plasma sphingolipids be diagnostic or prognostic biomarkers for Alzheimer’s disease? Clin. Lipidol. 7, 525–536. doi: 10.2217/clp.12.59
Mielke, M. M., Bandaru, V. V., Han, D., An, Y., Resnick, S. M., Ferrucci, L., et al. (2015). Demographic and clinical variables affecting mid- to late-life trajectories of plasma ceramide and dihydroceramide species. Aging Cell 2015, 1014–1023. doi: 10.1111/acel.12369
Mielke, M. M., Bandaru, V. V., Haughey, N. J., Rabins, P. V., Lyketsos, C. G., and Carlson, M. C. (2010a). Serum sphingomyelins and ceramides are early predictors of memory impairment. Neurobiol. Aging 31, 17–24. doi: 10.1016/j.neurobiolaging.2008.03.011
Mielke, M. M., Bandaru, V. V., Haughey, N. J., Xia, J., Fried, L. P., Yasar, S., et al. (2012). Serum ceramides increase the risk of Alzheimer disease: the Women’s Health and Aging Study II. Neurology 79, 633–641. doi: 10.1212/WNL.0b013e318264e380
Mielke, M. M., Haughey, N. J., Bandaru, V. V., Schech, S., Carrick, R., Carlson, M. C., et al. (2010b). Plasma ceramides are altered in mild cognitive impairment and predict cognitive decline and hippocampal volume loss. Alzheimers Dement. 6, 378–385. doi: 10.1016/j.jalz.2010.03.014
Mielke, M. M., Haughey, N. J., Bandaru, V. V., Weinberg, D. D., Darby, E., Zaidi, N., et al. (2011). Plasma sphingomyelins are associated with cognitive progression in Alzheimer’s disease. J. Alzheimers Dis. 27, 259–269. doi: 10.3233/JAD-2011-110405
Mielke, M. M., Haughey, N. J., Bandaru, V. V., Zetterberg, H., Blennow, K., Andreasson, U., et al. (2014). Cerebrospinal fluid sphingolipids, beta-amyloid, and tau in adults at risk for Alzheimer’s disease. Neurobiol. Aging 35, 2486–2494. doi: 10.1016/j.neurobiolaging.2014.05.019
Mielke, M. M., Haughey, N. J., Han, D., An, Y., Bandaru, V. V. R., Lyketsos, C. G., et al. (2017). The Association Between Plasma Ceramides and Sphingomyelins and Risk of Alzheimer’s Disease Differs by Sex and APOE in the Baltimore Longitudinal Study of Aging. J. Alzheimers Dis. 60, 819–828. doi: 10.3233/JAD-160925
Mirzaian, M., Wisse, P., Ferraz, M. J., Marques, A. R. A., Gabriel, T. L., van Roomen, C., et al. (2016). Accurate quantification of sphingosine-1-phosphate in normal and Fabry disease plasma, cells and tissues by LC-MS/MS with (13)C-encoded natural S1P as internal standard. Clin. Chim. Acta 459, 36–44. doi: 10.1016/j.cca.2016.05.017
Mirzaian, M., Wisse, P., Ferraz, M. J., Marques, A. R. A., Gaspar, P., Oussoren, S. V., et al. (2017). Simultaneous quantitation of sphingoid bases by UPLC-ESI-MS/MS with identical (13)C-encoded internal standards. Clin. Chim. Acta 466, 178–184. doi: 10.1016/j.cca.2017.01.014
Mulder, M., Blokland, A., van den Berg, D. J., Schulten, H., Bakker, A. H., Terwel, D., et al. (2001). Apolipoprotein E protects against neuropathology induced by a high-fat diet and maintains the integrity of the blood-brain barrier during aging. Lab. Invest. 81, 953–960. doi: 10.1038/labinvest.3780307
Mulder, M., Ravid, R., Swaab, D. F., de Kloet, E. R., Haasdijk, E. D., Julk, J., et al. (1998). Reduced levels of cholesterol, phospholipids, and fatty acids in cerebrospinal fluid of Alzheimer disease patients are not related to apolipoprotein E4. Alzheimer Dis. Assoc. Disord. 12, 198–203. doi: 10.1097/00002093-199809000-00012
Mullen, T. D., and Obeid, L. M. (2012). Ceramide and apoptosis: exploring the enigmatic connections between sphingolipid metabolism and programmed cell death. Anticancer Agents Med. Chem. 12, 340–363. doi: 10.2174/187152012800228661
Mullen, T. D., Hannun, Y. A., and Obeid, L. M. (2012). Ceramide synthases at the centre of sphingolipid metabolism and biology. Biochem. J. 441, 789–802. doi: 10.1042/BJ20111626
Oakley, H., Cole, S. L., Logan, S., Maus, E., Shao, P., Craft, J., et al. (2006). Intraneuronal beta-amyloid aggregates, neurodegeneration, and neuron loss in transgenic mice with five familial Alzheimer’s disease mutations: potential factors in amyloid plaque formation. J. Neurosci. 26, 10129–10140. doi: 10.1523/JNEUROSCI.1202-06.2006
Olsen, A. S. B., and Faergeman, N. J. (2017). Sphingolipids: membrane microdomains in brain development, function and neurological diseases. Open Biol. 7:170069. doi: 10.1098/rsob.170069
Park, W. J., and Park, J. W. (2015). The effect of altered sphingolipid acyl chain length on various disease models. Biol. Chem. 396, 693–705. doi: 10.1515/hsz-2014-0310
Pinto, S. N., Silva, L. C., de Almeida, R. F., and Prieto, M. (2008). Membrane domain formation, interdigitation, and morphological alterations induced by the very long chain asymmetric C24:1 ceramide. Biophys. J. 95, 2867–2879. doi: 10.1529/biophysj.108.129858
Prince, M., Bryce, R., Albanese, E., Wimo, A., Ribeiro, W., and Ferri, C. P. (2013). The global prevalence of dementia: a systematic review and metaanalysis. Alzheimers Dement. 9, 63–75e62. doi: 10.1016/j.jalz.2012.11.007
Schafer, S., Wirths, O., Multhaup, G., and Bayer, T. A. (2007). Gender dependent APP processing in a transgenic mouse model of Alzheimer’s disease. J. Neural Transm. 114, 387–394. doi: 10.1007/s00702-006-0580-9
Schwarz, A., and Futerman, A. H. (1997). Distinct roles for ceramide and glucosylceramide at different stages of neuronal growth. J. Neurosci. Offic. J. Soc. Neurosci. 17, 2929–2938. doi: 10.1523/JNEUROSCI.17-09-02929.1997
Seumois, G., Fillet, M., Gillet, L., Faccinetto, C., Desmet, C., Francois, C., et al. (2007). De novo C16- and C24-ceramide generation contributes to spontaneous neutrophil apoptosis. J. Leukoc. Biol. 81, 1477–1486. doi: 10.1189/jlb.0806529
Sharman, M. J., Shui, G., Fernandis, A. Z., Lim, W. L., Berger, T., Hone, E., et al. (2010). Profiling brain and plasma lipids in human APOE epsilon2, epsilon3, and epsilon4 knock-in mice using electrospray ionization mass spectrometry. J. Alzheimers Dis. 20, 105–111. doi: 10.3233/JAD-2010-1348
Sherrington, R., Rogaev, E. I., Liang, Y., Rogaeva, E. A., Levesque, G., Ikeda, M., et al. (1995). Cloning of a gene bearing missense mutations in early-onset familial Alzheimer’s disease. Nature 375, 754–760. doi: 10.1038/375754a0
Strazielle, N., and Ghersi-Egea, J. F. (2013). Physiology of blood-brain interfaces in relation to brain disposition of small compounds and macromolecules. Mol. Pharm. 10, 1473–1491. doi: 10.1021/mp300518e
Strittmatter, W. J., Saunders, A. M., Schmechel, D., Pericak-Vance, M., Enghild, J., Salvesen, G. S., et al. (1993). Apolipoprotein E: high-avidity binding to beta-amyloid and increased frequency of type 4 allele in late-onset familial Alzheimer disease. Proc. Natl. Acad. Sci. U S A. 90, 1977–1981. doi: 10.1073/pnas.90.5.1977
Tai, L. M., Balu, D., Avila-Munoz, E., Abdullah, L., Thomas, R., Collins, N., et al. (2017). EFAD transgenic mice as a human APOE relevant preclinical model of Alzheimer’s disease. J. Lipid Res. 58, 1733–1755. doi: 10.1194/jlr.R076315
Tai, L. M., Youmans, K. L., Jungbauer, L., Yu, C., and Ladu, M. J. (2011). Introducing Human APOE into Abeta Transgenic Mouse Models. Int. J. Alzheimers Dis. 2011:810981. doi: 10.4061/2011/810981
Van Brocklyn, J. R., and Williams, J. B. (2012). The control of the balance between ceramide and sphingosine-1-phosphate by sphingosine kinase: oxidative stress and the seesaw of cell survival and death. Comp. Biochem. Physiol. B Biochem. Mol. Biol. 163, 26–36. doi: 10.1016/j.cbpb.2012.05.006
van Doorn, R., Nijland, P. G., Dekker, N., Witte, M. E., Lopes-Pinheiro, M. A., van het Hof, B., et al. (2012). Fingolimod attenuates ceramide-induced blood-brain barrier dysfunction in multiple sclerosis by targeting reactive astrocytes. Acta Neuropathol. 124, 397–410. doi: 10.1007/s00401-012-1014-4
van Echten-Deckert, G., Hagen-Euteneuer, N., Karaca, I., and Walter, J. (2014). Sphingosine-1-phosphate: boon and bane for the brain. Cell Physiol. Biochem. 34, 148–157. doi: 10.1159/000362991
Verghese, P. B., Castellano, J. M., Garai, K., Wang, Y., Jiang, H., Shah, A., et al. (2013). ApoE influences amyloid-beta (Abeta) clearance despite minimal apoE/Abeta association in physiological conditions. Proc. Natl. Acad. Sci. U S A. 110, E1807–E1816. doi: 10.1073/pnas.1220484110
Vozella, V., Basit, A., Misto, A., and Piomelli, D. (2017). Age-dependent changes in nervonic acid-containing sphingolipids in mouse hippocampus. Biochim. Biophys. Acta Mol. Cell Biol. Lipids 1862, 1502–1511. doi: 10.1016/j.bbalip.2017.08.008
Vozella, V., Basit, A., Piras, F., Realini, N., Armirotti, A., Bossu, P., et al. (2019). Elevated plasma ceramide levels in post-menopausal women: a cross-sectional study. Aging 11, 73–88. doi: 10.18632/aging.101719
Wheeler, D., Knapp, E., Bandaru, V. V., Wang, Y., Knorr, D., Poirier, C., et al. (2009). Tumor necrosis factor-alpha-induced neutral sphingomyelinase-2 modulates synaptic plasticity by controlling the membrane insertion of NMDA receptors. J. Neurochem. 109, 1237–1249. doi: 10.1111/j.1471-4159.2009.06038.x
Youmans, K. L., Tai, L. M., Kanekiyo, T., Stine, W. B. Jr., Michon, S. C., Nwabuisi-Heath, E., et al. (2012a). Intraneuronal Abeta detection in 5xFAD mice by a new Abeta-specific antibody. Mol. Neurodegener. 7:8. doi: 10.1186/1750-1326-7-8
Youmans, K. L., Tai, L. M., Nwabuisi-Heath, E., Jungbauer, L., Kanekiyo, T., Gan, M., et al. (2012b). APOE4-specific changes in Abeta accumulation in a new transgenic mouse model of Alzheimer disease. J. Biol. Chem. 287, 41774–41786. doi: 10.1074/jbc.M112.407957
Zhang, L., and Hong, H. (2015). Genomic Discoveries and Personalized Medicine in Neurological Diseases. Pharmaceutics 7, 542–553. doi: 10.3390/pharmaceutics7040542
Keywords: ceramide, S1P, apolipoprotein E4, Alzheimer’s disease, aging, sex differences
Citation: den Hoedt S, Crivelli SM, Leijten FPJ, Losen M, Stevens JAA, Mané-Damas M, de Vries HE, Walter J, Mirzaian M, Sijbrands EJG, Aerts JMFG, Verhoeven AJM, Martinez-Martinez P and Mulder MT (2021) Effects of Sex, Age, and Apolipoprotein E Genotype on Brain Ceramides and Sphingosine-1-Phosphate in Alzheimer’s Disease and Control Mice. Front. Aging Neurosci. 13:765252. doi: 10.3389/fnagi.2021.765252
Received: 26 August 2021; Accepted: 29 September 2021;
Published: 27 October 2021.
Edited by:
Kristine Freude, University of Copenhagen, DenmarkReviewed by:
Malin Wennström, Lund University, SwedenRobert Piotr Strosznajder, Mossakowski Medical Research Centre, Polish Academy of Sciences (PAN), Poland
Copyright © 2021 den Hoedt, Crivelli, Leijten, Losen, Stevens, Mané-Damas, de Vries, Walter, Mirzaian, Sijbrands, Aerts, Verhoeven, Martinez-Martinez and Mulder. This is an open-access article distributed under the terms of the Creative Commons Attribution License (CC BY). The use, distribution or reproduction in other forums is permitted, provided the original author(s) and the copyright owner(s) are credited and that the original publication in this journal is cited, in accordance with accepted academic practice. No use, distribution or reproduction is permitted which does not comply with these terms.
*Correspondence: Pilar Martinez-Martinez, p.martinez@maastrichtuniversity.nl; Monique T. Mulder, m.t.mulder@erasmusmc.nl
†These authors share first authorship
‡These authors share senior authorship