- Composting Research Group, Department of Chemical, Biological and Environmental Engineering, Universitat Autònoma de Barcelona, Barcelona, Spain
In recent years, the high cost and availability of energy sources have boosted the implementation of strategies to obtain different types of renewable energy. Among them, methane contained in biogas from anaerobic digestion has gained special relevance, since it also permits the management of a big amount of organic waste and the capture and long-term storage of carbon. However, methane from biogas presents some problems as energy source: 1) it is a gas, so its storage is costly and complex, 2) it is not pure, being carbon dioxide the main by-product of anaerobic digestion (30%–50%), 3) it is explosive with oxygen under some conditions and 4) it has a high global warming potential (27–30 times that of carbon dioxide). Consequently, the conversion of biogas to methanol is as an attractive way to overcome these problems. This process implies the conversion of both methane and carbon dioxide into methanol in one oxidation and one reduction reaction, respectively. In this dual system, the use of effective and selective catalysts for both reactions is a critical issue. In this regard, nanomaterials embedded in metal organic frameworks have been recently tested for both reactions, with very satisfactory results when compared to traditional materials. In this review paper, the recent configurations of catalysts including nanoparticles as active catalysts and metal organic frameworks as support materials are reviewed and discussed. The main challenges for the future development of this technology are also highlighted, that is, its cost in environmental and economic terms for its development at commercial scale.
Introduction
In last years, the recent increase in the cost and scarcity of traditional energy sources has boosted the interest in renewable energy sources. Among them, anaerobic digestion of organic waste has gained especially interest among researchers, public administrations, and private companies (Lora Granado et al., 2017). The main advantages of anaerobic digestion are the worldwide availability of organic wastes and the low environmental impact of this technology when compared with other management strategies such as landfill (Mondello et al., 2017) and even composting, especially in energy terms (Colón et al., 2012).
Anaerobic digestion is a natural process that results in the production of biogas that can be a useful source of renewable energy. The production of methane from biogas has been reviewed from the point of view of operational strategies (Komilis et al., 2017) and the use of additives to increase the amount of biogas and its content in methane (Barrena et al., 2022). Methane, being the major component of natural gas, is a useful renewable source of energy when it comes from biogas. However, methane, to be used, presents several challenges: 1) being a gas, its storage requires high volumes, 2) it is not pure, being carbon dioxide the main by-product of anaerobic digestion (30%–50%), with other tracer gases like nitrogen or hydrogen, which are not a problem for biogas uses, being the most dangerous and undesirable hydrogen sulphide (Yuan Chen et al., 2015; Wang et al., 2019; Vu et al., 2022) 3) it is explosive and flammable with oxygen under some specific conditions, which are relatively low concentrations of methane (within 9%–15%), although other factors such as the geometry of pipelines is crucial (Kundu et al., 2016) and 4) it has a high global warming potential (27–30 times of that of carbon dioxide, approximately) (USEPA, 2022). Another point important to mention is that the contents of biogas and the relative percentage of its main gases (methane and carbon dioxide) can change as a result of the waste composition (Herout et al., 2011), the conditions of anaerobic digestion (Sołowski, 2022) and the use of some additives (Cerrillo et al., 2021). All these factors must be carefully considered when methane and carbon dioxide are intended to be transformed into methanol.
To overcome these problems, several strategies have been proposed, but the only one that can give a positive solution to all these challenges in the full conversion of biogas (including methane and carbon dioxide) into methanol (Figure 1). Methanol is liquid at ambient conditions, easy to transport and use as source of energy and a starting chemical for the synthesis of other more complex organic materials (Zhang et al., 2019; Pawelczyk et al., 2022).
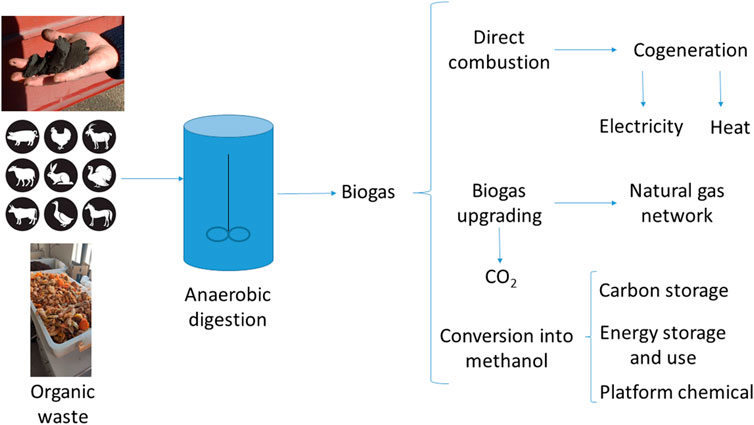
FIGURE 1. General perspective of the possible uses of biogas from anaerobic digestion including direct combustion, upgrading to natural gas, and conversion to methanol.
However, the conversion of the main compounds of biogas into methanol is not straightforward. On one hand, it implies the oxidation of methane to methanol, an exothermic oxidation reaction that needs very selective catalysts to avoid the formation of typical undesirable by-products from the combustion of methane: carbon dioxide and carbon monoxide (Zacaria and Kamarudin, 2016). One the other hand, the conversion of carbon dioxide to methanol is a hydrogen consuming reduction that needs effective catalysts that provide mild conditions in terms of temperature and pressure to be profitable in terms of economic cost and environmental impact, although the reaction is thermodynamically favourable (Zain and Mohamed, 2018). Finally, biogas is not pure, as it contains some compounds that could harm the catalyst, especially hydrogen sulphide (Wang et al., 2019).
The main objective of this paper is to give a general perspective of the overall conversion of biogas to methanol. Methanol can be used as a source of renewable energy that can be easily stored, transported and used, which gives it important advantages when compared to biogas. However, there are several shortcomings and challenges that hampers the implementation of this conversion, especially at full scale. This paper compiles the main advances and challenges in the development of new catalysts based on nanoparticles (NP) immobilized in metal organic frameworks (MOF) to improve the conversion of biogas to methanol.
Chemical reactions
Oxidation of methane to methanol
Conversion of methane into methanol can be achieved through two different routes. The indirect route is a two-step process: 1) partial oxidation or steam reforming of methane to syngas (CO + H2) and 2) catalytic conversion of syngas to methanol. It is known that the steam reforming step is an endothermic reaction (∆H298K0 = +206.2 kJ·mol−1), therefore, this process is extremely energy demanding. To overcome this problem, the direct route based on the conversion of methane to methanol at low temperature has been recently explored (Xie et al., 2018).
Partial oxidation of methane is an energy-saving process that converts methane to partially oxidized compounds such as methanol, formic acid or formaldehyde. This route is thermodynamically favorable and uses oxygen as oxidant (Eq. 1). Although oxygen is the oxidant used in practically all the cases, water vapor at high temperature and pressure has been also explored (Khirsariya and Mewada, 2013).
Regarding this direct route, different works have been published regarding its main drawback: overoxidation of methane to carbon monoxide and/or carbon dioxide. The main strategies involve the use of selective catalysts such as different types of zeolites working at low temperature resulting in moderate conversions (Tomkins et al., 2017) and the activation of methane in a liquid phase using H2O and H2O2 as oxidants (Hammond et al., 2012; Sushkevich et al., 2017). However, as explained later, selected NP and MOF offer a promising alternative for the direct route to convert methane into methanol, with high selectivity.
Reduction of carbon dioxide to methanol
Carbon capture and utilisation has the objective to transform carbon dioxide into useful products such as chemical feedstock and renewable fuels (Saeidi et al., 2021). Specifically, this paper focuses on the catalytic hydrogenation of carbon dioxide, since it is considered as the most used and simplest process (Li and Tsang, 2018). Several chemical products can be obtained from carbon dioxide hydrogenation, being methanol the most common. In the past decades, several routes have been proposed and industrially exploited for the synthesis of methanol using heterogeneous catalysts such as Cu, Cr, and Zn oxides, which typically need high pressure and low temperature (Ertl et al., 2008), Currently, the Cu/ZnO/Al2O3 catalyst is the most used material for the conversion of carbon dioxide to methanol.
The hydrogenation of carbon dioxide to methanol consists of two main competing reactions. The first is the methanol synthesis from carbon dioxide and hydrogen (Eq. 2):
The second reaction is the reverse water-gas shift reaction that produces carbon monoxide (Eq. 3):
Furthermore, catalytic hydrogenation of carbon dioxide to methanol can also occur indirectly from carbon monoxide formed through the previous reaction (Eq. 4).
As observed, the increase of pressure and the decrease of temperature will shift the reaction towards the products.
Among the different options of catalyst, copper is economically favourable. For the methanol synthesis through the hydrogenation of carbon monoxide and carbon dioxide, special emphasis on copper metal acting as the active phase and zinc oxide as the active promoter has been reported (Álvarez et al., 2017). This catalyst reduces the pressure up to 50–100 bar, while methanol selectivity is around 50%. In fact, bimetallic catalysts permit a change in the adsorption properties of metal surfaces, leading to an improved catalytic yield (Li and Tsang, 2018). Other examples of catalysts such as Pd-Zn and Pd-Ga (Collins et al., 2012) or Cu-Ni and Ga-Ni (Studt et al., 2014) have been proposed. Among them, Pd-Zn based catalysts can act as an unusually high kinetic barrier for Eq. 2 under low pressure conditions (20 bar). Another important issue in this conversion is the catalytic yield. To obtain acceptable values, several types of supports are used to increase the adsorption capacity of the active surfaces and to increase the surface area. Regarding supports, capping agents are probably the most used (Javed et al., 2020) with abundant examples of zeolite, activated carbon and alumina (Choi et al., 2009; Alonso et al., 2017; Carrasco-García, 2022).
At the same time, carbon dioxide presents two points that make its conversion into methanol less favourable than that of methane: 1) it has a much lower global warming potential, 2) biogas contents more methane than carbon dioxide and 3) chemically, its conversion presents less favourable conditions and needs hydrogen. Therefore, a rigorous sustainable assessment (environmental and economic) should be performed to consider the entire conversion of biogas into methanol or the sole conversion of methane.
Integration of both reactions
Figure 2 explains a proposal for the integrated conversion of biogas to methanol, including all the required steps. Although the core of Figure 2 is the conversion of biogas into methanol using NP and MOF and will be discussed in the next point, two other pretreatment steps should be highlighted.
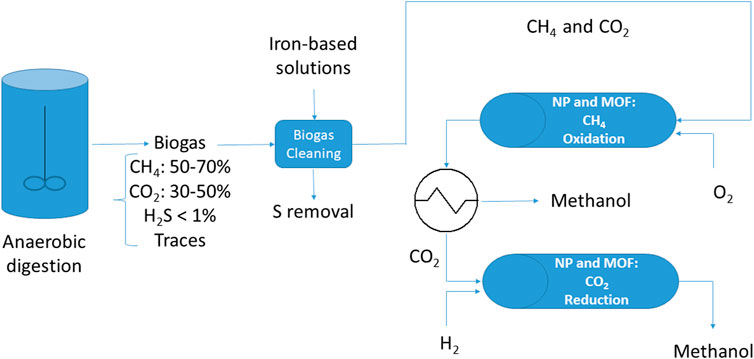
FIGURE 2. Proposal for the integrated conversion of biogas to methanol, including biogas purification and sequential conversion of methane (oxidation) and carbon dioxide (reduction) to methanol.
The first one is not represented and Figure 2 and may be optional, but it is critical for the next catalytic steps. A problem often not considered is the presence of water vapour in biogas. Although not being a product of anaerobic digestion of organic waste, biogas can be easily water-saturated (Petersson and WellinGer, 2018). The removal of water from the biogas stream can be easily achieved by cooling, compression, absorption or adsorption. This removal is critical because of several reasons: water may condensate in gas pipelines and cause corrosion, it causes damage to the catalysts and supports, it participates in some secondary reactions, thus altering kinetics and possible equilibria, and it hampers some biogas upgrading strategies (Duran et al., 2018).
Removal of biogas contaminants
The second important pretreatment is the removal of hydrogen sulphide from biogas. Hydrogen sulphide is inherently produced in the anaerobic digestion of organic waste (Vu et al., 2022) and it is a problematic impurity that can inhibit methanogenesis and cause equipment corrosion. Regarding the catalytic conversion of biogas to methanol, hydrogen sulphide and, in general, sulphur compounds, are one of the main chemicals provoking the poisoning of heterogeneous catalysts (Wachter et al., 2021). Traditionally, the use of iron-based formulations have been used for the total and partial removal of hydrogen sulphide in biogas (Magnone et al., 2018; Persson et al., 2021). However, emerging strategies using other approaches have been recently published. They are summarized in Table 1, where adsorption and biological treatments are predominant. It also worthwhile to mention that studies from various groups are focused on other detrimental gases found in biogas such as siloxanes (Yang and Corsolini, 2019; Piechota, 2021).
In general, all these strategies presented need to be carefully considered, as the effect of hydrogen sulphide on sophisticated high-cost catalyst based on NPs and MOF can limit the implementation of the entire process (Figure 2).
New catalysts: Nanoparticles and metal-organic frameworks
Traditional catalysts
Zeolite, in its different configurations, has been the traditional catalyst used in the conversion of methane and carbon dioxide to methanol. In 1997, Kudo and Ono investigated the catalytic activity of ZSM-5 as the first zeolites used for the partial oxidation of methane (Kudo and Ono, 1997). The maximum selectivity for methanol was not more than 10% and the major product of the catalysis was carbon dioxide with a selectivity of more than 80% at 0.01 bar methane partial pressure and 600°C–700°C after 1 h. Afterwards, over the last decade, copper-exchanged zeolites are the ones that have been more extensively studied (Zhu et al., 2020; Yu et al., 2021). In this case, and although better results have been obtained in terms of conversion and selectivity (more than 90% in both parameters), a major issue is the use of high temperatures up to 600°C (Michalkiewicz, 2004).
Methane to methanol
It is important to mention that, in this reaction, the use of nanomaterials has been widely reported without using MOF as support material. In this sense, zeolite and graphene have been the most widely used supports, apart from MOF (Lewis et al., 2019; Wang et al., 2022). With these supports, some excellent results in terms of conversion and selectivity have been obtained, although graphene and graphene oxide are the most promising ones (Impeng et al., 2014; Impeng et al., 2015; Shan et al., 2017; Sahoo et al., 2018; Yuan et al., 2018; Chang et al., 2020; Sirijaraensre and Limtrakul, 2022).
The hypothesis on which these results rely is the fact that these supports can mimic methane monooxygenases, which is also the case of MOF. Methane monooxygenases from methanotrophic bacteria convert methane to methanol under ambient conditions due to their ability to control the transport of oxygen and methane to the active site. In fact, hydrophobic cavities in methane monooxygenases act as an access gate to oxygen and methane in their way to the active site via a hydrophobic passage. Then, the activation of the oxygen in the metal centre of the monooxygenase proteins leads to the formation of an oxidative intermediate being able to perform the cleavage of the strong C-H bonds of methane (Sirajuddin and Rosenzweig, 2015). When these enzymes rearrange their conformation, cavities dissociate from each other resulting in the blockage of the hydrophobic passage and consequently restricting back diffusion and overoxidation of methanol while simultaneously opening separated hydrophilic pores for methanol to be released. This biological system has an extraordinary high selectivity to methanol and permit the control of mass transfer to and from the active sites. Therefore, it can be concluded that the presence of a hydrophobic cavity in the proximity of catalytic sites could lead to a higher affinity towards methane than methanol (Ikbal et al., 2019).
In Table 2, a summary of some representative studies recently reported with the combined use of NP and MOF for the conversion of methane into methanol is presented. Table 2 is not an exhaustive compilation of the totality of papers published on this topic, since each month new materials appear. However, it is useful for the reader to have a general perspective about the main materials used and their main challenges for a full implementation:
1) There is a wide diversity of materials used, both for NP and MOF. In the case of NP, it is important to note that noble metals can be substituted by low-cost metals such as Cu and Fe, an important issue when comparing with traditional catalysts. In the case of MOF, these are complex structures, which requires laborious synthesis protocols and a rigorous MOF characterization to have reproducible and reliable materials (Rogge et al., 2021).
2) Apart from the products coming from the overoxidation of methane, other side products can be obtained, such as ethanol or acid acetic (Xia et al., 2022), Obviously, the formation of these compounds decreases the selectivity towards methanol; however, they can also be considered products of interest.
3) Reactions conditions are variable, but generally in the mild range: temperature ranges between 50°C and 200°C and there are some works performed at atmospheric pressure. The maximum pressure is around 20 bar. This presumes an important economic issue, with some research focused on it. For instance, Hall and Bollini (2020) performed the reaction using tri-iron nodes in a MOF material at low temperatures (between 100°C–200°C) and even sub-ambient pressures. Room temperature has been also successfully checked with the help of photocatalysis (An et al., 2022).
4) MOF can catalyst the reaction without NP, which is an unexpected result that needs further research (Imyen et al., 2020). Apart from the obvious role of MOF as a support of NP to anchor the active site and to permit the diffusion of reactants and products, the conclusion is that a control with only MOF is necessary. However, no information about the mechanisms of this effect of MOF has been found in literature.
5) Mechanisms are not often clear, but the hypothesis of hydrophobic cavities are suggested in some studies.
In summary, catalysts based on NP and MOF seem to be a very promising option when compared to traditional ones in terms of conversion and selectivity. However, all the studies consulted are carried out at lab scale, with some milligrams of MOF (Table 2). It is mandatory to scale-up the synthesis of MOF. Another important point that is not often considered is the long-term operation of these novel catalysts and their reuse, two critical points that also needs further research.
Carbon dioxide to methanol
In this case, the number of studies is more limited. It is evident that, thermodynamically, this reaction is not favourable unless high pressures of hydrogen are used. Moreover, in kinetic terms, the possibilities of other secondary reactions is highly-dependent on the conditions used. In Table 3, some works reported for the conversion of carbon dioxide into methanol with the combined use of NP and MOF are presented. Again, the main conclusions that can be extracted from Table 3 are:
1) In general, the number of works published is much lower than those related to conversion to methane to methanol, which implies that the diversity of materials used for both NP and MOF synthesis in relatively scarce. Among them, Pt, Cu, and TiO2 are the main NP used, whereas most of the MOF are based on Zr.
2) There are some important variations of the catalyst process. For instance, not all the studies are carried out in gas phase, having aqueous solution and dissolved products a relative presence. Moreover, photocatalytic processes (visible light) are practically the half of the recent studies.
3) Efficiency is typically high but selectivity presents a feature that is worthy to mention. In some works, selectivity does not reach values higher than 90%, but the cause is that other products of interest apart from methanol are also the objectives of the study. This is the case of formic acid, formaldehyde, ethanol, and methane, among others in less proportion.
4) Contrarily to the conversion of methane to methanol, there are scarce references on the mechanisms of these reactions, especially in the case of gas-phase conditions. For instance, Duma et al. (2022) reports the results using copper-zinc bimetallic catalysts supported on a Zirconium-based MOF, where using advanced techniques they revealed the presence of copper active sites after impregnation and thermal activation. In general, research is clearly focused on having highly active sites to destabilize the molecules of carbon dioxide and hydrogen, where Ni, Co, Ru, Rh, and Pd are the most used materials (Cheng et al., 2021). To my knowledge, the most complete mechanism to date for the conversion of carbon dioxide to methanol/ethanol mixtures is presented by An et al. (2019) using Cu NP on a zirconium-based MOF. In this case, a complete mechanism is presented: the catalytic cycle is likely to proceed via bimetallic oxidative addition to activate hydrogen followed by hydrogenating carbon dioxide to methanol, and then coupling of methanol and formyl species to form C2 oxygenates. In other different conditions, MOF can be employed for the electrochemical reduction of carbon dioxide to methanol, where redox electrocatalysts is used to lower the kinetic barriers and give the higher reaction efficiency. In this case, MOF are hybrid materials that are made up of three components: a metallic component, pore space and the organic linker, which are the sites attributed to the catalytic activity of the MOF (Fayez Nasir et al., 2018).
5) Working conditions are mild. Temperature from 80°C to 200°C are reported, whereas pressure is around 5–10 bars.
To sum up, the use of NP and MOF for the conversion of carbon dioxide to methanol is still in an embryonic stage of research, and some questions about mechanisms and production of several compounds needs to be assessed. Again, most of the studies reported are carried out at lab scale or with small quantity of catalyst (Table 2) and the process scale-up is an important challenge to develop this technology. Long-term operation and reuse should be also considered in new publications, as they are very scarce in literature. Very recently, Lu et al. (2022) have used the term “reusable MOF” in scientific literature. Although it is not applied for the reactions discussed in this study, they appear as a very flexible and low-cost material for a lot of organic reactions.
A critical issue that can be applied to both reactions is the absence of economic data of the catalysts cost and its potential benefits to transform biogas into methanol. This is especially important as it hampers the implementation of this strategy at full-scale. Recently, some preliminary works have presented some techniques to synthetize these catalyst in a low-cost protocol (Duan et al., 2020; Ghorbani-Choghamarani et al., 2021; Kumari et al., 2021), but it is evident that other essential data are still unavailable to present a preliminary economic assessment.
Conclusion
In the current situation of energy scarcity and availability, it is evident that biogas from the anaerobic digestion of organic waste will have a key role in the development of easily and locally available low-impact renewable energy sources. Biogas can be used directly in cogeneration units, upgraded to methane and injected in the existing natural gas network or, taking a step forwards on that point, converted into methanol. Using this strategy, several problems of methane are overcome, but the need of effective and selective catalysts for all the chemical reactions involved is a critical issue. In this sense, nanomaterials embedded in metal organic frameworks provide very satisfactory results. It is evident that this field needs further research, but the results are promising. On the things-to-do side, the main challenges for the future development of this technology is its full-scale implementation and a complete economic assessment, which considers both the catalysts cost and the benefits of the conversion of biogas into methanol.
Author contributions
All authors listed have made a substantial, direct, and intellectual contribution to the work and approved it for publication.
Funding
This research was funded by Fundación Ramón Areces, in the hallmark of the META2NOL project granted in the XIX national call of life and matter sciences projects, grant number CIVP19A5952.
Conflict of interest
The authors declare that the research was conducted in the absence of any commercial or financial relationships that could be construed as a potential conflict of interest.
Publisher’s note
All claims expressed in this article are solely those of the authors and do not necessarily represent those of their affiliated organizations, or those of the publisher, the editors and the reviewers. Any product that may be evaluated in this article, or claim that may be made by its manufacturer, is not guaranteed or endorsed by the publisher.
References
Aadil, B., Lee, J., Younis, S. A., and Kim, K.-H. (2022). Recent advances in photocatalytic reduction of CO2 by TiO2– and MOF–based nanocomposites impregnated with metal nanoparticles. Mat. Today Chem. 24, 100870. doi:10.1016/j.mtchem.2022.100870
Alonso, A., Moral-Vico, J., Abo Markeb, A., Busquets-Fité, M., Komilis, D., Puntes, V., et al. (2017). Critical review of existing nanomaterial adsorbents to capture carbon dioxide and methane. Sci. Total Environ. 595, 51–62. doi:10.1016/j.scitotenv.2017.03.229
Alqarni, D. S., Marshall, M., Gengenbach, T. R., Lippi, R., and Chaffee, A. L. (2022). Rh/ZrO2@C(MIL) catalytic activity and TEM images. CO2 conversion performance and structural systematic evaluation of novel catalysts derived from Zr-MOF metallated with Ru, Rh, Pd or in. Microporous Mesoporous Mat. 336, 111855. doi:10.1016/j.micromeso.2022.111855
Álvarez, A., Bansode, A., Urakawa, A., Bavykima, A. V., Wezendonk, T. A., Makkee, M., et al. (2017). Challenges in the greener production of formates/formic acid, methanol and DME by heterogeneously catalyzed CO2 hydrogenation processes. Chem. Rev. 117, 9804–9838. doi:10.1021/acs.chemrev.6b00816
An, B., Li, Z., Song, Y., Zhang, J., Zeng, L., Wang, C., et al. (2019). Cooperative copper centres in a metal–organic framework for selective conversion of CO2 to ethanol. Nat. Catal. 2, 709–717. doi:10.1038/s41929-019-0308-5
An, B., Li, Z., Wang, Z., Zeng, X., Han, X., Cheng, Y., et al. (2022). Author Correction: Direct photo-oxidation of methane to methanol over a mono-iron hydroxyl site. Nat. Mat. 21, 959–938. doi:10.1038/s41563-022-01328-9
An, B., Zhang, J., Cheng, K., Ji, P., Wang, C., and Lin, W. (2017). Confinement of ultrasmall Cu/ZnOx nanoparticles in metal-organic frameworks for selective methanol synthesis from catalytic hydrogenation of CO2. J. Am. Chem. Soc. 139, 3834–3840. doi:10.1021/jacs.7b00058
Andreides, M., Pokorná-Krayzelová, L., Ambrožová, R., Volcke, E. I. P., and Bartáček, J. (2021). Key parameters influencing hydrogen sulfide removal in microaerobic sequencing batch reactor. Biochem. Eng. J. 168, 107951. doi:10.1016/j.bej.2021.107951
Baek, J., Rungtaweevoranit, B., Pei, X., Park, M., Fakra, S. C., Liu, Y-S., et al. (2018). Bioinspired metal-organic framework catalysts for selective methane oxidation to methanol. J. Am. Chem. Soc. 140, 18208–18216. doi:10.1021/jacs.8b11525
Bahraminia, S., Anbia, M., and Koohsaryan, E. (2020). Hydrogen sulfide removal from biogas using ion-exchanged nanostructured NaA zeolite for fueling solid oxide fuel cells. Int. J. Hydrogen Energy 45, 31027–31040. doi:10.1016/j.ijhydene.2020.08.091
Barrena, R., Moral-Vico, J., Font, X., and Sánchez, A. (2022). Enhancement of anaerobic digestion with nanomaterials: A mini review. Energies 15, 5087. doi:10.3390/en15145087
Cardoso, J. C., Stulp, S., de Brito, J. F., Flor, J. B. S., Frem, R. C. G., and Zanoni, M. V. B. (2018). MOFs based on ZIF-8 deposited on TiO2 nanotubes increase the surface adsorption of CO2 and its photoelectrocatalytic reduction to alcohols in aqueous media. Appl. Catal. B Environ. 225, 563–573. doi:10.1016/j.apcatb.2017.12.013
Carrasco-García, A. C., Moral-Vico, J., Abo Markeb, A., and Sánchez, A. (2022). Conversion of carbon dioxide into methanol using Cu–Zn nanostructured materials as catalysts. Nanomaterials 12, 999. doi:10.3390/nano12060999
Cerrillo, M., Burgos, L., Ruiz, B., Barrena, R., Moral-Vico, J., Font, X., et al. (2021). In-situ methane enrichment in continuous anaerobic digestion of pig slurry by zero-valent iron nanoparticles addition under mesophilic and thermophilic conditions. Renew. Energy 180, 372–382. doi:10.1016/j.renene.2021.08.072
Chang, C. C., Liu, C. Y., and Sun, Y. C. (2020). Effective methane conversion to methanol on bi-functional graphene-oxide-supported platinum nanoclusters (Pt5)-a DFT study. Phys. Chem. Chem. Phys. 22, 4967–4973. doi:10.1039/c9cp06002b
Cheng, Z., Yong-yong, N., Fei, Z., Hai-feng, T., Xiao-hua, T., and Yue, C. (2021). Application of metal-organic frameworks in CO2 hydrogenation. J. Fuel Chem. Technol. 49, 1444–1457. doi:10.1016/S1872-5813(21)60097-X
Choi, S., Drese, J. H., and Jones, C. W. (2009). Adsorbent materials for carbon dioxide capture from large anthropogenic point sources. ChemSusChem 9, 796–854. doi:10.1002/cssc.200900036
Collins, S. E., Delgado, J. J., Mira, C., Calvino, J. J., Bernal, S., Chiavassa, D. L., et al. (2012). The role of Pd-Ga bimetallic particles in the bifunctional mechanism of selective methanol synthesis via CO2 hydrogenation on a Pd/Ga2O3 catalyst. J. Catal. 292, 90–98. doi:10.1016/j.jcat.2012.05.005
Colón, J., Cadena, E., Pognani, M., Barrena, R., Sánchez, A., Font, X., et al. (2012). Determination of the energy and environmental burdens associated with the biological treatment of source-separated Municipal Solid Wastes. Energy Environ. Sci. 5, 5731–5741. doi:10.1039/C2EE01085B
Das, J., Nolan, S., and Lensa, P. N. L. (2022). Simultaneous removal of H2S and NH3 from raw biogas in hollow fibre membrane bioreactors. Environ. Technol. Innov. 28, 102777. doi:10.1016/j.eti.2022.102777
Díaz, I., Lopes, A. C., Pérez, S. I., and Fdz-Polanco, M. (2010). Performance evaluation of oxygen, air and nitrate for the microaerobic removal of hydrogen sulphide in biogas from sludge digestion. Bioresour. Technol. 101, 7724–7730. doi:10.1016/j.biortech.2010.04.062
Duan, M. B., Jiang, L. B., Zeng, G. M., Wang, D. B., Tang, W. W., Liang, J., et al. (2020). Bimetallic nanoparticles/metal-organic frameworks: Synthesis, applications and challenges. Appl. Mat. Today 19, 100564. doi:10.1016/j.apmt.2020.100564
Duma, Z. G., Dyosiba, X., Moma, J., Langmi, H. W., Louis, B., Parkhomenko, K., et al. (2022). Thermocatalytic hydrogenation of CO2 to methanol using Cu-ZnO bimetallic catalysts supported on metal–organic frameworks. Catalysts 12, 401. doi:10.3390/catal12040401
Durán, N., Álvarez-Gutiérrez, F., and Pevida, R. C. (2018). Biogas purification by means of adsorption on pine sawdust-based activated carbon: Impact of water vapour. Chem. Eng. J. 353, 197–207. doi:10.1016/j.cej.2018.07.100
Ertl, G., Knözinger, H., and Weitkamp, J. (2008). Handbook of heterogeneous catalysis. Weinheim (Germany): Wiley-VCH GmbH. Volumes 1-5.
Fayez Nasir, A-R., Jamal, A., Ba Shammakh, M. S., and Rana, A. (2018). A review on recent advances for electrochemical reduction of carbon dioxide to methanol using metal–organic framework (MOF) and non-MOF catalysts: Challenges and future prospects. ACS Sustain. Chem. Eng. 6, 15895–15914. doi:10.1021/acssuschemeng.8b03843
Gasquet, V., Kim, B., Bonhomme, A., and Benbelkacem, H. (2021). Sewage sludge ash-derived materials for H2S removal from a landfill biogas. Waste Manag. 136, 230–237. doi:10.1016/j.wasman.2021.10.023
Ghorbani-Choghamarani, A., Taherinia, Z., and Mohammadi, M. (2021). Facile synthesis of Fe3O4@GlcA@Ni-MOF composites as environmentally green catalyst in organic reactions. Environ. Technol. Innov. 24, 102050. doi:10.1016/j.eti.2021.102050
Gutterød, E. S., Lazzarini, A., Fjermestad, T., Kaur, G., Manzoli, M., Bordiga, S., et al. (2020). Hydrogenation of CO2 to methanol by Pt nanoparticles encapsulated in UiO-67: Deciphering the role of the metal-organic framework. J. Am. Chem. Soc. 142, 999–1009. doi:10.1021/jacs.9b10873
Hall, J. N., and Bollini, P. (2020). Low‐temperature, ambient pressure oxidation of methane to methanol over every tri‐iron node in a metal–organic framework material. Chem. Eur. J. 26, 16639–16643. doi:10.1002/chem.202003894
Hammond, C., Forde, M. M., Ab Rahim, M. H., Thetford, A., He, Q., Jenkins, R. L., et al. (2012). Direct catalytic conversion of methane to methanol in an aqueous medium by using copper-promoted Fe-ZSM-5. Angew. Chem. Int. Ed. 51, 5129–5133. doi:10.1002/anie.201108706
Han, X., Li, M., Chang, X., Hao, Z., Chen, J., Pan, Y., et al. (2022). Hollow structured Cu@ZrO2 derived from Zr-MOF for selective hydrogenation of CO2 to methanol. J. Energy Chem. 71, 277–287. doi:10.1016/j.jechem.2022.03.034
Herout, M., Malaťák, J., Kučera, L., and Dlabaja, T. (2011). Biogas composition depending on the type of plant biomass used. Res. Agric. Eng. 57, 137–143. doi:10.17221/41/2010-RAE
Ikbal, S. A., Colomban, C., Zhang, D., Delecluse, M., Brotin, T., Dufaud, V., et al. (2019). Bioinspired oxidation of methane in the confined spaces of molecular cages. Inorg. Chem. 58, 7220–7228. doi:10.1021/acs.inorgchem.9b00199
Ikuno, T., Zheng, J., Vjunov, A., Sanchez-Sanchez, M., Ortuño, M. A., Pahls, D. R., et al. (2017). Methane oxidation to methanol catalyzed by Cu-oxo clusters stabilized in NU-1000 metal-organic framework. J. Am. Chem. Soc. 139, 10294–10301. doi:10.1021/jacs.7b02936
Impeng, S., Khongpracha, P., Sirijaraensre, J., Jansang, B., Ehara, M., and Limtrakul, J. (2015). Methane activation on Fe- and FeO-embedded graphene and boron nitride sheet: Role of atomic defects in catalytic activities. RSC Adv. 5, 97918–97927. doi:10.1039/c5ra17984j
Impeng, S., Khongpracha, P., Warakulwit, C., Jansang, B., Sirijaraensre, J., Eharad, M., et al. (2014). Direct oxidation of methane to methanol on Fe-O modified graphene. RSC Adv. 4, 12572–12578. doi:10.1039/c3ra47826b
Imyen, T., Znoutine, E., Suttipat, D., Iadrat, P., Kidkhunthod, P., Bureekaew, S., et al. (2020). Methane utilization to methanol by a hybrid Zeolite@Metal-organic framework. ACS Appl. Mat. Interfaces 12, 23812–23821. doi:10.1021/acsami.0c02273
Javed, R., Zia, M., Naz, S., Aisida, S. O., Ain, N., and Ao, Q. (2020). Role of capping agents in the application of nanoparticles in biomedicine and environmental remediation: Recent trends and future prospects. J. Nanobiotechnol. 18, 172. doi:10.1186/s12951-020-00704-4
Khirsariya, P., and Mewada, R. K. (2013). Single step oxidation of methane to methanol–towards better understanding. Procedia Eng. 51, 409–415. doi:10.1016/j.proeng.2013.01.057
Komilis, D., Barrena, R., Lora Grando, R., Vogiatzi, V., Sánchez, A., and Font, X. (2017). A state of the art literature review on anaerobic digestion of food waste: Influential operating parameters on methane yield. Rev. Environ. Sci. Biotechnol. 16, 347–360. doi:10.1007/s11157-017-9428-z
Kudo, H., and Ono, T. (1997). Partial oxidation of CH4 over ZSM-5 catalysts. Appl. Surf. Sci. 121, 413–416. doi:10.1016/S0169-4332(97)00348-6
Kumar Gupta, N., Bae, J., and Soo Kim, K. (2022). Metal-organic framework-derived NaMnxOy hexagonal microsheets for superior adsorptive-oxidative removal of hydrogen sulfide in ambient conditions. Chem. Eng. J. 427, 130909. doi:10.1016/j.cej.2021.130909
Kumari, A., Kaushal, S., and Singh, P. P. (2021). Bimetallic metal organic frameworks heterogeneous catalysts: Design, construction, and applications. Mat. Today Energy 20, 100667. doi:10.1016/j.mtener.2021.100667
Kundu, S., Zanganeh, J., and Moghtaderi, B. (2016). A review on understanding explosions from methane-air mixture. J. Loss Prev. Process Ind. 40, 507–523. doi:10.1016/j.jlp.2016.02.004
Lewis, R. J., Bara-Estaun, A., Agarwal, N., Freakley, S. J., Morgan, D. J., and Hutchings, G. J. (2019). The direct synthesis of H2O2 and selective oxidation of methane to methanol using HZSM-5 supported AuPd catalysts. Catal. Lett. 149, 3066–3075. doi:10.1007/s10562-019-02876-7
Li, M. M. J., and Tsang, S. C. E. (2018). Bimetallic catalysts for green methanol production via CO2 and renewable hydrogen: A mini-review and prospects. Catal. Sci. Technol. 8, 3450–3464. doi:10.1039/C8CY00304A
López, L. R., Dorado, A. D., Mora, M., Gamisans, X., Lafuente, J., and Gabriel, D. (2016). Modeling an aerobic biotrickling filter for biogas desulfurization through a multi-step oxidation mechanism. Chem. Eng. J. 294, 447–457. doi:10.1016/j.cej.2016.03.013
Lora Granado, R., Souza Antune, A. D., Valéria da Fonseca, F., Sánchez, A., Barrena, R., and Font, X. (2017). Technology overview of biogas production in anaerobic digestion plants: A European evaluation of research and development. Renew. Sustain. Energy Rev. 80, 44–53. doi:10.1016/j.rser.2017.05.079
Lu, Y., Chai, H., Yu, K., Huang, C., Li, Y., Wang, J., et al. (2022). A reusable MOF supported single-site nickel-catalyzed direct N-alkylation of anilines with alcohols Tetrahedron, 132993. doi:10.1016/j.tet.2022.132993
Magnone, E., Kim, S. D., and Park, J. H. (2018). A systematic study of the iron hydroxide-based adsorbent for removal of hydrogen sulphide from biogas. Microporous Mesoporous Mat. 270, 155–160. doi:10.1016/j.micromeso.2018.05.018
Maina, J. W., Schütz, J. A., Grundy, L., Des Ligneris, E., Yi, Z., Kong, L., et al. (2017). Inorganic nanoparticles/metal organic framework hybrid membrane reactors for efficient photocatalytic conversion of CO2. ACS Appl. Mat. Interfaces 9, 35010–35017. doi:10.1021/acsami.7b11150
Mangal, S., Priya, S. S., Lewis, N. L., and Jonnalagadda, S. (2016). Synthesis and characterization of metal organic framework-based photocatalyst and membrane for carbon dioxide conversion. Mater. Today Proc. 5, 16378–16389. doi:10.1016/j.matpr.2018.05.134
Michalkiewicz, B. (2004). Partial oxidation of methane to formaldehyde and methanol using molecular oxygen over Fe-ZSM-5. Appl. Catal. A General 277, 147–153. doi:10.1016/j.apcata.2004.09.005
Mondello, G., Salomone, R., Ioppolo, G., Saija, G., Sparacia, S., and Lucchetti, M. C. (2017). Comparative LCA of alternative scenarios for waste treatment: The case of food waste production by the mass-retail sector. Sustainability 9, 827. doi:10.3390/su9050827
Montebello, M., Fernández, M., Almenglo, F., Ramírez, M., Cantero, D., Baeza, M., et al. (2022). Simultaneous methylmercaptan and hydrogen sulfide removal in the desulfurization of biogas in aerobic and anoxic biotrickling filters. Chem. Eng. J. 200-202, 237–246. doi:10.1016/j.cej.2012.06.043
Nagababu, P., Prabhu, Y. T., Kularkar, A., Subbalakshmi, M. S., Nagarkar, J., and Rayalu, S. (2021). Manifestation of Cu-MOF-templated TiO2 nanocomposite for synergistic photoreduction of CO2 to methanol production. emergent Mat. 4, 503–514. doi:10.1007/s42247-021-00187-5
Okonkwo, C. N., Lee, J. J., De Vylder, A., Chiang, Y., Thybaut, J. V., and Jonesa, C. W. (2020). Selective removal of hydrogen sulfide from simulated biogas streams using sterically hindered amine adsorbents. Chem. Eng. J. 379, 122349. doi:10.1016/j.cej.2019.122349
Olsbye, U., Nova, A., Gutterød, E. S., Sri Harsha, P., Gurpreet, K., Andrea, L., et al. (2020). Influence of defects and H2O on the hydrogenation of CO2 to methanol over Pt nanoparticles in UiO-67 metal-organic framework. J. Am. Chem. Soc. 142, 17105–17118. doi:10.1021/jacs.0c07153
Osadchii, D. Y., Olivos-Suarez, A. I., Szécsényi, A., Li, G., Nasalevich, M. A., Dugulan, I. A., et al. (2018). Isolated Fe sites in metal organic frameworks catalyze the direct conversion of methane to methanol. ACS Catal. 8, 5542–5548. doi:10.1021/acscatal.8b00505
Pawelczyk, E., Łukasik, N., Wysocka, I., Rogala, A., and Gębicki, J. (2022). Recent progress on hydrogen storage and production using chemical hydrogen carriers. Energies 15, 4964. doi:10.3390/en15144964
Persson, T., Persson, K. M., and Åström, J. (2021). Ferric oxide-containing waterworks sludge reduces emissions of hydrogen sulfide in biogas plants and the needs for virgin chemicals. Sustainability 13, 7416. doi:10.3390/su13137416
Petersson, A., and Wellinger, A. (2018). Biogas upgrading technologies – developments and innovations. London: IEA Bioenergy. Task 37 - Energy from biogas and landfill gasURL:. Available at: https://www.ieabioenergy.com/wp-content/uploads/2009/10/upgrading_rz_low_final.pdf (accessed July, 2022).
Piechota, G. (2021). Removal of siloxanes from biogas upgraded to biomethane by cryogenic temperature condensation system. J. Clean. Prod. 308, 127404. doi:10.1016/j.jclepro.2021.127404
Rayder, T. M., Bensalah, A. T., Li, B., Byers, J. A., and Tsung, C.-K. (2021). Engineering second sphere interactions in a host-guest multicomponent catalyst system for the hydrogenation of carbon dioxide to methanol. J. Am. Chem. Soc. 143, 1630–1640. doi:10.1021/jacs.0c08957
Ren, M., Shib, Q., Mi, L., Liang, W., Yuan, M., Wang, L., et al. (2021). Isothermal conversion of methane to methanol over CuxOy@UiO-bpy. Mater. Today Sustain. 11-12, 100061–100112. doi:10.1016/j.mtsust.2021.100061
Rogge, S. M. J., Bavykina, A., Hajek, J., Garcia, H., Olivos-Suarez, A. I., Sepúlveda-Escribano, A., et al. (2021). Metal-organic and covalent organic frameworks as single-site catalysts. Chem. Soc. Rev. 46, 3134–3184. doi:10.1039/c7cs00033b
Saeidi, S., Najari, S., Hessel, V., Wilson, K., Keil, F. J., Concepción, P., et al. (2021). Recent advances in CO2 hydrogenation to value-added products-Current challenges and future directions. Prog. Energy Combust. Sci. 85, 100905. doi:10.1016/j.pecs.2021.100905
Sahoo, S., Suib, S. L., and Alpay, S. P. (2018). Graphene supported single atom transition metal catalysts for methane activation. ChemCatChem 10, 3229–3235. doi:10.1002/cctc.201800465
Shan, J., Li, M., Allard, L. F., Lee, S., and Flytzani-Stephanopoulos, M. (2017). Mild oxidation of methane to methanol or acetic acid on supported isolated rhodium catalysts. Nature 551, 605–608. doi:10.1038/nature24640
Sirajuddin, S., and Rosenzweig, A. C. (2015). Enzymatic oxidation of methane. Biochemistry 54, 2283–2294. doi:10.1021/acs.biochem.5b00198
Sirijaraensre, J., and Limtrakul, J. (2015). Modification of the catalytic properties of the Au4 nanocluster for the conversion of methane-to-methanol: Synergistic effects of metallic adatoms and a defective graphene support. Phys. Chem. Chem. Phys. 17, 9706–9715. doi:10.1039/c4cp05131a
Sołowski, G. (2022). Analysis of biogas component production during anaerobic digestion of sour cabbage in microaeration conditionsunder different pH conditions. Biomass 2, 14–26. doi:10.3390/biomass2010002
Studt, F., Sharafutdinov, I., Abild-Pedersen, F., Elkjaer, C. F., Hummelshøj, J. S., Dahl, S., et al. (2014). Discovery of a Ni-Ga catalyst for carbon dioxide reduction to methanol. Nat. Chem. 6, 320–324. doi:10.1038/nchem.1873
Su, J-J., and Hong, Y-Y. (2020). Removal of hydrogen sulfide using a photocatalytic livestock biogas desulfurizer. Renew. Energy 149, 181–188. doi:10.1016/j.renene.2019.12.068
Sushkevich, V. L., Palagin, D., Ranocchiari, M., and van Bokhoven, J. A. (2017). Selective anaerobic oxidation of methane enables direct synthesis of methanol. Science 356, 523–527. doi:10.1126/science.aam9035
Thanakunpaisit, N., Jantarachat, N., and Onthong, U. (2017). Removal of hydrogen sulfide from biogas using laterite materials as an adsorbent. Energy Procedia 138, 1134–1139. doi:10.1016/j.egypro.2017.10.215
Tomkins, P., Ranocchiari, M., and van Bokhoven, J. A. (2017). Direct conversion of methane to methanol under mild conditions over Cu-zeolites and beyond. Acc. Chem. Res. 50, 418–425. doi:10.1021/acs.accounts.6b00534
US Environmental Protection Agency (2005). Understanding global warming potentials. Available at: https://www.epa.gov/ghgemissions/understanding-global-warming-potentials (accessed July, 2022).
Vu, H. P., Nguyen, L. N., Wang, Q., Ngo, H. H., Liu, Q., Zhang, X., et al. (2022). Hydrogen sulphide management in anaerobic digestion: A critical review on input control, process regulation, and post-treatment. Bioresour. Technol. 346, 126634. doi:10.1016/j.biortech.2021.126634
Wachter, P., Gaber, C., Raic, J., Demuth, M., and Hochenauer, C. (2021). Experimental investigation on H2S and SO2 sulphur poisoning and regeneration of a commercially available Ni-catalyst during methane tri-reforming. Int. J. Hydrogen Energy 46, 3437–3452. doi:10.1016/j.ijhydene.2020.10.214
Wang, H., Larson, R. A., and Runge, T. (2019). Impacts to hydrogen sulfide concentrations in biogas when poplar wood chips, steam treated wood chips, and biochar are added to manure-based anaerobic digestion systems. Bioresour. Technol. Rep. 7, 100232. doi:10.1016/j.biteb.2019.100232
Wang, S., Xin, Y., Zhang, W., and Wang, L. (2022). Conversion of methane to methanol on cobalt-embedded graphene: A theoretical perspective. Catal. Lett. 152, 1331–1337. doi:10.1007/s10562-021-03742-1
Xia, M., Qiu, L., Li, Y., Shen, T., Sui, Z., Feng, L., et al. (2022). A metal-organic frameworks composite catalyst containing platinum and polyoxometalate for direct conversion of methane. Mat. Lett. 307, 131078. doi:10.1016/j.matlet.2021.131078
Xie, J., Jin, R., Li, A., Bi, Y., Ruan, Q., Deng, Y., et al. (2018). Highly selective oxidation of methane to methanol at ambient conditions by titanium dioxide-supported iron species. Nat. Catal. 1, 889–896. doi:10.1038/s41929-018-0170-x
Xu, G., Yu, A., Xu, Y., and Sun, C. (2021). Selective oxidation of methane to methanol using AuPd@ZIF-8. Catal. Commun. 158, 106338. doi:10.1016/j.catcom.2021.106338
Yang, L., and Corsolini, S. I. (2019). Online removal of volatile siloxanes in solid-state anaerobic digester biogas using a biofilter and an activated carbon filter. J. Environ. Chem. Eng. 7, 103284. doi:10.1016/j.jece.2019.103284
Yang, L., Huang, J., Ma, R., You, R., Zeng, H., and Rui, Z. (2019). Metal-organic framework-derived IrO2/CuO catalyst for selective oxidation of methane to methanol. ACS Energy Lett. 4, 2945–2951. doi:10.1021/acsenergylett.9b01992
Yu, T., Li, Z., Lin, L., Chu, S., Su, Y., Song, W., et al. (2021). Highly selective oxidation of methane into methanol over Cu-promoted monomeric Fe/ZSM-5. ACS Catal. 11, 6684–6691. doi:10.1021/acscatal.1c00905
Yuan Chen, X., Vinh-Thang, H., Avalos Ramirez, A., Rodriguez, D., and Kaliaguine, S. (2015). Membrane gas separation technologies for biogas upgrading. RSC Adv. 5, 24399–24448. doi:10.1039/C5RA00666J
Yuan, J., Zhang, W., Li, X., and Yang, J. (2018). A high performance catalyst for methane conversion to methanol: Graphene supported single atom Co. Chem. Commun. 54, 2284–2287. doi:10.1039/c7cc08713f
Zain, M. M., and Mohamed, A. R. (2018). An overview on conversion technologies to produce value added products from CH4 and CO2 as major biogas constituents. Renew. Sustain. Energy Rev. 98, 56–63. doi:10.1016/j.rser.2018.09.003
Zakaria, Z., and Kamarudin, S. K. (2016). Direct conversion technologies of methane to methanol: An overview. Renew. Sustain. Energy Rev. 65, 250–261. doi:10.1016/j.rser.2016.05.082
Zhang, M., Yuan, X-j., Zhang, C., Zhu, L-p., Mo, X-h., Chen, W-j., et al. (2019). Bioconversion of methanol into value-added chemicals in native and synthetic methylotrophs. Curr. Issues Mol. Biol. 33, 225–236. doi:10.21775/cimb.033.225
Zhao, F., Fan, L., Xu, K., Hua, D., Zhan, G., and Zhou, S. F. (2019). Hierarchical sheet-like Cu/Zn/Al nanocatalysts derived from LDH/MOF composites for CO2 hydrogenation to methanol. J. CO2 Util. 33, 222–232. doi:10.1016/j.jcou.2019.05.021
Keywords: biogas, metal organic framework, methanol, nanoparticles, carbon dioxide, carbon storage, energy storage
Citation: Sánchez A (2022) Biogas improvement as renewable energy through conversion into methanol: A perspective of new catalysts based on nanomaterials and metal organic frameworks. Front. Nanotechnol. 4:1012384. doi: 10.3389/fnano.2022.1012384
Received: 05 August 2022; Accepted: 26 September 2022;
Published: 11 October 2022.
Edited by:
Dr. Surender Kumar, Advanced Materials and Processes Research Institute (CSIR), IndiaReviewed by:
Dr. Swati Dubey, Samrat Ashok Technological Institute, IndiaViplov Chauhan, Jawahar Navodaya Vidyalaya, Aurangabad, India
Archana Charanpahari,India
Divyaratan Kumar, Linköping University, Sweden
Copyright © 2022 Sánchez. This is an open-access article distributed under the terms of the Creative Commons Attribution License (CC BY). The use, distribution or reproduction in other forums is permitted, provided the original author(s) and the copyright owner(s) are credited and that the original publication in this journal is cited, in accordance with accepted academic practice. No use, distribution or reproduction is permitted which does not comply with these terms.
*Correspondence: Antoni Sánchez, YW50b25pLnNhbmNoZXpAdWFiLmNhdA==