- 1Centre of Excellence for Alzheimer’s Disease Research & Care, School of Medical and Health Sciences, Edith Cowan University, Joondalup, WA, Australia
- 2Cooperative Research Centre for Mental Health, Carlton, VIC, Australia
- 3School of Biomedical Sciences and Pharmacy, Faculty of Health and Medicine, University of Newcastle, Callaghan, NSW, Australia
- 4Riddet Institute, Massey University, Palmerston North, New Zealand
- 5School of Pharmacy and Biomedical Sciences, Faculty of Health Sciences, Curtin Health Innovation Research Institute, Curtin University, Bentley, WA, Australia
- 6Australian Alzheimer’s Research Foundation, Ralph and Patricia Sarich Neuroscience Research Institute, Nedlands, WA, Australia
- 7Department of Biomedical Sciences, Macquarie University, Sydney, NSW, Australia
Alzheimer’s disease (AD) is a devastating neurodegenerative disorder and the most common form of dementia worldwide. The classical AD brain is characterized by extracellular deposition of amyloid-β (Aβ) protein aggregates as senile plaques and intracellular neurofibrillary tangles (NFTs), composed of hyper-phosphorylated forms of the microtubule-associated protein Tau. There has been limited success in clinical trials for some proposed therapies for AD, so attention has been drawn toward using alternative approaches, including prevention strategies. As a result, nutraceuticals have become attractive compounds for their potential neuroprotective capabilities. The objective of the present study was to derive a synergistic nutraceutical combination in vitro that may act as a potential preventative therapy for AD. The compounds of interest were docosahexaenoic acid (DHA), luteolin (LUT), and urolithin A (UA). The cell viability and cytotoxicity assays MTS and LDH were used to evaluate the compounds individually and in two-compound combinations, for their ability to inhibit Aβ1–42-induced toxicity in human neuroblastoma BE(2)-M17 cells. The LDH-derived% protection values were used in the program CompuSyn v.1.0 to calculate the combination index (CI) of the two-compound combinations. The software-predicted potentially synergistic (CI < 1) two-compound combinations were validated using CellTiter Glo assay. Finally, a three-compound combination was predicted (D5L5U5) and shown to be the most effective at inhibiting Aβ1–42-induced toxicity. The synergistic combination, D5L5U5 warrants further research for its mechanism of action; however, it can serve as a basis to develop an advanced functional food for the prevention or co-treatment of AD.
Introduction
Plants, the immobile life on earth, have the inherent ability to synthesize defensive secondary metabolites, commonly known as phytochemicals, to withstand attacks by different organisms such as pathogens, insects, and herbivores. These phytochemicals have proven bioactivity through modulating molecular targets in living beings (Efferth and Koch, 2011). These bioactive phytochemicals are used in traditional medicine in China and Sri Lanka, and Ayurveda in India. These medicine systems use herbal mixtures consisting of many different herbs to treat diseases (Thomas and Egon, 2011).
The unraveling of complex disease mechanisms in modern medicine by technological advancement has immensely contributed to a greater understanding of drug interactions and usage of drug combinations in therapeutic regimes. In combination drug therapies, the simultaneous action of drugs in low doses increases therapeutic efficacy and decreases toxicity effects and drug resistance (Sun et al., 2016). Combination drug therapies are widely researched in treating diseases such as cancer (De Kok et al., 2008), human immunodeficiency virus (HIV) infection (Moreno et al., 2019), and many other ailments. Furthermore, combining natural compounds is popularizing in dealing with medical conditions where there is a shortage of discovery and approval of new drugs, and the existing monotherapies have shown limited therapeutic efficacy (Patti et al., 2017; Santana-Gálvez et al., 2019).
Alzheimer’s disease (AD) is a progressive neurodegenerative disorder and is the second major cause of death in Australia. The classical AD brain is characterized by extracellular deposition of amyloid-β (Aβ) protein aggregates as senile plaques and intracellular neurofibrillary tangles (NFTs), composed of hyper-phosphorylated forms of the microtubule-associated protein Tau. Amyloid beta peptides are formed by the normal metabolic processing of amyloid precursor protein (APP). The predominant (90%) Aβ peptides are Aβ1–40 and Aβ1–42, respectively, with the latter being the most toxic (Selkoe, 2001; Murphy and Levine, 2010; Tiwari and Kepp, 2016).
It has been reported that by February 2020, there were 121 drugs studied in 136 AD therapeutic trials (Cummings et al., 2020). However, considering the past decade, many clinical trials have failed outright while the efficacy and effect size have been problematic in the ones that have indicated a positive outcome (Banik et al., 2015). There has been no new drug approved within the past 16 years until the controversial approval of the drug Aducanumab recently in 2021 (Rabinovici, 2021).
Due to the toxicity associated with the use of currently available drugs and their limited therapeutic effectiveness, the purposed drugs for AD are being repositioned as combinations (Cummings et al., 2019; Kabir et al., 2020). Considering the multifactorial nature of AD, combinations of therapeutic agents may be effective than monotherapies. One study reported that a drug combination of two approved drugs, acamprosate and baclofen synergistically protected rat cortical neurons and human brain-derived microvascular endothelial cells against Aβ oligomer-induced toxicity (Chumakov et al., 2015). Furthermore, this combination has alleviated cognitive deficits in an acute Aβ25–35 peptide injection mouse model and a mutant APP transgenic mouse model (Chumakov et al., 2015). Many studies and clinical trials have been conducted for AD drug combinations, to name a few, the N-methyl-D-aspartate (NMDA) receptor antagonist Memantine with various Acetyl Cholinesterase inhibitors such as Memantine and Rivastigmine (Dantoine et al., 2006; Riepe et al., 2007), Memantine, and Donepezil (Tariot et al., 2004; Cummings et al., 2006) and Memantine and Galantamine (Simoni et al., 2012).
Prevention of AD has become an important consideration, particularly since disease-modifying treatment trials have proven unsuccessful. As AD is a complex multifactorial disorder, there may also be multiple ways to prevent or delay the onset of AD (Galvin, 2017). It suggests that prevention studies focusing on risk reduction and lifestyle modification by diet and exercise may be an alternative approach offering additional benefits. In the modulation of lifestyle, diet plays a major role. The Mediterranean diet (MeDi) plays an important role to reduce the risk for AD (Scarmeas et al., 2006; Panza et al., 2018). MeDi is characterized by a high intake of vegetables, fruits, unsaturated fatty acids (in the form of olive oil), fish, a low-to-moderate intake of dairy products such as cheese or yogurt, a low intake of meat, and poultry and a regular but moderate amount of red wine (Scarmeas et al., 2006). These vital food items in a typical MeDi are rich in bioactive components that are reported as potentially beneficial for cognitive performance in AD (Cremonini et al., 2019; Grodzicki and Dziendzikowska, 2020).
One rich source of polyphenols is pomegranate, which possesses many polyphenolic compounds such as ellagitannins (ETs) and flavonoids (Sreekumar et al., 2014). Punicalagin is the most abundant ET in pomegranate juice with a very low bioavailability (Cerda et al., 2003). In the lower digestive tract, punicalagins are converted by the gut microbiota into urolithin A (UA), which has a relatively higher bioavailability (Seeram et al., 2006; Espin et al., 2013). According to Hartman et al. (2006), mice treated with pomegranate juice had significantly less (∼50%) soluble Aβ42 and amyloid deposition in the hippocampus as compared to control mice (Hartman et al., 2006). However, the anti-AD effects of pomegranate are due to UA (Yuan et al., 2016; Gong et al., 2019). Luteolin (LUT) is a prominent flavone compound in pomegranate peel (Van Elswijk et al., 2004; Chaudhari et al., 2014; Liu et al., 2017). It shows potent anti-inflammatory and antioxidant activities (Xia et al., 2014). It also inhibits BACE1 by suppressing the BACE1 promoter by NF-κB signaling (Zheng et al., 2015). Moreover, LUT has been reported to reduce zinc-induced Tau hyperphosphorylation in SH-SY5Y Cells (Zhou et al., 2012). Luteolin has also been shown to ameliorate neurotoxicity in an Aβ toxicity model that used Aβ25–35 peptide in murine cortical neurons (Choi et al., 2014). Overall, there are only a limited number of studies carried out on the activity of these pomegranate-related polyphenols on the inhibition of Aβ1–42 induced toxicity.
Omega-3 polyunsaturated fatty acids including docosahexaenoic acid (DHA) naturally occur in marine food sources such as fish and algae (Tocher, 2015; Peltomaa et al., 2017). An in vivo experiment carried out in mouse expressing human APP K670N-M671L (APPsw) transgenic mouse model (Tg2576) has shown that DHA treatment lowers Aβ40 and Aβ42 levels and Aβ plaque burden (Lim et al., 2005). Some in vitro experiments demonstrated that DHA decreases the BACE1 and γ-secretase activity and increases the α-secretase activity. It has been reported that DHA effectively reduced Aβ release by driving the amyloidogenic processing of APP toward non-amyloidogenic processing (Grimm et al., 2011). An in vitro study indicated that DHA reduced soluble Aβ oligomer levels and further inhibited formation of Aβ1–42 fibrils (Hossain et al., 2009). Furthermore, another study showed that DHA reduced formation of Aβ oligomers and fibrils in the cerebral cortex of Aβ-infused rats (Hashimoto et al., 2009). However, the few studies that have investigated DHA on Aβ1–42 induced toxicity need confirmation by a more thorough investigation.
Some reports have investigated the combined effect of multi-targeting nutraceutical compounds in AD in vitro models (Espargaró et al., 2017). It has recently been shown in Tg2576 transgenic mice that a combination of food-derived compounds, EGCG, DHA, and α-lipoic acid exerted potent anti-inflammatory and neuroprotective effects (Sharman et al., 2019). However, similar studies targeting bioactive compound combinations against AD are still limited in the literature.
The objective of the present study was to investigate the compounds, DHA, LUT, and UA (Figure 1) in vitro for any nutraceutical combinations potentially effective against AD. The compounds were initially screened for their activity to inhibit Aβ1–42-induced toxicity and were subsequently used to determine synergistic combinations in vitro that may be more potent in action against Aβ1–42 compared to single compounds. For drug combinations, quantifying synergism and antagonism through CI calculations was performed by the third-generation computer software, “CompuSyn” written by Ting-Chao Chou and Nick Martin (MIT, MA, United States) in 2005 (ComboSyn, Inc., MA, United States).
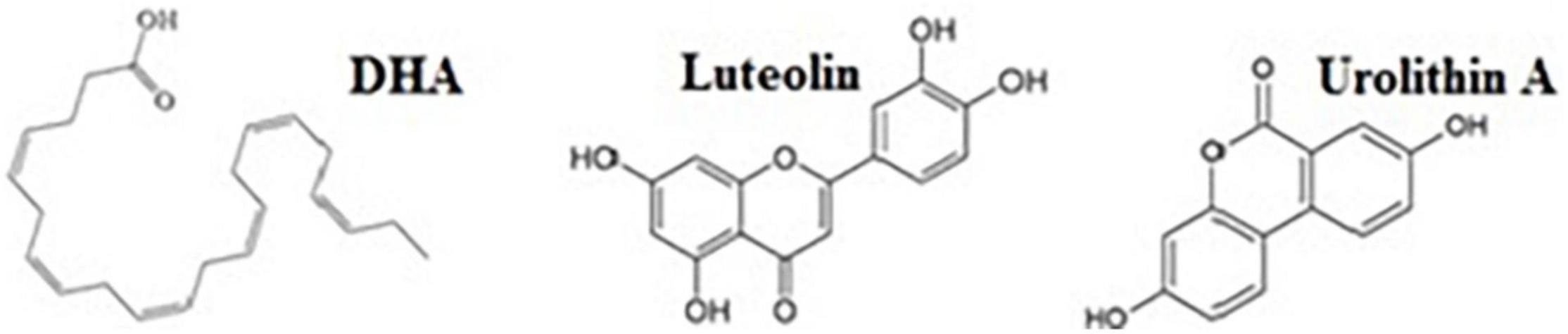
Figure 1. The compounds of interest. The compounds investigated in the present study were, omega-3 polyunsaturated fatty acid, docosahexaenoic acid (DHA) and pomegranate-derived compounds: luteolin (LUT), urolithin A (UA).
Materials and Methods
Materials
cis-4,7,10,13,16,19-Docosahexaenoic acid (DHA: D2534), LUT (L9283), UA (SML1791), and dimethyl sulfoxide (DMSO) were obtained from Sigma Aldrich, United States. BE(2)-M17 cells (ATCC® CRL2267™) were purchased from American Type Cell Culture Collection (ATCC, Manassas, VA, United States). All cell culture reagents including Dulbecco’s Modified Eagle Medium (DMEM), Ham’s F12 medium, Hank’s balanced salt solution (HBSS), fetal calf serum (FCS) and Trypsin-EDTA (0.5%) were purchased from GIBCO by Life Technologies (United States). Human Aβ1–42 peptides were synthesized, purified and characterized by high pressure liquid chromatography (HPLC) and mass spectrometry (MS) by The ERI Amyloid Laboratories LLC, United States. Anhydrous DMSO was purchased from Molecular Probes by Life Technologies (United States). CellTiter 96® AQueous One Solution Cell Proliferation assay (MTS: 3-(4,5-Dimethylthiazol-2-yl)-5-(3-carboxymethoxyphenyl)-2-(4-sulfophenyl)-2H-tetrazolium) and CytoTox-ONE™ Homogeneous Membrane Integrity assay kits (Lactate dehydrogenase: LDH assay), and CellTiter-Glo luminiscent cell viability assay kits were purchased from Promega (Madison, WI, United States).
Cell Culture
Human neuroblastoma BE(2)-M17 cells were maintained in T75 culture flasks containing 15 mL of DMEM/F12 (1:1 ratio) growth media supplemented with 10% (v/v) FCS and placed in a humidified incubator with 5% CO2/95% air at 37°C. Upon reaching about 80% confluency, the cells were sub-cultured on to fresh cell culture flasks. For all cell culture experiments, passage number did not exceed 30.
Preparation of Oligomeric Aβ1–42
The oligomeric Aβ1–42 was prepared according to the method of Stine et al. (2011) with some modifications (Stine et al., 2011). The detailed method used for Aβ1–42 preparation is explained in our previous work (Jayatunga et al., 2021).
Aβ1–42 Induced Toxicity/Lactate Dehydrogenase Assay
For Aβ1–42 toxicity experiments, cells were plated in 96-well tissue culture microplates at a density of 1.5 × 104 cells/well and incubated for 24 hours. The cell culture media was then replaced with treatment media (1% FCS) and the cells were pre-treated with different concentrations of the compounds, DHA, LUT, and UA (5 μM to 40 μM) for 24 hours. The cells were then treated with oligomeric 20 μM Aβ1–42 with appropriate controls (vehicle-treatment: negative control; Aβ1–42–treatement: positive control). The microplates were incubated in the humidified incubator with 5% CO2/95% air for 72 h at 37°C. The percentage LDH release for all treatments were determined using LDH assay. LDH release results of Aβ1–42-induced toxicity assays for the compounds DHA, LUT, and UA.
The% LDH release results of Aβ1–42–induced toxicity assays for the compounds DHA, LUT, and UA were normalized according to the method used by Chumakov et al. (2015). As shown below, the vehicle and the Aβ1–42 added treatments were considered as 1 and 0, respectively (Chumakov et al., 2015). The coded data were considered as the fractions affected (Fa) and used along with their respective concentrations (5 to 40 μM) as input for the computer program CompuSyn v.1.0.
Calculation of Fa - method adapted from Chumakov et al. (2015).
Preparation of Compound Solutions
The compounds DHA, LUT, and UA were dissolved in dimethylsulfoxide (DMSO) and 10 mM stock solutions were prepared from each. The stock solutions were frozen and working solutions were prepared using treatment media (DMEM/F12 supplemented with 1% FCS). Control solutions were used for all compounds at all concentrations.
Combination Studies
MTS Assay
Alternatively, all possible two-compound combinations (n = 75) for the compounds DHA, LUT, and UA (for the concentration range of 5 μM to 40 μM) were used for screening the combinations with the greatest efficacy to inhibit Aβ1–42–induced toxicity. For that, BE(2)-M17 cells were maintained in DMEM/F12 medium supplemented with 10% FCS, in 5% CO2 at 37°C. For Aβ1–42 toxicity experiments, cells were plated in 96-well tissue culture microplates at a density of 1.5 × 104 cells/well and incubated for 24 h. After replacing the media with treatment media, the cells were pre-treated with each two-compound combinations for 24 h. The cells were then treated with oligomeric 20 μM Aβ including controls for vehicle (negative control) and Aβ1–42 (positive control). The microplates were incubated in a humidified incubator with 5% CO2/95% air for 72 h at 37°C. Percentage cell viability for each combination was determined (N = 4) using the MTS assay.
Determination of Best Combinations by LDH Assay
The combinations with higher% cell viabilities were re-screened with the LDH assay. Percentage protection was calculated from the% LDH release for all compound combinations. The experiments consisted of all 3 compounds combining with each other at 5, 10, 20, and 40 μM concentrations. The coded data were considered as data of Fa and used along with their respective concentrations (doses) as input in the computer program CompuSyn v.1.0 for calculating CI values. Thirteen synergistic combinations were recognized by the CI values less than 1.
Validation of the Synergistic Two-Compound Combinations Using CellTiter Glo Assay
The synergistic combinations were further validated and confirmed by cellular ATP levels using the CelltiterGlo assay. Briefly, the BE(2)-M17 cells in DMEM/F12 medium supplemented with 10% FCS were seeded in 96-well tissue culture microplates at a density of 1.5 × 104 cells/well and were incubated at 37°C for 24 h. After the respective treatment of compounds and incubation at 37°C, cellular ATP levels were measured using CellTiter Glo ATP detection kit as per the manufacturer’s instructions (Promega). Briefly, cells were placed at RT for 30 min and then lysed by adding 100 μL of ATP-releasing reagent. The lysates were incubated with the luciferin substrate and luciferase enzyme in the dark for 10 min to stabilize the luminescence signal. The luminescence (RLU) was measured using a Perkin Elmer EnSpire multi-mode plate reader.
Prediction of a Potentially Synergistic Three-Compound Combination and Validation
Based on the validation data for the two-compound combinations, a new three-compound combination was predicted. This combination was repeated and confirmed as efficiently inhibiting Aβ1–42-induced toxicity using MTS, LDH, and CellTiter Glo assays.
Statistical Analysis
All results were expressed as mean ± standard deviation (SD) from four (N = 4) independent experiments. Statistical significance was determined by one-way ANOVA and Tukey’s post hoc test in SPSS v25. Significance was defined as P < 0.05.
Results
Thirteen Synergistic Two-Compound Combinations Derived in silico
There were thirteen two-compound combinations that were determined to be synergistic based on their CI values, being less than 1 (Table 1). Table 1 summarizes the thirteen synergistic combinations (numbered as combinations 1-13) that belong to UA-DHA, LUT-DHA, and UA-LUT. The inferences on synergy with subtle definitions were based on the work of Chou and Talalay (1984).
Two Best Synergistic Two-Compound Combinations Based on Validations for Relative ATP Levels
Based on the validation of results for all 13 synergistic combinations, the combination 2 (D5U5: DHA 5 μM and UA 5 μM) and 11 (L5U5: LUT 5 μM and UA 5 μM) were selected as the best combinations based on two reasons. First, their significantly higher relative ATP levels compared to both components in the combinations independently. Second, they both had the lowest possible concentrations used in this study. Relative ATP level of the cells was reduced to 51.9 + 7.0% of the control treatment after 72 h exposure to 20 μM Aβ1–42 treatment. Pre-treatment with the combination 2 (D5U5) increased the cellular ATP levels to 71.3 + 7.9% (P < 0.001) (Figure 2).
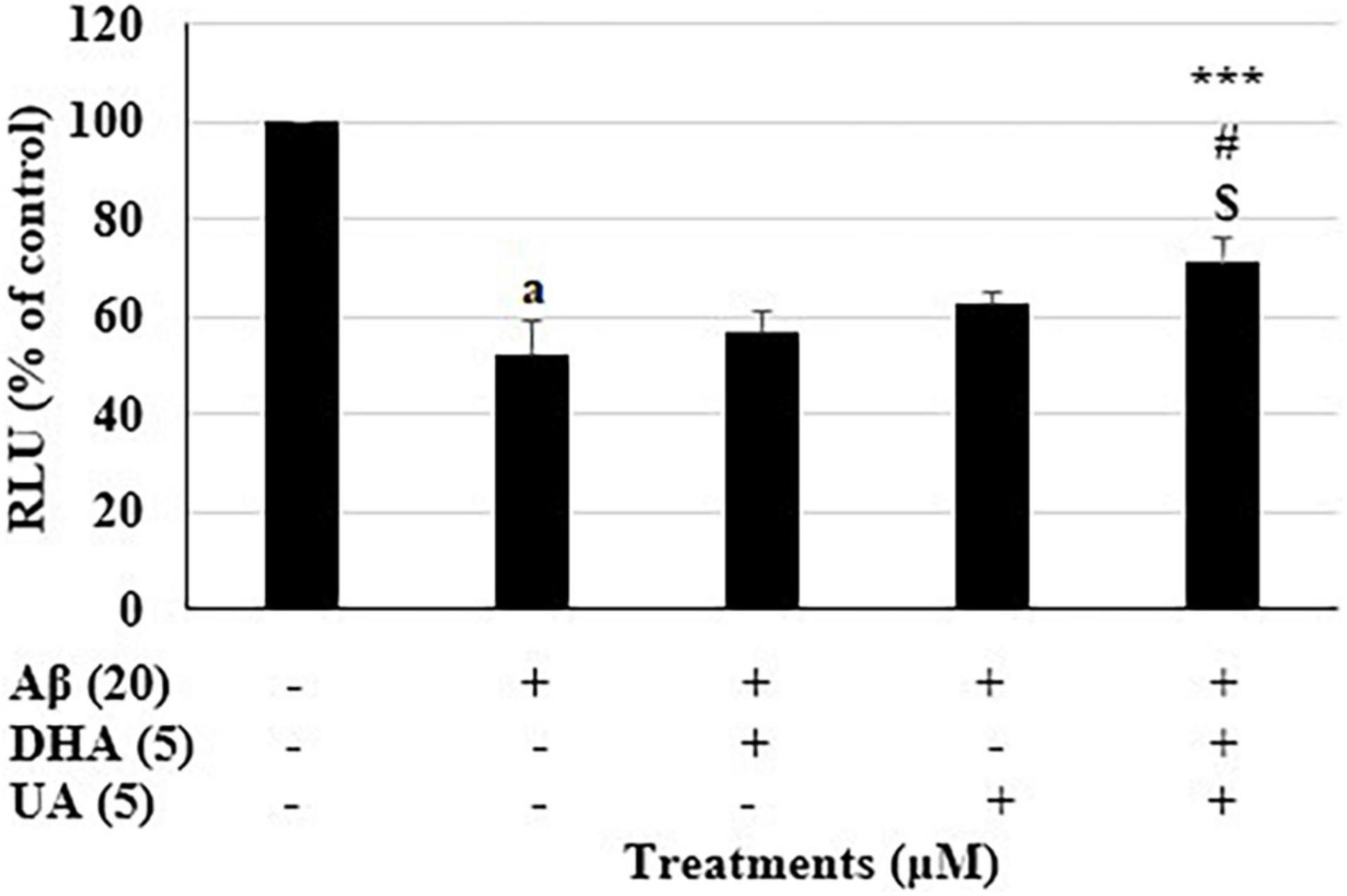
Figure 2. Relative ATP levels for the combination 2 (D5U5) and its components. ATP levels for the combination 2 (D5U5) and its constituents were determined using CellTiter Glo assay. Data are expressed as mean ± SD from four (N = 4) independent experiments. Differences are significant at aP < 0.001 vs. vehicle control, ***P < 0.001 vs. Aβ1–42-treated control, #P < 0.05 vs. DHA 5 μM and $P < 0.05 vs. UA 5 μM.
Similarly, pre-treatment with the combination 11 (L5U5) increased the ATP levels to 99.6 ± 4.2% (P < 0.001) (Figure 3). In either case, the combinations gave significantly higher ATP levels against the Aβ1–42-treated controls than the component compound concentrations (DHA, LUT, and UA 5 μM each). These results suggest that pre-treatment with these combinations effectively attenuated Aβ1–42-induced toxicity.
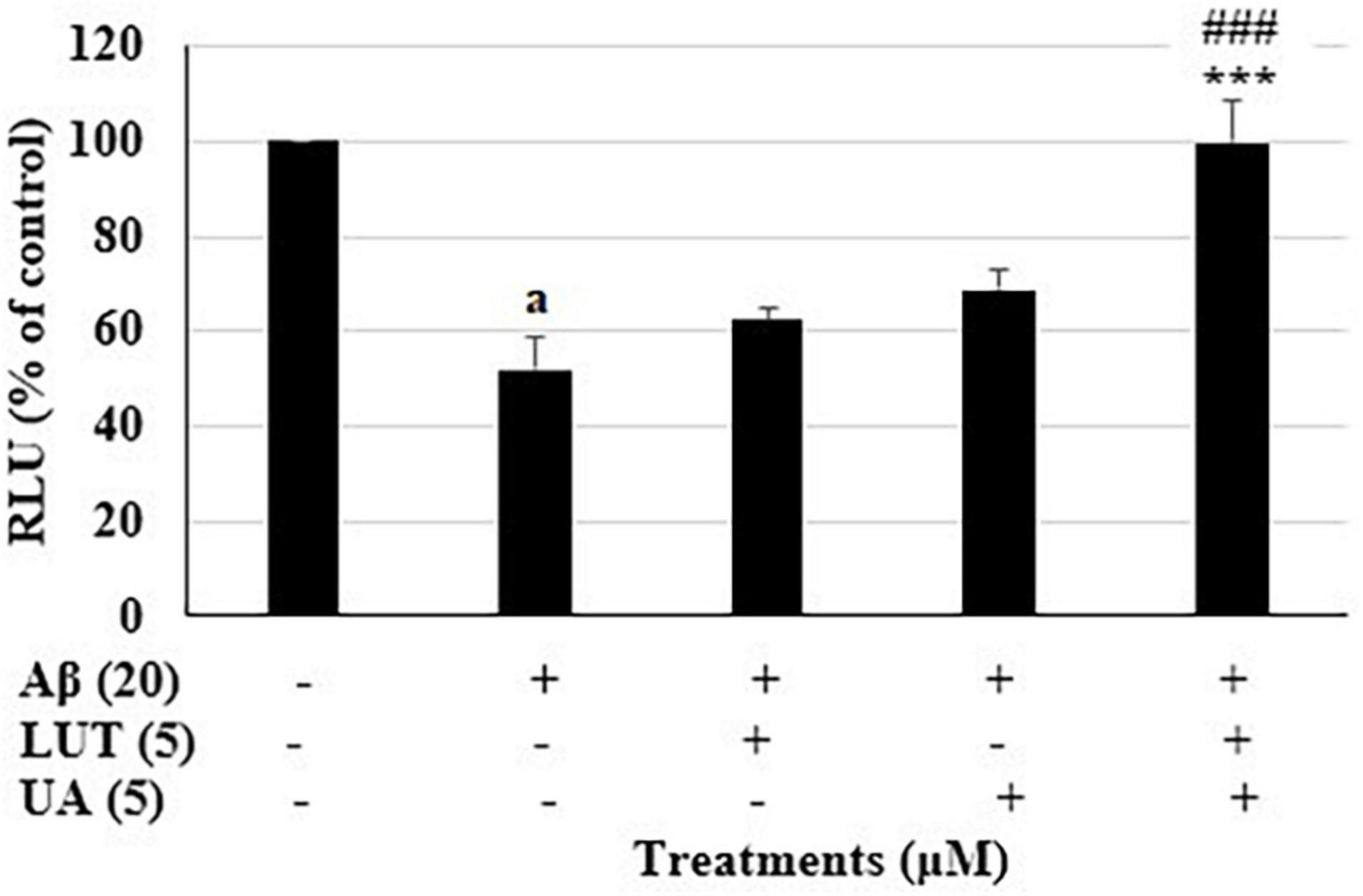
Figure 3. Relative ATP levels for the combination 11 (L5U5) and its components. ATP levels for the combination 11 (L5U5) and its constituents were determined using CellTiter Glo assay. Data are expressed as mean ± SD from four (N = 4) independent experiments. Differences are significant at aP < 0.001 vs vehicle control, ***P < 0.001 vs. Aβ1–42-treated control, ###P < 0.001 vs. LUT 5 μM and UA 5 μM.
Prediction and Validation of a New Three-Compound Combination-D5L5U5 (DHA 5 μM, LUT 5 μM, UA 5 μM)
Based on the two best combinations identified, namely 2 and 11, a new combination was predicted. This composed of all the three compounds, DHA, LUT, and UA, each at a concentration of 5 μM and namely, D5L5U5. The predicted three-compound combination, D5L5U5 was analyzed for its ability to inhibit Aβ1–42-induced toxicity using MTS and LDH assays. The analysis of LDH results by the program CompuSyn v.1.0 determined the three-compound combination, D5L5U5 as synergistic with a CI value of 0.01 (Table 2). Percentage cell viability of BE(2)-M17 cells was decreased to 46.0 ± 3.7% of control (P < 0.001) after 72 h of 20 μM Aβ1–42 treatment, while pre-treatment with D5L5U5 improved the cell viability to 103.6 ± 8.7% (P < 0.001) (Figure 4A). Additionally, 20 μM Aβ1–42 treatment increased the release of LDH in the cells from 7.39 ± 0.04% (vehicle-treated cells) to 25.4 ± 0.5% (Aβ1–42-treated cells) (P < 0.001) and the D5L5U5 pre-treatment significantly reduced the LDH release to 7.3 ± 1.4% (P < 0.001) Figure 4B. Cells after treating with D5L5U5 showed an intact morphology with visually reduced toxic effects and increased proliferation compared to Aβ1–42-treated cells (Figure 5). These results together indicated that pre-treatment with the three-compound combination, D5L5U5 attenuated Aβ1–42-induced toxicity very effectively.

Table 2. Predicted three-compound combination D5L5U5 and its combination index derived by CompySyn v. 1.0.
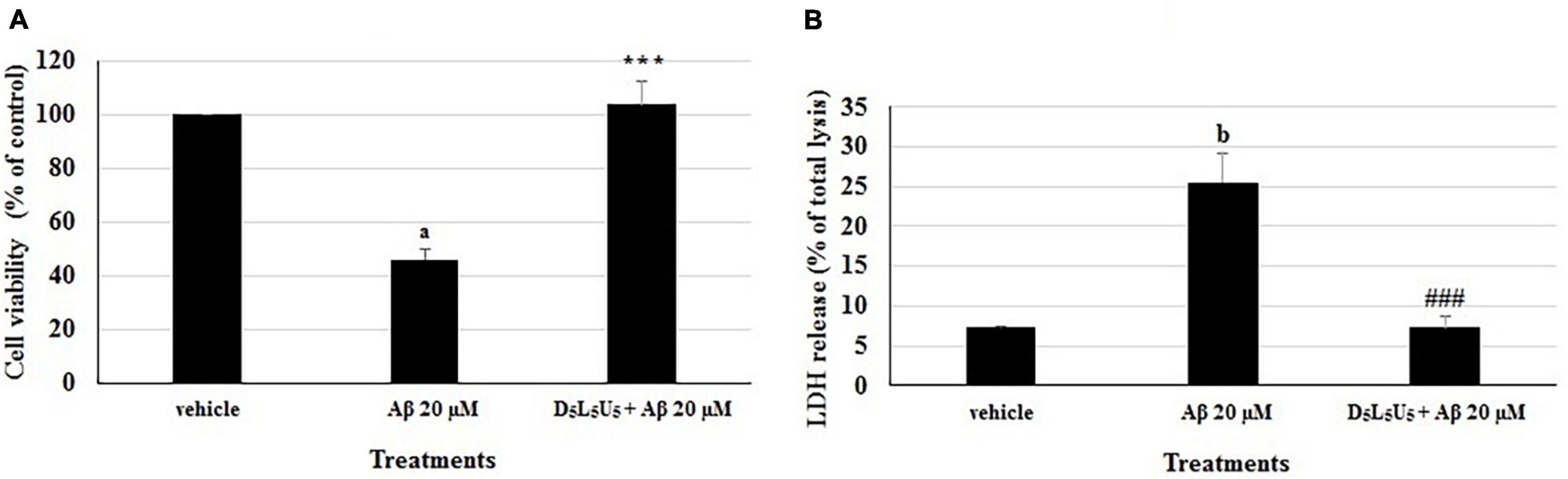
Figure 4. The effect of D5L5U5 on Aβ1–42-induced toxicity. (A) % cell viability (B) % LDH release determined from MTS and LDH assays, respectively, with pre-treatment of D5L5U5 in BE(2)-M17 cells for 24 h followed by incubation with 20 μM Aβ1–42 for 72 h at 37°C. Data are expressed as mean ± SD from four (N = 4) independent experiments. Differences are significant at a,bP < 0.001 vs vehicle control, ***P < 0.001, ###P < 0.001 vs. Aβ1–42-treated control.
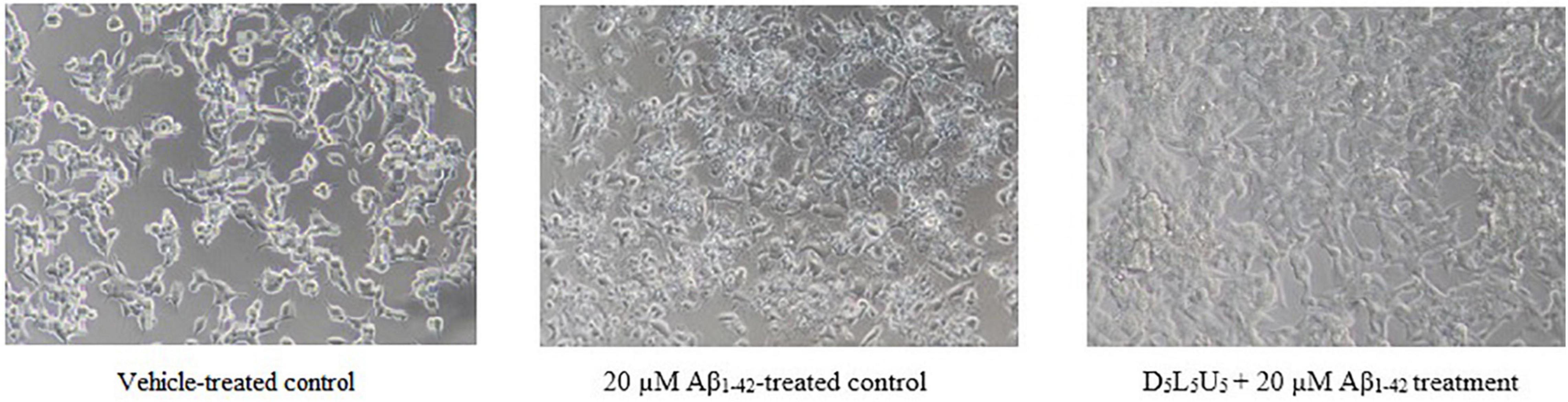
Figure 5. BE(2)-M17 cell morphology for vehicle-treated control, Aβ1–42-treated control and the D5L5U5 treatment. Comparison of cells for 20 μM Aβ1–42-treatment with and without D5L5U5 pre-treatment. The BE(2)-M17 cells are pre-treated with D5L5U5 followed by exposure of 20 μM Aβ1–42 for 72 h. Cell morphology was imaged using a Nikon phase-contrast microscope (X40).
Validation of the combinations 2 and 11 and the three-compound combination along with their single components. ATP level of BE(2)-M17 cells was decreased to 51.9 ± 7.0% of control (P < 0.001) after 72 h of 20 μM Aβ1–42 treatment. Pre-treatment with the combination 2 (D5U5) increased the cellular ATP levels to 71.3 ± 7.9% (P < 0.001)while the combination 11 (L5U5) increased the ATP levels to 99.6 ± 4.2% which is a significantly increased ATP level (P < 0.001) compared to the combination 2 (D5U5). However, pre-treatment with the three-compound combination (D5L5U5) resulted in the highest most ATP levels which amounted to 110.8 ± 4.2%. This ATP level is significantly higher compared to that of both combination 2 (D5U5) (P < 0.001) and 11 (L5U5) (P = 0.001) (Figure 6). These results reflect the previous data that the three-compound combination, D5L5U5 attenuates Aβ1–42-induced toxicity better than its two-compound combination counterparts, D5U5 and L5U5.
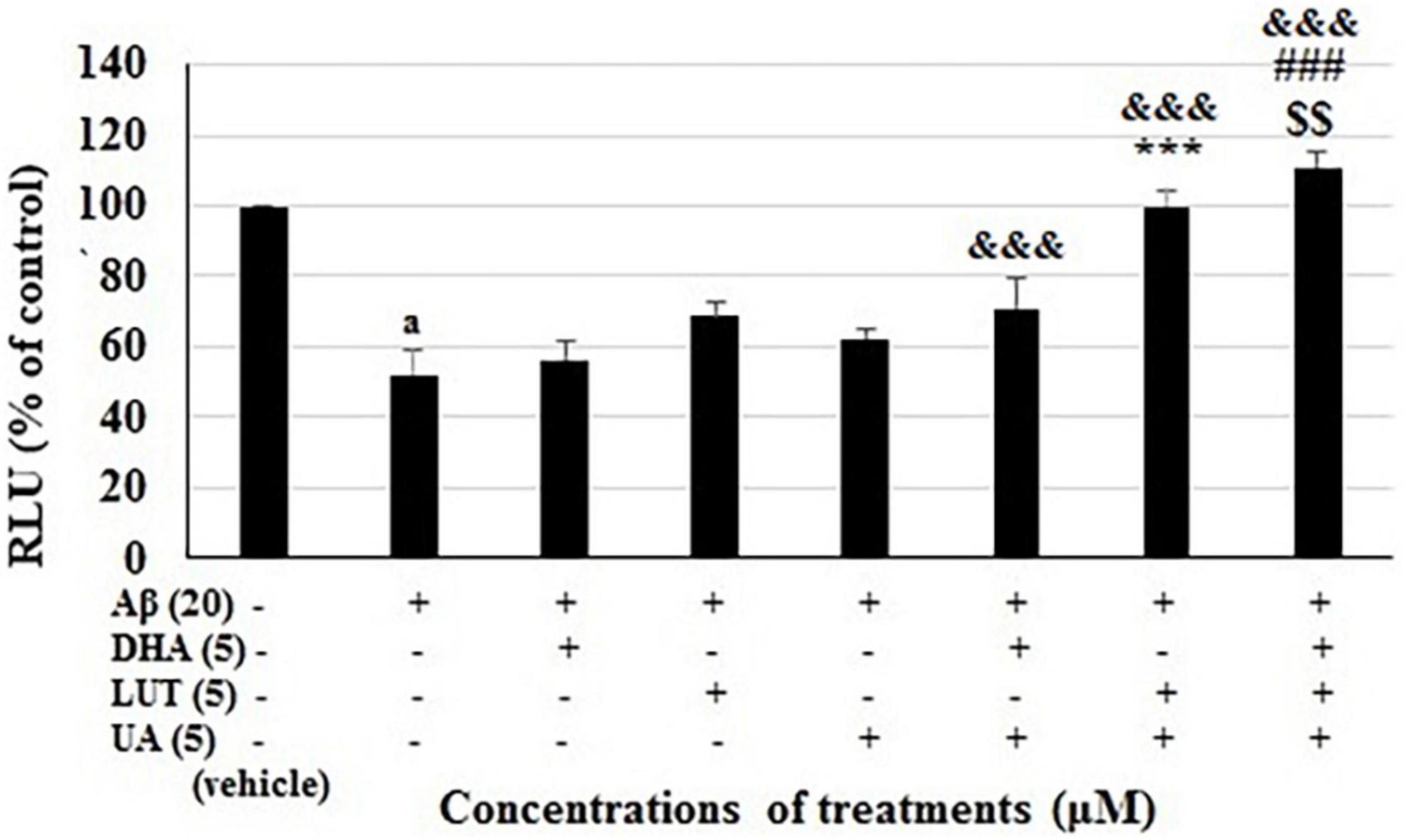
Figure 6. Comparison of the two-compound combinations D5U5, L5U5 and the three-compound combination D5L5U5. ATP levels for the D5U5, L5U5, D5L5U5 and their single components were determined using CellTiter Glo assay. Data are expressed as mean ± SD from four (N = 4) independent experiments. Differences are significant at aP < 0.001 vs. vehicle control, &&&P < 0.001 vs. Aβ1–42-treated control, ***P < 0.001, ###P < 0.001 vs. D5U5 and $$P = 0.001 vs. L5U5.
Discussion
Effective alternate approaches to AD drug development are critically needed as most of all clinical drug immunotherapy trials have failed to date (Anderson et al., 2017; Mehta et al., 2017). Thus, food-derived compounds warrant investigation being potential therapeutic agents (Thaipisuttikul and Galvin, 2012; Lange et al., 2019; Peng et al., 2021). These emerging alternative strategies using natural compounds hold promise for early intervention by targeting the prodromal phase of the disease (Lange et al., 2019). Considering the complexity and the multi-faceted nature of AD neuropathology, a combination of multiple therapeutic targets that can intervene several pathophysiological pathways is preferred. An advantage of combination therapy is where there is a disparity among the drugs of interest. For instance, if one drug has a desirable profile and the other gives undesirable side-effects at a selected dose, it may be possible to combine the two drugs by using different combination ratios, in obtaining a synergistic outcome (Chou, 2006, 2010).
In vitro studies are important as a starting point for drug combination studies. Even though in vitro and in vivo drug combination analyses follow the same principles, animal studies are more expensive, time consuming and often subjected to more variability of data. Although the latter is an essential next step in the evaluation process, initial investigations under in vitro conditions are a cost reduction and thereby, is the logical first step. Furthermore, in vitro studies are more flexible in liability considerations and in using death as an endpoint of toxicity (Chou, 2010). It is well known that in vitro data may not always predict in vivo results, and in vivo animal data may not always predict clinical results (Van Norman, 2020). However, drug combination studies strictly need an initial in vitro component as analyzing the effects of sub-optimal doses in vivo is not ethical. Therefore, it is recommended to initiate preclinical studies in cells before animal or human investigations (Chou, 2010). Reporting antagonistic drug combinations is equally important as it may hint on possible contraindications in vivo and thus avoid unnecessary preclinical and clinical trials (Chou, 2010). The current study used human neuroblastoma BE(2)-M17 cells for their relative convenience to use and ability to induce neuronal differentiation (Andres et al., 2013) that is required at next stages of this research work.
A synergistic three-compound combination (D5L5U5) comprising of three nutraceutical compounds was identified in vitro from the present study. It was found to exert significantly higher ATP levels in the presence of Aβ1–42 compared to the two two-compound combinations (D5U5 and L5U5) from which the three-compound combination was derived (Figure 6). This finding was aided by the Chou-Talalay method of drug combinations which is based on median-effect principle (Chou and Talalay, 1984). This method is widely used in drug combinations for cancer, where the goal is selective cytotoxicity. Opposingly, the context for AD is cytoprotection, which may be a reason for the sparse use of this method in the field of AD. The novelty of the present study lies on the fact that it adapted the Chou-Talalay method to screen nutraceutical combinations that inhibited Aβ1–42–induced toxicity. The idea of prevention was explored in the current in vitro work by pre-treating with the compounds and the insult of Aβ1–42 introduced secondarily. This implicates that the current results indicative of AD prevention rather than treatment. As mentioned earlier, combination index (CI) is a quantitative assessment of drug combinations which uses dose-effect data of single compounds and the combinations and statistically derived doses of single compounds that give the same effect as that of the combinations to calculate CI. Combination index equals 1 for additive effect, CI is less than 1 (CI < 1) for synergistic effects and higher than 1 (CI > 1) for antagonistic effects (Chou and Talalay, 1984). The predicted three-compound combination was shown to be synergistic based on its CI value calculated by CompuSyn v.1.0. Dose reduction is an important aspect in drug combinations. The validation studies on relative ATP levels confirmed that the combination itself significantly inhibited Aβ1–42–induced toxicity compared to its constituents; DHA, LUT, and UA in equimolar doses (5 μM each) (Figure 6). The significance of the resulted combination is that it includes three neuroprotective compounds in relatively low concentrations (5 μM each) so that their multi-modes of actions are elicited without causing toxicity issues as observed for higher concentrations. Furthermore, dissolving these compounds in a single solvent (DMSO) was an added advantage that they could be combined within a single matrix without causing any solvent-based incompatibilities that may have resulted in cytotoxicity.
Polyphenolic conjugation is a novel strategy used to enhance the efficiency and biological activity of polyphenolic compounds (Cirillo et al., 2016). Similarly, fatty acid conjugation is reported to increase potency of therapeutic agents (Prakash et al., 2019). This technique is used in cancer drug therapy that anticancer drugs are conjugated with lipids such as DHA (lipid-drug conjugate) for targeted tumor therapy (Wang et al., 2012; Li et al., 2014; Irby et al., 2017). In fact, formation of fatty acid esters of polyphenols such as quercetin-3-O-glucoside have been shown beneficial for cell viability and survival of both human lung fibroblasts and human primary hepatocytes against H2O2-induced cytotoxicity (Warnakulasuriya et al., 2016). As DHA is a constitutive fatty acid in cell membranes, it may facilitate the passage of the conjugated polyphenols into cells increasing their bioavailability. In a similar manner, DHA in the three-compound combination may potentially conjugate with LUT and UA, leading to their increased bioavailability and thereby resulting in increased cell viability. All possible structures of DHA ester derivatives of LUT and UA and polyphenolic associations that may form during the cellular pre-treatment of the three-compound combination are shown in the Figure 7.
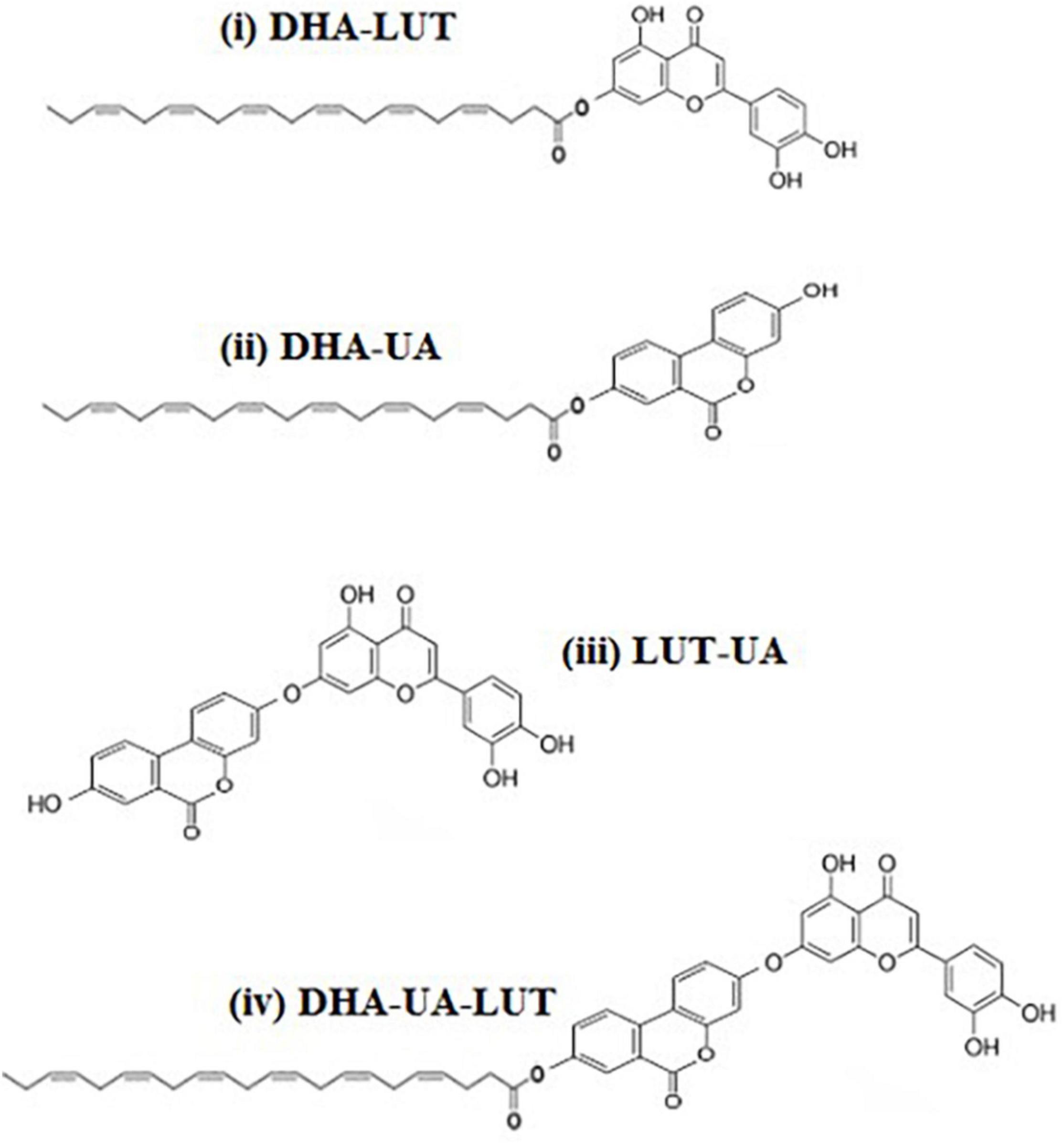
Figure 7. Predicted chemical conjugations for the three-compound combination, D5L5U5. For the DHA-LUT-UA combination, four chemical conjugations are predicted as (i) DHA-LUT (ii) DHA-UA (iii) LUT-UA (iv) DHA-UA-LUT.
Meanwhile, mechanisms of inhibiting Aβ1–42-induced toxicity by D5L5U5 are yet unknown. However, modified mitochondrial dehydrogenase activity which is indicated by the MTS results (Figure 4A) as well as the relatively higher ATP levels for D5L5U5 (Figure 6), are suggestive of profound beneficial effects on mitochondria. It can be hypothesized, further, that the exact mechanisms are similar to that of individual drugs in a combination (Chou, 2010). Considering the components of D5L5U5, DHA is thought to exert protection to neuronal mitochondria. Multiple lines of evidence show that dietary n-3 PUFA, specifically DHA gives beneficial effects on mitochondrial membrane organization (Fan et al., 2003; Khairallah et al., 2012) and mitochondrial function (Mayurasakorn et al., 2016). DHA has shown to reduce ROS production in vitro and Ca2+-induced mitochondrial membrane permeabilization in neonatal C57BL/6J mice following hypoxia-ischemia-brain injury (Mayurasakorn et al., 2016). Manipulation of membrane phospholipids in the mitochondrial membrane such as increasing cardiolipin content is proposed to be the mechanism of many of the beneficiary effects of DHA (Pepe et al., 1999). Mitochondria determine cell survival through the opening of the mPTP, which occurs under conditions of cell stress, causing mitochondrial depolarization and triggering of cell death as well as mitophagy. It has recently been found that dietary supplementation with a mixture of DHA and EPA (70:30 ratio) increased DHA and EPA in cardiac mitochondrial phospholipids and the tolerance of isolated mitochondria to Ca2+-induced mPTP opening (O’Shea et al., 2009). Moreover, it has been shown that supplementation with DHA per se also delayed Ca2+-induced mPTP opening (Khairallah et al., 2010). Luteolin, the second component of D5L5U5, is also widely appreciated in the literature for its mitoprotective activities. It has been shown to ameliorate mitochondrial damage in isoproterenol-induced myocardial infarction by maintaining lipid peroxidation metabolism due to its free radical scavenging, mitochondrial lipids, antioxidants and mitochondrial enzymes (Murugesan and Manju, 2013). It is thought to associate with up-regulation of autophagy (Hu et al., 2016; Cao et al., 2017) and improvement of mitochondrial biogenesis through inhibition of macrophage stimulating 1 protein (Hu et al., 2016). Interestingly, a growing body of evidence suggests that UA restores mitochondrial dysfunction by inducing mitophagy (Ryu et al., 2016; Andreux et al., 2019; Lin et al., 2020). Overall, further in vitro studies are warranted to identify the mechanisms of action of the synergistic three-compound nutraceutical combination for may be a steppingstone toward developing an advanced functional food for the prevention or co-treatment of AD.
Conclusion
The present study identified a synergistic three-compound combination, D5L5U5 that inhibits Aβ1–42-induced toxicity in vitro. This compound combination consisted of nutraceuticals: DHA, luteolin and Urolithin A each in 5 μM concentration, and Chou-Talalay method of drug combinations was used to derive it. Further in vitro and in vivo investigations are required to determine the mechanisms of action and validate this synergistic three-compound combination in the journey toward identifying an advanced functional food for the prevention or co-treatment of AD.
Data Availability Statement
The original contributions presented in the study are included in the article/Supplementary Material, further inquiries can be directed to the corresponding author/s.
Author Contributions
RM and DJ designed the study. DJ carried out all experiments and wrote the manuscript. EH closely supervised the experiments. RM, EH, GV, MG, and WF reviewed the manuscript intensively. EH, RM, MG, and WF edited the manuscript. All authors have read and agreed to the final version of this manuscript.
Funding
Edith Cowan University funding was provided to RM.
Conflict of Interest
The authors declare that the research was conducted in the absence of any commercial or financial relationships that could be construed as a potential conflict of interest.
Publisher’s Note
All claims expressed in this article are solely those of the authors and do not necessarily represent those of their affiliated organizations, or those of the publisher, the editors and the reviewers. Any product that may be evaluated in this article, or claim that may be made by its manufacturer, is not guaranteed or endorsed by the publisher.
Acknowledgments
Edith Cowan University is acknowledged for the award of Edith Cowan University postgraduate research scholarship (ECUPRS) for DJ.
Supplementary Material
The Supplementary Material for this article can be found online at: https://www.frontiersin.org/articles/10.3389/fnagi.2022.780602/full#supplementary-material
Supplementary Figure 1 | Relative ATP levels for the combination 1 (D5U10) and its components. ATP levels for the combination 1 (D5U10) and its components were determined using the CellTiter Glo assay. Data are expressed as mean ± SD from four (N = 4) independent experiments. Differences are significant at aP < 0.001 vs. vehicle control, ***P < 0.001 vs. Aβ1–42-treated control, #P < 0.05 vs. DHA 5 μM and UA 5 μM.
Supplementary Figure 2 | Relative ATP levels for the combination 1 (D10U10) and its components. ATP levels for the combination 3 (D10U10) and its constituents were determined using CellTiter Glo assay. Data are expressed as mean ± SD from four (N = 4) independent experiments. Differences are significant at aP < 0.001 vs. vehicle-treated control, ***P < 0.001 vs. Aβ1–42-treated control, DHA 5 μM and UA 5 μM.
Supplementary Figure 3 | Relative ATP levels for the combination 1 (D10U5) and its components. ATP levels for the combination 3 (D10U5) and its constituents were determined using CellTiter Glo assay. Data are expressed as mean ± SD from four (N = 4) independent experiments. Differences are significant at aP < 0.001 vs. vehicle control, ***P < 0.001 vs. Aβ1–42-treated control, DHA 10 μM and UA 5 μM.
Supplementary Figure 4 | Relative ATP levels for the combination 1 (D20L5) and its components. ATP levels for the combination 4 (D20L5) and its constituents were determined using CellTiter Glo assay. Data are expressed as mean ± SD from four (N = 4) independent experiments. Differences are significant at aP < 0.001 vs. vehicle-treated control, ***P < 0.001 vs. Aβ1–42-treated control, DHA 10 μM.
Supplementary Figure 5 | Relative ATP levels for the combination 1 (D10L20) and its components. ATP levels for the combination 6 (D10U5) and its constituents were determined using CellTiter Glo assay. Data are expressed as mean ± SD from four (N = 4) independent experiments. Differences are significant at aP < 0.001 vs. vehicle-treated control, ***P < 0.001 vs. Aβ1–42-treated control, DHA 10 μM and UA 5 μM.
Supplementary Figure 6 | Relative ATP levels for the combination 1 (D5L10) and its components. ATP levels for the combination 6 (D5L10) and its constituents were determined using CellTiter Glo assay. Data are expressed as mean ± SD from four (N = 4) independent experiments. Differences are significant at aP < 0.001 vs. vehicle control, ***P < 0.001 vs. Aβ1–42-treated control, DHA 5 μM.
Supplementary Figure 7 | Relative ATP levels for the combination 7 (D10L10) and its components. ATP levels for the combination 7 (D10L10) and its constituents were determined using CellTiter Glo assay. Data are expressed as mean ± SD from four (N = 4) independent experiments. Differences are significant at aP < 0.001 vs. vehicle control, ***P < 0.001 vs Aβ1–42-treated control, DHA 10 μM.
Supplementary Figure 8 | Relative ATP levels for the combination 9 (D20L10) and its components. ATP levels for the combination 9 (D20L10) and its constituents were determined using CellTiter Glo assay. Data are expressed as mean ± SD from four (N = 4) independent experiments. Differences are significant at aP < 0.001 vs. vehicle control, ***P < 0.001 vs. Aβ1–42-treated control, DHA 20 μM.
Supplementary Figure 9 | Relative ATP levels for the combination 10 (D10L5) and its components. ATP levels for the combination 10 (D10L5) and its constituents were determined using CellTiter Glo assay. Data are expressed as mean ± SD from four (N = 4) independent experiments. Differences are significant at aP < 0.001 vs vehicle control, ***P < 0.001 vs. Aβ1–42-treated control, DHA 10 μM.
Supplementary Figure 10 | Relative ATP levels for the combination 12 (L20U5) and its components. ATP levels for the combination 12 (L20U5) and its constituents were determined using CellTiter Glo assay. Data are expressed as mean ± SD from four (N = 4) independent experiments. Differences are significant at aP < 0.001 vs. vehicle control, ***P < 0.001 vs. Aβ1–42-treated control, LUT 20 μM and UA 5 μM.
Supplementary Figure 11 | Relative ATP levels for the combination 13 (L10U5) and its components. ATP levels for the combination 13 (L10U5) and its constituents were determined using CellTiter Glo assay. Data are expressed as mean ± SD from four (N = 4) independent experiments. Differences are significant at aP < 0.001 vs. vehicle control, ***P < 0.001 vs. Aβ1–42-treated control, LUT 10 μM and UA 5 μM.
References
Anderson, R. M., Hadjichrysanthou, C., Evans, S., and Wong, M. M. (2017). Why do so many clinical trials of therapies for Alzheimer’s disease fail? Lancet 390, 2327–2329.
Andres, D., Keyser, B. M., Petrali, J., Benton, B., Hubbard, K. S., Mcnutt, P. M., et al. (2013). Morphological and functional differentiation in BE(2)-M17 human neuroblastoma cells by treatment with Trans-retinoic acid. BMC Neurosci. 14:49. doi: 10.1186/1471-2202-14-49
Andreux, P. A., Blanco-Bose, W., Ryu, D., Burdet, F., Ibberson, M., Aebischer, P., et al. (2019). The mitophagy activator urolithin A is safe and induces a molecular signature of improved mitochondrial and cellular health in humans. Nat. Metab. 1, 595–603. doi: 10.1038/s42255-019-0073-4
Banik, A., Brown, R. E., Bamburg, J., Lahiri, D. K., Khurana, D., Friedland, R. P., et al. (2015). Translation of pre-clinical studies into successful clinical trials for Alzheimer’s disease: what are the roadblocks and how can they be overcome? J. Alzheimer’s Dis. 47, 815–843. doi: 10.3233/JAD-150136
Cao, Z., Zhang, H., Cai, X., Fang, W., Chai, D., Wen, Y., et al. (2017). Luteolin promotes cell apoptosis by inducing autophagy in hepatocellular carcinoma. Cell Physiol. Biochem. 43, 1803–1812. doi: 10.1159/000484066
Cerda, B., Llorach, R., Ceron, J. J., Espin, J. C., and Tomas-Barberan, F. A. (2003). Evaluation of the bioavailability and metabolism in the rat of punicalagin, an antioxidant polyphenol from pomegranate juice. Eur. J. Nutr. 42, 18–28. doi: 10.1007/s00394-003-0396-4
Chaudhari, S. M., Patel, K. Y., and Badole, S. L. (2014). “Chapter 106 - Punica granatum (Pomegranate Fruit): in Cancer Treatment,” in Polyphenols in Human Health and Disease, eds R. R. Watson, V. R. Preedy, and S. Zibadi (San Diego, CA: Academic Press), 1393–1400.
Choi, S. M., Kim, B. C., Cho, Y. H., Choi, K. H., Chang, J., Park, M. S., et al. (2014). Effects of flavonoid compounds on beta-amyloid-peptide-induced neuronal death in cultured mouse cortical neurons. Chonnam. Med. J. 50, 45–51. doi: 10.4068/cmj.2014.50.2.45
Chou, T. C. (2006). Theoretical basis, experimental design, and computerized simulation of synergism and antagonism in drug combination studies. Pharmacol. Rev. 58, 621–681.
Chou, T. C. (2010). Drug combination studies and their synergy quantification using the Chou-Talalay method. Cancer Res. 70, 440–446.
Chou, T. C., and Talalay, P. (1984). Quantitative analysis of dose-effect relationships: the combined effects of multiple drugs or enzyme inhibitors. Adv. Enzyme Regul. 22, 27–55.
Chumakov, I., Nabirotchkin, S., Cholet, N., Milet, A., Boucard, A., Toulorge, D., et al. (2015). Combining two repurposed drugs as a promising approach for Alzheimer’s disease therapy. Sci. Rep. 5:7608.
Cirillo, G., Curcio, M., Vittorio, O., Iemma, F., Restuccia, D., Spizzirri, U. G., et al. (2016). Polyphenol conjugates and human health: a perspective review. Crit. Rev. Food Sci. Nutr. 56, 326–337. doi: 10.1080/10408398.2012.752342
Cremonini, A. L., Caffa, I., Cea, M., Nencioni, A., Odetti, P., and Monacelli, F. (2019). Nutrients in the prevention of Alzheimer’s disease. Oxid. Med. Cell. Longev. 2019:9874159.
Cummings, J., Lee, G., Ritter, A., Sabbagh, M., and Zhong, K. (2020). Alzheimer’s disease drug development pipeline: 2020. Alzheimers Dement. Transl. Res. Clin. Intervent. 6:e12050.
Cummings, J. L., Schneider, E., Tariot, P. N., and Graham, S. M. (2006). Behavioral effects of memantine in Alzheimer disease patients receiving donepezil treatment. Neurology 67:57.
Cummings, J. L., Tong, G., and Ballard, C. (2019). Treatment combinations for Alzheimer’s disease: current and future pharmacotherapy options. J. Alzheimers Dis. 67, 779–794.
Dantoine, T., Auriacombe, S., Sarazin, M., Becker, H., Pere, J.-J., and Bourdeix, I. (2006). Rivastigmine monotherapy and combination therapy with memantine in patients with moderately severe Alzheimer’s disease who failed to benefit from previous cholinesterase inhibitor treatment. Int. J. Clin. Pract. 60, 110–118. doi: 10.1111/j.1368-5031.2005.00769.x
De Kok, T. M., Van Breda, S. G., and Manson, M. M. (2008). Mechanisms of combined action of different chemopreventive dietary compounds: a review. Eur. J. Nutr. 47(Suppl. 2), 51–59.
Efferth, T., and Koch, E. (2011). Complex interactions between phytochemicals. The multi-target therapeutic concept of phytotherapy. Curr. Drug Targets 12, 122–132. doi: 10.2174/138945011793591626
Espargaró, A., Ginex, T., Vadell, M. D. M., Busquets, M. A., Estelrich, J., Muñoz-Torrero, D., et al. (2017). Combined in vitro cell-based/in silico screening of naturally occurring flavonoids and phenolic compounds as potential anti-alzheimer drugs. J. Nat. Prod. 80, 278–289. doi: 10.1021/acs.jnatprod.6b00643
Espin, J. C., Larrosa, M., Garcia-Conesa, M. T., and Tomas-Barberan, F. (2013). Biological significance of urolithins, the gut microbial ellagic Acid-derived metabolites: the evidence so far. Evid. Based Complement. Alternat. Med. 2013:270418. doi: 10.1155/2013/270418
Fan, Y. Y., Mcmurray, D. N., Ly, L. H., and Chapkin, R. S. (2003). Dietary (n-3) polyunsaturated fatty acids remodel mouse T-cell lipid rafts. J. Nutr. 133, 1913–1920. doi: 10.1093/jn/133.6.1913
Galvin, J. E. (2017). Prevention of Alzheimer’s disease: lessons learned and applied. J. Am. Geriatr. Soc. 65, 2128–2133.
Gong, Z., Huang, J., Xu, B., Ou, Z., Zhang, L., Lin, X., et al. (2019). Urolithin A attenuates memory impairment and neuroinflammation in APP/PS1 mice. J. Neuroinflam. 16:62. doi: 10.1186/s12974-019-1450-3
Grimm, M. O., Kuchenbecker, J., Grosgen, S., Burg, V. K., Hundsdorfer, B., Rothhaar, T. L., et al. (2011). Docosahexaenoic acid reduces amyloid beta production via multiple pleiotropic mechanisms. J. Biol. Chem. 286, 14028–14039. doi: 10.1074/jbc.M110.182329
Grodzicki, W., and Dziendzikowska, K. (2020). The role of selected bioactive compounds in the prevention of alzheimer’s disease. Antioxidants 9:229.
Hartman, R. E., Shah, A., Fagan, A. M., Schwetye, K. E., Parsadanian, M., Schulman, R. N., et al. (2006). Pomegranate juice decreases amyloid load and improves behavior in a mouse model of Alzheimer’s disease. Neurobiol. Dis. 24, 506–515. doi: 10.1016/j.nbd.2006.08.006
Hashimoto, M., Shahdat, H. M., Katakura, M., Tanabe, Y., Gamoh, S., Miwa, K., et al. (2009). Effects of docosahexaenoic acid on in vitro amyloid beta peptide 25-35 fibrillation. Biochim. Biophys. Acta 1791, 289–296.
Hossain, S., Hashimoto, M., Katakura, M., Miwa, K., Shimada, T., and Shido, O. (2009). Mechanism of docosahexaenoic acid-induced inhibition of in vitro Aβ1–42 fibrillation and Aβ1–42-induced toxicity in SH-S5Y5 cells. J. Neurochem. 111, 568–579.
Hu, J., Man, W., Shen, M., Zhang, M., Lin, J., Wang, T., et al. (2016). Luteolin alleviates post-infarction cardiac dysfunction by up-regulating autophagy through Mst1 inhibition. J. Cell Mol. Med. 20, 147–156. doi: 10.1111/jcmm.12714
Irby, D., Du, C., and Li, F. (2017). Lipid-drug conjugate for enhancing drug delivery. Mol. Pharm. 14, 1325–1338.
Jayatunga, D. P. W., Hone, E., Fernando, W. M. A. D. B., Garg, M. L., Verdile, G., and Martins, R. N. (2021). Mitoprotective effects of a Synergistic Nutraceutical Combination: basis for a prevention strategy against Alzheimer’s Disease. Front. Aging Neurosci. (in press).
Kabir, M. T., Uddin, M. S., Mamun, A. A., Jeandet, P., Aleya, L., Mansouri, R. A., et al. (2020). Combination drug therapy for the management of Alzheimer’s disease. Int J Mol Sci 21:3272.
Khairallah, R. J., Kim, J., O’Shea, K. M., O’connell, K. A., Brown, B. H., Galvao, T., et al. (2012). Improved mitochondrial function with diet-induced increase in either docosahexaenoic acid or arachidonic acid in membrane phospholipids. PLoS One 7:e34402. doi: 10.1371/journal.pone.0034402
Khairallah, R. J., Sparagna, G. C., Khanna, N., O’Shea, K. M., Hecker, P. A., Kristian, T., et al. (2010). Dietary supplementation with docosahexaenoic acid, but not eicosapentaenoic acid, dramatically alters cardiac mitochondrial phospholipid fatty acid composition and prevents permeability transition. Biochim. Biophys. Acta 1797, 1555–1562.
Lange, K. W., Guo, J., Kanaya, S., Lange, K. M., Nakamura, Y., and Li, S. (2019). Medical foods in Alzheimer’s disease. Food Sci. Hum. Wellness 8, 1–7.
Li, S., Qin, J., Tian, C., Cao, J., Fida, G., Wang, Z., et al. (2014). The targeting mechanism of DHA ligand and its conjugate with Gemcitabine for the enhanced tumor therapy. Oncotarget 5, 3622–3635. doi: 10.18632/oncotarget.1969
Lim, G. P., Calon, F., Morihara, T., Yang, F., Teter, B., Ubeda, O., et al. (2005). A diet enriched with the omega-3 fatty acid docosahexaenoic acid reduces amyloid burden in an aged Alzheimer mouse model. J. Neurosci. 25, 3032–3040. doi: 10.1523/JNEUROSCI.4225-04.2005
Lin, J., Zhuge, J., Zheng, X., Wu, Y., Zhang, Z., Xu, T., et al. (2020). Urolithin A-induced mitophagy suppresses apoptosis and attenuates intervertebral disc degeneration via the AMPK signaling pathway. Free Radic. Biol. Med. 150, 109–119. doi: 10.1016/j.freeradbiomed.2020.02.024
Liu, H., Zeng, Z., Wang, S., Li, T., Mastriani, E., Li, Q.-H., et al. (2017). Main components of pomegranate, ellagic acid and luteolin, inhibit metastasis of ovarian cancer by down-regulating MMP2 and MMP9. Cancer Biol. Ther. 18, 990–999. doi: 10.1080/15384047.2017.1394542
Mayurasakorn, K., Niatsetskaya, Z. V., Sosunov, S. A., Williams, J. J., Zirpoli, H., Vlasakov, I., et al. (2016). DHA but not EPA emulsions preserve neurological and mitochondrial function after brain hypoxia-ischemia in neonatal mice. PLoS One 11:e0160870. doi: 10.1371/journal.pone.0160870
Mehta, D., Jackson, R., Paul, G., Shi, J., and Sabbagh, M. (2017). Why do trials for Alzheimer’s disease drugs keep failing? A discontinued drug perspective for 2010-2015. Expert Opin. Investig. Drugs 26, 735–739. doi: 10.1080/13543784.2017.1323868
Moreno, S., Perno, C., Mallon, P., Behrens, G., Corbeau, P., Routy, J.-P., et al. (2019). Two-drug vs. three-drug combinations for HIV-1: do we have enough data to make the switch? HIV Med. 20, 2–12.
Murphy, M. P., and Levine, H. III (2010). Alzheimer’s disease and the amyloid-beta peptide. J. Alzheimers Dis. 19, 311–323.
Murugesan, M., and Manju, V. (2013). Luteolin promotes mitochondrial protection during acute and chronic periods of isoproterenol induced myocardial infarction in rats. Egypt. Heart J. 65, 319–327.
O’Shea, K. M., Khairallah, R. J., Sparagna, G. C., Xu, W., Hecker, P. A., Robillard-Frayne, I., et al. (2009). Dietary omega-3 fatty acids alter cardiac mitochondrial phospholipid composition and delay Ca2+-induced permeability transition. J. Mol. Cell Cardiol. 47, 819–827.
Panza, F., Lozupone, M., Solfrizzi, V., Custodero, C., Valiani, V., D’introno, A., et al. (2018). “Chapter 9 - Contribution of Mediterranean Diet in the Prevention of Alzheimer’s Disease,” in Role of the Mediterranean Diet in the Brain and Neurodegenerative Diseases, eds T. Farooqui and A. A. Farooqui (Cambridge, MA: Academic Press), 139–155.
Patti, A. M., Toth, P. P., Giglio, R. V., Banach, M., Noto, M., Nikolic, D., et al. (2017). Nutraceuticals as an important part of combination therapy in dyslipidaemia. Curr. Pharm. Des. 23, 2496–2503.
Peltomaa, E., Johnson, M. D., and Taipale, S. J. (2017). Marine cryptophytes are great sources of EPA and DHA. Mar. Drugs 16:3. doi: 10.3390/md16010003
Peng, Y., Tao, H., Wang, S., Xiao, J., Wang, Y., and Su, H. (2021). Dietary intervention with edible medicinal plants and derived products for prevention of Alzheimer’s disease: a compendium of time-tested strategy. J. Funct. Foods 81:104463.
Pepe, S., Tsuchiya, N., Lakatta, E. G., and Hansford, R. G. (1999). PUFA and aging modulate cardiac mitochondrial membrane lipid composition and Ca2+ activation of PDH. Am. J. Physiol. 276, H149–H158. doi: 10.1152/ajpheart.1999.276.1.H149
Prakash, T. P., Mullick, A. E., Lee, R. G., Yu, J., Yeh, S. T., Low, A., et al. (2019). Fatty acid conjugation enhances potency of antisense oligonucleotides in muscle. Nucleic Acids Res. 47, 6029–6044.
Rabinovici, G. D. (2021). Controversy and progress in Alzheimer’s disease — FDA approval of aducanumab. N. Engl. J. Med. 385, 771–774.
Riepe, M. W., Adler, G., Ibach, B., Weinkauf, B., Tracik, F., and Gunay, I. (2007). Domain-specific improvement of cognition on memantine in patients with Alzheimer’s disease treated with rivastigmine. Dement. Geriatr. Cogn. Disord. 23, 301–306.
Ryu, D., Mouchiroud, L., Andreux, P. A., Katsyuba, E., Moullan, N., Nicolet-Dit-Félix, A. A., et al. (2016). Urolithin A induces mitophagy and prolongs lifespan in C. elegans and increases muscle function in rodents. Nat. Med. 22, 879–888. doi: 10.1038/nm.4132
Santana-Gálvez, J., Cisneros-Zevallos, L., and Jacobo-Velázquez, D. A. (2019). A practical guide for designing effective nutraceutical combinations in the form of foods, beverages, and dietary supplements against chronic degenerative diseases. Trends Food Sci. Technol. 88, 179–193.
Scarmeas, N., Stern, Y., Tang, M.-X., Mayeux, R., and Luchsinger, J. A. (2006). Mediterranean diet and risk for Alzheimer’s disease. Ann. Neurol. 59, 912–921.
Seeram, N. P., Henning, S. M., Zhang, Y., Suchard, M., Li, Z., and Heber, D. (2006). Pomegranate Juice Ellagitannin Metabolites Are Present in Human Plasma and Some Persist in Urine for Up to 48 Hours. J. Nutr. 136, 2481–2485. doi: 10.1093/jn/136.10.2481
Sharman, M. J., Gyengesi, E., Liang, H., Chatterjee, P., Karl, T., Li, Q. X., et al. (2019). Assessment of diets containing curcumin, epigallocatechin-3-gallate, docosahexaenoic acid and alpha-lipoic acid on amyloid load and inflammation in a male transgenic mouse model of Alzheimer’s disease: are combinations more effective? Neurobiol. Dis. 124, 505–519. doi: 10.1016/j.nbd.2018.11.026
Simoni, E., Daniele, S., Bottegoni, G., Pizzirani, D., Trincavelli, M. L., Goldoni, L., et al. (2012). Combining galantamine and memantine in multitargeted, new chemical entities potentially useful in Alzheimer’s disease. J. Med. Chem. 55, 9708–9721. doi: 10.1021/jm3009458
Sreekumar, S., Sithul, H., Muraleedharan, P., Azeez, J. M., and Sreeharshan, S. (2014). Pomegranate fruit as a rich source of biologically active compounds. Biomed. Res. Int. 2014:686921.
Stine, W. B., Jungbauer, L., Yu, C., and Ladu, M. J. (2011). Preparing synthetic Abeta in different aggregation states. Methods Mol. Biol. 670, 13–32.
Sun, W., Sanderson, P. E., and Zheng, W. (2016). Drug combination therapy increases successful drug repositioning. Drug Discov. Today 21, 1189–1195.
Tariot, P. N., Farlow, M. R., Grossberg, G. T., Graham, S. M., Mcdonald, S., Gergel, I., et al. (2004). Memantine treatment in patients with moderate to severe Alzheimer disease already receiving DonepezilA randomized controlled trial. JAMA 291, 317–324.
Thaipisuttikul, P., and Galvin, J. E. (2012). Use of medical foods and nutritional approaches in the treatment of Alzheimer’s disease. Clin. Pract. 9, 199–209.
Thomas, E., and Egon, K. (2011). Complex interactions between phytochemicals. The multi-target therapeutic concept of phytotherapy. Curr. Drug Targets 12, 122–132.
Tiwari, M. K., and Kepp, K. P. (2016). β-Amyloid pathogenesis: chemical properties versus cellular levels. Alzheimers Dement. 12, 184–194. doi: 10.1016/j.jalz.2015.06.1895
Tocher, D. R. (2015). Omega-3 long-chain polyunsaturated fatty acids and aquaculture in perspective. Aquaculture 449, 94–107.
Van Elswijk, D. A., Schobel, U. P., Lansky, E. P., Irth, H., and Van Der Greef, J. (2004). Rapid dereplication of estrogenic compounds in pomegranate (Punica granatum) using on-line biochemical detection coupled to mass spectrometry. Phytochemistry 65, 233–241. doi: 10.1016/j.phytochem.2003.07.001
Van Norman, G. A. (2020). Limitations of animal studies for predicting toxicity in clinical trials: part 2: potential alternatives to the use of animals in preclinical trials. JACC Basic Transl. Sci. 5, 387–397. doi: 10.1016/j.jacbts.2020.03.010
Wang, J., Luo, T., Li, S., and Zhao, J. (2012). The powerful applications of polyunsaturated fatty acids in improving the therapeutic efficacy of anticancer drugs. Expert Opin. Drug Deliv. 9, 1–7. doi: 10.1517/17425247.2011.618183
Warnakulasuriya, S. N., and Ziaullah Rupasinghe, H. P. V. (2016). Long chain fatty acid esters of Quercetin-3-O-glucoside attenuate H2O2-induced acute cytotoxicity in human lung fibroblasts and primary hepatocytes. Molecules 21, 452–452. doi: 10.3390/molecules21040452
Xia, F., Wang, C., Jin, Y., Liu, Q., Meng, Q., Liu, K., et al. (2014). Luteolin protects HUVECs from TNF-alpha-induced oxidative stress and inflammation via its effects on the Nox4/ROS-NF-kappaB and MAPK pathways. J. Atheroscler. Thromb. 21, 768–783. doi: 10.5551/jat.23697
Yuan, T., Ma, H., Liu, W., Niesen, D. B., Shah, N., Crews, R., et al. (2016). Pomegranate’s neuroprotective effects against Alzheimer’s disease are mediated by urolithins, its ellagitannin-gut microbial derived metabolites. ACS Chem. Neurosci. 7, 26–33.
Zheng, N., Yuan, P., Li, C., Wu, J., and Huang, J. (2015). Luteolin reduces BACE1 expression through NF-kappaB and through estrogen receptor mediated pathways in HEK293 and SH-SY5Y Cells. J. Alzheimers Dis. 45, 659–671. doi: 10.3233/JAD-142517
Keywords: Alzheimer’s disease, docosahexaenoic acid, in vitro, Luteolin, synergistic nutraceutical combinations, urolithin A
Citation: Jayatunga DPW, Hone E, Fernando WMADB, Garg ML, Verdile G and Martins RN (2022) A Synergistic Combination of DHA, Luteolin, and Urolithin A Against Alzheimer’s Disease. Front. Aging Neurosci. 14:780602. doi: 10.3389/fnagi.2022.780602
Received: 21 September 2021; Accepted: 10 January 2022;
Published: 16 February 2022.
Edited by:
Christian Neri, Institut National de la Santé et de la Recherche Médicale (INSERM), FranceReviewed by:
Małgorzata Kujawska, Poznan University of Medical Sciences, PolandAvijit Banik, Emory University, United States
Copyright © 2022 Jayatunga, Hone, Fernando, Garg, Verdile and Martins. This is an open-access article distributed under the terms of the Creative Commons Attribution License (CC BY). The use, distribution or reproduction in other forums is permitted, provided the original author(s) and the copyright owner(s) are credited and that the original publication in this journal is cited, in accordance with accepted academic practice. No use, distribution or reproduction is permitted which does not comply with these terms.
*Correspondence: Ralph N. Martins, ci5tYXJ0aW5zQGVjdS5lZHUuYXU=